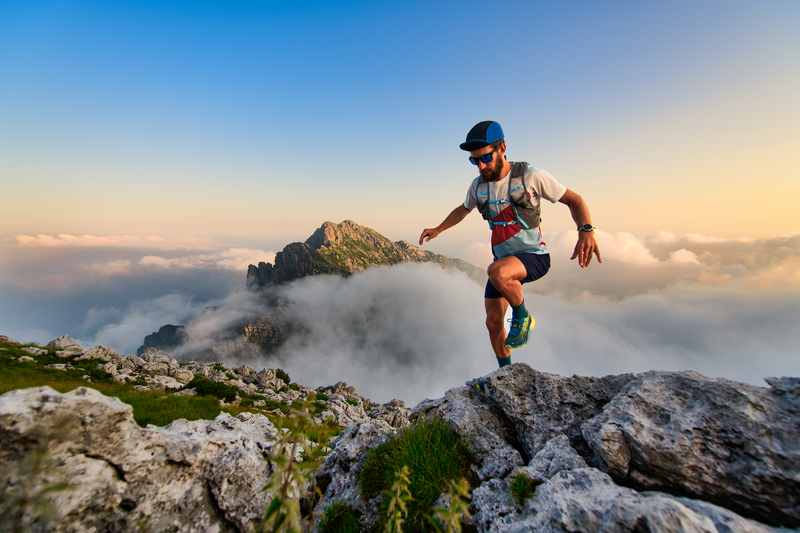
94% of researchers rate our articles as excellent or good
Learn more about the work of our research integrity team to safeguard the quality of each article we publish.
Find out more
REVIEW article
Front. Energy Res. , 09 June 2020
Sec. Carbon Capture, Utilization and Storage
Volume 8 - 2020 | https://doi.org/10.3389/fenrg.2020.00108
This article is part of the Research Topic Emerging Technologies and Associated Scientific Advancements for CCUS Deployment View all 6 articles
Recent advances in modular nuclear reactors could facilitate the use of irradiation to produce fuels and chemicals using anthropogenic CO2. One of the challenges in CO2 conversion reactions is the stability or low reactivity of CO2. Chemical activation of CO2 via thermochemical or electrochemical approaches contributes to the high energy intensity of CO2 conversion reactions. Activation of the CO2 molecule via irradiation at significantly lower temperatures may now allow us to use heterogeneous CO2-bearing waste gas streams and low-carbon emitting nuclear energy resources to produce high value chemicals and fuels in a distributed manner. In this paper, we review the radiolytic behavior of CO2 and particularly, irradiation pathways relevant for producing fuels and chemicals using CO2, technological advances and research directions for advancing radiolytic conversion of CO2 to chemicals and fuels.
Energy security and climate change are two of humankind's primary concerns in the twenty-first century. While our reliance on carbonaceous fuel resources over the past century has elevated economic activity globally, rapid advancements in carbon mitigation and removal technologies are needed to reduce detrimental impacts on climate. Energy security and climate systems are intrinsically connected to carbon emitting power plants, particularly and to renewable energy systems (Chen et al., 2019). Variability and intermittence are intrinsic characteristics of renewable power generation that have direct consequences in changing climate and environmental conditions. This inconsistency is absent from nuclear power generation, making nuclear a reliable, and secure low-carbon emitting energy resource.
Nuclear energy has long been championed as the carbon-neutral solution to replace base-load electrical energy demand—currently satiated by contributions from fossil fuels (e.g., coal and natural gas). In addition to its attribute of being an energy source with low carbon emissions, nuclear energy produces a unique combination of energy types that can be used for chemical transformations, such as ionizing radiation, electrons, and heat. These capabilities attempt to harness a reactor's excess thermal load (temperature), off-peak current (electricity), and inherent multi-component radiation field (neutrons, fast electrons, and gamma/X-rays). Recent advancements in micro- and small modular nuclear reactors have the potential to advance distributed decarbonization strategies. Additionally, developments include all ranges of nuclear reactors for the different ranges of neutron spectrum, of different core designs, of different coolant and moderator types. The status of the technological readiness levels vary across this wide range of nuclear reactor technologies, such as Molten Salt Reactors, Fluoride Salt-cooled High, Temperature Reactors, Liquid Metal-cooled Fast Reactors, High Temperature Gas Reactors, Pebble Bed Reactors, Nuclear Battery Reactors, Designs Advanced Nuclear Fuels, Fusion Reactors, Super-Critical CO2 Reactors, and Accelerator Driven Systems (Milko et al., 2018) can be adapted and harnessed for specific applications. The Generation IV nuclear reactors merit special attention. These reactors operate at temperatures in the range of 550–850°C (US Department of Energy, 2002) where a range of chemical reactions could be applied for producing value-added chemicals in a competitive and cost-effective manner.
Moreover, temperatures even higher have been considered with outlet temperatures in the range of 1,000°C for the Very High Temperature Reactor (VHTR) or of 1,300°C for the Ultra High Temperature Reactor (UHTR) (Fumizawa et al., 2009). The development of VHTR systems has been pursued through international collaboration in the Generation IV International Forum (GIF), since 1945 (Fütterer et al., 2014). The cooperation also addressed non-electric applications of VHTR power, such as the production of process steam in the short term (moderate temperature application) and thermochemical production of bulk hydrogen (high temperature application) in the longer term. However, current technologies for hydrogen production are energy intensive processes. Any attempt for integration to chemical processes should avoid leveraging inefficiencies, and efforts in the intensification of potential chemical processes should be incentivized. The VHTR inherent safety characteristics are considered the most valuable feature for integration to chemical processes. Additionally, VHTR's have reached a high technology readiness level, creating a great deal of research and development opportunities for chemical process integration.
A specific integrated energy system is one that integrates nuclear power plants (NPPs) with chemical plants, diversifying the product slate from the NPP beyond electricity into chemicals. As an example, the concept of the chemical heat pipe as an integrated energy system was proposed in Germany in the 1970s (Harth and Boltendahl, 1981). Conceptually, this process involves storing nuclear energy in the form of chemical energy and transporting it to a remote location where it could be converted back to energy or to chemical products (Figure 1). A specific example is the Adam-Eva Process (Höhlein et al., 1981) which involves the use of heat from a High Temperature Gas-cooled Nuclear Reactor, to drive an endothermic reaction (steam reforming of methane to produce a mixture of carbon monoxide and hydrogen, synthesis gas or syngas), through the Eva half-cycle of the process. The produced syngas was transported to a different location and converted through an exothermic reaction (the Adam half-cycle), for instance into methane, producing additional heat that could be used for electricity generation or direct heating (Figure 2).
