- Department of Civil and Environmental Engineering, University of Maryland, College Park, MD, United States
Polychlorinated biphenyls (PCBs) are one of the persistent organic pollutants (POPs) used worldwide between the 1930 and 1980s. Many PCBs can still be found in the environment such as in soils and sediments, even though their use has been heavily restricted. This review summarizes the most frequent remediation solutions including, phytoremediation, microbial degradation, dehalogenation by chemical reagent, and PCBs removal by activated carbon. New insights that emerged from recent studies of PCBs remediation including supercritical water oxidation, ultrasonic radiation, bimetallic systems, nanoscale zero-valent iron based reductive dehalogenation and biofilm covered activated carbon, electrokinetic remediation, and nZVI particles in combination with a second metal are overviewed. Some of these methods are still in the initial development stage thereby requiring further research attention. In addition, the advantages and disadvantages of each general treatment strategy and promising technology for PCBs remediation are discussed and compared. There is no well-developed single technology, although various possible technologies have been suggested. Therefore, the possibility of using combined technologies for PCB remediation is also here investigated. It is hoped that this present paper can provide a basic framework and a more profound prospect to select successful PCB remediation strategies or combined technologies.
Introduction
Polychlorinated biphenyls (PCBs) have been used for industrial purposes since 1929 (Alcock et al., 1994). The physical and chemical properties of PCBs allow them for a wide range of industrial applications. Their electrical insulating properties allow for their use with electrical equipment, such as in cooling, for instance (Merkel et al., 1999). PCBs are classified as persistent organic pollutants (POPs) with high toxicity, and have undesirable effects on the environment and on humans (Lallas, 2001). Once released into the environment PCBs they could bioaccumulate within the food chain, due to their high affinity for organic materials. They have been found in human's tissues, blood, and breast milk and are introduced via the consumption of meat, fish, and dairy products (Van den Berg et al., 2006). Consequentially, they have been linked to chronic effects in humans including immune system damage, decreased pulmonary function, bronchitis, and interferences with hormones leading to cancer (Schecter et al., 2006). Additionally, studies indicated that children will show serious developmental problems such as low birth-weight, behavioral disorders, and hearing loss at relatively high exposure to PCBs (more than 10 pg/kg body weight per day) (Urban et al., 2014). Literature also provides evidence of the effects of PCBs exposure to animals (e.g., rats) such as liver damage, immune system suppression, abnormalities in fetal development, enzyme induction, sarcomas, non-Hodgkin lymphomas, and serum lipids (Kogevinas, 2001; Goncharov et al., 2008).
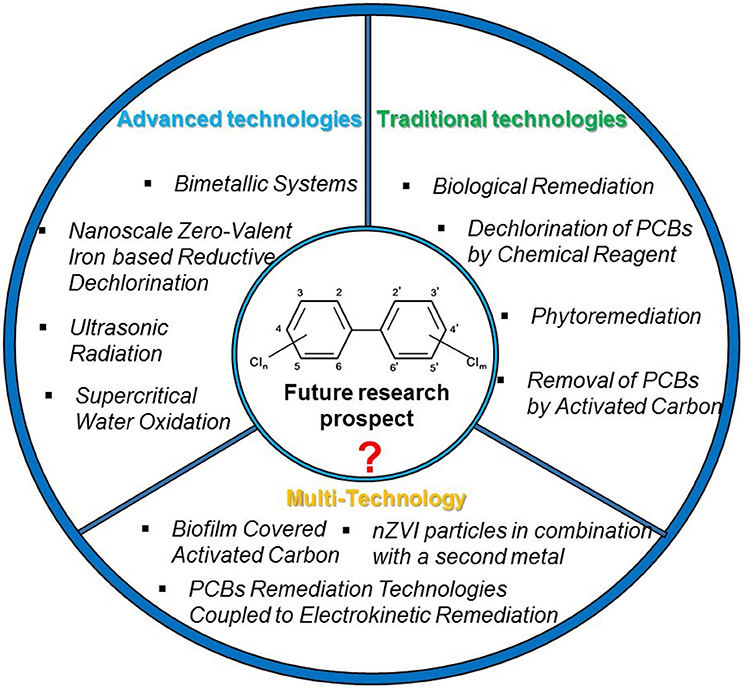
Graphical Abstract. Current and prospective technologies for remediation of polychlorinated biphenyls (PCBs) in the environment.
The PCB molecule consists of two connected benzene rings and chlorine atoms that can attach to any or all of 10 different positions allowing for 209 different congeners and 10 different homologs (McFarland and Clarke, 1989; Alder et al., 1993). High chlorinated PCB congeners typically have relatively high octanol-water partition coefficients (Kow) thus are often found in organic matter such as soils and sediments. Due to a low water solubility and vapor pressure, PCBs partition between the aquatic and solid phase thus exist in multiple compartments resulting in widespread contamination (Tanabe, 1988).
PCBs can be released into the atmosphere by incineration of PCB-containing waste, leaking from landfills with PCB containing products, and disposed industrial waste, among others (Van Gerven et al., 2004). In US, the most common remediation technology for PCB-contaminated soil or sediments are incineration or disposal in landfills (Gomes et al., 2013). Other strategies such as biological, chemical, physical and thermal methods are also widely applied in PCB remediation. However, most of these solutions are disruptive, unsustainable and transfer PCBs to different sections of the environment, rather than ridding them (Agarwal et al., 2007). Therefore, current research is being conducted to find sustainable, alternative remediation technologies for persistent organic pollutants. This review paper aims to: (1) critically evaluate the state of these promising technologies for PCB contaminated soils, sediments, and waters; (2) discuss the possibility of improving PCB remediation by simultaneously combing multiple treatment strategies; (3) explore future research prospects regarding PCB remediation on the basis of a comprehensive review of the current PCB remediation technologies discussed in this paper.
Traditional PCB Remediation Technologies
Phytoremediation of PCBs
Phytoremediation has been recognized as an ecologically responsible alternative for removing organic pollutants in soils, water and sediments (Meagher, 2000). The most precise of phytoremediation techniques is plant-mediated bioremediation (Macek et al., 2000). Phytoremediation processes can be summarized as two steps: the biodegradation of organic pollutants in soil or groundwater and uptake into plant tissues through their roots followed by transformation by plant enzymes or direct volatilization into the atmosphere (Harvey et al., 2002). Plant species used for PCB phytoremediation including Medicago sativa (alfalfa), Lespedeza cuneate (Chinese bushclover), Lathyrus sylvestris (everlasting pea), Phalaris arundinacea (reed canary grass), Cucurbitaceae (cucurbits), Sparganium (bur-reed), Salix alaxensis (Alaska willow), and Picea glauca (white spruce) as well as 27 different weeds (Chekol et al., 2004; Ficko et al., 2010; Slater et al., 2011). Tu et al. (2011) showed a decrease in PCB soil concentrations by 31.4 and 78.4% after the first and second years of field scale phytoremediation by M. sativa via situ phytoremediation. Sparganium has been shown to promote the oxidation of the low-chlorinated PCBs via rhizodegradation, while panicum virgatum (switchgrass) and other popularly used plants have shown degradation of a high and low congener mix (Meggo et al., 2013). Wyrwicka et al. (2014) found that Cucurbitaceae could be used to reduce PCBs concentration in sludge and sediment by 38.63 and 27.38% respectively.
Microorganisms play a significant role in PCB biodegradation during rhizoremediation process due to a relatively large Kow. Several studies demonstrated that a distributed network of plant roots can activate microbial processes i.e., “rhizodegradation” or “rhizoremediation,” which are capable of degradation of PCBs in contaminated soils (Passatore et al., 2014). For example, plant roots can release microbial growth factors and extracellular enzymes or organic acids which can be used as electron donors for anaerobic dehalogenation processes or facilitate microbial metabolism (Gerhardt et al., 2009).
Phytoremediation is a green (environmentally friendly) and low-tech remediation method with low environmental impacts. An ideal plant for phytoremediation should include the following characteristics: high biomass production, broad root distribution, and an ability to tolerate and accumulate contaminants (Gomes et al., 2013). Phytoremediation is a low cost method due to the absence of energy-consuming equipment (Pivetz, 2001). In addition, phytoremediation has little to no destructive impact on soil fertility and structure, while the introduction of plants can improve the overall condition of the soil due to the plant and mirobes introducing minerals and nutrients (Gerhardt et al., 2009). It should be noted that PCBs intaking by plants mainly attributed by uptake and translocation. However, plants are not only capable of PCB attenuation in soil and sediments, but also the capability of plants to metabolize PCBs. Some studies indicated that dichloro-, trichloro-, and tetrachlorobiphenyl congeners can be metabolized by plant cell cultures of Rosa spp. (Lee and Fletcher, 1992). More persistent 2,2′,5,5′-tetrachlorobiphenyl could be oxidized to 3,4-dihydroxy-2,2′,5,5′-tetrachlorobiphenyl by plant cell cultures of Rosa spp. (Harms et al., 2003). Other studies have indicated that the PCB concentration increased in the stems and leaves of pumpkins (Cucurbita sp.) in Aroclor contaminated soil (Åslund et al., 2008). However, the PCB concentration in the pumpkin roots was unchanged. These observations support that the mechanism of PCB transport in plants can mainly be attributed to uptake and translocation compared to volatilization and deposition (Aken et al., 2009). PCBs have high octanol-water partition coefficients (Kow) from 104.10 (20°C) for mono-chlorobiphenyl to 107.93 for deca-chlorobiphenyl influencing the mobility of PCBs in the environment (Zhang et al., 2013). Therefore, the higher-chlorinated PCB congeners with a high Kow (log Kow > 6) tend to be present in soils and sediments compared to lower-chlorinated PCB congeners. As a result, soils often contain a higher proportion of highly chlorinated PCB congeners. More importantly, most of the studies suggested that the metabolism of PCBs by plants is only limited to tetrachlorinated and lower congeners (Aken et al., 2009). Due to the high hydrophobicity of higher-chlorinated PCB congeners, these are less often taken up and transported inside of the plants thereby experiencing limited metabolism by plant tissues. Due to these mechanisms. High-chlorinated PCB congeners are usually more resistant to the metablism process than the lower chlorinated congeners in most of the cases. As a result, the high-chlorinated PCB congeners accumulate in biomass and tend to release to the environment, when the process of plant decomposition occurs after the plants are dead. However, phytoremediation also offers several limitations. One of the drawbacks is that PCB remediation generally implies long-term monitoring. One estimate was that an industrial site contaminated with a PCB mixture (trichlorobiphenyl and tetrachlorobiphenyl congeners) requires 20 years to be remediated (Kaštánek et al., 1999). More importantly, plants lack the biochemical pathway to achieve mineralization of pollutants. Another significant disadvantage is that phytoremediation systems may lose their effectiveness, when plant growth slows or stops due to extreme weather and other such factors (Pivetz, 2001). Furthermore, the effectiveness of phytoremediation systems can be restricted by plant depth due to the generally shallow distribution of plant roots or by the transfer of contaminants from plants to other ecosystems (Pivetz, 2001). Additionally, the introduction of noxious or invasive vegetation can bring negative impacts to animals and other plants in the ecosystem (Pivetz, 2001).
Microbial Degradation of PCBs
Microbial degradation or bioremediation is defined as a natural biological process that relies on microorganisms (e.g., bacteria, fungi) to degrade, break down, transform, and remove contaminants or hazardous materials (Vidali, 2001). Evidence for the microbial degradation of PCBs in natural environments such as soil, sediment, and surface water has been well reported in various studies (Bedard, 2008; Anyasi and Atagana, 2011). Microbial degradation of PCBs encompasses two possible pathways: anaerobic dehalogenation and aerobic degradation.
Microbial degradation of highly chlorinated PCB congeners is generally achieved by organohalide respiration under anaerobic conditions (Field and Sierra-Alvarez, 2008). Organohalide respiration of PCBs is a biological process that potentially decreases the toxicity of PCBs through the removal of chlorines (Häggblom et al., 2003). During this process the chlorine substituent is replaced with hydrogen. The PCB congeners serves as the terminal electron acceptor with three potential chlorine substituent positions; para, meta, and ortho. (Brown et al., 1987). Substitution of a chlorine with a hydrogen atom preferentially occurs at the first two sites. The potential pathway for anaerobic dehalogenation of a highly chlorinated PCB congener is illustrated in Figure 1, using 2,3,4,5,6-Pentachlorobiphenyl (PCB-116) as an illustrated example (Van Dort et al., 1997). Here, the highly chlorinated PCB is transformed to a lighter chlorinated congener (Abramowicz and Olson, 1995). The first case study of anaerobic dehalogenation of PCBs was reported by Brown et al. (1987). Since then, studies have validated the potential of anaerobic organohalide respiration in marine sediments (Quensen III et al., 1988).
