- 1Bioenergy Systems Department, DBFZ Deutsches Biomasseforschungszentrum (German Biomass Research Centre), Leipzig, Germany
- 2Department of Bioenergy, Helmholtz Centre for Environmental Research – UFZ, Leipzig, Germany
- 3Chair Bioenergy Systems, Institute for Infrastructure and Resources Management, Leipzig University, Leipzig, Germany
In 2019, the German government agreed on a Climate Protection Program intended to deliver its 2030 climate targets. Concrete measures, such as a carbon price, will be put in place as early as 2021. But how to plan beyond 2030? Scenarios can be powerful tools to envision the world in 20, 30, or 50 years, to describe pathways toward different visions of the future, and ultimately to investigate technology portfolios and policy options against their performance toward the achievement of a decarbonized future. This is why scenarios are especially popular with energy and climate scholars. In particular, scenarios with biomass-based carbon removal options (BCO2) can help to highlight how we may reach a net negative emission world. Hence, in this study, 66 energy and decarbonization scenario studies are systematically reviewed for Germany from the years 2002 to 2019 to assess how inclusive they are with regard to BCO2 concepts. The portfolio of BCO2 concepts within those scenarios is studied over time and a qualitative analysis of the scenario documentation is performed to identify the rationales for their inclusion or exclusion. The results indicate “blind spots” of the scenarios with regard to bioeconomy aspects, as biomass for material use is only sparsely covered. Likewise, only about 10% of the studies provide a framework for land use changes and corresponding emission accounting to adequately represent biomass-based negative emission technologies (NETs) in their assessments. The analysis for carbon capture and storage (CCS) further reveals the necessity of revisiting the public acceptance argument which has previously served so far for many studies as the ultimate, though not well-grounded deal-breaker. Based on the detected gaps and shortcomings in the current German scenario landscape, recommendations for a more transparent and holistic representation of BCO2 in the scenario framework are given.
Introduction
With the Paris Agreement of 2015, the international community of states has set itself stringent targets for the reduction of greenhouse gas (GHG) emissions. However, the agreement does not prescribe any specific measures—this is at the discretion of the respective states. In Germany, the federal government adopted the Climate Action Plan 2050 in November 2016, specifying its implementation strategy on national level: a long-term goal of GHG neutrality by 2050 and an intermediate target of at least 55 percent GHG emission reduction compared to 1990 by 2030. With its Climate Protection Program published in 2019, the German government further reaffirmed this ambition by outlining strategic policy ideas to reach the 2030 climate targets.
Energy and decarbonization scenarios can help to investigate technology options and regulatory measures that can make significant contributions to the achievement of these targets. Although scenarios are not projections or predictions of the future, they are internally coherent narratives that describe pathways toward different visions of the future. Due to their explorative character, energy and decarbonization scenarios should be as technology-open as possible, incorporating innovative concepts and emerging technologies.
A set of such technologies is Carbon Dioxide Removal (CDR), i.e., technologies and processes that absorb CO2 from the atmosphere. If the carbon thus obtained is not used but sequestered, then this concept is referred to as Negative Emission Technologies (NETs) due to their ability of removing more CO2 from the atmosphere over their entire lifecycle than emitting CO2, thus being net negative in their emissions balance. The most recent special report of the Intergovernmental Panel on Climate Change (IPCC) on the impacts of global warming of 1.5°C above pre-industrial levels (SR1.5) acknowledges the important role of NETs for stabilizing atmospheric CO2 concentrations (IPCC., 2018). However, most Integrated Assessment Models (IAMs) that submitted scenarios for consideration in the SR1.5 database are limited in their analysis to two NETs, namely Bioenergy with Carbon Capture and Storage (BECCS)1 and afforestation/reforestation. In fact, 9 out of 10 IAMs that successfully submitted scenarios explicitly model BECCS; 7 out of 10 consider afforestation/reforestation. In contrast, only two modeling frameworks directly address both Direct Air Capture and Storage (DACS) and Soil Carbon Sequestration (SCS). Restoration of wetlands and biochar are—if at all—only assessed implicitly and in an exogenous manner, i.e., they are not represented in any of these models but can be explored through comparison of multiple alternative scenarios. Similarly, ocean fertilization and enhanced weathering are not represented in any IAM reported by Forster et al. (2018).
To better understand potential challenges and synergies of biomass-based NETs, various scholars conducted more detailed analyses on subsets of NETs. In this regard, “biomass-based negative emissions” (Heck et al., 2018) or similar concepts such as “plant-based CDR” (Lenton, 2014a), “biomass-based CDR” (Turner et al., 2018), “land-based biological CO2 removal” (Lenton, 2014b), and also “terrestrial CDR (tCDR)” (Boysen et al., 2017) have been proposed for more refined assessments focusing mainly on BECCS, biochar, standing biomass, and to a lesser extent on biomass burial. Similarly, “natural climate solutions” (Griscom et al., 2017; Fargione et al., 2018) were assessed which consist in a set of 20 improved land management actions that increase the carbon sink function of forests, wetlands, grasslands, and agricultural lands. All these concepts compete for available land and available biomass. In fact, pathways for limiting global warming to 1.5°C might require the area of up to 12 million km2–representing the size of the entire European continent—to increase forest coverage in 2050 compared to 2010 levels (IPCC, 2019).
However, biomass-based NETs are not the only contenders for land and biomass. Food production, renewable energy provision, and some industries that rely on biomaterials also compete for these scarce resources. Therefore, a holistic approach is needed to address all biomass-based CO2 emissions reduction options (hereafter referred to as BCO2 concepts) that either (a) rely on the energetic or material use of biomass, (b) substitute fossil carbon sources and products through biogenic CO2, or (c) provide negative emissions based on biomass and land resources (Figure 1). Capturing the range of these concepts as comprehensively as possible in energy and decarbonization scenarios serves two main purposes: on the one hand, to assess their CO2 reduction and negative emissions potentials, and on the other hand, to identify possible competition for land and biomass use.
Taking Germany as case study, a structured review is carried out for 66 energy and decarbonization scenario studies from the years 2002 to 2019 to assess how inclusive they are with regard to BCO2 concepts. The evolution of the portfolio of BCO2 concepts within those scenarios is studied over time and a qualitative analysis of the scenario documentations is performed to identify the rationales for their (non-)inclusion. Furthermore, gaps in the scenario coverage of BCO2 options are addressed.
Methodology
In times of rapidly expanding scientific knowledge, the systematic review of published research serves multiple purposes at once: identifying and synthesizing the vast amount of studies to assess the state of science in a given field of knowledge and steer further progress in that field. By combining the results of relevant studies in a structured and transparent manner, this can further enhance the credibility of scientific assessments in general and allow for a more robust communication toward policymakers (Petticrew and McCartney, 2011; Minx et al., 2017).
In the more specific case of reviewing energy scenario studies, a systematic review can provide insights into the design of the future energy mix, CO2 mitigation options and driving factors for change. In addition, comparatively assessing scenario studies, their assumptions, and results enables highlighting system interdependences and evaluating different policy options. In fact, energy system and climate scholars regularly use scenario instruments to investigate possible future developments. By formulating fundamentally different assumptions for the future, scenarios can create awareness for uncertainties, opportunities, and risks associated with each of these possible futures.
For this study, a comprehensive list of German energy and decarbonization scenarios was established based on (a) study compilations of previous meta-analyses (Kronenberg et al., 2011; Haller et al., 2016; Peter et al., 2017; Szarka et al., 2017; Runkel, 2018; Samadi et al., 2019), (b) studies identified via scientific journals using Scopus and Web of Science, and (c) gray literature found via Google. Additionally, the reference lists of all identified documents were screened for collecting further scenario studies. The following word combinations were used as search terms, in both English and German: German* AND scenario* AND energy and climate change related terms (“energy system,” “energy transition,” “decarbonization,” “climate change mitigation”) AND the time horizons 2020–2100 in 5 year increments. Cutoff date for considering studies was November 2019, i.e., when performing the literature research. To qualify for the assessment, all scenario studies had to fulfill the following criteria:
• Explicit focus on either the energy transition (“Energiewende”), a 100% renewable energy system, or ambitious decarbonization and GHG reduction targets;
• Coverage of at least two of the three energy sectors, i.e., power, heat, and transport, thus excluding sector-specific studies;
• Quantification and/or modeling of the scenarios, therefore excluding Del phi surveys (BDEW, 2016) and expert elicitation of future trends and policy measures (BMU, 2016; Renn, 2017) for comparability reasons.
A catalog of the identified studies is shown in Table 1 with key information on their respective year of publication, source of funding, investigated scenarios and time horizon.
For the analysis, all scenario-based studies were screened for biomass-related carbon removal options as conceptualized in Figure 1. Both qualitative and quantitative mentions of all BCO2 concepts were gathered. Information was collected on whether they were (a) mentioned at all, (b) excluded on purpose, (c) considered within the scenario narratives, and/or (d) quantitatively assessed as part of the scenario modeling.