Figure 2. Schematic representation of the Adam—Eva Process (original drawing based on concepts from Höhlein et al., 1981).
Complementary to the Adam-Eva process, Power-to-Gas pathways were developed in Japan (Hashimoto et al., 1999) and more recently in Europe (Buchholz et al., 2014) as a long-term solution for renewable electricity storage. Power-to-Gas pathways involve producing gases such as CH4 and H2 from carbon sources and renewable electricity (Figure 3). The process chain first proposed in Japan in the 1980–1990s included a CO2 recycling pilot plant built in 2003 and using seawater (Hashimoto et al., 2014). The use of biomass and CO2 as feedstocks to produce gases such as CH4 and H2 received significant interest in Europe (Kaltschmitt et al., 2009; Sterner, 2009; Hoekman et al., 2010; Graf et al., 2011; Budzianowski, 2012; Götz et al., 2016). These processing pathways were reviewed by Kaltschmitt et al. (2009) and summarized by Sterner (2009), as shown in Figure 4. These pathways support the concept of a bioeconomy in which the manufacture of materials, chemical products and energy—is based on biological and renewable resources (Mccormick and Kautto, 2013). Briefly, the concept of a bioeconomy involves the use of carbon-based renewable feedstocks obtained from biological resources of plant or animal origin as well as wastes of renewable origin, which are processed in a mechanical, biomechanical or thermal, and chemical manner to produce fuels, chemicals, bioproducts, food, and fodder as well as cosmetics and medicines (Adamowicz, 2017) (Figure 5). Economic sectors that are influenced by the bioeconomy concept are food, medicines, industrial and energy products and services, industrial biotechnologies, biofuels, biorefineries, chemical industry, transport or recycling [(European Technology Platforms (ETP), 2011; Obama, 2012)]. The concept of a Bioeconomy is closely aligned with that of a circular economy (CE). According to the 7th Environmental Action Program proposed by the European Commission, a circular economy (CE) is one “where nothing is wasted and where natural resources are managed sustainably, and biodiversity is protected, valued and restored in ways that enhance our society's resilience; and our low-carbon growth has long been decoupled from resource use, setting the pace for a safe and sustainable global society” (European Commission, 2014).
Figure 3. Schematic representation of the processing pathways for producing gases such as CH4 and H2 from carbon sources and renewable electricity (original drawing based on concepts from Götz et al., 2016).
Figure 4. Simplified description of bioenergy pathways (adapted from Sterner, 2009; with permission from the author).
Accordingly, the CE value chain was distinguished by a closed loop of material flow and was suggested to be driven by renewable energy (Kalmykova et al., 2018). In Figure 6, we extend this proposition to include carbon-neutral energy resources. CE is seen as a promoter for economic growth by creating new businesses and job opportunities, saving materials' cost, dampening price volatility, and improving the security of supply while at the same time reducing environmental pressures and impacts (Olabi, 2019). Kalmykova has reviewed the diverging approaches that hampered CE implementation and has also pointed out the lack of analysis of the available CE implementation strategies and experiences (Kalmykova et al., 2018). A more recent assessment of CE throughout a set of indicators concluded that most of the implementation strategies were focused on the preservation of materials, especially recycling. Further indicating that, although recycling was essential to the economy, it was not the only aspect of a sustainable CE. Similar conclusions were reached by the European Economic and Social Committee, in this case analysis concentrated on waste data (Lohan and Kylä-Harakka-Ruonala, 2018). In this regard, only a fraction of the waste separated for recycling will turn into the recycled material, whereas the efficiency and quality of those materials and processes were not assessed. Furthermore, while functionality and product sharing could be assessed by methodologies such as Life Cycle Assessments (LCA), so far these have not been covered. Nevertheless, new comprehensive methodologies are needed for the evaluation of strategies involving recycling, reuse, repurposing, multifunctionality, and co-production (Moraga et al., 2019). CE strategies that demand socio-institutional changes in the product chain increase the complexity of the involved challenges. All these issues and challenges are relevant to CO2 utilization or reuse.
Figure 6. Value chain of the circular economy (original drawing based on concepts from Kalmykova et al., 2018).
At this point, one could bring the topic of CO2 utilization or reuse to establish a connection between the Bioeconomy and the Circular Economy since one of the causes for the lack of circularity of the Bioeconomy is the existing gap in the C-loop. In many instances, the carbon loop could be closed by integrating CO2 conversion processes. The primary motivation for closing the carbon loop emerges from the need to mitigate greenhouse gas-driven changes in climate. In this regard, CO2 emissions create an additional link between energy security and climate change since the Energy Sector is responsible for more than 60% of the global CO2 emissions. The reported CO2 global emissions exceeded the 36 gigaton range in 2017 (Global Carbon Project, 2020), from which more than 5 gigaton corresponded to the US (EIA, 2019).
One of the challenges in designing CO2 mitigation strategies is the stability or low reactivity of CO2 associated with a very low ΔG° of −396 kJ/mol, which contributes to the high energy intensity of its conversion reactions. CO2, as one of the most stable molecules on the planet, requires high temperature processes for its conversion. Thermal energy in chemical processes is typically provided by the combustion of fossil fuels such as coal and natural gas resulting in the release of a significant quantity of greenhouse gases, including carbon oxides. The processing and valorization of several thousand tons of CO2 and the rising need to decarbonize energy supply motivate the use of energy resources with low carbon emissions such as nuclear energy. Nuclear energy is a reliable, continuous, and low carbon energy resource. One of the unique features of a nuclear power plant is the availability of excess energy otherwise wasted, in the form of diverse sources, such as ionizing radiation, electrons, and heat. The economic incentives of producing chemicals by promoting reactions with ionizing radiation from NPPs have been recently assessed for the particular case of propylene production. A process concept consisting of using gamma radiation from a molten salt nuclear power reactor to promote the radiolysis of propane was techno-economically analyzed (Schmeda-Lopez et al., 2018). This review concerns the radiolytic behavior of CO2 in diverse chemical environments. Conversion pathways based on the radiolysis of CO2 can be taken as additional utilization alternatives of the many more needed for the production of chemicals and fuels, from gaseous waste streams. As mentioned above for the case of hydrogen production, energy intense processes, and leveraging inefficiencies through integration of chemical processes to NPPs have to be avoided. The use of irradiation in an alternative conversion pathway for CO2 might contribute to pave the way for the development of more energy efficient processes.