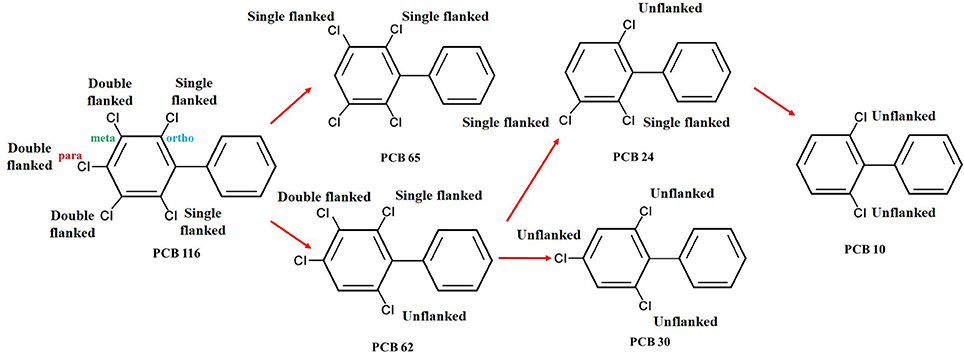
Figure 1. Possible dechlorination pathway of 2,3,4,5,6-Pentachlorobiphenyl and the distribution of products (Freely after Van Dort et al., 1997).
Over the past decade in-situ bioremediation technologies which can be applied to treatment of PCB impacted soils and sediments in the field have been conducted (Tyagi et al., 2011; Sowers and May, 2013). Two major types of bioremediation techniques include biostimulation and bioaugmentation. Some studies indicated that biostimulation by halopriming with halogenated aromatic compounds can increase the dehalogenating microbial catalysts of indigenous PCB dechlorinating bacteria and induce genes required for dehalogenation (Sowers and May, 2013). Biostimulation is a process to add the nutrients or substrates to a contamination site to stimulate the activities of autochthonous microorganisms (Azubuike et al., 2016). For the cases of anaerobic degradation of PCBs, biostimulation of PCB indigenous dechlorinating bacteria can be achieved by halopriming with halogenated aromatic compounds such as halogenated benzoates (Sowers and May, 2013). The chlorine atoms of PCB congener are replaced with hydrogen during this process. Over the past decade the biostimulation of anaerobic PCB degradation was widely applied to treatment of PCB impacted soils and sediments, however, the precise mechanism is still unclear. On the other hand, biostimulation can also apply to aerobic bioremediation that the microorganisms can use oxygen to breakdown the low-chlorine content PCBs. Biphenyl is the primary substrate that can support co-metabolism of PCBs (Sharma et al., 2018). During the aerobic degradation, the benzene ring with less chlorines of the PCB molecular is destructed. This process involves several genes which are mainly bph gene clusters i.e., bphA (dioxygenation of the biphenyl ring), bphB (dehydrogenase), bphC (ring cleavage dioxygenase). The bphA gene is involved in dioxygenation of the biphenyl ring with the formation of dihydrodiol. The bphB gene is involved the oxidation of biphenyl-2,3-dihydrodiol to 2,3-dihydroxybiphenyl. The bphC gene is responsible for biphenyl ring cleavage to generate phenylcatechol. An earlier example is the stimulation of Aroclor 1260 in sediment slurries which demonstrated that the addition of bromated biphenyl congeners (PBBs) achieve more effective stimulation leading to complete dehalogenation. Other haloprimers such as tetrachlorobenzene (TeCB), pentachloronitrobenzene (PCNB), tetrabromobisphenol A (TBBPA) have resulted in an increase of native PCB dechlorinating bacteria such as Dehalococcoides sp., Ochrobactrum sp., Parasegetibacter sp., Thermithiobacillus sp., Phenylobacterium sp., and Sphingomonas sp. (Krumins et al., 2009; Park et al., 2011; Li et al., 2016). Biostimulation has also been achieved by using electrochemical techniques to treat the PCB contaminated groundwater or sediment (Chun et al., 2013; Yu et al., 2016). Voltage was applied to the contaminated sediments from a superfund site (Fox River) to stimulate the oxidative and reductive transformation of Aroclor 1242 with an overall concentration reduction of 40–60% (Chun et al., 2013). In Guangdong, China, an application of bioanode stimulation resulted in dehalogenation of 2,3,4,5-tetrachlorobiphenyl in an electronic waste recycling site by 42% after 110 days of incubation (Yu et al., 2016).
Bioaugmentation, a feasible, in-situ, PCB transformation pathway, is defined as the addition of bacterial cultures to accelerate the degradation rate of a contaminant (Tyagi et al., 2011). May et al. (2008) reported that the isolated ultramicrobacterium from sediment is capable of dechlorinating Aroclor 1260, containing double-flanked chlorines, when they were added to the contaminated soil (May et al., 2008). Similarly, Payne et al. (2011) found that Dehalobium chlorocoercia DF1 could enhance the dehalogenation of weathered Aroclor 1260, containing double and single flanked chlorines (Payne et al., 2011). The total concentration of pentapolychlorinated biphenyls in mesocosm experiments decreased by 56% after bioaugmentation with DF1 for 120 days. More recently, biofilm covered activated carbon particles as a delivery system for bioaugmentation to enhance PCB dehalogenation have been studied. For example, Kjellerup and Edwards (2013) showed that the dehalogenation of Aroclor 1248 was enhanced in a mesocosm study with sediment. These studies provide convincing evidence that biofilm covered activated carbon as an in-situ treatment of weathered PCBs exhibits a number of advantages. Therefore, this is will be discussed in more detail as an independent technology for PCB remediation in the following section. The major environmental condition that could affect the efficiency of bioaugmentation technique is different phases. When PCBs release into the environments, the PCB distribution among different phases such as solid phase and aqueous phase is driven by their partition coefficient. The transport and partitioning behaviors of the 10 different PCB homologs vary with their structural differences, which represent by different octanol-water partition coefficients (Kow) indicating the fugacity of PCBs in the environment, from 104.10 (20°C) for monochlorobiphenyl to 107.93 (20°C) for decachlorobiphenyl (Zhang et al., 2013). As a result, the concentration of PCBs in different phases could significant vary. The average air concentrations of PCBs may range from 120 to 170 pg/m3, however, the average concentration of PCBs in background soil could be from 100 to 1,000 pg/g (Batterman et al., 2009). One example could be monochlorobiphenyl which is more likely to accumulate to air phase based on its aqueous solubility, vapor pressure and log Kow. Bioaugmentation is mainly applied in sediment, soil, and solid phases. Therefore, it is expect that the use of bioaugmentation approaches may considerably limited by the environmental fate of monochlorobiphenyl. In addition, the distribution of high-chlorine content PCB congeners in different phases also highly depend on their partitioning coefficients. Some highly chlorinated PCB congeners sharing similar chemical properties such as the similar vapor pressure for both PCB-77 and PCB-153 (4.4 × 10−7 mm Hg at 25°C and 3.80 × 10−7 mm Hg at 25°C) (Mackay et al., 1992; Erickson, 1997). However, it does not mean they have the similar chemical fate. The logKow of the PCB-153 (8.35 at 25°C) is two orders of magnitude higher than that of PCB-77 (6.04–6.63 at 25°C) resulting a significantly different distribution in soil or water phase (Dunnivant et al., 1992). As a result, the bioavailability of PCB-153 is significantly higher than that of the PCB-77 in soil phase resulting in a high removal efficiency of bioaugmentation.
Lightly chlorinated PCB congeners, with three or less chlorine atoms per molecule, could be biodegraded by aerobic bacteria (Borja et al., 2005). Two different clusters of genes are involved during this aerobic, oxidative, destruction process: one is responsible for converting the PCB congeners to chlorobenzoic acid, the other for the degradation of the chlorobenzoic acid (bphA) through cometabolic aerobic oxidation (Borja et al., 2005). Many bacterial strains are capable of oxidative degradation of PCBs such as Pseudomonas, Burkholderia, Comamonas, Rhodococcus and Bacilus (Anyasi and Atagana, 2011). The common by-product of biphenyl degradation is chlorobenzoic acid through 1,2-dioxygenative ring cleavage. Monochlorobiphenyl, for example, can serve as substrates for bacteria growth, because the biphenyl can be used as a carbon and an energy source when PCB congeners degrade by a cometabolic process (Anyasi and Atagana, 2011). Some biphenyl-utilizing bacteria such as Burkholderia sp. LB400 can convert PCBs into chlorobenzoic acids using biphenyl dioxygenase (bphA) through cometabolic aerobic oxidation (Furukawa et al., 2004). Other bacterial species can further breakdown chlorobenzoic acid into less toxic compounds (Bianucci et al., 2004). This has been observed in many studies regarding Pseudomonas sp. and Micrococcus sp. (Bevinakatti and Ninnekar, 1922; Boyle et al., 1992).
The aerobic and anaerobic bacterial biodegradation of PCBs has been well studied and successfully used. Microbial degradation has relatively low technical barriers as compared with the promising remediation technologies for PCBs. As mentioned previously, the residues for microbial degradation of PCBs are generally less-toxic products and cell biomass. Highly chlorinated molecules can be degraded through the pathway of reductive dehalogenation resulting in 2–3 chlorine congeners as the major metabolites. In addition, microbial degradation of PCBs does not disrupt normal activities. This eliminates the waste transported off site thereby reducing the potential threats to human health and the environment. It is worth noting that microbial degradation has a smaller environmental footprint than incineration of PCB contaminated soils. The results of a life cycle assessment indicated that the impact on global warming was nine times less than that of incineration producing 6.5 × 105 kg CO2-eq and 7.2 × 104 kg CO2-eq, respectively (Busset et al., 2012). However, microbial degradation of PCBs is also considered problematic, even though it is used worldwide (Kumar et al., 2011). The anaerobic dechloronation of PCBs is a long-term, labor-intensive process with efficiencies specific and limited to target PCB and the bacterial species. Not all PCB congeners can be rapidly or even completely degraded (Kumar et al., 2011). Many aquatic environments are not suitable for the growth of aerobic microorganisms, because only the top layer of sediments is aerobic.
Dehalogenation of PCBs by Chemical Reagent
The objective of dehalogenation using chemical reagents is converting PCBs to low level toxic compounds through progressive replacement of chlorines (Kulkarni et al., 2008). In order to destroy PCBs, chemical reagents additionally need high temperatures and pressures. The chemical reagents most commonly used are Mg and Zn/acidic or basic solution, Fenton's reagent (FR), and low-valent metals (e.g., alkali metal in alcohol) (Kulkarni et al., 2008). The first application of dehalogenation using chemical reagents can be traced back 90 years when commercial phenols were first used to dehalogenate dioxins and furans. Likewise, chemical reagents can also be used to dehalogenate PCBs, converting them to non-hazardous or less toxic compounds during the treatment. PCB congeners can be dehalogenated with a relatively high efficiency, in short to medium time periods compared to biological treatments (Huling and Pivetz, 2006). Mitoma et al. (2004) conducted their study for detoxification of PCBs using metallic calcium in ethanol for 24 h under atmospheric pressure and room temperature, resulting in 98% reduction. Ryoo et al. (2007) developed a practical disposal of PCBs using polyethylene glycol 600, potassium hydroxide and aluminum. The average removal efficiency of PCBs was about 78% at 100°C with 2 h, which increased to 99% at 150°C and 4 h, particularly for PCB-77, PCB-105, PCB-118, PCB-123, and PCB-169. Nah et al. (2008) used a fine metal powder, glycol and alkali to remove PCBs from waste insulating oil resulting in a removal efficiency of 99.9% for total PCB concentration.
In addition, some studies reported that a combination of chemical solutions and catalysts i.e., catalytic hydrodehalogenation, can result in a higher dechlorination performance (Xu and Bhattacharyya, 2007). It is usually performed with transition metals such as Ni or Pd as heterogeneous catalysts in organic solvents. Extensive research has been conducted on catalytic hydrodehalogenation of PCBs. Gomes et al. (2013) summarized the studies on reductive dehalogenation of PCB in their review paper (Gomes et al., 2013). Such a combination of chemical solution and catalysts could allow PCB dehalogenation in short times under mild conditions (e.g., ambient temperature) with low energy requirement (Ehsan et al., 2003). Nonetheless, this technology is still difficult to apply to the actual presence of PCB contaminated sites because of necessary consideration of multiple uncertainties. For example, when this technology is used to treat contaminants at high concentrations, excessive amounts of reagents are necessary (Gomes et al., 2013). Furthermore, this technology is more impactful on soils due to high temperatures and strong acid and alkali conditions (Gomes et al., 2013).