Additionally, with respect to the energetic and material use of biomass, all studies were screened for their biomass potentials, sectoral coverage of biomass use, imports, and sustainability considerations. For the energetic and material use of biogenic CO2, data was systematically gathered on CO2 supply volumes from both biogenic and other sources, as well as on CO2 utilization pathways in the energy and industrial sectors. The BCO2-focused analysis extends to non-biogenic CO2 sources as the latter ones can be used interchangeably in subsequent CO2 utilization applications. Furthermore, alongside information on BECCS, data was also gathered on fossil carbon capture and storage (CCS), industrial CCS and direct air capture with carbon storage (DACCS) as their concepts are similar to BECCS in terms of infrastructure requirements and geological storage. This was further combined with the collection of arguments within the studies for/against the use of CCS. Finally, references to other biomass-based NETs (i.e., afforestation/reforestation, biochar, soil carbon sequestration, and ecosystem restoration) were collected.
Results and Discussion
Publication Trends in German Energy and Decarbonization Scenarios
After the thorough scenario study collection and selection according to the criteria set out in the previous section, 66 relevant studies were identified, published in the years from 2002 to 2019 and containing a total of 189 scenarios. Interest in scenario studies started to increase notably around 2010, doubling in numbers until 2015 and tripling until today (Figure 2). Given the studies' time horizons, three different waves of studies can be identified: Those addressing the year 2020 leveled out after 2010. A similar dynamic can be observed right now for scenarios running up to 2030. This confirms that scenarios serve long-term planning purposes, with more than 10–15 years lead time. The third observable pattern is a rapid increase of studies addressing mid-century with scenarios running up to 2050. This is also in line with the time horizons of most global assessments, including the IPCC SR1.5 report that highlights that carbon neutrality should be achieved around this time for a 1.5°C consistent pathway (IPCC., 2018).
When looking at the source of funding for the 66 analyzed studies, the results reveal that the majority of funding bodies are ministries or governmental agencies, representing 52% of the study sample. An additional 26% comes from industry bodies and companies. Non-governmental organizations (NGOs), think tanks and foundations funded around 12% of the studies and research institutions another 11%. The large share of funding from the political realm and industry confirms their interest in scenarios as a tool for assessing long-term policy options and measures. Therefore, the scenarios should be as technology-open as possible and not confined to a specific sub-set of technologies. As part of a broader climate change mitigation portfolio, BCO2 concepts should be assessed from a systems and scenario perspective first, before ultimately deciding on desired futures and technologies.
Unbalanced Representation of Energetic and Material Use of Biomass
Bioenergy is contributing to fossil fuel replacement, potentially achieving zero to near-zero emissions in the areas of its application. This is due to its carbon intake during plant growth i.e., CO2 capture from the atmosphere through photosynthesis. In contrast, biomass from a material use perspective contributes to emission reductions by substituting either fossil fuel-based products (e.g., cotton products instead of polyester) or otherwise energy-intensive products (e.g., wooden building materials instead of concrete or steel). In both cases, bioenergy's potential to offset emissions compared to fossil fuels has to be determined via a full life-cycle analysis (LCA) (Cherubini and Strømman, 2011).
Regarding the scenario coverage of biomass for energetic and material use, all 66 studies deal to some extent with bioenergy in their scenarios. As such, it is the only BCO2 category that is addressed—and most often also quantified—in terms of GHG emission reductions within all the scenarios. In contrast, the consideration of non-energy related biomass use is scarce: only 13 out of the 66 studies mention at least once the material use dimension. This picture of unbalanced biomass use representation in the scenarios is further confirmed when assessing the biomass potentials allocated to energetic and material purposes.
With respect to domestic bioenergy potentials, only every third study (n = 24) provides data, resulting in a range of 216–2,300 PJ (Figure 3). This discrepancy by the factor 10 is due to the fact that there is no common methodology for determining either the underlying biomass potential or its attribution to the energy sector. For instance, dating back to the year 2004, a scenario study financed by the Federal Ministry for the Environment (BMU) assessed from a nature conservation point-of-view the land availability for bioenergy crops as well as various biomass waste and residue streams, resulting in a sustainable bioenergy potential of 1,440 PJ (BMU, 2004). Another eight scenario studies subsequently used this publication as basis for their modeling. However, despite this joint reference point, the stated potentials differ due to further assumptions made by the studies individually. On the lower end of the spectrum, studies only rely on biogenic waste and residues as bioenergy carriers, explicitly excluding dedicated energy crops due to sustainability concerns. Taking an even more conservative approach, a study commissioned by the German Environment Agency (UBA) study further divides the biogenic waste and residues by setting aside solid ones, such as straw and scrap wood, for material use, thus reducing the available biomass for energetic purposes even further (UBA, 2010). In contrast, the upper end of the spectrum consists of studies that do not provide any further information as to how their bioenergy potential is composed.
Detailed information about the material use of biomass is even less abundant. Besides the already cited UBA study, only five other studies reserve some biomass for non-energetic purposes, either by limiting the available land for bioenergy production to account for the material supply (BMU, 2015a; BMWi, 2018), by using industrial waste streams directly for industrial products and processes (UBA, 2019b), or by not fully exploiting the technical bioenergy potential, thus leaving a certain share to material use options (BMWi, 2014, 2015).
Biomass imports can extend the available resource base, alleviating to some extent the competition for limited biomass resources. However, the question of imports causes a clear divide within the scenario studies: without stating explicit concerns, a group of eight studies allows imports to supplement the domestic resource availability, in some cases to an extent of up to 900 PJ (BMU, 2014). In contrast, another 16 studies explicitly exclude biomass imports from their scope to avoid direct and/or indirect land use changes abroad that are likely to aggravate food security issues in emerging and developing countries (BMU, 2010, 2012). In addition, they argue that countries with climate mitigation efforts similar to the ones in Germany will tap their own biomass resources, so that relying on imports is not a sustained option in the long term (BMU, 2012).
Challenges in Matching Biogenic and Industrial CO2 Supply and Demand
Similar to biomass that faces the challenge of reconciling various demands with the existing, limited potential, biogenic CO2 is also subject to supply and demand dynamics. On the supply side, biogas upgrading, bioethanol plants, and biomass-to-liquid processes yield relatively pure CO2 side-streams. CO2 capture is also possible in combination with biomass gasification or combustion but comes with a higher energy and efficiency penalty due to a lower CO2 concentration in their exhaust gases. Further adding to the equation, CO2 supply is not only possible through biogenic sources but also captured from fossil-based power stations, industrial plants, and/or directly from the air. However, fossil CO2 sources will not be further discussed in this paper, given that all analyzed studies that deal with carbon capture and utilization (CCU) options exclude fossil sources for CO2 supply. Besides the already mentioned energy penalties, the main reason for this exclusion is linked to carbon accounting issues, as fossil-based CCU only delays net CO2 being released into the atmosphere. On the demand side, CO2 can be an input for various CCU pathways that are further described below.
With regard to potential biogenic CO2 supply volumes, only few scenario studies provide data for the German case. For 2050, estimates suggest an availability of 5.3–11 Mt CO2 from biogas upgrading plants (BMU, 2012; UBA, 2014). Biofuel production may lead to 36 Mt CO2 as side product (WWF, 2009). Other conservative figures indicate a maximum potential of 15–25 Mt CO2 from biogas upgrading, bioethanol production, and stationary bioenergy use combined (UBA, 2014; BMWi, 2018). Based on these studies, the theoretical availability of biogenic CO2 will likely range between 15 and 36 Mt. Assuming 90% CO2 capture rates from bioenergy plants (WWF, 2009), the technical availability is slightly lower. In addition, due to the remote and dispersed location of some of these plants, only up to 70% of this technical potential might be achievable (BMU, 2012). While these figures give a first appraisal of future biogenic CO2 availability, data is sparse and related assumptions are either not stated at all or not in a transparent manner. Therefore, a robust evaluation and comparison of the biogenic CO2 sources and supply volumes cannot be performed. For instance, biogenic CO2 supply is heavily dependent on the assumed biomass potential as well as the biomass allocation within the energy sector. When prioritized as fuel for mobile consumption in the transport sector, a CO2 fraction can be recovered in the biofuel production but not in its end-use application.