The exploration of energy resources available from NPPs, in the context of chemical transformations of CO2 for utilization, has been limited. Recent advancements in more versatile, flexible and safer reactors of different size scales and configurations present the potential for distributed synthesis of products and fuels from CO2. One representative example is that of Generation IV nuclear reactors, which have the potential to be operated at temperatures in the range of 550–850°C (Fütterer et al., 2014).
The effects of ionizing radiation on CO2 include excitation, ionization, neutralization, activation, and conversion via chemical reactions. The formation of atomic species is also accompanied by the production of molecular species and even polymeric products. Investigation of CO2 radiolysis phenomena started as early as 1920's (Lind and Bardwell, 1925; Hirschfelder and Taylor, 1938), with renewed interest in the 50s' (Harteck and Dondes, 1955, 1957) and then in the 60s' and 70s' (Anderson et al., 1962; Dominey and Palmer, 1963; Dominey and Wickham, 1971; Ali and Clay, 1976; Ikezoe and Sato, 1976; Kummler et al., 1977; Wickham et al., 1977). Prior interest in exploring the effects of ionizing radiation on CO2 was based on its potential use as a coolant or a moderator.
Early studies showed that under the effects of ionizing radiation (such as radon or in a nuclear reactor), CO2 decomposed producing carbon monoxide and oxygen (Reaction 1) (Harteck and Dondes, 1955). The influence of radiation on chemical transformations is represented using G-value. The G-value is the number of molecules, atoms or free radicals formed or lost per 100 eV of energy absorbed. In these studies, the extent of the decomposition of solid CO2 only reached 0.1%, with a G value of 9–10. The reverse reaction in which the products were recombined contributed to the observed low yield. Based on previously reported results from other research groups (Lind and Bardwell, 1925; Hirschfelder and Taylor, 1938), a reaction network represented by Reaction 1–11 was proposed to explain the observed yields.
G values of CO2 decomposition were determined with and without inhibitors at CO2 partial pressures of 1–50 atm, in the liquid state by pile radiation and by the impact of fission fragments (Harteck and Dondes, 1957). Irradiating the gas phase using pile radiation or the fission fragments resulted in a low G value of 0.005 at atmospheric pressure and increased to 0.5 at 3 atm. G values for the decomposition of liquid CO2 using pile radiation and fission fragments irradiation were found to be in the range of 4–5 at atmospheric pressure, while reaching up to 8.5 in the presence of an inhibitor or at higher pressures (Harteck and Dondes, 1957). Willis and Boyd expressed the primary, or physical stage of CO2 radiolysis by reaction 12, which includes the G values for each specie (Willis and Boyd, 1976):
The physical stage is followed by the physicochemical stage in which ionic reactions take place among the species created in the physical stage. Subsequently, radiolysis is completed by a chemical stage when a series of reactions occur mainly involving the oxygen atoms (Ikezoe et al., 1981).
A review of the extensive experimental literature published in the two decades of the 1960s' and 1970s' on the radiolytic decomposition of CO2 led to the development of a numerical model that tried to incorporate the energy deposition process, prescribed diffusion, and the chemical kinetics. The model considered 76 reactions and 19 species which describe the real time concentrations of ionic and neutral constituents in the irradiated reacting medium. This model predicts a deleterious effect of the presence of excess oxygen at CO concentrations at about 1%. The discrepancies between the model and the experimental observations were attributed to the uncertainties in the rate constants of several reactions, mainly the ion-electron three-body charge rearrangement reactions. The model also predicted the need for pressures above 40 atm to attain higher yields (Kummler et al., 1977).
Radiolysis of CO2 and H2O can be controlled by changing the dose rate and ionizing radiation type (Kalashnikov et al., 1992a). Advances in transient absorption spectroscopy helped delineate the mechanisms and kinetics of recombination reactions and the influence of additives or impurities on the yields of radiolytic products starting from various precursors of gaseous, liquid, supercritical, or solid states of CO2 (Kalashnikov et al., 1992b). In the following sections, the radiolytic behavior of CO2 in gas, supercritical and solid states is discussed.
As can be deduced from the previous discussion, early studies of the radiolysis of CO2 focused mainly on gas phase reactions, though some effort was devoted to ice, as well. CO2 in the gas phase appears stable under irradiation, unless high doses are employed through isotopic exchange between CO and CO2, indicating the occurrence of both decomposition as well as recombination reactions. Thus, in summary, gas phase reactions are characterized by complex mechanisms and low radical yields, which are assumed to be caused by recombination reactions. Additionally, the CO yield is lowered when the γ-radiolysis of CO2 process is carried out in glass vessels packed with glass spheres. This decreasing effect was attributed to the deactivation at the walls resulting in CO re-oxidation (Ali and Clay, 1976).