Removal of PCBs by Activated Carbon
Activated carbon are widely applied for removal of hazardous organic and inorganic compounds due to the highly porous structure of carbonaceous materials that increase the surface area (500–2,500 m2/g) for adsorption or chemical reactions. Adsorption is a physical process where adsorbates are attracted onto the surface structure of materials such as activated carbon. Activated carbon are generally made from natural, renewable and low cost materials (e.g., coconut shell, pall fiber, hardwood, bamboo, lignite, bark husk, peanut hull, coir pith, maize cob, sawdust, and rice husk) (Das et al., 2015). Activated carbon can be classified into three categories depending on the pore size: micropores (diameter < 2 nm); mesopores (2 nm < diameter < 50 nm); and macropores (diameter >50 nm) (Kim et al., 2015). Activated carbon applied for removal of PCBs can also be classified on the basis of their morphologies: granular activated carbon (GAC), powdered activated carbon (PAC), bead activated carbon (BAC), activated carbon fibers (ACFs), and carbon nanotubes (CNTs). Physical properties of these selected activated carbon involved in removal of PCBs from literature are summarized in Table 1 (Mangun et al., 2001; Li et al., 2003; Kim et al., 2007, 2008; Vasilyeva et al., 2010; Hung et al., 2011; Ji et al., 2014).
The particle size and pore size of activated carbon result in relative larger contact surface area between adsorbents and adsorbates, which provide conditions for PCB adsorption to occur. Activated carbon has been widely applied for PCB-contaminated soils. Activated carbon is a sensible choice for PCB removal from contaminated soil and it is recognized as one of the most efficient fundamental approaches (Foo and Hameed, 2010). Choi et al. (2014) used activated carbon to enhance PCB immobilization. They also developed a pilot study in Hunters Point Shipyard at San Francisco in which there was a 73% decrease of PCBs transferred from sediments into the aquatic environment during a 60-month span, when activated carbon (3.7% dry wt.) was added to the sediment (Choi et al., 2009). Vasilyeva et al. (2010) evaluated the removal performances of PAC and GAC in soils contaminated with PCBs (i.e., trichlorobiphenyl, tetrachlorobiphenyl, and pentachlorinated congeners). The results of this study indicated that reduction of PCBs in AC-amended soil is mainly attributed to a decrease in trichlorobiphenyl and tetrachlorobiphenyl congeners. Kjellerup and Edwards (2013) used granular activated carbon to reduce the concentration of PCB contaminated sediments by sequestration. This study showed that the homolog distribution of PCB dechloronation products significantly changed after 500 days. The final dehalogenation products of penta- through hepta-chlorobiphenyl congeners were shifted from tri- through penta-chlorobiphenyls to mono- through tri-chlorobiphenyls. More specifically, Denyes et al. (2012) showed an 89% reduction in the bioavailability of PCBs in historically contaminated soils using biochar (Denyes et al., 2012). Comprehensive reviews of the possibility of biochar for PCB-contaminated soils and sediments can be found in Hilber and Bucheli (2010), Beesley et al. (2011), Rakowska et al. (2012), Gomes et al. (2013).
Moreover, activated carbon can easily be integrated with other auxiliary technologies (e.g., microwave decomposition) or catalysts and this combination of different technologies has been used in recent studies (Liu et al., 2007). Some examples of this combination of multi-technology are; the application of microwave and granular activated carbon for the treatment of soil contaminated by 2,4,5-trichlorobiphenyl (PCB-29) (Liu and Yu, 2006), synthesis of reactive nano-fe/pd bimetallic system-impregnated activated carbon for the simultaneous adsorption and dehalogenation of PCBs (Choi et al., 2008), substituted chlorines of high-chlorinated PCB congeners by activated carbon impregnated with Fe coupled with Pd (Choi et al., 2009), removal of dioxin-like PCBs from fish oil by countercurrent supercritical CO2 extraction and activated carbon (Kawashima et al., 2009), and nano-zerovalent iron contained porous carbon for the adsorption and dehalogenation of PCBs (Liu and Zhang, 2010). However, limited field application has been developed for the most of these studies, though they all show promising lab-scale results. It should be noted that considerable studies on PCB remediation by a combination of anaerobic bacteria (biofilm) and activated carbon as a microbial inoculum delivery system have been conducted and remarkable progress has been made in recent decades (Das et al., 2017). Therefore, it will be independently discussed in the next section.
Advanced PCB Remediation Technologies
Supercritical Water Oxidation
Supercritical water oxidation (SCWO) is a clean technology which occurs in water at temperatures and pressures above the critical point of water (647 K and 22.064 MPa) (Marulanda and Bolaños, 2010). Under these supercritical conditions, water loses its hydrogen bonds and starts to transition from a polar solvent to a nonpolar solvent. As a result, the solubility of PCBs increases in the supercritical water. PCBs are then degraded at these high temperatures and the final products are carbon dioxide, water and mineral acids (Rahuman et al., 2000). Typical operating conditions for commercial SCWO systems are 550–650°C, 250 bar and this technology has been shown to be very efficient, achieving over 99% PCB destruction (Marrone et al., 2004). Additionally, SCWO systems in high temperature environments can rapidly complete the oxidation of PCBs to CO2 and H2O without toxic byproducts.
Studies showed that SCWO is an effective technique for destroying PCBs. Hatakeda et al. (1999) determined the efficiencies of hydrogen peroxide and oxygen for destruction of 3-chlorobiphenyl at different temperatures and oxidant concentrations. In this study, over 99% of 3-chlorobiphenyl was decomposed. Weber et al. (2002) assessed the PCBs destruction in supercritical water under alkaline conditions and over 99% of PCBs were destroyed. Fang et al.'s study showed that SCWO can destroy 93% of decachlorobiphenyl with excess O2 (Fang et al., 2004). Marulanda and Bolaños (2010) used a large scale mineral transformer for PCB-contaminated oil and found that 99.6% of the mixture of PCBs and hydrocarbons was destroyed with 350% oxygen excess at 539°C.
SCWO, however, introduces a few complicating aspects that should be considered. For example, chlorine atoms from the biphenyl ring can produce hydrochloric acid during SCWO, which can corrode the system. Additionally, due to the low dielectric constant of supercritical water, both sticky salts and non-sticky solids are completely precipitated during SCWO (Fang, 2014). These salts deposit on equipment surfaces causing fouling, plugging, and erosion. Researchers have analyzed SCWO and conclude that the salts can accumulate on the surface of equipment requiring high cost maintenance and other operational maintenance procedures (Marrone et al., 2004).
Ultrasonic Radiation
Ultrasonic radiation is a promising method for PCB degradation. It is generally agreed upon that the possible mechanism of ultrasonic radiation is acoustic cavitation (Gedanken, 2004). Cavitation is a process in which mechanical activation destroys the attractive forces of molecules in the liquid phase thereby allowing bubble growth through the diffusion of solute vapor (Yeow and Peng, 2012). The energy inside of the bubbles will release and lead to high temperatures and pressures in microscopic regions resulting in chemical excitation that breaks chemical bonds. As a result, degradation of PCBs can be carried out with high effectiveness and simple handling conditions such as low temperatures and fast reaction times. Ultrasonic radiation is capable of high PCB removal efficiencies (more than 90%) (Lu et al., 2009). Rodríguez and Lafuente (2008) examined the dehalogenation of a PCB mixture using an ultrasonic radiation system at 40°C with a hydrazine hydrochloride/palladium (HZ/Pd) catalyst. Gas chromatography results indicated that the concentration of PCBs in this mixture sample decreased from 2,768 to 25 ppm after 15 min and the PCBs were completely dechlorinated after 30 min. It is important to note that these concentrations are high thus the removal efficiencies may be large due to this factor. Okuno et al. (2000) observed 80–90% degradation of 2-chlorobiphenyl, 4-chlorobiphenyl and 2,2′-dichlorobiphenyl in aqueous solutions in 30–60 min with a 200 kHz ultrasound. Lu and Weavers (2002) explored the laboratory scale application of ultrasound for the treatment of 4-chlorobiphenyl contaminated sediments. Over 90% of 4-chlorobiphenyl in the aqueous, homogeneous solution was destroyed at 20 kHz with a power density of 460 W/L after 20 min. It is to be noted that this sample contained only one congener thus the high efficiencies may be a result of this. A recent variation of PCB remediation by ultrasonic radiation is the ultrasound-assisted chemical process (UACP). Chen et al. (2013) developed a combination of ultrasonic irradiation and radical generations using di-tert-butyl peroxide as a radical initiator to dechlorinate Aroclor 1260. The results demonstrated that UACP has a PCBs removal efficiency of 97% within 3 h.
Even through ultrasonic radiation has many advantages such as high remediation efficiency, no byproducts production, and lowered environmental impact, it also has several drawbacks when compared to other conventional methods (e.g., thermal treatment). For example, ultrasonic radiation is costly due to catalyst loadings and operations; therefore, it cannot easily be operated for large-scale, industrial and commercial purposes. In addition, commercial ultrasonic radiation generally has high energy requirements (Nur Ismayuslini, 2010; Khoddami et al., 2013).
Catalytic Hydrodehalgenation of PCBs by Bimetallic Systems
A bimetallic system consists of two metals sharing an interface or boundary in separate phases such as core-shell bimetallics (Lens et al., 2013). Both phases play an active role in bimetallic systems and the alloyed shell is the catalytically active phase. Bimetallic systems are usually carried out with two different metals: one zero-valent form with a negative reduction potential and the other a transition metals with a high reduction potential as the reducing catalyst (Patel and Suresh, 2007; Gomes et al., 2013). In this process of hydrodehalogenation, the corrosion of the zero-valent metal with water will firstly generate hydrogen at room temperature and pressure. Following that, the hydrogen is absorbed onto the surface of the catalyst to form a metal hydride as the target substrate of dehalogenation (Patel and Suresh, 2007).
Core-shell bimetallics have been successfully utilized to dechlorinate PCBs and other chlorinated organics (Patel and Suresh, 2007; Wu et al., 2012). Studies have been conducted to investigate bimetallic formulations to improve catalyst activity for PCB degradation (Hennebel et al., 2013). Hydrogen gas is generated through the corrosion of Mg by the reduction of water, when the Mg/Pd bimetal system is applied to catalytic hydrodehalogenation of 2-chlorobiphenyl (Wu et al., 2012). The generated hydrogen then adsorbs to the Pd surface, relying on hydrogen bonds (Yang et al., 2011). This yields a stable adsorbed system of PdH2 while dehalogenating the PCB. Other general bimetallic systems for hydrodehalogenation of PCBs include iron/palladium (Fe/Pd), iron/nickel (Fe/Ni), and aluminum/palladium (Al/Pd). Table 2 summarizes experimental conditions and main findings in literature regarding hydrodehalogenation of PCBs by bimetallic systems (DeVor et al., 2008; Venkatachalam et al., 2008; Agarwal et al., 2009; He et al., 2010).
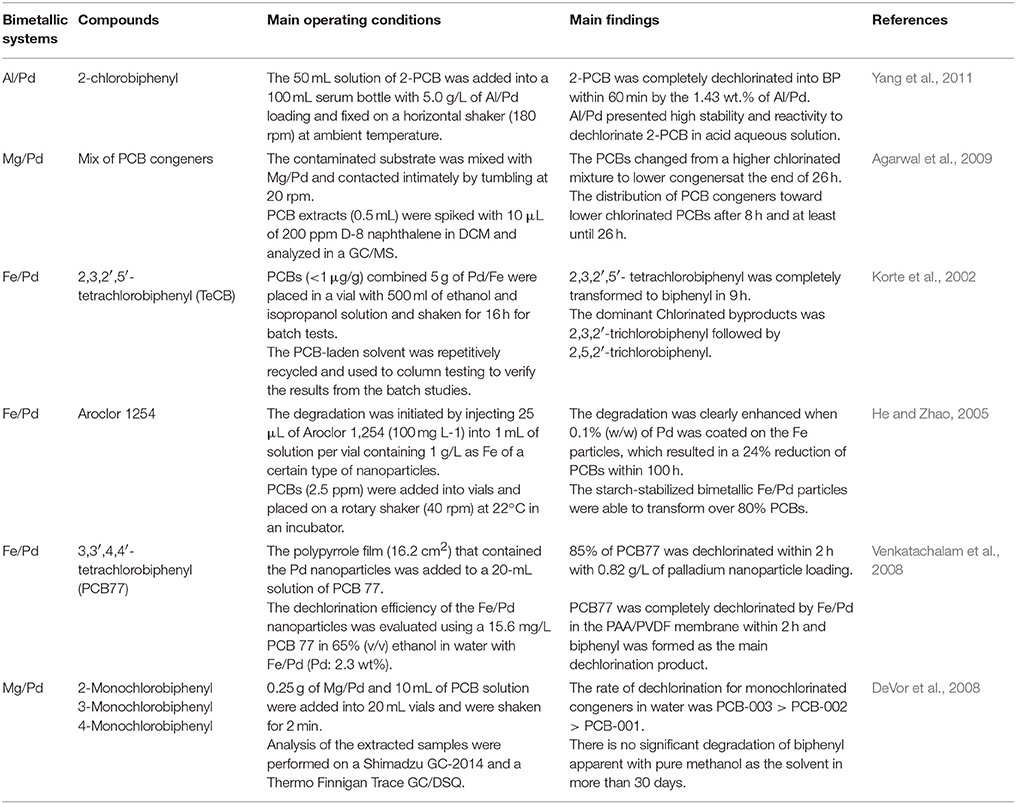
Table 2. Summary of experimental conditions and main findings of studies on hydrodechlorination of PCBs by bimetallic systems.