Industrial point sources are an alternative CO2 source. In contrast to biogenic CO2, the captured CO2 from industrial processes is not GHG neutral. However, if CO2 emissions are inherent to a given process and therefore unavoidable, for instance in the limestone calcination process, capturing this CO2 for subsequent use or storage is still the best option for climate change mitigation. In integrated steel mills, CO2 emissions mainly occur from iron production in the blast furnace, but also in the coke production step as well as in the sinter plant when preparing the iron ore. For 2050, conservative estimates suggest an availability of 13.8 Mt CO2 from industrial processes, notably but not exclusively within lime and cement production (UBA, 2014). Another study indicates that steel production alone could deliver 31 Mt CO2 in Germany (BMU, 2012). The highest estimate of 93 Mt CO2 from industrial point sources extends the scope to CO2 capture in steel, ammonia, and cement production, as well as refineries and waste incineration (BDI, 2018). As these ballpark figures show, the scope of industries considered for carbon capture strongly influences the potential for industrial CO2 supply. In addition, depending on alternative decarbonization options for the industry sector, the residual emissions and thus the capture volume might differ. According to the official German emission reporting to UNFCCC, CO2 emissions of the entire industry sector amounted to 181 Mt CO2 in 2017, out of which 56 Mt CO2 were caused by the iron and steel industry (UBA, 2019b). However, it has to be noted that these official numbers represent both process and energy-related CO2 emissions and can therefore not be directly compared to the above mentioned scenario estimates on CO2 capture from industrial processes. To avoid blast furnace emissions in the steel production, a hydrogen-based direct reduction plant and subsequent melting of the iron ore in an electric arc furnace could replace the blast furnace conversion route (UBA, 2019a). This would drastically reduce the emissions of the steel sector, thus also reducing the amount of CO2 available for capture, utilization and/or storage. However, the CO2 avoidance costs for this method amount to around € 490/t CO2eq, which is significantly more expensive than CO2 capture combined with either utilization or storage (BDI, 2018). Another uncertainty factor lies in the capture efficiency due to multiple emission streams in some industry sectors. Taking again the steel example, while the blast furnace is the biggest emitter, there are other emission sources in the steel production, namely the coke plant and the sinter plant (BDI, 2018), rendering the implementation of carbon capture more difficult and—with lower CO2 concentrations in these plants—also more expensive, in both cost and energy use.
In contrast to limited biogenic and industrial CO2 sources, direct air capture (DAC) can theoretically deliver an unlimited amount of CO2 captured from the atmosphere. However, none of the studies considers this technology in their mitigation portfolios. The main reasons for excluding it from their assessment are prohibitively high costs and low efficiency rates due to high energy demand (UBA, 2014; BUND, 2015; dena, 2018), especially if renewables are not yet predominant in the energy mix. Nevertheless, DAC is seen as a long-term option in Germany when there is no more untapped potential from biogenic and industrial sources (BMWi, 2018). Interestingly, some studies suggest that direct air capture is more likely to be applied abroad. Assuming that countries with more favorable conditions for renewable energies, i.e., higher solar radiation and/or wind availability than in Germany, would produce synthetic fuels for international export, these countries would incur a considerable need for CO2 to convert the hydrogen produced from renewable electricity to hydrocarbon fuels. With biogenic and/or industrial CO2 sources not necessarily being co-located with the fuel production sites, these countries would rely on DAC to satisfy the CO2 demand (UBA, 2014, 2019b; BDI, 2018).
The reference to synthetic hydrocarbon fuels leads to the question of CO2 utilization options. CO2 can be put to use for both energetic and material purposes. As such, CO2, along with hydrogen, is a building block for platform chemicals, plastics, and synthetic hydrocarbon fuels. Since the production of hydrogen through water electrolysis requires large quantities of electricity, these CCU pathways are also labeled as Power-to-X, whereas X can stand for gas (PtG), liquid fuels (PtL), chemicals (PtC), or other applications. As Figure 4 shows, none of these CO2 utilization concepts are adequately represented within the German scenario studies. Industry CCU, i.e., using CO2 as a feedstock for industrial processes and products (e.g., input for platform chemicals; use as refrigerant gas, solvent, dry ice etc.), is the least mentioned concept. Moreover, only three studies incorporate syngas-based CCU routes it into their assessments which allow to subsequently build-up all major platform chemicals (UBA, 2014, 2019a; dena, 2018). In the long-term, the potential for CO2 utilization as feedstock in the industrial realm in Germany is estimated by one scenario study to be limited to 5 Mt per year in addition to its current use (dena, 2018). In contrast, the energetic use of CO2 is better represented, with PtG being part of the scenario modeling in about 30% of the studies. However, this PtG—and to a lesser extent PtL—integration is not primarily motivated by the prospects of CO2 utilization. In fact, only two studies explicitly indicate the amount of CO2 needed, namely 27 Mt for synthetic methane production in one case (UBA, 2014) and 79 Mt for the production of methanol and dimethyl ether in another case (Hansen et al., 2019). Instead the focus with regard to PtG and PtL lies on their ability to (a) function as temporary storage of excess power from variable renewable energy sources, and (b) provide a renewable energy source for the otherwise difficult to decarbonize sectors, such as aviation and heavy duty trucks. The latter reason is also why various studies consider importing synthetic energy carriers, sometimes even up to 85–100% of the entire projected PtG or PtL consumption (BDI, 2018; BMU, 2019; UBA, 2019b). Shortcomings in CCU scenario modeling therefore are the lack of transparent assumptions and quantified data on CO2 as major input sources for PtX, both from an energy and even more importantly a material use perspective. As a consequence, a matching of domestically available CO2 supply with the potential CO2 demand is not possible.
Costs vs. Public Acceptance: Rationales for and Against (BE)CCS
While BECCS is implemented in almost all IAMs and therefore well-represented in global IPCC scenarios (Forster et al., 2018), the results for the German scenario landscape are much different. Figure 5 shows that BECCS was included in a scenario study for the first time around 2010. In this pioneering study, the modeling framework provided a CCS option for bioethanol and biodiesel production, leading to a scenario of capturing 32 Mt CO2 from biogenic origin in 2050 (WWF, 2009). Within the last 10 years, however, only one further study has assessed and quantified BECCS. Extending the scope to cover both biogas and bioethanol plants (but excluding biodiesel production), the calculations only resulted in 8.5 Mt CO2 captured and stored (BMU, 2014). Nevertheless, the number of studies that explicitly exclude BECCS also remained relatively low: only three studies deliberately decided against this technology option due to (a) general skepticism about CCS (BUND, 2015), (b) various environmental reasons (UBA, 2019b), and (c) a prioritization of biomass for the industrial sector, leaving only a small biomass share to the energy sector so that large-scale BECCS solutions wouldn't be feasible (BDI, 2018).
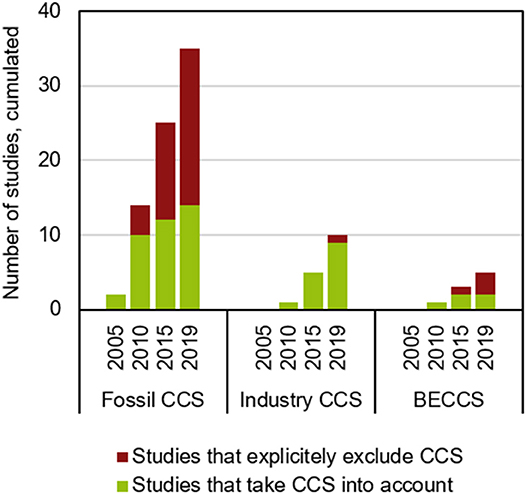
Figure 5. The exclusion of fossil, industrial and biomass-based CCS in German scenario studies over time.
CCS as mitigation option for coal or natural gas fired power stations was largely accepted and implemented until around 2010 but highly disputed afterwards (Figure 5). This strong opposition to fossil CCS might have negatively affected the perception toward CCS in general, causing more precaution as to the adoption of industrial or biomass-based CCS in German modeling frameworks. In fact, although first addressed in 2009, the intensified discussion of industrial CCS is a more recent development in Germany. The initial assumptions for CCS in the industry sector were very simplistic with unrealistic capture rates of 100% across all sub-sectors. This resulted in a first estimate of 37–53 Mt CO2 sequestered from industrial point sources in 2050 (WWF, 2009). Despite more sophisticated calculation methods in the latest scenarios with industry CCS, the range of modeling results is still in the same order of magnitude with 16–93 Mt CO2 (BMU, 2015a; BDI, 2018; BMWi, 2018; dena, 2018). The uncertainties already mentioned in section Challenges in Matching Biogenic and Industrial CO2 Supply and Demand with respect to CO2 availability from industrial sources apply here as well. Interestingly though, the depiction of industry CCS is mainly positively connoted as final resort for achieving carbon neutrality in the industrial sectors. However, despite its uncontested asset of creating negative emissions, BECCS did not mirror a similar dynamic in recent years.
The limited number of deliberate representation of BECCS—or CCS in general—is likely to be a result of various obstacles that scenario studies perceive in matters of CCS deployment (Table 2). Thirty out of the 66 analyzed studies raised at least one area of concern that either limited or prohibited CCS implementation in their scenarios. Over the entire assessment period from 2002 to 2019, technology-related items were the most prevalent. While techno-economic uncertainties such as the maturity and availability of capture technologies were more of a concern in the late 2000s, reservations are nowadays rather express with regard to the overall process efficiency and the energy penalty associated with the CO2 capture. Within the category of economic arguments, studies are most critical about investment costs and risks on both plant scale and overall infrastructure. Generalized and often not further specified long-term risks for the environment are noted in every third scenario study. While storage capacity and competition for storage space and/or locations is in 8 studies an area of concern, the systems perspective of integrating CCS activities is less of a preoccupation.