The two reducing species formed during the ionization of supercritical CO2 are a short-lived quasi-free electron and a multimer solvent radical anion . These reactive species were accompanied by a third long-lived neutral product that was associated with singlet carbon trioxide, CO3. The solvent radical anions can mediate the formation of complexes in supercritical CO2 containing dimers and trimers of water, acetonitrile, and alcohols (Shkrob and Sauer, 2001; Shkrob et al., 2002). The stability of the complexes is determined from the equilibrium constants of complexation (10–350 M−1), the reaction heat (−15 to −21 kJ/mol), and the negative reaction entropy. The stability of the complex increased with the dipole moment of the polar group and decreases with the substitution at the α-carbon. Additionally, the polar solutes did not directly react with the quasi-free electrons in scCO2 (Shkrob and Sauer, 2001), providing an alternative longer-lasting chemistry. Understanding the mechanism of radical formation provides insights into improving the yield. The proposed mechanism for electron radiolysis of scCO2 starts by ionization with G of about 5, then a solvent radical anion is formed by the trapping of the quasifree electrons by the solvent in <200 ps. Most of these pairs are comprised of the solvent hole and thermalized quasifree electron (G for the formation of in place of the quasifree electron is <2–3% of the total ion yield). It was possible to increase the yield of the solvent radical cations by the addition of SF6. There was considerable cross recombination of the quasifree electrons with non-geminate solvent holes that gradually accumulated in the reaction mixture. This cross recombination further shortens the electron lifetime and reduces the electric field effect on the solvent cation yield (Shkrob et al., 2002). These complexes can control the yields of specific molecules of interest.
The structures and optical properties of these solvent radical species have been predicted using the pulse radiolysis of scCO2 (Shkrob, 2002). Theoretical calculation and comparative analysis were the basis to propose the formation of the cation radical in supercritical CO2 and to predict that the dimer anion will occur as a stable electron center in dry ice. Although higher symmetric multimer cations, with a ladder-type structure might occur in the gas phase, it seems that their formation is not favored in the liquid. Apparently, the extended “ladder” structure is rapidly destroyed by molecular motions and collisions in the liquid (Shkrob, 2002).
Investigation of radiation-induced chemistries on dry ice was initially motivated by the possibility of these reactions occurring in extraterrestrial space since ice containing simple molecules such as H2O, CO, CO2, and NH3 are ubiquitous in the interstellar space. Exposed to ionizing radiation, these molecules could form radicals that combine to produce new molecular species. Laboratory studies and astronomical observations indicate that photolysis and radiolysis of this type of outer space ices can create simple molecules, such as CO, CO3, O3, H2CO3, and H2O2, and other more complex organic compounds such as formic acid, formaldehyde, and methanol.
The dissociation rate of CO2 to produce CO, CO3, and O3 was found to be linearly proportional to the electronic stopping power when irradiation of the pure solid CO2 was carried out using heavy ions such as Ti, Ni, and Xe ions. Under these conditions, the sputtering yield shows a quadric increase with electronic stopping power. This enhancement creates new dependencies to lifetimes of molecules, contributes to the release of molecules from the solid to the gas phase in dense molecular clouds, and controls the thickness of the solid or ice layer (Mejía et al., 2015).
Besides the astrophysical interests of studying the radiolysis of H2O:CO2 ices, there would be a preference for using water as a source of hydrogen for the reduction, hydrogenation and/or decomposition-hydrogenolysis reactions. The radiolysis of pure H2O and CO2 ices, induced by energetic (52 MeV) 58 ions, produced mainly H2O2 and CO, respectively. Mixed ices bearing CO2 and H2O produced CO3, O3, H2CO3, and H2CO and HCOOH in minor proportion. An interesting feature of these experiments is that the H2O2/H2O and CO2/CO ratios stabilized at 0.01 and 0.1, respectively. This stabilization behavior is independent of the initial H2O relative abundance and column density, and the initial CO2/H2O ratio, respectively (Pilling et al., 2010).
The influence of additives such as water, hydrocarbons, SO2 and NO2 on CO2 radiolysis have been investigated. These studies are reviewed in the following sections.
Water is usually co-present with CO2 as an impurity or in flue gas streams. If irradiation of such fluidic streams is of interest, then understanding the influence of water is important. Lower concentrations of CO were noted in wet CO2 compared to dry CO2. The formation of clustered ions such as (H2O)m(CO2)n contributes to the reverse reaction to produce CO2 (Ikezoe et al., 1981). The formation of radical anion of CO2, , a strong and versatile reductant was noted when the solvated electron in CH3CN is scavenged by CO2 (Grills and Lymar, 2018). This example demonstrates that aqueous chemistry can be tuned to direct the formation of specific radicals from CO2, and then into selective products or fuels.
Irradiation of solid CO2 in the presence of water and novel gases such as Ar, Kr, and Xe at 8–10 K aided the formation of CO and reduced the back reactions. High yields of stabilized oxygen atoms were noted in the radiolysis of CO2 in noble gas matrices. The IR spectra of HOCO and DOCO were first characterized in krypton and xenon matrices. It was concluded that the formation of HOCO was mainly due to the radiation-induced evolution of the weakly bound H2O···CO2 complexes. Weak intermolecular interactions contribute to the radiation-induced chemical processes in inert low temperature media (Ryazantsev and Feldman, 2015). Radiolysis of mixed gases including CO2 mixed with nitrogen dioxide and sulfur dioxide yielded lower oxygen concentrations (Anderson et al., 1962). The presence of metals was shown to enhance the γ-radiolysis of CO2 to produce CO (Watanabe et al., 2007). Lower energy electrons generated through the interactions of γ-photons with the coexisting metal materials enhances the decomposition of CO2 to CO (Watanabe et al., 2007).
The presence of hydrocarbons with CO2 has implications on the mechanisms of radiolysis that will affect the direct use of mixed gas streams for CO2 utilization. Trace amounts of methane at the level of ppm contribute to the radiolysis of CO2, resulting in the deposition of reactive carbon at interfaces of steel or graphite (Dyer and Moorse, 1982). The radiolysis of methane/carbon dioxide mixtures have been shown to produce a white, wax-like solid with an empirical formula of CH2O, assumed to be a polymer of formaldehyde (Lind and Bardwell, 1926). The major radiolytic products of methane, either alone or as a minor component in an inert mixture were hydrogen and ethane. Minor products include higher alkanes, ethane, and ethyne. Similarly, the formation of a polymeric liquid with a formula of CnH2n was noted (Lind and Bardwell, 1926; Honig and Sheppard, 1946). Detailed characterization of these polymeric compositions is needed so they can be treated to enhance the directed synthesis of desired products (Dyer and Moores, 1984). Trace organics such as butane, 2-methyl-propane, 2- 2′-dimethyl propane, acetaldehyde, and acetone are present in the system (Dyer and Moores, 1985). Further, CO production is evident in the radiation of CO2-C3H8 systems (Ikezoe and Sato, 1976). Time-resolved EPR spectroscopy of the pulse radiolysis of liquid alkanes showed the mechanisms leading to the formation of alkyl radicals. The mechanism is initiated by chemically-induced spin polarization, which increases with the shortening of the aliphatic chain. This polarization is expected to originate from the so-called ST- mechanism operating in the pairs of alkyl radicals and hydrogen atoms generated in the dissociation of excited alkane molecules (Shkrob and Trifunac, 1995a).