Bimetallic systems have several potential benefits. They have induced physicochemical properties, such as catalytic performance (Kang et al., 2011). Magnetic metals in combination with noble metals form bimetallic systems which exhibit irreplaceable advantages in pollutions remediation, since noble metals [e.g., silver (Ag), gold (Au), platinum (Pt)] have more recondite electron structures based on surface plasmon resonance (Duan and Wang, 2013). Additionally, two active metals of a bimetallic system can enhance catalytic activity thus treat PCBs and other chlorinated organic compounds in short times with no specialized laboratory equipment (Sakrittichai, 2001). Bimetallic systems also have distinct drawbacks. Some magnetic metals such as cobalt (Co), Ni, and noble metals such as Ag, Au, Pt, are too costly to be used in large commercial operations. Moreover, the reaction rate will significantly reduce, when the passivation of metals carry on during the remediation process (Doong and Wu, 1992). More generally, the zero-valent metals involved in hydrodehalogenation of PCBs by bimetallic systems such as iron, zinc or magnesium can generate partially dehalogenated products that may be more toxic than the previous target compounds (transformation of target compounds to phenol) (Grittini et al., 1995).
Nanoscale Zero-Valent Iron (nZVI) Based Reductive Dehalogenation
The diameter of nZVI particles is < 100 nm and they usually have a core-shell structure. The outside ion of nZVI particles can react with water and oxygen to form an outer (hydr)oxide layer in aqueous environments (Nurmi et al., 2005; O'carroll et al., 2013). As a result, this outer oxide layer allows electron transfer from the metal through the oxide conduction band or localized band. Furthermore, the outer oxide layer could serve as an adsorbent for PCBs. In 1994, Schreier and Reinhard (1994) reported dehalogenation of alkyl halides (RX) by Fe powder in oxygen-free and buffered water, previously a method with difficult to predict outcomes. Since then, a three step mechanisms was proposed to explain the dehalogenation process of alkyl halides (Matheson and Tratnyek, 1994). Regarding to PCBs, the electrons can directly transfer from Fe0 to the PCB moleculars under an acid environment and the Fe2+ will be generated as an product (Figure 2; Gomes et al., 2016). On the basis of the proposed mechanism, nZVI with a large surface area has been successfully used to achieve the dehalogenation process in water or PCB-contaminated soil in the presence of catalysts. Current research is exploring the ability of nZVI particles to dechlorinate highly chlorinated PCBs. A study conducted by Gardner et al. (2004) indicated that 3% of nZVI (w/w) can rapidly and extensively dechlorinate PCB-contaminated sediments in the New Bedford Harbor and the Housatonic River (Gardner et al., 2004). The nZVI particles removed 84% of PCBs from the Housatonic River sediments which had an initial concentration of approximately 50 ppm. In 2007, it was found that more than 95% of 10 different PCB congener remediation, with concentrations ranging up to 1 mg/kg soil or filter cake, can be catalyzed by iron oxide and V2O5/TiO2 at 300°C, when the iron nano-particles were applied to remediate PCB-contaminated soil (Varanasi et al., 2007). Long et al. (2014) found that dehalogenation of Aroclor 1260 in soil can be enhanced by anaerobic composting with nZVI. The nZVI can provide an appropriate pH (8–9) and reduce volatile fatty acid inhibition thereby stimulating the growth of microorganisms and by generating hydrogen gas via corrosion of nZVI, thereby enhance dehalogenation. The results showed that dehalogenation performance was enhanced by 34% after adding 10 mg g−1 of nZVI to soil containing 1 mg/kg for 140 days. Liu et al. (2014) used thermal desorption combined with nZVI to remediate PCB-contaminated soil at different temperature conditions (300°-600°C). They found that 97.40 % of trichlorobiphenyl (TrCB) and tetrachlorobiphenyl (TeCB) was removed without nZVI at 600°C. In contrast, 98.35% of TrCB and TeCB was eliminated with the use of 100 mg of nZVI at the same temperature.
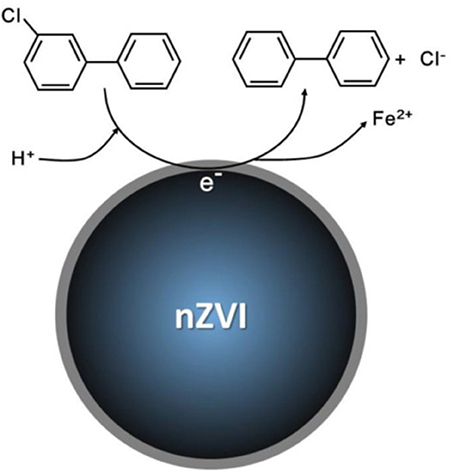
Figure 2. Proposed mechanisms for abiotic dechlorination of PCBs: Electron transfers from Fe0 to monochlorobiphenyl (Gomes et al., 2016).
NZVI-remediation could offer advantages as compared to other in situ remediation technologies. For example, nZVI has been used to remediate PCBs as an advanced technology in the environment due to its catalytic properties and high degradation performance (Ahmadi et al., 2011). A large surface area of nZVI particles can increase reactivity and, therefore, results in high speed contaminant degradation (Nurmi et al., 2005; Cook, 2009). Additionally, nZVI can be used in recalcitrant contaminated water such as deep contaminated ground water since nZVI can suspend in water for a longer time due to its small size (Singh et al., 2012). However, the application of nZVI is still controversial. To date, the nZVI technologies in practice are mostly the application of chlorinated solvents in aquifers and most cases of nZVI applications are lab scale and have taken place in North America in comparison to the few field applications in Europe (e.g., Czech Republic, Germany and Italy) (Bardos et al., 2015). In broad terms the surface of nZVI particles in an aquifer will be oxidized into iron (II) and (III) oxides/hydroxides. As a consequence, the particle surface reactivity will be limited (Lee and Jou, 2012). In addition, the nZVI particles will undesirably agglomerate and convert to large particles because the most reactive particles agglomerate more readily (Bardos et al., 2015). The toxicological effect of nZVI particles are usually related to their nano-scale size. The nZVI used in remediation are typically 10–100 nm as aforementioned and the unique toxicity of nZVI has been only observed in nanoparticles smaller than 30 nm (Bardos et al., 2014). Some studies reported the toxicity of nZVI is specific to bacteria and biota such as collembolan (srpingtails) and lumbricina (earthworms) (Bardos et al., 2015).
Possibility of PCB Remediation Using Multi-Technology
PCBs Removal by Biofilm Covered Activated Carbon
In the early 1970s, it was found that biofilm covered activated carbon particles can efficiently remove organic pollutants (Dussert and Tramposch, 1997). Since then, the combination of bacterial biofilm and activated carbon has been widely used as a practiced treatment method for wastewater treatment, post-treatment, potable water purification, and organic contaminants removal (Tammaro et al., 2014). Kolb and Wilderer (1997) used an integrated activated carbon adsorption and biodegradation system to reduce the concentration of critical components (e.g., Benzene and 2-chlorophenol) from industrial process wastewaters, thereby mitigating the inhibitory effects on the bacteria. Islam et al. (2016) recently reported that the biofilm treatment process with activated carbon adsorption can effectively treat naphthenic acids (NAs) in oil sand process affected waters (OSPW). After 28 days, the NAs removal efficiencies of OSPW for biodegradation and adsorption were 14 and 63% respectively. Such successful attempts have led to the recognition of the combination of bacterial biofilm and activated carbon as a viable remediation technology for PCB-contaminated sediment as suggested by the USEPA. Some preliminary research of PCB-contaminated sediments treated with granular activated carbon (GAC) have been conducted. A bench-scale study on bioaugmentation of PCBs in aqueous wastes conducted by Ghosh et al. demonstrated that 62% of PCBs were removed by a granular activated carbon column Ghosh et al. (2011) In addition, a field study indicated that the bioaccumulation of PCBs were reduced in clams, worms, and amphipods after the was sediment treated with 1–5% (w/w) of GAC. Sediment treated with 3.4% of coke activated carbon achieved 85 and 92% reductions in aqueous equilibrium PCB concentrations during 1- and 6-month experimental spans, respectively (Zimmerman et al., 2004). These studies showed that the indigenous bacteria found in sediment were capable of PCB dehalogenation. However, microbial degradation of PCBs are limited in performance due to the relatively low abundance of PCB-dechlorinating microorganisms and low bioavailability of PCBs, especially for in-situ microbial degradation (Kjellerup and Edwards, 2013). There was an insignificant decrease in the overall concentration of parent PCB congeners. Tri-, tetra-, and penta-chlorinated congener concentrations were still relatively high, while mono- and di- chlorinated congeners were dominant in the presence of activated carbon (Kjellerup et al., 2014). Therefore, a combination of adsorbent sequestration and bioaugmentation through biofilm covered activated carbon systems is proposed to enhance the biodegradation of low concentration PCBs in sediment. An adsorbent (e.g., activated carbon and biochars) could adsorb PCBs from aquatic sediments and concentrate PCB dechlorinating microorganisms onto its surface thereby applying the microbial communities to the PCB-contaminated sediment.
Such a combination of PCB-dechlorinating microbial communities in the form of biofilms on surfaces of activated carbon provide several advantages. The biofilm covered activated carbon system usually has a removal efficiency of over 60% due to simultaneous adsorption and biodegradation (Kjellerup and Edwards, 2013). A compact space between biofilms with large cell density and activated carbon surface could allow microorganisms to utilize PCBs as an electron acceptor thereby enabling subsequent degradation. In addition, the microorganisms embedded within an adherent biofilm attain a high resistance to toxic pollutants (Köhler et al., 2006). Furthermore, a biofilm covered activated carbon systems can maintain long solid retention times thereby biodegrading persistent organics at a low growth rate (Aktaş and Eçen, 2007). One drawback of biofilm covered activated carbon system is that some slow growing PCB dechlorinating microorganisms might be bloom-growing thus affected by other easily-degradable organic compounds or pollutants (Abromaitis et al., 2016). For example, some researchers pointed out that the concentration of persistent organic compounds (e.g., PCBs) in a municipal wastewater treatment plant effluent is very low (0.019–1.7 μg/L), though the biochemical oxygen demand of secondary effluent is generally between 1.7 and 7.7 mg/L (Deblonde et al., 2011; Abromaitis et al., 2016).
PCBs Remediation Technologies Coupled to Electrokinetic Remediation
Soil and aquatic environments (e.g., lake, river, and groundwater) contaminated with PCBs through direct or secondary exposure have resulted in detrimental environmental impacts (Beesley et al., 2011). More advanced alternatives to unsustainable PCB remediation techniques have been sought. Electrokinetic remediation uses low-level direct current as a “cleaning agent” to remove organic pollutants from the soil or other environments (Acar and Alshawabkeh, 1993). Electrokinetic remediation can be conducted in-situ. This generally includes an external, direct current source, and an anode and cathode immersed in the electrolytic solution. The organic pollutants driven by ionic migration and electrophoresis will migrate to the electrodes when the direct current is applied. Other technologies (e.g., nZVI dehalogenation) coupled to electrokinetic remediation could lead to new advancements in PCB remediation (Gomes et al., 2012). Fan et al. (2013) used nano Pd/Fe particles coupled with electrokinetic technology to remediate PCB contaminated soil. The results indicated that high electroosmotic flow can facilitate nano Pd/Fe transport, but the degradation was low without the solubilization of PCBs strongly adsorbed in soils. Gomes et al. (2014) proposed a cost-effective solution for PCB contaminated soil remediation by using electrodialytic remediation combined with nZVI particles. Electrodialytic remediation combines electrodialysis with the electrokinetic movement of ions to remove heavy metals (Ottosen et al., 1997). Two surfactants (saponin and Tween 80) were involved in this study to enhance PCB desorption and removal from polluted soil. The results show that the removal efficiencies of highly chlorinated PCB congeners (penta-, hexa-, hepta-, and octa-chlorobiphenyl) were between 9 and 96%. Chun et al. (2013) developed an innovative approach to significant dehalogenation of PCBs in sediment using electrical stimulation which provide electron donors and acceptors to PCB dechlorinating microorganisms. The results of their study indicated that the concentration of weathered PCBs decreased by 40–60%, from original concentrations of about 20 mg/kg dry sediments, in microcosms treated with electric current as compared to that of PCBs observed in control reactors.