As already mentioned, the frequency of these arguments, cumulated over time, points toward an early, and sustained preoccupation with techno-economic concerns (Figure 6). However, in parallel, a first sign of public awareness issues arises with the introduction of the EU CCS directive and its subsequent implementation in Germany. Similarly, in the post-Paris Agreement debate on decarbonization options, negative perceptions regarding public acceptance of CCS resurfaced in the scenarios. Interestingly, although none of the scenario studies provides evidence for the supposed societal non-acceptance, this arguments is the single most raised rationale against CCS. A study commissioned by the Federation of German Industries (BDI) partially attenuates this narrative: If there are no or only much more expensive alternatives to industrial CCS, both political and social acceptance is assumed (BDI, 2018). Insofar, public perception would only be a question of costs. Slightly more nuanced, the German Energy Agency (dena) approves CCS as last resort in spite of societal concern—but only if even more costly alternatives are already exploited (dena, 2018). The latest study sponsored by the Federal Ministry for Economic Affairs and Energy (BMWi) assumes that public acceptance is only a minor obstacle for CCS in the industrial sector due to the lack of cost-effective alternatives (BMWi, 2018). However, all of the recent publications supported by ministries, agencies or NGOs related to environmental matters or nature conservation either do not address or explicitly exclude CCS (BUND, 2015; BfN, 2018; BMU, 2019; UBA, 2019b). This being said, the political context of the CCS debate is likely to change with German Chancellor Angela Merkel putting the CCS debate back on the table in May 2019, veering away from the fossil CCS rhetoric to a focus on delivering the Paris Agreement (Bundeskanzleramt, 2019; Kornelius et al., 2019). These contrasting views suggest that the public acceptance argument is seemingly more a political one than a primarily societal one. This is further underpinned by the fact that there are also societal objections to and citizen initiatives against onshore wind parks (Reusswig et al., 2016; Langer et al., 2018), geothermal energy systems (Kunze and Hertel, 2017; Benighaus and Bleicher, 2019), or the extension of the transmission grid (Neukirch, 2016; Kühne and Weber, 2017). However, this does not prevent these options from being part of the future energy mix.
Other Biomass-Based NETs: Conserving Ecosystem Services
Besides BECCS, large-scale afforestation is the second most prominently featured NET option in numerous global IAMs such as AIM, GCAM, and MESSAGE-GLOBIOM (Forster et al., 2018). However, this is not the case in the present selection of scenario studies for Germany, at least not when it comes to large-scale implementation. For example, the word “afforestation” appears explicitly in only five studies, among which only a single analysis provides an estimation of the associated GHG emission reduction, namely an annual 18 t CO2 per hectare when converting pastureland into forests (UBA, 2014). In addition, however, various studies mention measures to preserve at least the existing net carbon sink function of forests (WWF, 2009; BMU, 2019). These include, for example, some reserved areas for natural forest development as well as the implementation of sustainable forest management practices in the public forests (Greenpeace, 2015). In addition, arable land and grassland are partially converted into forest (BMU, 2015a, 2017).
Within the analyzed scenarios, the category of wetlands and ecosystem restoration follows a similar logic: no large-scale negative emissions initiatives, but individual measures to keep the land use, land use change and forestry (LULUCF) overall as a natural sink. In the scenarios, it is common practice to stop peat extraction on the one hand and to rewet peat soils on the other (UBA, 2014, 2019a,b; Greenpeace, 2015; BMU, 2019). A partial implementation of the measures can amount to a reduction of 13.3 Mt CO2 in 2050. Savings of up to 37.5 Mt CO2 are feasible if a full implementation takes place (BMU, 2015a).
In comparison, soil carbon sequestration (SCS) is rarely taken into account in the scenarios, based on the assumption that carbon uptake of agricultural soil is reversible and cannot be sustained in the longer run (UBA, 2014). Only a Greenpeace study assessed the potential of natural CO2 sequestration, e.g., through increased cultivation of humus-building plants, potentially leading to a moderate GHG reduction of 3.8 Mt CO2 in 2050 (Greenpeace, 2015). Information available for biochar is even less abundant, having only been mentioned once in a single study. Hence, these NET options are largely non-existent in the German decarbonization scenarios.
Beyond the different depths in which the respective NETs categories are represented in the scenarios, a common trend can nevertheless be identified: without exception, all scenario studies that provide more detailed assumptions and/or quantification of emission savings for the biomass-based NETs and the LULUCF sector in general have been financed by environmental ministries, agencies and NGOs. Other branches of government or industry are mostly limited to integrated energy system models for electricity, heat, and transport. However, in view of an economy-wide approach and to better reflect non-energy related emissions, the integration should more systematically extend to the LULUCF sector.
Moving From BCO2 Blind Spots to an Overarching Scenario Framework
Revisiting the classification of BCO2 concepts as set out in Figure 1, and contrasting it with the previous findings on each of the BCO2 categories, there is only one bright spot: the energetic use of bio-resources. While this does not come as a surprise for the traditional bioenergy sector thanks to the longstanding tradition of biomass as energy carrier, the relatively strong representation of (biogenic) CO2 utilization is, however, a rather new phenomenon. This can mainly be attributed to the ever growing role of sector coupling in Germany, necessitating besides hydrogen also a carbon source. Regarding bioenergy in combination with CCS, many studies rejected CCS so far based on the grounds of a diffuse and not evidence-based assumption of societal and political non-acceptance. However, the analysis has also shown that this is not set in stone, at least for industrial CCS. In this respect, BECCS could possibly also become an accepted or at least tolerated option in the medium to long term, in particular thanks to its unique selling point of sustainable energy production with simultaneous generation of negative emissions. To strengthen the representation of biomass for energetic use, including BECCS, in future scenarios, the underlying assumptions for biomass resource types, potentials and international trade should be documented more transparently as they heavily affect all subsequent decisions on the allocation of biomass to different sectors and ultimately also the amount of GHG emissions savings that can come from biomass use. In this regard, GHG emission accounting along the bioenergy lifecycle should be given greater attention in the scenario studies, notably but not limited to direct and indirect land use change effects in developing and emerging countries. In addition, biomass allocation needs to be put in a systems perspective, especially if BECCS is to play a role in the future energy system as it requires more large-scale and centralized bioenergy plants.
The competition for biomass resources extends to the material-related BCO2 concepts. The analysis revealed that quantitative assessment of both biomass and biogenic CO2 for material applications are mostly non-existent. Furthermore, if the concept of material use is qualitatively mentioned, this is solely done as a means to illustrate examples rather than for taking a holistic and structured approach to analyzing biomass use for all non-energetic purposes. Leaving significant room for improvement, the introduction of material flow analyses within the scenario frameworks could be instrumental in assessing these competing biomass uses, identify opportunities for cascading use strategies, and ultimately guide biomass allocation. This also constitutes a first step into an integrated bioeconomy thinking, i.e., “the knowledge-based production and use of renewable resources, in order to provide products, processes and services in all areas of the economy, within the framework of an economic system that is viable for the future” (BMEL, 2014).
While energy and decarbonization scenario studies that were published within the last 10–15 years only scratched upon the surface of biomass-based NETs so far, modeling, and monitoring their carbon sink capacity and performance is a prerequisite for determining an acceptable amount of residual emissions in other sectors without compromising overall climate neutrality by the mid-century. However, this implies that competition for different land use options will increase as some of the land-based NETs are mutually exclusive. Therefore, it will be crucial to bridge the gap between energy and land use models in order to build an adequate framework for the integration of biomass-based NETs into German decarbonization scenarios.
Conclusion
This paper set out to explore the technology-openness of energy and decarbonization scenarios with respect to biomass-based carbon removal concepts (BCO2). By performing a systematic review of 66 studies containing a total of 189 scenarios for Germany, the aim was to provide a first appraisal of the representation of BCO2 in a scenario context and to formulate recommendations for their better integration into existing and future modeling frameworks.
Our findings indicate that bioenergy is the best-represented BCO2 concept, however assumptions made for bioenergy potentials either take outdated references as assessment basis or are not detailed enough with regard to biomass resource types and GHG emission accounting. In contrast, the material biomass use perspective is largely not addressed, especially lacking quantified data. The challenge with biogenic CO2 is to match its supply and demand, also in the light of other potential CO2 sources from industry and direct air capture. Techno-economic assumptions and data for BECCS is available but has only been implemented in few scenarios, mainly due to an assumption of non-existent public acceptance. A critical review however suggests to revisit the public acceptance argument as scenario studies failed to provide evidence for their claim. Other NETs have—if at all—not been addressed under a large-scale negative emissions perspective but rather as conservation measure for its current carbon sink function.
Acknowledging that the aim of scenario modeling is not to replicate the reality but to explore different technology options and their interdependencies, the findings of this study indicate two major axes of improvement, namely the need for integrated energy-land modeling frameworks as well as the adoption of bioeconomy perspectives in the scenario narrative. This being said, extending the modeling scope increases complexity and creates trade-offs with regard to granularity. To tackle these challenges, soft-linking otherwise separate models could be envisaged. This allows in an iterative process to converge on key parameters instead of requiring a full model integration. Existing global IAMs can serve as a blueprint in this regard, such as the energy supply model MESSAGE linked via an emulator to the land use model GLOBIOM. In order to facilitate such integrated energy-land modeling frameworks, interdisciplinary research, and cooperation is of crucial importance. Having shown in this study that environmental organizations from the governmental and non-governmental sphere were most likely to publish scenario studies that do include land use considerations, they could be instrumental in funding and facilitating such interactions. Finally, beyond the topic of BCO2 concepts, guidelines for standardized scenario documentations could support greater transparency and better comparability across models and scenarios.