Radiolytic studies of pure hydrocarbons provide useful insights into the products and mechanisms that yield radicals of interest. Radical cations in liquid alkanes such as cyclohexane yield multiple ionic species. One of the key features of these reactions is the formation of high mobility ions that are scavenged by solutes (Trifunac et al., 1997). Time-resolved spin-echo-detected EPR studies of the pulse radiolysis of phenols, ArOH, in alkanes at 290 K revealed the formation of phenoxy radicals ArO produced in multiple mechanisms including the dissociative capture of electrons, alkane holes and via reaction of phenols with hydrogen atoms (Shkrob and Trifunac, 1995b; Shkrob et al., 2001). The use of fluorescence detected magnetic resonance (FDMR) has been applied to study ion-molecule reactions in squalane (Shkrob and Trifunac, 1996). Radiolysis of squalane yields a mobile solvent hole and normally diffusing olefin ions, with the latter being observed directly by FDMR. In the presence of a saturated solution of CO2 as an electron scavenger, there is an increased yield of delayed fluorescence observed. Time-resolved FDMR measurements of the radiolysis of squalane showed that the ion-molecule reactions of radical anions are somewhat faster than reactions of radical anions (Shkrob and Trifunac, 1996).
The radiolysis of hydrocarbons is relevant to the topic of the additive effect on CO2 because hydrocarbons have the potential to increase radical yields and subsequently impact the chemical transformations of CO2. For example, time-resolved EPR studies of pulse radiolysis of liquid alkanes have shown that alkyl radicals derived from alkane radical cations exceeds the statistical prediction. In summary, these findings demonstrated the significance of fast reactions involving radical cations, excited alkane molecules and hydrogen atoms in the formation of alkyl radicals (Shkrob and Trifunac, 1995a). Radical cations generated by the radiation of liquid hydrocarbons exhibit high mobility in time-resolved, pulse radiolysis and transient adsorption, photoconductivity, and magnetic resonance (Trifunac et al., 1997). Ionizing radiation of hydrocarbons produce excited hydrocarbon molecules and electro-hole pairs. Rapid charge recombination of radical species dominates the radiation chemistry and competes against other chemical transformations (Shkrob et al., 2001). Pulse radiolysis of methane in cryogenic fluids, examined with spin-echo and free induction decay, suggested that ion reactions dominate over energy transfer reactions underscoring the micro-heterogeneous nature of the reaction mixtures (Shkrob and Trifunac, 1995c).
Nitrogen dioxide, when added at levels as low as 0.5%, increased the yield of CO by reacting with O and C radicals to produce CO and NO (Reactions 13–15). This scavenging reactions of C and O with NO2 prevent the backward recombination reactions to produce CO2. Thus, NO2 is considered an inhibitor in these reactions.
The apparent stability of CO2 under high-energy radiation, in the presence of sulfur dioxide and nitrogen dioxide was evidenced by the measured G value for CO production. Both oxides, SO2 and NO2 act as scavengers rendering a G(CO) = 3.51 (±0.23) for γ-radiation, within a wide range of additives, partial pressures, and radiation doses (Anderson et al., 1962). The reported G(CO) value are shown to be in agreement with the value measured by a different γ-radiation source within experimental error of the dosimetry measurement method. Transient IR spectroscopy combined with pulse radiolysis has resulted in the first IR detection of in acetonitrile with an asymmetric stretch at 1,650 cm−1 (Grills and Lymar, 2018). The properties and reactivity of in acetonitrile are of interest in understanding electrochemical CO2 reduction in aprotic solvents. Recombination reactions with solvent-derived radicals, in neat CH3CN are responsible for the rapid decay of the anion radicals (~10 μs), while the addition of formate () substantially increased the radiation yield of the anion radicals.
Electron beam (EB) radiation of carbon dioxide in the presence of ethanol as an additive is reported to yield hydrogen, carbon monoxide, methane, ethane, and organic acids (Hosokawa et al., 2019). The CO2 conversion yield is ~15 times larger than that observed for gas phase radiolysis, which yields mainly CO (~0.1%), while the presence of ethanol produces additionally formic, acetic and propionic acids. The high dose rate EB irradiation with organic additives facilitates CO2 capture by radicals with improved CO2 conversion. CO2 decomposition to CO during γ-radiolysis is assisted by the addition of solid materials (Watanabe et al., 2007). Low energy electrons generated by the interactions of γ photons with co-existing metal materials are ejected into gaseous CO2 enhancing CO formation. A similar finding was reported for the γ-radiolysis of CO2 in glass vessels packed with glass spheres (Ali and Clay, 1976). The yield of CO was four-fold greater in a vessel packed with glass spheres compared to an unpacked vessel.
High-dose rate electron beam (EB) irradiation of an acid-decomposed CaCO3/additive ethanol mixture yielded H2, CO, CH4, C2H6 and organic acids as the products (Hosokawa et al., 2019). This pathway represents the potential for converting CO2 to CaCO3 followed by irradiation to direct the synthesis of organic compounds. Irradiation also presents the opportunity to enhance the recovery of CO2 via the decomposition of zinc and manganous carbonates. Higher yields of CO2 were achieved when zinc and manganous carbonates were irradiated prior to thermal decomposition (Meyer et al., 1973). However, the underlying molecular-scale mechanisms of this observation are not well-understood.