nZVI Particles in Combination With a Second Metal as a Promising Technology for PCBs Remediation
The effectiveness of nZVI based reductive dehalogenation described in this paper are quite specific. One major concern in nZVI based reductive dehalogenation processes is the reduction of surface reactivity due to iron oxide formation on the surface of the nanoparticles. As aforementioned, combining two or several different remediation technologies as a potentially viable alternative for PCBs remediation can overcome the deficiencies associated with individual treatment methods (Naddeo et al., 2011). Furthermore, optimization of PCBs remediation efficiency can be achieved within appropriate costs. In recent years, the effectiveness of nZVI has been proven in the treatment of a wide variety of contaminants such as chlorinated methanes, brominated methanes, trihalomethanes, chlorinated ethenes, chlorinated benzenes, and other polychlorinated hydrocarbons (Nowack, 2008). Regarding nZVI particles coupled with electrokinetic remediation discussed above, some studies indicated that nZVI particles in combination with a second metal (e.g., Pt, Ag) are more effective in treating chlorine-containing organic pollutants with high reaction rates (Liou et al., 2005; Cao et al., 2011). Metal-covered nZVI particles can effectively reduce the activation energy of the pollutants and increase the reaction rate of dechlorinaton reactions (Liou et al., 2005). Additionally, metal-covered nZVI particles can rapidly achieve dehalogenation through increasing the particle surface area and surface activity (Clark et al., 2003). Zhuang et al. (2011) have proven that 2,3,4-trichlorobiphenyl (PCB-21) can be rapidly dechlorinated by palladized, nanosized ZVI (Pd/nFe) (Zhuang et al., 2011). Their results suggested that the degradation rate of PCB-21 (normalized rate constant of 10−1) by using Pd/nFe was three orders of magnitude faster than that of PCB-21 when using unpalladized ZVI (normalized rate constant of 10−4). Le et al. (2015) developed an integrated remediation system for dehalogenation of Aroclor 1248 using bimetallic nanoparticles Pd/nFe and biodegradation via burkholderia xenovorann LB400. The dehalogenation efficiencies of tri-, tetra-, penta-, and hexachlorinated biphenyls were 99, 92, 84, and 28%, respectively. After nano-bio treatment, the toxicity of the residual PCBs in terms of toxic equivalent values decreased from 33.8 × 10−5 to 9.5 × 10−5 μg/g.
Assessment of PCB Remediation Technologies
Four criteria including the cost, removal efficiency, time duration, and environmental burdens were provided to determine a comprehensive framework for PCB remediation strategies and the combinations of these technologies. As shown in Table 3, chemical reagent, supercritical water oxidation, ultrasonic radiation, bimetallic systems, nZVI, and nZVI combination with a second metal have a high remediation efficiency (78–99%) with a rapid reaction time. More importantly, most of these technologies does not generate toxic byproducts except the supercritical water oxidation and bimetallic systems. The generated byproducts from these technologies are the major drawbacks that could be released to the environment with negative impacts. However, these technologies usually require excessive costs. Therefore, any efforts relative to the implementation of these technologies to the contaminated filed sites or large commercial operations will encounter with challenges. Phytoremediation and microbial degradation have a limited remediation efficiency (40–60%). They usually cannot immediately apply to the PCB sources due to requiring a long remediation and monitoring time as compared to the technologies mentioned above. Even though phytoremediation and microbial degradation have a limited remediation efficiency with a long-term remediation time, the major advantage is the relatively low implementation costs. Therefore, they could be applied to an in-situ contamination site as a full-scale application. Activated carbon and the biofilm covered activated carbon obtained the highest scores according to this table. A low cost and relatively high remediation efficiency allow them to be applied to either in-situ or ex-situ PCBs remediation. More specifically, the biofilm covered activated carbon system has a considerable removal efficiency which is usually over 60% due to simultaneous adsorption and biodegradation. A compact space between biofilms and activated carbon surface could allow bacteria to efficiently attack PCB molecular as an electron acceptor thereby. However, it should be noted that some studies on PCBs remediation by a combination of anaerobic biofilm and activated carbon involved in this paper were conducted under a strictly experimental condition (e.g., mesocosms with extremely anaerobic environments), even though a remarkable progress has been made in recent decades. A successful treatment of PCBs not only depends on its remediation efficiency, it is also need to consider the cost, time duration, and environmental burdens. On the basis of the discussion of this paper, therefore, the future vision of PCBs remediation could be a comprehensive treatment based on the biofilm covered activated carbon particles as a microbial inoculum delivery system.
PCBs are very toxic chemical compounds. Thus, a comprehensive assessment of the environmental impacts of PCB destruction is an important criterion for selection of PCB remediation technologies. However, there is little evidence/information that supports this sort of assessment for most of the proposed PCBs destruction technologies involved in this paper (Weber, 2007). The comprehensive assessment of PCB formation and destruction are only available for the incineration process (Buekens and Huang, 1998; Weber, 2007). In addition, assessments of PCB removal stability is another significant parameter for PCB destruction technologies. For instance, the destruction efficiency of a wastewater treatment facility could be difficult to evaluate due to low concentration levels in the effluent streams. This would require continuous PCB sampling and long-term data monitoring and collection such as time series analysis on the basis of PCB emission. Therefore, the assessment of the PCB destruction technologies should include two essential parts: (1) assessment of destruction efficiencies for in-situ and ex-situ PCB remediation technologies and the environmental impacts or risks associated with the implementation and operation of the respective technology; (2) continuous PCB sampling and long-term data monitoring and collection in order to assess the reliability of the proposed PCB remediation technology.
Conclusions
This paper reviewed the treatment strategies for PCBs remediation. Chemical reagent, supercritical water oxidation, ultrasonic radiation, bimetallic systems, nZVI, and nZVI combination with a second metal have a high remediation efficiency (78–99%) with a rapid reaction time. However, these technologies usually require excessive costs. Phytoremediation and microbial degradation have a limited remediation efficiency (40–60%). Even though phytoremediation and microbial degradation have a limited remediation efficiency with a long-term remediation time, the major advantage is the relatively low implementation costs. Therefore, they are not appropriate for an in-situ contamination site as a full-scale application. Activated carbon and the biofilm covered activated carbon approaches obtained the highest scores according to this table as compared to the technologies mentioned above. A low cost and relatively high remediation efficiency (more than 60%) allow them to be applied to either in-situ or ex-situ PCBs remediation. PCBs are complex chemicals, so knowledge of their chemical and physical properties is important to better understand their transport and fate thereby for selecting appropriate remediation approaches. The possibility of PCB remediation by using multiple technologies discussed in this paper need more data and pilot scale experiments in order to evaluate the effectiveness. The future vision of PCB remediation could be a comprehensive treatment solution. Because successful treatment of PCBs not only depends on the appreciated selection of the most effective remediation technology, it is also needs to consider the public acceptance and environmental and human health impacts of the remediation technology, neither of which have been achieved.
Author Contributions
RJ and BK wrote this paper. SF did the language editing. BK conceived this study.
Funding
Funding was obtained from SERDP grant ER-2135 and University of Maryland, Clark School of Engineering to BK.
Conflict of Interest Statement
The authors declare that the research was conducted in the absence of any commercial or financial relationships that could be construed as a potential conflict of interest.
References
Abromaitis, V., Racys, V., Van Der Marel, P., and Meulepas, R. (2016). Biodegradation of persistent organics can overcome adsorption–desorption hysteresis in biological activated carbon systems. Chemosphere 149, 183–189 doi: 10.1016/j.chemosphere.2016.01.085
Acar, Y. B., and Alshawabkeh, A. N. (1993). Principles of electrokinetic remediation Environ. Sci. Technol. 27, 2638–2647 doi: 10.1021/es00049a002
Agarwal, S., Al-Abed, S. R., and Dionysiou, D. D. (2007). In situ technologies for reclamation of PCB-contaminated sediments: current challenges and research thrust areas. J. Environ. Eng. 133, 1075–1078. doi: 10.1061/(ASCE)0733-9372(2007)133:12(1075)
Agarwal, S., Al-Abed, S. R., and Dionysiou, D. D. (2009). A feasibility study on Pd/Mg application in historically contaminated sediments and PCB spiked substrates. J. Hazard. Mater. 172, 1156–1162. doi: 10.1016/j.jhazmat.2009.07.117
Ahmadi, M., Mashhoon, F., Kaveh, R., and Tarkian, F. (2011). Use of mechanicaly prepared Iron nano particles for nitrate removal from water. Asian J. Chem. 23:1205.
Aken, B. V., Correa, P. A., and Schnoor, J. L. (2009). Phytoremediation of polychlorinated biphenyls: new trends and promises. Environ. Sci. Technol. 44, 2767–2776. doi: 10.1021/es902514d.
Aktaş, O., and Eçen, F. (2007). Adsorption, desorption and bioregeneration in the treatment of 2-chlorophenol with activated carbon. J. Hazard. Mater. 141, 769–777. doi: 10.1016/j.jhazmat.2006.07.050
Alcock, R. E., Halsall, C. J., Harris, C. A., Johnston, A., Lead, W. A., Sanders, G., et al. (1994). Contamination of environmental samples prepared for PCB analysis. Environ. Sci. Technol. 28, 1838–1842. doi: 10.1021/es00060a013
Alder, A. C., Haggblom, M. M., Oppenheimer, S. R., and Young, L. (1993). Reductive dechlorination of polychlorinated biphenyls in anaerobic sediments. Environ. Sci. Technol. 27, 530–538. doi: 10.1021/es00040a012
Anyasi, R., and Atagana, H. (2011). Biological remediation of polychlorinated biphenyls (PCB) in the environment by microorganisms and plants. Afr. J. Biotechnol. 10, 18916–18938. doi: 10.5897/AJB10.557
Åslund, M. L. W., Rutter, A., Reimer, K. J., and Zeeb, B. A. (2008). The effects of repeated planting, planting density, and specific transfer pathways on PCB uptake by Cucurbita pepo grown in field conditions. Sci. Total Environ. 405, 14–25. doi: 10.1016/j.scitotenv.2008.07.066
Azubuike, C. C., Chikere, C. B., and Okpokwasili, G. C. (2016). Bioremediation techniques–classification based on site of application: principles, advantages, limitations and prospects. World J. Microbiol. Biotechnol. 32, 180. doi: 10.1007/s11274-016-2137-x
Bardos, P., Bone, B., Daly, P., Elliott, D., Jones, S., Lowry, G., et al. (2014). A Risk/Benefit Appraisal for the Application of Nano-Scale Zero Valent iron (nZVI) for the Remediation of Contaminated Sites. WP9 NanoRem.
Bardos, P., Bone, B., Cerník, M., Elliott, D. W., Jones, S., and Merly, C. (2015). Nanoremediation and international environmental restoration markets. Remed. J. 25, 83–94. doi: 10.1002/rem.21426
Batterman, S., Chernyak, S., Gouden, Y., Hayes, J., Robins, T., and Chetty, S. (2009). PCBs in air, soil and milk in industrialized and urban areas of KwaZulu-Natal, South Africa. Environ. Pollut. 157, 654–663. doi: 10.1016/j.envpol.2008.08.015
Bedard, D. L. (2008). A case study for microbial biodegradation: anaerobic bacterial reductive dechlorination of polychlorinated biphenyls-from sediment to defined medium. Annu. Rev. Microbiol. 62, 253–270. doi: 10.1146/annurev.micro.62.081307.162733
Beesley, L., Moreno-Jiménez, E., Gomez-Eyles, J. L., Harris, E., Robinson, B., and Sizmur, T. (2011). A review of biochars' potential role in the remediation, revegetation and restoration of contaminated soils. Environ. Pollut. 159, 3269–3282. doi: 10.1016/j.envpol.2011.07.023
Bevinakatti, B. G., and Ninnekar, H. Z. (1922). Degradation of biphenyl by a micrococcus species. Appl. Microbiol. Biotechnol. 38, 273–275.
Bianucci, A., Ryslava, E., Mackova, M., and Demnerova, K. (2004). Bacterial PCB degradation in presence of compounds of plants origin. Int. Biodeter. Biodegrad. 53:277.