Author Contributions
AH devised the project with supervision by NS and DT. AH collected, analyzed, interpreted the data, and wrote the paper. All authors discussed the results and critically commented on the manuscript.
Funding
This research received funding from the Federal Ministry of Food and Agriculture (BMEL) pursuant to a resolution by the German Bundestag.
Conflict of Interest
The authors declare that the research was conducted in the absence of any commercial or financial relationships that could be construed as a potential conflict of interest.
Acknowledgments
We thank Indrani Kar for thorough proof reading and the two reviewers for their valuable comments and recommendations that helped to improve this work.
Footnote
1. ^If not explicitly stated otherwise, BECCS is understood in this paper as all kinds of bioenergy production combined with carbon capture and storage. It therefore encompasses dedicated bioenergy plants, biomass co-firing plants, biofuel production, and industrial uses of biomass, for example in the iron and steel industry.
References
50Hertz (2016). Energiewende Outlook 2035: Entwicklungspfade der Energiewende und deren Folgen. Abschlussbericht. Available online at: http://www.50hertz.com/Portals/1/Dokumente/Netz/Netzentwicklung/Downloadbox%20Energiewende%20Outlook%202035/Abschlussbericht.pdf (accessed July 01, 2019).
Agora (2013). Kostenoptimaler Ausbau der Erneuerbaren Energien in Deutschland: Ein Vergleich möglicher Strategien für den Ausbau von Wind- und Solarenergie in Deutschland bis 2033. Available online at: http://www.agora-energiewende.de/fileadmin2/Projekte/2012/Kostenoptmaler-Ausbau-EE/Agora_Studie_Kostenoptimaler_Ausbau_der_EE_Web_optimiert.pdf (accessed June 12, 2019).
Bartholdsen, H. K., Eidens, A., Löffler, K., Seehaus, F., Wejda, F., Burandt, T., et al. (2019). Pathways for Germany's low-carbon energy transformation towards 2050. Energies 12:2988. doi: 10.3390/en12152988
BDEW, GIZ, and PwC (2016). Delphi Energy Future 2040: Delphi-Study on the Future of Energy Systems in Germany, Europe and the world by the Year 2040. Available online at Available online at: http://www.pwc.com/gx/en/energy-utilities-mining/pdf/delphi-energy-future.pdf (accessed November 21, 2019).
BDI (2013). Trendstudie 2030+: Kompetenzinitiative Energie des BDI. Im Auftrag des Bundesverbandes der Deutschen Industrie BDI. Available online at: http://image-src.bcg.com/Images/Trendstudie%202030_tcm108-141125.pdf (accessed April 08, 2019).
BDI (2018). Klimapfade für Deutschland. Im Auftrag des Bundesverbandes der Deutschen Industrie (BDI). Availabel online at: Available online at: http://www.prognos.com/uploads/tx_atwpubdb/20180118_BDI_Studie_Klimapfade_fuer_Deutschland_01.pdf (accessed April 08, 2019).
BDI, DEBRIV, GVSt, VDN,VGB PowerTech, and VRE (2007). Energiewirtschaftliches Gesamtkonzept 2030: Szenariendokumentation. Im Auftrag des Verband der Elektrizitätswirtschaft (VDEW), gemeinsam mit den Verbänden BDI, DEBRIV, GVSt, VDN, VGB PowerTech und VRE. Available online at: http://braunkohle.de/index.php?article_id=98&fileName=studie_2030_endbericht_final.pdf (accessed April 12, 2019).
BEE (2014a). Aktuelle Szenarien der deutschen Energieversorgung unter Berücksichtigung der Eckdaten des Jahres 2014. Kurzexpertise für den Bundesverband Erneuerbare Energien e.V. Available online at: http://www.bee-ev.de/fileadmin/Publikationen/20150419-Szenarien_SZEN-15.pdf (accessed April 05, 2019).
BEE (2014b). GROKO – II: Szenarien der deutschen Energieversorgung auf der Basis des EEG-Gesetzentwurfs - insbesondere Auswirkungen auf den Wärmesektor. Kurzexpertise für den Bundesverband Erneuerbare Energien e.V. Available online at: http://www.bee-ev.de/fileadmin/Publikationen/Studien/20140827_SzenarienderdeutschenEnergieversorgung_Waermesektor.pdf (accessed July 03, 2019).
BEE (2015). Szenarien der deutschen Energieversorgung vor dem Hintergrund der Vereinbarungen der Großen Koalition. Kurzexpertise für den Bundesverband Erneuerbare Energien e.V. Available online at: http://www.bee-ev.de/fileadmin/Publikationen/Studien/20140205_BEE-Szenarien_GROKO_Nitsch.pdf (accessed April 05, 2019).
BEE (2016). Die Energiewende nach COP 21: Aktuelle Szenarien der deutschen Energieversorgung. Kurzstudie für den Bundesverband Erneuerbare Energien e.V. Avaliable online at: Available online at: http://www.bee-ev.de/fileadmin/Publikationen/Studien/Joachim_Nitsch_Energiewende_nach_COP21_Langversion.pdf (accessed April 05, 2019).
BEE (2017). Erfolgreiche Energiewende nur mit verbesserter Energieeffizienz und einem klimagerechten Energiemarkt: Aktuelle Szenarien 2017 der deutschen Energieversorgung. Available online at: http://www.bee-ev.de/fileadmin/Publikationen/Studien/Erfolgreiche_Energiewende_Szenarien_2017_Nitsch.pdf (accessed April 05, 2019).
BEE (2018). Was für einen erfolgreichen Klimaschutz erforderlich ist: Schlussfolgerungen aus aktuellen Szenarien der deutschen Energieversorgung. Available online at: http://co2abgabe.de/wp-content/uploads/2018/03/Klimaschutz-18.pdf (accessed July 03, 2019).
Benighaus, C., and Bleicher, A. (2019). Neither risky technology nor renewable electricity: Contested frames in the development of geothermal energy in Germany. Energy Res. Soc. Sci. 47, 46–55. doi: 10.1016/j.erss.2018.08.022
BfN (2018). Naturverträgliche Energieversorgung aus 100% erneuerbaren Energien: Gefördert durch das Bundesamt für Naturschutz. Avaliable online at: Available online at: http://www.bfn.de/fileadmin/BfN/service/Dokumente/skripten/Skript501.pdf (accessed July 08, 2019).
BMEL (2014). National Policy Strategy on Bioeconomy: Renewable Resources and Biotechnological Processes as a Basis for Food, Industry and Energy. Available online at: http://www.bmel.de/SharedDocs/Downloads/EN/Publications/NatPolicyStrategyBioeconomy.pdf?__blob=publicationFile (accessed January 16, 2020).
BMU (2004). Ökologisch optimierter Ausbau der Nutzung erneuerbarer Energien in Deutschland: Langfassung. Studie im Auftrag des Bundesministeriums für Umwelt, Naturschutz und Reaktorsicherheit, FKZ 901 41 803. Available online at: http://www.ifeu.de/landwirtschaft/pdf/Oekologisch_optimierter_Ausbau_Langfassung.pdf (accessed June 13, 2019).
BMU (2008). Weiterentwicklung der “Ausbaustrategie Erneuerbare Energien” vor dem Hintergrund der aktuellen Klimaschutzziele Deutschlands und Europas: Leitstudie 2008. Im Auftrag des Bundesministeriums für Umwelt, Naturschutz und Reaktorsicherheit (BMU). Available online at: http://www.erneuerbare-energien.de/EE/Redaktion/DE/Downloads/Studien/leitstudie-2008.pdf (accessed April 10, 2019).
BMU (2009a). Langfristszenarien und Strategien für den Ausbau erneuerbarer Energien in Deutschland: Leitszenario 2009. Im Auftrag des Bundesministeriums für Umwelt, Naturschutz und Reaktorsicherheit (BMU). Available online at: http://www.erneuerbare-energien.de/EE/Redaktion/DE/Downloads/Studien/leitszenario-2009.pdf?__blob=publicationFile&v=2 (accessed April 10, 2019).
BMU (2009b). Projektionsbericht 2009 gemäß Entscheidung 280/2004/EG. Available online at: http://cdr.eionet.europa.eu/de/eu/ghgpro/envsgwza/ (accessed April 16, 2019).
BMU (2010). Langfristszenarien für den Ausbau der erneuerbaren Energien in Deutschland bei Berücksichtigung der Entwicklung in Europa und global: Leitstudie 2010. Im Auftrag des Bundesministeriums für Umwelt, Naturschutz und Reaktorsicherheit (BMU). Available online at: http://elib.dlr.de/68660/1/EEG_Kosten_Langfristszenarien.pdf (accessed April 10, 2019).