The utilization of renewable feedstocks and integration with CO2 utilization to produce low carbon energy carriers is gaining attention. For example, the pyrolytic conversion of biomass to a combustible gas bearing CO, H2, and CH4 has been extensively studied at temperatures above 500°C. Pyrolysis experiments were performed on untreated and irradiated biomass materials. Pyrolysis of untreated biomass yielded different percentages of CO2 and CH4, while irradiated biomass yielded pure methane. Nano-carbon black particles and amorphous structured carbon particles were the by-products of the pyrolysis of untreated and irradiated biomass materials (El Naggar et al., 2015). To enhance the use of lignin, irradiation of these materials was proposed. Sodium ligno-sulfonate in distilled water was used to prepare the lignin solution. The solution was placed in a Pyrex reaction vessel and irradiated with 60Co γ-rays at room temperature (Wickham et al., 1977). The OH radicals and oxygen generated during the irradiation of the sodium ligno-sulfonate solution aids the deconstruction of the lignin molecule (Nagai and Suzuki, 1978). Similarly, the degradation of calcium lignosulfonate to produce CO2, H2O and sulfates was aided by the formation of •OH radicals (Zhang et al., 2004). Further, irradiation of cellulose has been shown to reduce the temperature needed for the thermal decomposition of cellulose to produce valuable organic products via chain mechanisms (Ponomarev and Ershov, 2014). The mutations of microalgae such as Chlorella sp. were explored to enhance the biomass or lipid yield. Mutated microalgae produced more long-chain unsaturated fatty acids and less short-chain saturated fatty acids compared to untreated microalgae (Cheng et al., 2016).
CO2 utilization to produce useful organic compounds via irradiation is dependent on the (i) conversion of CO2 to transient species such as COO−/COOH and (ii) the formation of a suitable organic radical (R.) (Getoff and Schenck, 1967). Carboxylic acid can be successfully synthesized by combining both radical types as shown by the reactions below:
Radiation induced carboxylation of organic compounds has been harnessed to produce amino acids from amines (Getoff and Schenck, 1967), salicylic acid from phenol (Getoff, 1965; Gütlbauer and Getoff, 1965; Krapfenbauer and Getoff, 1997), and malonic acid from acetic acid (Getoff et al., 1997, 2003; Krapfenbauer and Getoff, 1997; Getoff, 1999). Linear low density polyethylene was γ-irradiated in the presence of CO2 under supercritical conditions to graft carboxylated functional groups to the polyethylene chains (Dispenza et al., 1997). Supercritical CO2 was also used to charge maleic anhydride (MA) and dicumyl peroxide (DCP) into polypropylene matrices. MA was grafted into polymer chains through gamma irradiation in CO2 atmosphere (Spadaro et al., 2000). γ-radiation induced polymerization of vinyl monomers in dense CO2 yields spherical particles with narrow particle size distributions (Caputo et al., 2002).
Further, irradiation can also contribute to the release of CO2. For example, γ-irradiation of tannins extracted from Pinus caribaea bark suggested the loss of carbonyl groups and decarboxylation corresponding to the release of CO2 from the molecule (Velasco et al., 2014). Radiolysis of malonic acid yielded carbon dioxide, acetic acid, and di- and tricarboxylic acids (Cruz-Castañeda et al., 2015). The major decomposition products from the radiation of solid succinic acid are carbon dioxide, succinic semi-aldehyde, propionic acid and water, along with the formation of a polymer, hydroxyacids, CO, and H2 (Bartoníček, 1973). Radiolysis of hydrated and anhydrous oxalic acid, and Li2C2O4, Na2C2O4, and K2C2O4 yielded CO2 and water (Dougherty and Gottschall, 1976). EPR studies of radicals generated by γ-radiation in carbonate containing nanocrystalline hydroxyapatites resulted in , , , and O− radicals (Sadło et al., 2012).
Currently, technologies based on radiolytic approaches for CO2 conversion do not exist. For this reason, this section focuses on the generated information and the discovered knowledge that can contribute to technology development and on the findings that could lead to technological applications.
Among organic compounds, the high stability of CO2 also makes it the least reactive among carbon containing molecules. While several technologies for capturing CO2 from point sources exist, pathways to directly convert heterogenous CO2-bearing gas streams to high-value streams are limited. Irradiation of CO2 is one approach to activate the CO2 molecule or to decompose it from its fully oxidized form to a reduced form. For example, radiation induced CO2 reduction in iron containing solutions yielded CO as well as saturated hydrocarbons such as CH4, C2H6, C3H8, and C4H10 (Fujita and Matsuura, 1994). Gamma radiation of a solution suspended with iron powder and saturated with CO2 yielded H2 as the dominant product (Fujita et al., 1994). The effect of γ-rays and metal ion additives has been studied using solutions suspended with iron powder and saturated with CO2. The products were H2, CO and several hydrocarbons, with CO being formed only under irradiation, unlike other products (Fujita et al., 1995, 1996a). Integrated radiolytic pathways in complex environments bearing CO2 and catalysts yielded important and transformative insights.
The incorporation of CO2 via carboxylation reactions induced by γ-radiation has been demonstrated in several instances. Supercritical CO2 used as solvent media for the reaction acts beyond its solvency power, as a co-reactant due to the activation effect of the irradiation. In the case of linear low-density polyethylene, in supercritical CO2 under γ-radiation resulted in grafted carboxylated functions to the polyethylene chains (Dispenza et al., 1997). Supercritical CO2 facilitated the γ-radiation induced maleation of polypropylene. Maleic anhydride and dicumyl peroxide were the grafting co-reactants for this functionalization reaction (Spadaro et al., 2000). Supercritical CO2 has been also used to take advantage of dispersion polymerization reactions, which can be induced by ionizing radiation. Free radicals created by γ-radiation are key reactive species for the initialization of polymerization reactions. Methyl methacrylate as monomer, 2,2′-azobis(isobutyronitrile) as initiator and polysiloxanes as dispersing stabilizers were used to prove this concept producing regular spherical particles (average diameter of 2.5 μm) with narrow particle size distribution (Caputo et al., 2002). The electron-induced radiolysis of methanol at ~350°C resulted in the formation of ethylene glycol, formaldehyde, dimethyl ether, methane, CO2, CO, and hydroxyl methyl radical (CH2OH) (Sullivan et al., 2016). This result needs to be considered when developing novel irradiation-induced chemical pathways for the hydrogenation of CO2 to produce methanol.