Borja, J., Taleon, D. M., Auresenia, J., and Gallardo, S. (2005). Polychlorinated biphenyls and their biodegradation. Process Biochem. 40, 1999–2013. doi: 10.1016/j.procbio.2004.08.006
Boyle, A. W., Silvin, C. J., Hassett, J. P., Nakas, J. P., and Tanenbaum, S. (1992). Bacterial PCB biodegradation. Biodegradation 3, 285–298. doi: 10.1007/BF00129089
Brown, J. F., Bedard, D. L., Brennan, M. J., Carnahan, J. C., Feng, H., and Wagner, R. E. (1987). Polychlorinated biphenyl dechlorination in aquatic sediments. Science 236, 709–712. doi: 10.1126/science.236.4802.709
Buekens, A., and Huang, H. (1998). Comparative evaluation of techniques for controlling the formation and emission of chlorinated dioxins/furans in municipal waste incineration. J. Hazard. Mater. 62, 1–33. doi: 10.1016/S0304-3894(98)00153-8
Busset, G., Sangely, M., Montrejaud-Vignoles, M., Thannberger, L., and Sablayrolles, C. (2012). Life cycle assessment of polychlorinated biphenyl contaminated soil remediation processes. Int. J. Life Cycle Assess. 17, 325–336. doi: 10.1007/s11367-011-0366-7
Cao, J., Xu, R., Tang, H., Tang, S., and Cao, M. (2011). Synthesis of monodispersed CMC-stabilized Fe–Cu bimetal nanoparticles for in situ reductive dechlorination of 1, 2, 4-trichlorobenzene. Sci. Total Environ. 409, 2336–2341. doi: 10.1016/j.scitotenv.2011.02.045
Chekol, T., Vough, L. R., and Chaney, R. L. (2004). Phytoremediation of polychlorinated biphenyl-contaminated soils: the rhizosphere effect. Environ. Int. 30, 799–804. doi: 10.1016/j.envint.2004.01.008
Chen, J. R., Kim, D., Park, J. S., Gil, K. I., and Yen, T. F. (2013). Reductive dechlorination of polychlorinated biphenyls (PCBs) by ultrasound-assisted chemical process (UACP). Environ. Earth Sci. 69, 1025–1032. doi: 10.1007/s12665-012-2191-0
Choi, H., Al-Abed, S. R., and Agarwal, S. (2009). Catalytic role of palladium and relative reactivity of substituted chlorines during adsorption and treatment of PCBs on reactive activated carbon. Environ. Sci. Technol. 43, 7510–7515. doi: 10.1021/es901298b
Choi, H., Al-Abed, S. R., Agarwal, S., and Dionysiou, D. D. (2008). Synthesis of reactive nano-Fe/Pd bimetallic system-impregnated activated carbon for the simultaneous adsorption and dechlorination of PCBs. Chem. Mater. 20, 3649–3655. doi: 10.1021/cm8003613
Choi, Y., Cho, Y.-M., and Luthy, R. G. (2014). In situ sequestration of hydrophobic organic contaminants in sediments under stagnant contact with activated carbon. 1. Column studies. Environ. Sci. Technol. 48, 1835-1842. doi: 10.1021/es403335g
Chun, C. L., Payne, R. B., Sowers, K. R., and May, H. D. (2013). Electrical stimulation of microbial PCB degradation in sediment. Water Res. 47, 141–152. doi: 10.1016/j.watres.2012.09.038
Clark, C. J., Rao, P. S. C., and Annable, M. D. (2003). Degradation of perchloroethylene in cosolvent solutions by zero-valent iron. Sci. Total Environ. 96, 65–78. doi: 10.1016/S0304-3894(02)00162-0
Cook, S. M. (2009). Assessing the Use and Application of Zero-Valent Iron Nanoparticle Technology for Remediation at Contaminated Sites. Washington, DC: Jackson State University.
Das, D., Samal, D. P., and Meikap, B. (2015). Preparation of activated carbon from green coconut shell and its characterization. J. Chem. Eng. Process Technol. 6:248. doi: 10.4172/2157-7048.1000248
Das, N., Basak, L. V. G., Salam, J. A., and Abigail, E. A. (2017). Application of biofilms on remediation of pollutants-an overview. J. Microbiol. Biotechnol. Res. 2, 783–790.
Deblonde, T., Cossu-Leguille, C., and Hartemann, P. (2011). Emerging pollutants in wastewater: a review of the literature. Int. J. Hyg. Environ. Health 214, 442–448. doi: 10.1016/j.ijheh.2011.08.002
Denyes, M. J., Langlois, V. S., Rutter, A., and Zeeb, B. A. (2012). The use of biochar to reduce soil PCB bioavailability to Cucurbita pepo and Eisenia fetida. Sci. Total Environ. 437, 76–82. doi: 10.1016/j.scitotenv.2012.07.081
DeVor, R., Carvalho-Knighton, K., Aitken, B., Maloney, P., Holland, E., Talalaj, L., et al. (2008). Dechlorination comparison of mono-substituted PCBs with Mg/Pd in different solvent systems. Chemosphere 73, 896–900. doi: 10.1016/j.chemosphere.2008.07.006
Doong, R.A., and Wu, S.C. (1992). Reductive dechlorination of chlorinated hydrocarbons in aqueous solutions containing ferrous and sulfide ions. Chemosphere 24, 1063–1075. doi: 10.1016/0045-6535(92)90197-Y
Duan, S., and Wang, R. (2013). Bimetallic nanostructures with magnetic and noble metals and their physicochemical applications. Prog. Nat. Sci. 23, 113–126. doi: 10.1016/j.pnsc.2013.02.001
Dunnivant, F. M., Elzerman, A. W., Jurs, P. C., and Hasan, M. N. (1992). Quantitative structure-property relationships for aqueous solubilities and Henry's law constants of polychlorinated biphenyls. Environ. Sci. Technol. 26, 1567–1573. doi: 10.1021/es00032a012
Dussert, B., and Tramposch, W. (1997). Impact of support media on the biological treatment of ozonated drinking water. J. Int. Ozone Assoc. 19, 97–108. doi: 10.1080/01919519708547308
Ehsan, S., Prasher, S. O., and Marshall, W. D. (2003). Estimates of total polychlorinated biphenyl (PCB) compounds in soils/sediments by hydrogenolysis to dicyclohexyl. J. Environ. Monit. 5, 644–648. doi: 10.1039/b304716d
Fan, G., Cang, L., Qin, W., Zhou, C., Gomes, H. I., and Zhou, D. (2013). Surfactants-enhanced electrokinetic transport of xanthan gum stabilized nanoPd/Fe for the remediation of PCBs contaminated soils. Sep. Purif. Technol. 114, 64–72. doi: 10.1016/j.seppur.2013.04.030
Fang, Z. (2014). Near-Critical and Supercritical Water and Their Applications for Biorefineries. Springer.
Fang, Z., Xu, S., Butler, I. S., Smith, R. L., and Kozinski, J. A. (2004). Destruction of decachlorobiphenyl using supercritical water oxidation. Energy Fuels 18, 1257–1265. doi: 10.1021/ef0499630
Ficko, S. A., Rutter, A., and Zeeb, B. A. (2010). Potential for phytoextraction of PCBs from contaminated soils using weeds. Sci. Total Environ. 408, 3469–3476. doi: 10.1016/j.scitotenv.2010.04.036
Field, J. A., and Sierra-Alvarez, R. (2008). Microbial degradation of chlorinated dioxins. Chemosphere 71, 1005–1018. doi: 10.1016/j.chemosphere.2007.10.039
Foo, K. Y., and Hameed, B. H. (2010). Detoxification of pesticide waste via activated carbon adsorption process. J. Hazard. Mater. 175, 1–11. doi: 10.1016/j.jhazmat.2009.10.014
Furukawa, K., Suenaga, H., and Goto, M. (2004). Biphenyl dioxygenases: functional versatilities and directed evolution. J. bacteriol. 186, 5189–5196. doi: 10.1128/JB.186.16.5189-5196.2004
Gardner, K., Aulisio, D., and Spear, J. M. (2004). “In-situ dechlorination of polychlorinated biphenyls in sediments using zero-valent iron,” in PowerPoint Presentation at the RTDF Sediments Meeting, 18–19.
Gedanken, A. (2004). Using sonochemistry for the fabrication of nanomaterials. Ultrason. Sonochem. 11, 47–55. doi: 10.1016/j.ultsonch.2004.01.037
Gerhardt, K. E., Huang, X.-D., Glick, B. R., and Greenberg, B. M. (2009). Phytoremediation and rhizoremediation of organic soil contaminants: potential and challenges. Plant Sci. 176, 20–30. doi: 10.1016/j.plantsci.2008.09.014
Ghosh, U., Luthy, R. G., Cornelissen, G., Werner, D., and Menzie, C. A. (2011). In-situ sorbent amendments: a new direction in contaminated sediment management. Environ. Sci. Technol. 45, 1163–1168. doi: 10.1021/es102694h
Gomes, H. I., Dias-Ferreira, C., Ottosen, L. M., and Ribeiro, A. B. (2014). Electrodialytic remediation of polychlorinated biphenyls contaminated soil with iron nanoparticles and two different surfactants. J. Colloid Interface Sci. 433, 189–195. doi: 10.1016/j.jcis.2014.07.022
Gomes, H. I., Dias-Ferreira, C., and Ribeiro, A. B. (2012). Electrokinetic remediation of organochlorines in soil: enhancement techniques and integration with other remediation technologies. Chemosphere 87, 1077–1090. doi: 10.1016/j.chemosphere.2012.02.037
Gomes, H. I., Dias-Ferreira, C., and Ribeiro, A. B. (2013). Overview of in situ and ex situ remediation technologies for PCB-contaminated soils and sediments and obstacles for full-scale application. Sci. Total Environ. 445, 237–260. doi: 10.1016/j.scitotenv.2012.11.098
Gomes, H. I., Fan, G., Ottosen, L. M., Dias-Ferreira, C., and Ribeiro, A. B. (2016). “Nanoremediation coupled to electrokinetics for PCB removal from soil,” in Electrokinetics Across Disciplines and Continents, eds A. B. Ribeiro, E. P. Mateus, and N. Couto (Caparica: Springer), 331–350.
Goncharov, A., Haase, R. F., Santiago-Rivera, A., Morse, G., Mccaffrey, R. J., Rej, R., et al. (2008). High serum PCBs are associated with elevation of serum lipids and cardiovascular disease in a Native American population. Environ. Res. 106, 226–239. doi: 10.1016/j.envres.2007.10.006
Grittini, C., Malcomson, M., Fernando, Q., and Korte, N. (1995). Rapid dechlorination of polychlorinated biphenyls on the surface of a Pd/Fe bimetallic system. Environ. Sci. Technol. 29, 2898–2900. doi: 10.1021/es00011a029
Häggblom, M. M., Ahn, Y.-B., Fennell, D. E., Kerkhof, L. J., and Rhee, S.-K. (2003). Anaerobic dehalogenation of organohalide contaminants in the marine environment. Adv. Appl. Microbiol. 53, 61–84. doi: 10.1016/S0065-2164(03)53002-7
Harms, H., Bokern, M., Kolb, M., and Bock, C. (2003). “Transformation of organic contaminants by different plant systems,” in Phytoremediation Transformation and Control of Contaminants, eds S. C. McCutcheon and J. L. Schnoor (Braunschweig: John Wiley & Sons, Inc.) 285–316.
Harvey, P. J., Campanella, B. F., Castro, P. M., Harms, H., Lichtfouse, E., Schäffner, A. R., et al. (2002). Phytoremediation of polyaromatic hydrocarbons, anilines and phenols. Environ. Sci. Pollut. Res. 9, 29–47. doi: 10.1007/BF02987315
Hatakeda, K., Ikushima, Y., Aizawa, O. S., and and, N. (1999). Supercritical water oxidation of polychlorinated biphenyls using hydrogen peroxide. Chem. Eng. Sci. 54, 3079–3084. doi: 10.1016/S0009-2509(98)00392-3
He, F., and Zhao, D. (2005). Preparation and characterization of a new class of starch-stabilized bimetallic nanoparticles for degradation of chlorinated hydrocarbons in water. Environ. Sci. Technol. 39, 3314–3320. doi: 10.1021/es048743y
He, F., Zhao, D., and Paul, C. (2010). Field assessment of carboxymethyl cellulose stabilized iron nanoparticles for in situ destruction of chlorinated solvents in source zones. Water Res. 44, 2360–2370. doi: 10.1016/j.watres.2009.12.041
Hennebel, T., Fitts, J., Van Nevel, S., and Boon, N. (2013). “Pd nanocatalysts for PCB removal,” in Nanotechnology for Water and Wastewater Treatment, eds P. Lens, J. Virkutyte, V. Jegatheesan, and S. Al-Abed (IWA Publishing), 209–227.