BMU (2011). Projektionsbericht 2011 gemäß Entscheidung 280/2004/EG. Available online at: http://cdr.eionet.europa.eu/de/eu/ghgpro/envtcqp5g/ (accessed April 16, 2019).
BMU (2012). Langfristszenarien für den Ausbau der erneuerbaren Energien in Deutschland bei Berücksichtigung der Entwicklung in Europa und global: Leitstudie 2011. Im Auftrag des Bundesministeriums für Umwelt, Naturschutz und Reaktorsicherheit (BMU). Available online at: http://www.dlr.de/dlr/Portaldata/1/Resources/bilder/portal/portal_2012_1/leitstudie2011_bf.pdf (accessed April 10, 2019).
BMU (2013). Projektionsbericht 2013 gemäß Entscheidung 280/2004/EG. Available online at: http://cdr.eionet.europa.eu/de/eu/ghgpro/envuucoda/130313_Projektionsbericht_DE_final.doc (accessed April 16, 2019).
BMU (2014). Klimaschutzszenario 2050: 1. Modellierungsrunde. Studie im Auftrag des Bundesministeriums für Umwelt, Naturschutz, Bau und Reaktorsicherheit (BMU). Available online at: http://www.oeko.de/oekodoc/2065/2014-638-de.pdf (accessed May 28, 2019).
BMU (2015a). Klimaschutzszenario 2050: 2. Endbericht. Studie im Auftrag des Bundesministeriums für Umwelt, Naturschutz, Bau und Reaktorsicherheit (BMU). Available online at: http://www.oeko.de/oekodoc/2451/2015-608-de.pdf (accessed May 28, 2019).
BMU (2015b). Projektionsbericht 2015 gemäß Verordnung 525/2013/EU. Availabel online at: http://cdr.eionet.europa.eu/de/eu/mmr/art04-13-14_lcds_pams_projections/envvqlq8w/150422_Projektionsbericht_2015_final.pdf (accessed April 16, 2019).
BMU (2016). Klimaschutzplan 2050: Klimaschutzpolitische Grundsätze und Ziele der Bundesregierung. Available online at: http://www.bmu.de/fileadmin/Daten_BMU/Download_PDF/Klimaschutz/klimaschutzplan_2050_bf.pdf (accessed May 21, 2019).
BMU (2017). Projektionsbericht 2017 für Deutschland gemäß Verordnung (EU) Nr. 525/2013. Available online at: http://cdr.eionet.europa.eu/de/eu/mmr/art04-13-14_lcds_pams_projections/projections/envwqc4_g/170426_PB_2017_-_final.pdf (accessed April 16, 2019).
BMU (2019). Folgenabschätzung zu den ökologischen, sozialen und wirtschaftlichen Folgewirkungen der Sektorziele für 2030 des Klimaschutzplans 2050 der Bundesregierung: Studie im Auftrag des Bundesministeriums für Umwelt, Naturschutz, Bau und Reaktorsicherheit (BMU). Available online at: http://www.oeko.de/fileadmin/oekodoc/Folgenabschaetzung-Klimaschutzplan-2050-Endbericht.pdf (accessed July 04, 2019).
BMWi (2005). Energiereport IV - Die Entwicklung der Energiemärkte bis zum Jahr 2030: Energiewirtschaftliche Referenzprognose. Kurzfassung. Im Auftrag des Bundesministeriums für Wirtschaft und Arbeit (BMWi). Available online at: http://www.prognos.com/uploads/tx_atwpubdb/050400_Prognos_EWI_Energiereport_IV.pdf (accessed April 18, 2019).
BMWi (2007). Energieszenarien für den Energiegipfel 2007: Endbericht inklusive Anhang 2 %-Variante. Im Auftrag des Bundesministeriums für Wirtschaft und Technologie(BMWi). Available online at: http://www.prognos.com/fileadmin/pdf/publikationsdatenbank/Energieszenarien-fuer-den-Energiegipfel-D-2007.pdf (accessed April 18, 2019).
BMWi (2010a). Die Entwicklung der Energiemärkte bis 2030: Energieprognose 2009. Available online at: ftp://ftp.zew.de/pub/zew-docs/gutachten/Energieprognose_2009_Hauptbericht.pdf (accessed April 18, 2019).
BMWi (2010b). Energieszenarien für ein Energiekonzept der Bundesregierung: Projekt Nr. 12/10 des Bundesministeriums für Wirtschaft und Technologie (BMWi). Available online at: http://www.prognos.com/uploads/tx_atwpubdb/100827_Prognos_Studie__Energieszenarien_fuer_ein_energiekonzept_der_Bundesregierung.pdf (accessed April 17, 2019).
BMWi (2011). Energieszenarien 2011: Projekt Nr. 12/10 des Bundesministeriums für Wirtschaft und Technologie (BMWi). Available online at: http://www.prognos.com/fileadmin/pdf/publikationsdatenbank/11_08_12_Energieszenarien_2011.pdf (accessed April 18, 2019).
BMWi (2014). Entwicklung der Energiemärkte: Energiereferenzprognose. Studie im Auftrag des Bundesministeriums Studie im Auftrag des Bundesministeriums BMWi(Projekt Nr. 57/12). Available online at: http://www.bmwi.de/Redaktion/DE/Publikationen/Studien/entwicklung-der-energiemaerkte-energiereferenzprognose-endbericht.pdf (accessed May 28, 2019).
BMWi (2015). Interaktion EE-Strom, Wärme und Verkehr: Analyse der Interaktion zwischen den Sektoren Strom, Wärme/Kälte und Verkehr in Deutschland in Hinblick auf steigende Anteile fluktuierender Erneuerbarer Energien im Strombereich unter Berücksichtigung der europäischen Entwicklung. Ableitung von optimalen strukturellen Entwicklungspfaden für den Verkehrs- und Wärmesektor. Gefördert mit Mitteln des Bundesministeriums für Wirtschaft und Energie (BMWi) (Förderkennzeichen 0325444A-C). Available online at: http://www.iee.fraunhofer.de/content/dam/iwes-neu/energiesystemtechnik/de/Dokumente/Veroeffentlichungen/2015/Interaktion_EEStrom_Waerme_Verkehr_Endbericht.pdf (accessed May 28, 2019).
BMWi (2018). Langfristszenarien für die Transformation des Energiesystems in Deutschland. Studie im Auftrag des Bundesministeriums für Wirtschaft und Energie (BMWi). Available online at: http://www.bmwi.de/Redaktion/DE/Artikel/Energie/langfrist-und-klimaszenarien.html (accessed May 18, 2019).
Boysen, L. R., Lucht, W., Gerten, D., Heck, V., Lenton, T. M., and Schellnhuber, H. J. (2017). The limits to global-warming mitigation by terrestrial carbon removal. Earth's Future 5, 463–474. doi: 10.1002/2016EF000469
BUND (2015). Grundlagen und Konzepte einer Energiewende 2050. Available online at: http://www.bund.net/fileadmin/user_upload_bund/publikationen/klimawandel/klima_energie_energiewendekonzept.pdf (accessed September 26, 2019).
Bundeskanzleramt (2019). Pressekonferenz von Bundeskanzlerin Merkel und dem Ministerpräsidenten des Königreichs der Niederlande, Mark Rutte: 16. Mai 2019. Available online at: http://www.bundeskanzlerin.de/bkin-de/aktuelles/pressekonferenz-von-bundeskanzlerin-merkel-und-dem-ministerpraesidenten-des-koenigreichs-der-niederlande-mark-rutte-1612118 (accessed May 18, 2020).
Bundestag (2002). Endbericht Nachhaltige Energieversorgung unter den Bedingungen der Globalisierung und der Liberalisierung: Drucksache 14/9400. Available online at: http://dip21.bundestag.de/dip21/btd/14/094/1409400.pdf (accessed April 18, 2019).
Cherubini, F., and Strømman, A. H. (2011). Life cycle assessment of bioenergy systems: State of the art and future challenges. Bioresour Technol. 102, 437–451. doi: 10.1016/j.biortech.2010.08.010
dena (2018). dena-Leitstudie Integrierte Energiewende: Impulse für die Gestaltung des Energiesystems bis 2050: Teil B - Gutachterbericht. Available online at: https://www.dena.de/fileadmin/dena/Dokumente/Pdf/9261_dena-Leitstudie_Integrierte_Energiewende_lang.pdf (accessed April 16, 2019).
EnBW, EON Energie RWE Power, and Vattenfall Europe (2009). Energiezukunft 2050: Teil II - Szenarien. Studie im Auftrag von EnBW, EON Energie, RWE Power und Vattenfall Europe. Available online at: http://www.ffe.de/download/berichte/Endbericht_Energiezukunft_2050_Teil_II.pdf (accessed May 21, 2019).
ExxonMobil (2011). Energieprognose Deutschland 2011-2030. Available online at: http://www.forschungsnetzwerk.at/downloadpub/Energieprognose_2011.pdf (accessed April 17, 2019).