In this context, the role of predictive modeling of irradiation-based reaction pathways is essential. In an effort to understand synthetic pathways that might have taken place as prebiotic chemistry on the primeval Earth, theoretical modeling of chemical systems subjected to high energy sources (examples include UV, ionizing radiation, and plasma sparks) was carried out, with randomly generated chemical networks. The model tried to solve multi-component reaction systems with no a priori knowledge of reacting species, and the intermediates involved if system components were sufficiently interconnected. The results indicated that complex mixtures of compounds could be generated (Dondi et al., 2012). Such predictive modeling studies can be used in the rational design of irradiation-induced pathways for CO2 conversion.
The direct reduction of CO2 to CO using solid carbon (the reversed Boudouard reaction, Reaction 19) is a desirable reaction for CO2 remediation. However, this reaction is highly endothermic (ΔH = 172 kJ/mol) and requires temperatures above 700°C, to be carried out under convective heating. This challenge is overcome by microwave radiation. The apparent activation energy of CO2 irradiation by conventional convective heating is 118.4 kJ/mol, which is lowered to 38.5 kJ/mol by microwave radiation. Microwave radiation facilitates the conversion of CO2 to CO at temperatures as low as 213°C (Hunt et al., 2013). The observed enhanced reactivity was explained by a mechanism involving the interaction of the CO2 gas molecules, with a steady-state concentration of electron–hole pairs present at the surface of the carbon. The high space-charge heating of the microwaves to solid carbon contributes to enhanced reactivity. This unique microwave-induced heating might lead to energy savings for driving a general class of gas–carbon reactions.
To produce hydrocarbons via hydrogenation within a decarbonizing strategy, H2 from a low-carbon emitting source of energy or renewable energy source is needed. The ability to harness H2 for producing synthetic fuels via nuclear energy has been explored. One approach to produce H2 is via water splitting. Further, new processes that could take advantage of available energy sources such as radiation, electrons and heat in nuclear power plants via thermochemical and electrochemical splitting cycles are being considered (Gomberg and Gordus, 1982). For example, H2 and CO were formed by reacting steam with graphite, activated charcoal or semicoke at temperatures between 20 and 500°C via γ-radiation. This temperature range is lower compared to conventional gasification pathways due to the formation of active radicals of H2O and CO2. The gasification of semicoke yielded the highest rates of H2 and CO formation. In general, the rate of the gasification reaction (under γ-rays) was observed to depend on the type of carbon (Mustafaev et al., 1988), as opposed to in thermally driven gasification (Wigmans et al., 1981). The difference in reactivity of the different types of carbon was associated with their structural features. For example, only edge-sites were active on graphite, defects increased the reactivity of activated carbon and pre-irradiation decreased the reactivity of semicoke (Mustafaev et al., 1988). Given the versatile uses of carbons as catalysts and adsorbents, the adsorption behavior of these materials was studied using irradiation. Irradiation doses of 80 and 100 Mrad increases the pore size of carbons. This change in pore size is attributed to the radiolytic decomposition of oxygen complexes located on the surfaces of steam-activated carbons (El-Shobaky et al., 1987).
A few radiolytic pathways involving CO2 have been patented. These approaches include the incorporation of nitrogen oxide to scavenge oxygen and prevent recombination and back reactions of CO2 radiolysis (Lewis et al., 1979). Alpha-radiolysis of pure carbon dioxide has been proposed to produce CO. At a pressure of 25.2–25.8 atm, 12 molecules of CO are produced per 100 eV, which is the maximum chemical yield of CO (Kalinichenko et al., 1989). Further, the radiolysis of water vapor to produce H2 was also proposed (Kalinichenko et al., 1993). The potential of combining carbon dioxide radiolysis with the water-gas shift reaction for hydrogen production has been proposed based on the energy efficiency attained through the radiolysis process (Ikezoe et al., 1982).
While irradiation pathways facilitate conversion at lower temperatures due to the formation of reactive intermediates, recombination and back reactions may result in low radical yields. The use of various forms of catalysis including but not limited to homogeneous, heterogeneous, electrocatalysis and/or biocatalysis has been proposed to achieve mechanistic controls on irradiation reactions. For example, heterogeneous radiolysis of CO2 in the presence of zeolites such as CaA, NaX, NaY, LiNaY, and BaM showed that the catalytic action of oxides in radiolytic processes involves transferring the energy of ionizing radiation absorbed by a catalyst to an adsorbate. Decomposition of CO2 to CO is enhanced in the presence of these zeolites by the creation of surface defects holding (+) or (–) charge that could be transferred to adsorbed species (Garibov et al., 1987). The surface and catalytic properties of metal oxides are enhanced by doping the surfaces with MoO3 and V2O5. γ-irradiation reduced the particle size of manganese oxide, enhanced the specific surface areas, reduced the amount of surface excess oxygen and decreased the catalytic activities (El-Shobaky and Shaheen, 2003). A CO2 reduction electrocatalyst was prepared by the condensed-phase pulse radiolysis of manganese complexes. The dissolution of fac-MnBr(tBu2-bpy)(CO)3 precatalyst in CH3CN, in the presence of excess Mn-formate produced [Mn(tBu2-bpy)(CO)3]2, upon one electron reduction. The Mn free radicals dimerized selectively forming Mn–Mn dimer centers, with very specific catalytic characteristics (Grills et al., 2014). Photo-irradiated metal oxide semiconductors, such as TiO2, which convert CO2 to CH4 are a representative example of how two formyl radicals or glycolaldehyde combine to produce methane. Complex organic molecules such as glycolaldehyde, acetaldehyde, and methylformate are also co-produced (Shkrob et al., 2012a). This reduction was explained as essentially involving reaction 20, in which the reacting heteroatom (B) is transferred to the metal center of the catalyst. The CO2 photocatalytic methanogenesis on metal oxides involves such reactions on its path to methane formation. Furthermore, the mode of CO2 surface binding was suggested to be critically important. In fact, the mode of chemisorption of the radical (which is the reaction intermediate of one-electron reduction of CO2) fully determines the outcome: if this radical is doubly bound through its oxygens to the metal ions at the surface, this radical can be further reduced to formate. If it is singly bound or physisorbed by the oxide surface, this reduction is stalled (Shkrob et al., 2012b).