Hilber, I., and Bucheli, T. D. (2010). Activated carbon amendment to remediate contaminated sediments and soils: a review. Global Nest. J. 12, 305–307. doi: 10.30955/gnj.000723
Hung, P. C., Lo, W. C., Chi, K. H., Chang, S. H., and Chang, M. B. (2011). Reduction of dioxin emission by a multi-layer reactor with bead-shaped activated carbon in simulated gas stream and real flue gas of a sinter plant. Chemosphere 82, 72–77. doi: 10.1016/j.chemosphere.2010.10.004
Islam, M. S., Zhang, Y., Mcphedran, K. N., Liu, Y., and El-Din, M. G. (2016). Mechanistic investigation of industrial wastewater naphthenic acids removal using granular activated carbon (GAC) biofilm based processes. Sci. Total Environ. 541, 238–246. doi: 10.1016/j.scitotenv.2015.09.091
Ji, S.-S., Ren, Y., Buekens, A., Chen, T., Lu, S.-Y., Cen, K.-F., et al. (2014). Treating PCDD/Fs by combined catalysis and activated carbon adsorption. Chemosphere 102, 31–36. doi: 10.1016/j.chemosphere.2013.12.008
Kang, K.-M., Kim, H.-W., Shim, I.-W., and Kwak, H.-Y. (2011). Catalytic test of supported Ni catalysts with core/shell structure for dry reforming of methane. Fuel Process. Technol. 92, 1236–1243. doi: 10.1016/j.fuproc.2011.02.007
Kaštánek, F., Demnerova, K., Pazlarova, J., Burkhard, J., and Maleterova, Y. (1999). Biodegradation of polychlorinated biphenyls and volatile chlorinated hydrocarbons in contaminated soils and ground water in field condition. Int. Biodeterior. Biodegradat. 44, 39–47. doi: 10.1016/S0964-8305(99)00051-7
Kawashima, A., Watanabe, S., Iwakiri, R., and Honda, K. (2009). Removal of dioxins and dioxin-like PCBs from fish oil by countercurrent supercritical CO2 extraction and activated carbon treatment. Chemosphere 75, 788–794. doi: 10.1016/j.chemosphere.2008.12.057
Khoddami, A., Wilkes, M. A., and Roberts, T. H. (2013). Techniques for analysis of plant phenolic compounds. Molecules 18, 2328–2375. doi: 10.3390/molecules18022328
Kim, B.-H., Lee, S., Maken, S., Song, H.-J., Park, J.-W., and Min, B. (2007). Removal characteristics of PCDDs/Fs from municipal solid waste incinerator by dual bag filter (DBF) system. Fuel 86, 813–819. doi: 10.1016/j.fuel.2006.09.007
Kim, M., Oh, I., and Kim, J. (2015). Supercapacitive behavior depending on the mesopore size of three-dimensional micro-, meso-and macroporous silicon carbide for supercapacitors. Phys. Chem. Chem. Phys. 17, 4424–4433. doi: 10.1039/C4CP05357E
Kim, T. Y., Jin, H. J., Park, S. S., Kim, S. J., and Cho, S. Y. (2008). Adsorption equilibrium of copper ion and phenol by powdered activated carbon, alginate bead and alginate-activated carbon bead. J. Ind. Eng. Chem. 14, 714–719. doi: 10.1016/j.jiec.2008.07.004
Kjellerup, B., and Edwards, S. (2013). Application of Biofilm Covered Activated Carbon Particles as a Microbial Inoculum Delivery System for Enhanced Bioaugmentation of PCBs in Contaminated Sediment. Technical Report, SERDP Project ER-2135.
Kjellerup, B., Naff, C., Edwards, S., Ghosh, U., Baker, J., and Sowers, K. (2014). Effects of activated carbon on reductive dechlorination of PCBs by organohalide respiring bacteria indigenous to sediments. Water Res. 52, 1–10. doi: 10.1016/j.watres.2013.12.030
Kogevinas, M. (2001). Human health effects of dioxins: cancer, reproductive and endocrine system effects. Hum. Reprod Update 7, 331–339. doi: 10.1111/j.1600-0463.2001.tb05771.x
Köhler, A., Hellweg, S., Escher, B. I., and Hungerbühler, K. (2006). Organic pollutant removal versus toxicity reduction in industrial wastewater treatment: the example of wastewater from fluorescent whitening agent production. Environ. Sci. Technol. 40, 3395–3401. doi: 10.1021/es060555f
Kolb, F. R., and Wilderer, P. A. (1997). Activated carbon sequencing batch biofilm reactor to treat industrial wastewater. Water Sci. Technol. 35, 169–176. doi: 10.2166/wst.1997.0039
Korte, N. E., West, O. R., Liang, L., Gu, B., Zutman, J. L., and Fernando, Q. (2002). The effect of solvent concentration on the use of palladized-iron for the step-wise dechlorination of polychlorinated biphenyls in soil extracts. Waste Manage. 22, 343–349. doi: 10.1016/S0956-053X(01)00050-2
Krumins, V., Park, J.-W., Son, E.-K., Rodenburg, L. A., Kerkhof, L. J., Häggblom, M. M., et al. (2009). PCB dechlorination enhancement in Anacostia River sediment microcosms. Water Res. 43, 4549–4558. doi: 10.1016/j.watres.2009.08.003
Kulkarni, P., Crespo, J., and Afonso, C. (2008). Dioxins sources and current remediation technologies—a review. Environ. Int. 34, 139–153. doi: 10.1016/j.envint.2007.07.009
Kumar, A., Bisht, B. S., Joshi, V. D., and Dhewa, T. (2011). Review on bioremediation of polluted environment: a management tool. Int. J Environ. Sci. 1:1079.
Lallas, P. L. (2001). The Stockholm Convention on persistent organic pollutants. Am. J. Int. Law 95, 692–708. doi: 10.2307/2668517
Le, T. T., Nguyen, K. H., Jeon, J. R., Francis, A. J., and Chang, Y. S. (2015). Nano/bio treatment of polychlorinated biphenyls with evaluation of comparative toxicity. J. Hazard. Mater. 287, 335–341. doi: 10.1016/j.jhazmat.2015.02.001
Lee, C. L., and Jou, C. J. G. (2012). Integrating fluidized copper/iron bimetal nanoparticles and microwave irradiation for treating chlorobenzene in aqueous solution. Environ. Pollut. 1, 159. doi: 10.5539/ep.v1n2p159
Lee, I., and Fletcher, J. S. (1992). Involvement of mixed function oxidase systems in polychlorinated biphenyl metabolism by plant cells. Plant Cell Reports 11, 97–100. doi: 10.1007/BF00235262
Lens, P., Virkutyte, J., Jegatheesan, V., and Al-Abed, S. (2013). Nanotechnology for Water and Wastewater Treatment. Iwa Publishing.
Li, G., Xiong, J., Wong, P. K., and An, T. (2016). Enhancing tetrabromobisphenol A biodegradation in river sediment microcosms and understanding the corresponding microbial community. Environ. Pollut. 208, 796–802. doi: 10.1016/j.envpol.2015.11.001
Li, Y.-H., Wang, S., Luan, Z., Ding, J., Xu, C., and Wu, D. (2003). Adsorption of cadmium (II) from aqueous solution by surface oxidized carbon nanotubes. Carbon 41, 1057–1062. doi: 10.1016/S0008-6223(02)00440-2
Liou, Y. H., Lo, S. L., Lin, C. J., Kuan, W. H., and Weng, S. C. (2005). Chemical reduction of an unbuffered nitrate solution using catalyzed and uncatalyzed nanoscale iron particles. J. Hazard. Mater. 127, 102–110. doi: 10.1016/j.jhazmat.2005.06.029
Liu, J., Chen, T., Qi, Z., Yan, J., Buekens, A., and Li, X. (2014). Thermal desorption of PCBs from contaminated soil using nano zerovalent iron. Environ. Sci. Pollut. Res. 21, 12739–12746. doi: 10.1007/s11356-014-3226-8
Liu, X., and Yu, G. (2006). Combined effect of microwave and activated carbon on the remediation of polychlorinated biphenyl-contaminated soil. Chemosphere 63, 228–235. doi: 10.1016/j.chemosphere.2005.08.030
Liu, X., Yu, G., and Han, W. (2007). Granular activated carbon adsorption and microwave regeneration for the treatment of 2, 4, 5-trichlorobiphenyl in simulated soil-washing solution. J. Hazard. Mater. 147, 746–751. doi: 10.1016/j.jhazmat.2007.01.076
Liu, Z., and Zhang, F.-S. (2010). Nano-zerovalent iron contained porous carbons developed from waste biomass for the adsorption and dechlorination of PCBs. Bioresour. Technol. 101, 2562–2564. doi: 10.1016/j.biortech.2009.11.074
Long, Y. Y., Zhang, C., Du, Y., Tao, X. Q., and Shen, D. S. (2014). Enhanced reductive dechlorination of polychlorinated biphenyl-contaminated soil by in-vessel anaerobic composting with zero-valent iron. Environ. Sci. Pollut. Res. 21, 4783–4792. doi: 10.1007/s11356-013-2420-4
Lu, J.-Y., Du, X., and Lipscomb, G. (2009). “Cleaning membranes with focused ultrasound beams for drinking water treatment,” in Ultrasonics Symposium (IUS), 2009 IEEE International (Rome: IEEE), 1195–1198.
Lu, Y., and Weavers, L. K. (2002). Sonochemical desorption and destruction of 4-chlorobiphenyl from synthetic sediments. Environ. Sci. Technol. 36, 232–237. doi: 10.1021/es010641+
Macek, T., Mackova, M., and Káš, J. (2000). Exploitation of plants for the removal of organics in environmental remediation. Biotechnol. Adv. 18, 23–34. doi: 10.1016/S0734-9750(99)00034-8
Mackay, D., Paterson, S., and Shiu, W. (1992). Generic models for evaluating the regional fate of chemicals. Chemosphere 24, 695–717. doi: 10.1016/0045-6535(92)90531-U
Mangun, C. L., Benak, K. R., Economy, J., and Foster, K. L. (2001). Surface chemistry, pore sizes and adsorption properties of activated carbon fibers and precursors treated with ammonia. Carbon 39, 1809–1820. doi: 10.1016/S0008-6223(00)00319-5
Marrone, P. A., Hodes, M., Smith, K. A., and Tester, J. W. (2004). Salt precipitation and scale control in supercritical water oxidation—part B: commercial/full-scale applications. J. Supercrit. Fluids 29, 289–312. doi: 10.1016/S0896-8446(03)00092-5
Marulanda, V., and Bolaños, G. (2010). Supercritical water oxidation of a heavily PCB-contaminated mineral transformer oil: laboratory-scale data and economic assessment. J. Supercrit. Fluids 54, 258–265. doi: 10.1016/j.supflu.2010.04.008
Matheson, L. J., and Tratnyek, P. G. (1994). Reductive dehalogenation of chlorinated methanes by iron metal. Environ. Sci. Technol. 28, 2045–2053. doi: 10.1021/es00061a012
May, H. D., Miller, G. S., Kjellerup, B. V., and Sowers, K. R. (2008). Dehalorespiration with polychlorinated biphenyls by an anaerobic ultramicrobacterium. Appl. Environ. Microbiol. 74, 2089–2094. doi: 10.1128/AEM.01450-07
McFarland, V. A., and Clarke, J. U. (1989). Environmental occurrence, abundance, and potential toxicity of polychlorinated biphenyl congeners: considerations for a congener-specific analysis. Environ. Health Perspect. 81, 225. doi: 10.1289/ehp.8981225
Meagher, R. B. (2000). Phytoremediation of toxic elemental and organic pollutants. Curr. Opin. Plant Biol. 3, 153–162. doi: 10.1016/S1369-5266(99)00054-0
Meggo, R. E., Schnoor, J. L., and Hu, D. (2013). Dechlorination of PCBs in the rhizosphere of switchgrass and poplar. Environ. Pollut. 178, 312–321. doi: 10.1016/j.envpol.2013.02.035
Merkel, T., Graeber, M., and Pagel, L. (1999). A new technology for fluidic microsystems based on PCB technology. Sens. Actuators A Phys. 77, 98–105. doi: 10.1016/S0924-4247(99)00062-X
Mitoma, Y., Uda, T., and Egashira, N. (2004). Approach to highly efficient dechlorination of PCDDs, PCDFs, and coplanar PCBs using metallic calcium in ethanol under atmospheric pressure at room temperature. Environ. Sci. Technol. 38, 1216–1220. doi: 10.1021/es034379b
Naddeo, V., Rizzo, L., and Belgiorno, V. (2011). Water, Wastewater and Soil Treatment by Advanced Oxidation Processes. ASTER.
Nah, I. W., Hwang, K.-Y., and Shul, Y.-G. (2008). Effect of metal and glycol on mechanochemical dechlorination of polychlorinated biphenyls (PCBs). Chemosphere 73, 138–141. doi: 10.1016/j.chemosphere.2008.04.051
Nowack, B. (2008). Pollution prevention and treatment using nanotechnology. Nanotechnology 2, 1–15. doi: 10.1002/9783527628155.nanotech010
Nur Ismayuslini, S. (2010). Degradation of Phenol by Ultrasonic Irradiation in Addition of Salt. Universiti Malaysia Pahang.