ExxonMobil (2012). Energieprognose Deutschland 2012-2040. Available online at: http://services.exxonmobil.de/downloads/Energieprognose_2012.pdf (accessed April 17, 2019).
ExxonMobil (2016). Energieprognose Deutschland 2016-2040. Available online at: http://cdn.exxonmobil.com//~media/germany-natural-gas/files/publikationen/energie/energieprognose-2040.pdf (accessed April 17, 2019).
ExxonMobil (2018). Energieprognose Deutschland 2018-2040. Available online at: http://cdn.exxonmobil.com//~media/germany/files/energieprognose/exxonmobil_energiegrognose_2018.pdf (accessed April 08, 2019).
Fargione, J. E., Bassett, S., Boucher, T., Bridgham, S. D., Conant, R. T., Cook-Patton, S. C., et al. (2018). Natural climate solutions for the United States. Sci. Adv. 4:eaat1869. doi: 10.1126/sciadv.aat1869
Forster, P. M., Huppmann, D., Kriegler, E., Mundaca, L., Smith, C., Rogelj, J., et al. (2018). “Mitigation pathways compatible with 1.5°C in the context of sustainable development: Supplementary material,” in Global Warming of 1.5°C: An IPCC Special Report on the Impacts of Global Warming of 1.5°C Above Pre-industrial Levels and Related Global Greenhouse Gas Emission Pathways, in the Context Of Strengthening the Global Response to the Threat of Climate Change, Sustainable Development, And Efforts To Eradicate Poverty (Geneva: IPCC).
Fraunhofer ISE (2012). 100 % erneuerbare Energien für Strom und Wärme in Deutschland. Available online at: http://www.ise.fraunhofer.de/content/dam/ise/de/documents/publications/studies/studie-100-erneuerbare-energien-fuer-strom-und-waerme-in-deutschland.pdf (accessed April 18, 2019).
Fraunhofer ISE (2013). Energiesystem Deutschland 2050: Sektor- und Energieträgerübergreifende, modellbasierte, ganzheitliche Untersuchung zur langfristigen Reduktion energiebedingter CO2-Emissionen durch Energieeffizienz und den Einsatz Erneuerbarer Energien. Available online at: http://www.ise.fraunhofer.de/content/dam/ise/de/documents/publications/studies/Fraunhofer-ISE_Energiesystem-Deutschland-2050.pdf (accessed April 18, 2019).
Fraunhofer ISE (2015). Was kostet die Energiewende? Wege zur Transformation des deutschen Energiesystems bis 2050. Henning, Hans-Martin; Palzer, Andreas. Available online at: http://www.fraunhofer.de/content/dam/zv/de/Forschungsfelder/Energie-Rohstoffe/Fraunhofer-ISE_Transformation-Energiesystem-Deutschland_final_19_11%20(1).pdf (accessed April 12, 2019).
FVEE (2010). Energiekonzept 2050: Eine Vision für ein nachhaltiges Energiekonzept auf Basis von Energieeffizienz und 100 % erneuerbaren Energien. Available online at: http://www.fvee.de/fileadmin/politik/10.06.vision_fuer_nachhaltiges_energiekonzept.pdf (accessed May 21, 2019).
Gelsenwasser, Open Grid Europe, and RheinEnergie (2017). Energiemarkt 2030 und 2050: Der Beitrag von Gas- und Wärmeinfrastruktur zu einer effizienten CO2-Minderung. Studie im Auftrag von Gelsenwasser, Open Grid Europe und RheinEnergie. Available online at: http://www.ewi.research-scenarios.de/cms/wp-content/uploads/2017/11/ewi_ERS_Energiemarkt_2030_2050.pdf (accessed May 21, 2019).
Greenpeace (2007). Klimaschutz: Plan B: Nationales Energiekonzept bis 2020. Studie wurde im Auftrag von Greenpeacedurchgeführt von EUtech Energie und Management. Available online at: http://www.greenpeace.de/sites/www.greenpeace.de/files/publications/energiewende_final_neu2.pdf (accessed April 02, 2019).
Greenpeace (2010). Klimaschutz: Plan B 2050: Energiekonzept für Deutschland. Studie wurde im Auftrag von Greenpeacedurchgeführt von EUtech Energie und Management. Available online at: http://www.greenpeace.de/sites/www.greenpeace.de/files/plan_b_2050_lang_0.pdf (accessed April 02, 2019).
Greenpeace (2015). Klimaschutz: Der Plan: Energiekonzept für Deutschland. Available online at: http://www.greenpeace.de/sites/www.greenpeace.de/files/publications/klimaschutz-der-plan-greenpeace-20151117.pdf (accessed April 02, 2019).
Greenpeace (2018). Wie Deutschland sein Klimaziel noch erreichen kann: Teilergebnisse eines 1,5°C-Szenarios. Studie wurde im Auftrag von Greenpeacedurchgeführt von Fraunhofer IEE. Available online at: http://www.greenpeace.de/sites/www.greenpeace.de/files/publications/energieszenario_fuer_2020.pdf (accessed April 02, 2019).
Griscom, B. W., Adams, J., Ellis, P. W., Houghton, R. A., Lomax, G., Miteva, D. A., et al. (2017). Natural climate solutions. Proc. Natl. Acad. Sci. U.S.A. 114, 11645–11650. doi: 10.1073/pnas.1710465114
Haller, M., Repenning, J., Vogel, M., Schlomann, B., Reitze, F., Schön, M., et al. (2016). Überblick über vorliegende Szenarienarbeiten für den Klimaschutz in Deutschland bis 2050. Arbeitspaket 1.1 im Forschungs- und Entwicklungsvorhaben des Bundesministeriums für Umwelt, Naturschutz, Bau und Reaktorsicherheit: Wissenschaftliche Unterstützung “Erstellung und Begleitung des Klimaschutzplans 2050” für das FKZ UM 15 41 1860. Available online at: http://www.oeko.de/oekodoc/2445/2016-602-de.pdf (accessed January 16, 2020).
Hansen, K., Mathiesen, B. V., and Skov, I. R. (2019). Full energy system transition towards 100% renewable energy in Germany in 2050. Renew. Sust. Energy Rev. 102, 1–13. doi: 10.1016/j.rser.2018.11.038
Heck, V., Gerten, D., Lucht, W., and Popp, A. (2018). Author Correction: Biomass-based negative emissions difficult to reconcile with planetary boundaries. Nat. Clim. Change 8:345. doi: 10.1038/s41558-018-0107-z
IPCC, (ed.). (2018). Global warming of 1.5°C: An IPCC Special Report on the Impacts of Global Warming of 1.5°C Above Pre-industrial Levels and Related Global Greenhouse Gas Emission Pathways, in the Context of Strengthening the Global Response to the Threat of Climate Change, Sustainable Development, and Efforts to Eradicate Poverty. Geneva.
IPCC (2019). “Summary for Policymakers,” in Climate Change and Land: An IPCC Special Report On Climate Change, Desertification, Land Degradation, Sustainable Land Management, Food Security, and Greenhouse Gas Fluxes In Terrestrial Ecosystems, eds P. R. Shukla, J. Skea, E. Calvo Buendia, V. Masson-Delmotte, H.-O. Pörtner, D. C. Roberts, et al. (Geneva).
IRENA (2015). Renewable Energy Prospects: Germany: REmap 2030 Analysis. Available online at: http://www.irena.org/-/media/Files/IRENA/Agency/Publication/2015/IRENA_REmap_Germany_report_2015-(1).pdf (accessed June 13, 2019).
Kornelius, S., Fried, N., and Oltermann, P. (2019). Merkel: Europe Must Unite to Stand Up to China, Russia and US: German Chancellor Also Shares Views on Brexit and Climate Crisis in Interview. Available online at: http://www.theguardian.com/world/2019/may/15/angela-merkel-interview-europe-eu-unite-challenge-us-russia-china (accessed May 18, 2020).
Kronenberg, T., Martinsen, D., Pesch, T., Sander, M., Fischer, W., Hake, J.-F., et al. (2011). Energieszenarien für Deutschland: Stand der Literatur und methodische Auswertung. Available online at: http://www.fz-juelich.de/SharedDocs/Downloads/IEK/IEK-STE/EN/report_13_2011.pdf?__blob=publicationFile (accessed November 19, 2019).