Nurmi, J. T., Tratnyek, P. G., Sarathy, V., Baer, D. R., Amonette, J. E., Pecher, K., et al. (2005). Characterization and properties of metallic iron nanoparticles: spectroscopy, electrochemistry, and kinetics. Environ. Sci. Technol. 39, 1221–1230. doi: 10.1021/es049190u
O'carroll, D., Sleep, B., Krol, M., Boparai, H., and Kocur, C. (2013). Nanoscale zero valent iron and bimetallic particles for contaminated site remediation. Adv. Water Resour. 51 104–122. doi: 10.1016/j.advwatres.2012.02.005
Okuno, H., Yim, B., Mizukoshi, Y., Nagata, Y., and Maeda, Y. (2000). Sonolytic degradation of hazardous organic compounds in aqueous solution. Ultrason. Sonochem. 7, 261–264. doi: 10.1016/S1350-4177(00)00053-5
Ottosen, L. M., Hansen, H. K., Laursen, S., and Villumsen, A. (1997). Electrodialytic remediation of soil polluted with copper from wood preservation industry. Environ. Sci. Technol. 31, 1711–1715. doi: 10.1021/es9605883
Park, J.-W., Krumins, V., Kjellerup, B. V., Fennell, D. E., Rodenburg, L. A., Sowers, K. R., et al. (2011). The effect of co-substrate activation on indigenous and bioaugmented PCB dechlorinating bacterial communities in sediment microcosms. Appl. Microbiol. Biotechnol. 89, 2005–2017. doi: 10.1007/s00253-010-2958-8
Passatore, L., Rossetti, S., Juwarkar, A. A., and Massacci, A. (2014). Phytoremediation and bioremediation of polychlorinated biphenyls (PCBs): state of knowledge and research perspectives. J. Hazard. Mater. 278, 189–202. doi: 10.1016/j.jhazmat.2014.05.051
Patel, U. D., and Suresh, S. (2007). Dechlorination of chlorophenols using magnesium–palladium bimetallic system. J. Hazard. Mater. 147. doi: 10.1016/j.jhazmat.2007.01.029
Payne, R. B., May, H. D., and Sowers, K. R. (2011). Enhanced reductive dechlorination of polychlorinated biphenyl impacted sediment by bioaugmentation with a dehalorespiring bacterium. Environ. Sci. Technol. 45, 8772–8779. doi: 10.1021/es201553c
Pivetz, B. E. (2001). Phytoremediation of Contaminated Soil and Ground Water at Hazardous Waste Sites. United States Environmental Protection Agency, Office of Research and Development, Office of Solid Waste and Emergency Response: Superfund Technology Support Center for Ground Water, National Risk Management Research Laboratory, Subsurface Protection and Remediation Division, Robert S. Kerr Environmental Research Center.
Quensen Iii, J. F., Tiedje, J. M., and Boyd, S. A. (1988). Reductive dechlorination of polychlorinated biphenyls by anaerobic microorganisms from sediments. Science 242, 752–754. doi: 10.1126/science.242.4879.752
Rahuman, M. S. M. M., Pistone, L., Trifirò, F., and Miertus, S. (2000). “Destruction technologies for polychlorinated biphenyls (PCBs),” in Proceedings of Expert Group Meetings on POPs and Pesticides Contamination (Bologna), 10–12.
Rakowska, M. I., Kupryianchyk, D., Harmsen, J., Grotenhuis, T., and Koelmans, A. A. (2012). In situ remediation of contaminated sediments using carbonaceous materials. Environ. Toxicol. Chem. 31, 693–704. doi: 10.1002/etc.1763
Rodríguez, J. G., and Lafuente, A. (2008). Effective elimination of PCBs catalyzed by the palladium/hydrazine system as an ecological sustainable process. Ind. Eng. Chem. Res. 47, 7993–7996. doi: 10.1021/ie800110p
Ryoo, K.-S., Byun, S.-H., Choi, J.-H., Hong, Y.-P., Ryu, Y. T., Song, J. S., et al. (2007). Destruction and removal of PCBs in waste transformer oil by a chemical dechlorination process Bull. Korean Chem. Soc. 28, 520–528. doi: 10.5012/bkcs.2007.28.4.520
Sakrittichai, P. (2001). Dechlorination of p-Chlorophenol on a Palladium Based Metal Support Catalyst in a Microreactor: Experiment and Theory. thesis, Master of Science.
Schecter, A., Birnbaum, L., Ryan, J. J., and Constable, J. D. (2006). Dioxins: an overview. Environ. Res. 101, 419–428. doi: 10.1016/j.envres.2005.12.003
Schreier, C. G., and Reinhard, M. (1994). Transformation of chlorinated organic compounds by iron and manganese powders in buffered water and in landfill leachate. Chemosphere 29, 1743–1753. doi: 10.1016/0045-6535(94)90320-4
Sharma, J. K., Gautam, R. K., Nanekar, S. V., Weber, R., Singh, B. K., Singh, S. K., et al. (2018). Advances and perspective in bioremediation of polychlorinated biphenyl-contaminated soils. Environ. Sci. Pollut. Res. 25, 16355–16375. doi: 10.1007/s11356-017-8995-4
Singh, R., Misra, V., and Singh, R. P. (2012). Removal of Cr (VI) by nanoscale zero-valent iron (nZVI) from soil contaminated with tannery wastes. Bull. Environ. Contam. Toxicol. 88, 210–214. doi: 10.1007/s00128-011-0425-6
Slater, H., Gouin, T., and Leigh, M. B. (2011). Assessing the potential for rhizoremediation of PCB contaminated soils in northern regions using native tree species. Chemosphere 84, 199–206. doi: 10.1016/j.chemosphere.2011.04.058
Sowers, K. R., and May, H. D. (2013). In situ treatment of PCBs by anaerobic microbial dechlorination in aquatic sediment: are we there yet? Curr. Opin. Biotechnol. 24, 482–488. doi: 10.1016/j.copbio.2012.10.004
Tammaro, M., Salluzzo, A., Perfetto, R., and Lancia, A. (2014). A comparative evaluation of biological activated carbon and activated sludge processes for the treatment of tannery wastewater. J. Environ. Chem. Eng. 2, 1445–1455. doi: 10.1016/j.jece.2014.07.004
Tanabe, S. (1988). PCB problems in the future: foresight from current knowledge. Environ. Pollut. 50, 5–28. doi: 10.1016/0269-7491(88)90183-2
Tu, C., Teng, Y., Luo, Y., Sun, X., Deng, S., Li, Z., et al. (2011). PCB removal, soil enzyme activities, and microbial community structures during the phytoremediation by alfalfa in field soils. J. Soils Sediments 11, 649–656. doi: 10.1007/s11368-011-0344-5
Tyagi, M., Da Fonseca, M. M. R., and De Carvalho, C. C. (2011). Bioaugmentation and biostimulation strategies to improve the effectiveness of bioremediation processes. Biodegradation 22, 231–241. doi: 10.1007/s10532-010-9394-4
Urban, J. D., Wikoff, D. S., Bunch, A. T., Harris, M. A., and Haws, L. C. (2014). A review of background dioxin concentrations in urban/suburban and rural soils across the United States: implications for site assessments and the establishment of soil cleanup levels. Sci. Total Environ. 466, 586–597. doi: 10.1016/j.scitotenv.2013.07.065
Vasilyeva, G. K., Strijakova, E. R., Nikolaeva, S. N., Lebedev, A. T., and Shea, P. J. (2010). Dynamics of PCB removal and detoxification in historically contaminated soils amended with activated carbon. Environ. Pollut. 158, 770–777. doi: 10.1016/j.envpol.2009.10.010
Van den Berg, M., Birnbaum, L. S., Denison, M., De Vito, M., Farland, W., Feeley, M., et al. (2006). The 2005 World Health Organization reevaluation of human and mammalian toxic equivalency factors for dioxins and dioxin-like compounds. Toxicol. Sci. 93, 223–241. doi: 10.1093/toxsci/kfl055
Van Dort, H. M., Smullen, L. A., May, R. J., and Bedard, D. L. (1997). Priming microbial meta-dechlorination of polychlorinated biphenyls that have persisted in Housatonic River sediments for decades. Environ. Sci. Technol. 31, 3300–3307. doi: 10.1021/es970347a
Van Gerven, T., Geysen, D., and Vandecasteele, C. (2004). Estimation of the contribution of a municipal waste incinerator to the overall emission and human intake of PCBs in Wilrijk, Flanders. Chemosphere 54, 1303–1308. doi: 10.1016/S0045-6535(03)00233-9
Varanasi, P., Fullana, A., and Sidhu, S. (2007). Remediation of PCB contaminated soils using iron nano-particles. Chemosphere 66, 1031–1038. doi: 10.1016/j.chemosphere.2006.07.036
Venkatachalam, K., Arzuaga, X., Chopra, N., Gavalas, V. G., Xu, J., Bhattacharyya, D., et al. (2008). Reductive dechlorination of 3, 3′, 4, 4′-tetrachlorobiphenyl (PCB77) using palladium or palladium/iron nanoparticles and assessment of the reduction in toxic potency in vascular endothelial cells. J. Hazard. Mater. 159, 483–491. doi: 10.1016/j.jhazmat.2008.02.109
Vidali, M. (2001). Bioremediation. an overview. Pure Appl. Chem. 73, 1163–1172. doi: 10.1351/pac200173071163
Weber, R. (2007). Relevance of PCDD/PCDF formation for the evaluation of POPs destruction technologies–review on current status and assessment gaps. Chemosphere 67, S109–S117. doi: 10.1016/j.chemosphere.2006.05.094
Weber, R., Yoshida, S., and Miwa, K. (2002). PCB destruction in subcritical and supercritical water evaluation of PCDF formation and initial steps of degradation mechanisms. Environ. Sci. Technol. 36, 1839–1844. doi: 10.1021/es0113910
Wu, B.-Z., Chen, H.-Y., Wang, S. J., Wai, C. M., Liao, W., and Chiu, K. (2012). Reductive dechlorination for remediation of polychlorinated biphenyls. Chemosphere 88, 757–768. doi: 10.1016/j.chemosphere.2012.03.056
Wyrwicka, A., Steffani, S., and Urbaniak, M. (2014). The effect of PCB-contaminated sewage sludge and sediment on metabolism of cucumber plants (Cucumis sativus). Ecohydrol. Hydrobiol. 14, 75–82. doi: 10.1016/j.ecohyd.2014.01.003
Xu, J., and Bhattacharyya, D. (2007). Fe/Pd nanoparticle immobilization in microfiltration membrane pores: synthesis, characterization, and application in the dechlorination of polychlorinated biphenyls. Ind. Eng. Chem. Res. 46, 2348–2359. doi: 10.1021/ie0611498
Yang, B., Deng, S., Yu, G., Zhang, H., Wu, J., and Zhuo, Q. (2011). Bimetallic Pd/Al particles for highly efficient hydrodechlorination of 2-chlorobiphenyl in acidic aqueous solution. J. Hazard. Mater. 189, 76–83. doi: 10.1016/j.jhazmat.2011.02.001
Yu, H., Feng, C., Liu, X., Yi, X., Ren, Y., and Wei, C. (2016). Enhanced anaerobic dechlorination of polychlorinated biphenyl in sediments by bioanode stimulation. Environ .Pollut. 211, 81–89. doi: 10.1016/j.envpol.2015.12.039
Zhang, F.-L., Yang, X.-J., Xue, X.-L., Tao, X.-Q., Lu, G.-N., and Dang, Z. (2013). Estimation of n-octanol/water partition coefficients (log) of polychlorinated biphenyls by using quantum chemical descriptors and partial least squares. J. Chem. 2013:740548. doi: 10.1155/2013/740548
Zhuang, Y., Ahn, S., Seyfferth, A. L., Masue-Slowey, Y., Fendorf, S., and Luthy, R. G. (2011). Dehalogenation of polybrominated diphenyl ethers and polychlorinated biphenyl by bimetallic, impregnated, and nanoscale zerovalent iron. Environ. Sci. Technol. 45, 4896–4903. doi: 10.1021/es104312h
Keywords: bioremediation, dehalogenation, ex-situ, in-situ, polychlorinated biphenyls (PCBs), remediation technologies
Citation: Jing R, Fusi S and Kjellerup BV (2018) Remediation of Polychlorinated Biphenyls (PCBs) in Contaminated Soils and Sediment: State of Knowledge and Perspectives. Front. Environ. Sci. 6:79. doi: 10.3389/fenvs.2018.00079
Received: 13 April 2018; Accepted: 26 June 2018;
Published: 17 July 2018.
Edited by:
Aziz Ullah, Kohat University of Science and Technology, PakistanReviewed by:
George Prpich, University of Virginia, United StatesPierre-Marie Badot, University of Franche-Comté, France
Copyright © 2018 Jing, Fusi and Kjellerup. This is an open-access article distributed under the terms of the Creative Commons Attribution License (CC BY). The use, distribution or reproduction in other forums is permitted, provided the original author(s) and the copyright owner(s) are credited and that the original publication in this journal is cited, in accordance with accepted academic practice. No use, distribution or reproduction is permitted which does not comply with these terms.
*Correspondence: Birthe V. Kjellerup, bvk@umd.edu