Kühne, O., and Weber, F. (2017). Conflicts and negotiation processes in the course of power grid extension in Germany. Landsc. Res. 43, 529–541. doi: 10.1080/01426397.2017.1300639
Kunze, C., and Hertel, M. (2017). Contested deep geothermal energy in Germany—the emergence of an environmental protest movement. Energy Res. Soc. Sci. 27, 174–180. doi: 10.1016/j.erss.2016.11.007
Langer, K., Decker, T., Roosen, J., and Menrad, K. (2018). Factors influencing citizens' acceptance and non-acceptance of wind energy in Germany. J. Clean. Product. 175, 133–144. doi: 10.1016/j.jclepro.2017.11.221
Lenton, T. M. (2014a). “Chapter 3. the global potential for carbon dioxide removal,” in Geoengineering of the Climate System, eds R. Harrison, and R. Hester (Cambridge: Royal Society of Chemistry), 52–79. doi: 10.1039/9781782621225-00052
Lenton, T. M. (2014b). The potential for land-based biological CO2 removal to lower future atmospheric CO2 concentration. Carbon Manage. 1, 145–160. doi: 10.4155/cmt.10.12
Minx, J. C., Lamb, W. F., Callaghan, M. W., Bornmann, L., and Fuss, S. (2017). Fast growing research on negative emissions. Environ. Res. Lett. 12:35007. doi: 10.1088/1748-9326/aa5ee5
Neukirch, M. (2016). Protests against German electricity grid extension as a new social movement? A journey into the areas of conflict. Energ. Sustain. Soc. 6:13. doi: 10.1186/s13705-016-0069-9
Peter, M., Bertschmann, D., and Lückge, H. (2017). Metastudie nationale Energieszenarien und deutsche Energiepolitik. Im Auftrag des Umweltbundesamtes, Forschungskennzahl 3712 46 103. Available online at: http://www.umweltbundesamt.de/sites/default/files/medien/1410/publikationen/2017-11-06_climate-change_27-2017_metastudie-energieszenarien.pdf (accessed November 19, 2019).
Petticrew, M., and McCartney, G. (2011). Using systematic reviews to separate scientific from policy debate relevant to climate change. Am. J. Prevent. Med. 40, 576–578. doi: 10.1016/j.amepre.2010.12.022
Renn, O. (2017). Das Energiesystem resilient gestalten: Szenarien - Handlungsspielräume - Zielkonflikte. München; Halle (Saale); Mainz: Deutsche Akademie der Technikwissenschaften e.V; Deutsche Akademie der Naturforscher Leopoldina e.V; Union der Deutschen Akademie der Wissenschaften e.V.
Reusswig, F., Braun, F., Heger, I., Ludewig, T., Eichenauer, E., and Lass, W. (2016). Against the wind: local opposition to the German energiewende. Utilities Policy 41, 214–227. doi: 10.1016/j.jup.2016.02.006
Runkel, M. (2018). Literaturrecherche zu Studien, die eine Dekarbonisierung der Stromerzeugung und anderer Anwendungsbereiche in Deutschland, Europa und weltweit untersuchen. Im Auftrag des Umweltbundesamtes, Projektnummer 75030. Available online at: http://www.umweltbundesamt.de/sites/default/files/medien/1410/publikationen/2018-06-05_climate-change_14-2018_dekarbonisierung.pdf (accessed November 19, 2019).
Samadi, S., Terrapon-Pfaff, J., Lechtenböhmer, S., and Knoop, K. (2019). Long-term low greenhouse gas emission development strategies for achieving the 1.5 °C target – insights from a comparison of German bottom-up energy scenarios. Carbon Manage. 9, 549–562. doi: 10.1080/17583004.2018.1475174
Schmid, E., and Knopf, B. (2012). Ambitious mitigation scenarios for Germany: a participatory approach. Energy Policy 51, 662–672. doi: 10.1016/j.enpol.2012.09.007
Shell (2017). Shell Energie Szenarien Deutschland. Available online at: http://www.shell.de/medien/shell-publikationen/energieszenarien/_jcr_content/par/relatedtopics_8666.stream/1505305389981/dadc5c9800ca13cc2e7a5f4682b326a3487901b82efa6891053ad51ef4004edf/german-etcc-brochure.pdf (accessed April 08, 2019).
Szarka, N., Eichhorn, M., Kittler, R., Bezama, A., and Thrän, D. (2017). Interpreting long-term energy scenarios and the role of bioenergy in Germany. Renew. Sust. Energ. Rev. 68, 1222–1233. doi: 10.1016/j.rser.2016.02.016
Turner, P. A., Field, C. B., Lobell, D. B., Sanchez, D. L., and Mach, K. J. (2018). Unprecedented rates of land-use transformation in modelled climate change mitigation pathways. Nat. Sust. 1, 240–245. doi: 10.1038/s41893-018-0063-7
UBA (2008). Politikszenarien für den Klimaschutz IV: Szenarien bis 2030. Studie erstellt durch Öko-Institut, IEF-STE, DIW und Fraunhofer ISI (Forschungskennzahl 20546434). Available online at: http://www.umweltbundesamt.de/sites/default/files/medien/publikation/long/3361.pdf (accessed April 08, 2019).
UBA (2009). Politikszenarien für den Klimaschutz V – auf dem Weg zum Strukturwandel: Treibhausgas-Emissionsszenarien bis zum Jahr 2030. Studie erstellt durch Öko-Institut, IEF-STE, DIW und Fraunhofer ISI (Forschungskennzahl 20642106). Available online at: http://www.umweltbundesamt.de/sites/default/files/medien/publikation/long/3764.pdf (accessed April 08, 2019).
UBA (2010). Energieziel 2050: 100% Strom aus erneuerbaren Quellen. Available online at: http://www.umweltbundesamt.de/sites/default/files/medien/378/publikationen/energieziel_2050.pdf (accessed June 11, 2019).
UBA (2013a). Modellierung einer vollständig auf erneuerbaren Energien basierenden Stromerzeugung im Jahr 2050 in autarken, dezentralen Strukturen. Available online at: http://www.umweltbundesamt.de/sites/default/files/medien/376/publikationen/climate_change_14_2013_modellierung_einer_vollstaendig_auf_erneuerbaren_energien.pdf (accessed July 19, 2019).
UBA (2013b). Politikszenarien für den Klimaschutz VI: Treibhausgas-Emissionsszenarien bis zum Jahr 2030. Studie erstellt durch Öko-Institut, IEF-STE, DIW und Fraunhofer ISI (Forschungskennzahl 370941109). Available online at: http://www.umweltbundesamt.de/sites/default/files/medien/461/publikationen/4412.pdf (accessed April 08, 2019).
UBA (2014). Treibhausgasneutrales Deutschland im Jahr 2050. Available online at: http://www.umweltbundesamt.de/sites/default/files/medien/378/publikationen/07_2014_climate_change_dt.pdf (accessed May 28, 2019).
UBA (2018). Politikszenarien für den Klimaschutz VII: Treibhausgas-Emissionsszenarien bis zum Jahr 2035. Studie erstellt durch Öko-Institut und Fraunhofer ISI (Forschungskennzahl 3714411040). Available online at: http://www.umweltbundesamt.de/sites/default/files/medien/1410/publikationen/2018-01-11_climate-change_01-2018_politikszenarien-vii.pdf (accessed April 08, 2019).
UBA (2019a). Den Weg zu einem treibhausgasneutralen Deutschland ressourcenschonend gestalten: 2. Auflage mit methodischen Anpassungen und Teilneuberechnung in Kapitel 2 und 3. Available online at: http://www.umweltbundesamt.de/sites/default/files/medien/376/publikationen/190215_uba_fachbrosch_rtd_bf.pdf (accessed April 17, 2019).
UBA (2019b). Wege in eine ressourcenschonende Treibhausgasneutralität – RESCUE. Available online at: http://www.umweltbundesamt.de/sites/default/files/medien/376/publikationen/rescue_studie_cc_36-2019_wege_in_eine_ressourcenschonende_treibhausgasneutralitaet.pdf (accessed December 05, 2019).
WWF (2009). Modell Deutschland - Klimaschutz bis 2050: Vom Ziel her denken. Die Studie wurde erstellt von Öko-Institut und Prognos. Available online at: http://www.wwf.de/fileadmin/fm-wwf/Publikationen-PDF/WWF_Modell_Deutschland_Endbericht.pdf (accessed April 02, 2019).
Keywords: energy scenarios, Germany, negative emission technology (NET), Carbon Dioxide Removal (CDR), Bioenergy with Carbon Capture and Storage (BECCS), biogenic carbon, carbon capture and utilization (CCU), power-to-gas (PtG)
Citation: Hahn A, Szarka N and Thrän D (2020) German Energy and Decarbonization Scenarios: “Blind Spots” With Respect to Biomass-Based Carbon Removal Options. Front. Energy Res. 8:130. doi: 10.3389/fenrg.2020.00130
Received: 17 January 2020; Accepted: 27 May 2020;
Published: 02 July 2020.
Edited by:
Mai Bui, Imperial College London, United KingdomReviewed by:
Ajay Gambhir, Imperial College London, United KingdomPraveen Bains, International Energy Agency, France
Copyright © 2020 Hahn, Szarka and Thrän. This is an open-access article distributed under the terms of the Creative Commons Attribution License (CC BY). The use, distribution or reproduction in other forums is permitted, provided the original author(s) and the copyright owner(s) are credited and that the original publication in this journal is cited, in accordance with accepted academic practice. No use, distribution or reproduction is permitted which does not comply with these terms.
*Correspondence: Alena Hahn, YWxlbmEuaGFobkBkYmZ6LmRl; Daniela Thrän, ZGFuaWVsYS50aHJhZW5AdWZ6LmRl