A potential difference between electrodes was induced by γ-radiation when the electrodes were immersed in solutions purged with Ar, CO2, O2, and N2O, and one of the electrodes was subject to irradiation. This potential difference was negative for the first three gases and positive for the last one and was associated, respectively, with H2 and H+ generated through radiolysis. Suppression of the purging gas (except for O2) and/or the irradiation ceased the potential difference. The application of these results has been proposed for the mitigation of intergranular stress corrosion cracking (IGSCC) or irradiation assisted stress corrosion cracking (IASCC), found in some nuclear power plants (Fujita et al., 1997). Relevant to corrosion in nuclear power plants, the reactions induced by γ-radiation on systems like CO/CO2/graphite (Wickham et al., 1977) and H2O/CO2/iron (Fujita et al., 1994; Domae et al., 2014) are of significant importance for gas-cooled and water-cooled reactors, respectively. In the former, the stable ions in the system are and , and when traces of CO are present and (CO2·CO·CO)+ species are formed. In the proposed corrosion mechanism of the graphite system, the positively charged irradiated CO2 radicals are the oxidizing species. A need for further work upon gas-phase and surface ion neutralization processes is identified. Although the effects of the added CO were accounted for, in both gas-phase systems and in graphite attack, areas of uncertainty remain in explaining the difference between the yield of the isotope exchange reaction *CO + CO2 ⇌ CO + *CO2 and the ion yield in such systems (Wickham et al., 1977).
For the latter, Primary Water Stress Corrosion Cracking (PWSCC) has been considered as one of the most important aging issues in Pressurized Water Reactor (PWR) systems. PWSCC has been associated with high concentrations of dissolved hydrogen. While methanol has been used for testing PWSCC (Domae et al., 2014), the above reported studies indicated the formation of hydrogen during the co-radiolysis of H2O and CO2. Furthermore, during the radiation-induced conversion of CO2 to CO and hydrocarbon, using a solution suspended with iron powder and saturated with CO2, H2 became the most dominant product of all, due to the corrosion enhanced by γ-rays (Fujita et al., 1994). Under these conditions, the effect of γ-rays on the CO2-saturated solution was to decrease the pH by almost 2.5, and the rate of H+ generation was collectively enhanced 27 times as much as that due to radiolysis alone (Fujita et al., 1996b).
While radiolytic pathways have been extensively investigated for applications related to treating contaminated water resources and polymerization, there is a limited fundamental understanding of approaches to (i) activate stable and inert molecules into their free radical states, (ii) achieve tunable controls on the combination reactions associated with the use of these radicals, and (iii) direct the synthesis of targeted molecules. The need to create this new understanding of free radical chemical transformations with the objective of directing specific reaction pathways still exist. Additionally, addressing these needs also requires the advancement and integration of in-operando characterization methods, validated modeling of the chemical pathways, and the synthesis of novel materials (catalysts, electrodes, electrocatalysts, additives, etc.) to direct specific reaction pathways. In the context of CO2 conversion to chemicals, CO2 is the target molecule, while water, methane, carbon or hydrogen are relevant co-reactants, for the production of syngas, chemical building blocks, or higher order hydrocarbons.
Our suggestion is to address these needs and fill these knowledge gaps, through direct efforts of multidisciplinary teams in the following areas: (i) determination of the kinetics and mechanisms of free radicals generation, and of the recombination and back reactions from heterogeneous CO2-bearing feedstocks, (ii) directing new pathways for free radical transformations, and (iii) predictive and analytical modeling of the free radical generation and transformations. Developing predictive controls on radiolytic conversions of small molecules such as CO2 is now possible by determining the ultrafast transformations associated with producing radicals (Couceiro et al., 1990; Crowell et al., 2004; Oulianov et al., 2007). Advancements in nanosecond time-resolved infrared (TRIR) detection methods for pulse radiolysis (Grills et al., 2015) and laser flash photolysis (Dyer et al., 2003) allow us to delineate the mechanisms underlying radiolytic and catalytic processes at time scales to the order of picoseconds and nanoseconds. Picosecond and nanosecond time-resolved infrared (TRIR) spectroscopy measurements based on combining UV flash photolysis and fast infrared detection now allow us to probe excited state and detect reaction intermediates (Kuimova et al., 2003). These techniques can be applied on a wide range of states of matter including but not limited to supercritical fluids, gases, and liquids. These ultrafast measurements will allow us to design processes to selectively trap ions in molecular cavities (Shkrob and Schlueter, 2006), determine the rates of ion-molecule reactions, ultrafast charge transfer mechanisms and the release of high mobility ions (Shkrob et al., 1996a,b,c). The mechanisms underlying isotope exchange reactions such as that of 13C (Dominey and Wickham, 1971), 14C (Dominey and Palmer, 1963) and 18O (Dominey and Wickham, 1971). In addition to radiolytic pathways, electrocatalytic reactions associated with CO2 reduction and the underlying protonation pathways can be probed using time-resolved infrared (TRIR) spectroscopy combined with pulse-radiolysis (PR-TRIR) approaches (Ngo et al., 2017). The design and use of novel ligands in the photo- and electrochemical reduction of CO2 to produce formate and methanol via hydrogenation can be informed by these techniques (Wang et al., 2015). Deconstructing, tuning, accelerating specific pathways while circumventing less desirable pathways will allow us to design more efficient reaction systems (Perkins et al., 2014; Simmons et al., 2015) for radiation-induced chemical conversions of CO2. The influence of radiolytic reactions on the corrosion of reactor materials (Wickham et al., 1977) requires further investigation.
Rational design of radiolytic pathways has the potential to advance the distributed production of chemicals using anthropogenic CO2. Specific examples include the radiation-induced carboxylation of amines, phenol, and acetic acid to produce amino acids or short chain proteins, salicylic acid, and malonic acid, respectively (Getoff, 2006). One of the unique features of radiolytic pathways is that they can be designed to deconstruct complex molecules into simpler molecules. For example, chemicals that can be made from CO2 via carboxylation such as malonic acid, succinic acid, and oxalic acid can be decarboxylated into their respective building blocks (Bartoníček, 1973; Dougherty and Gottschall, 1976; Velasco et al., 2014; Cruz-Castañeda et al., 2015). In addition to producing chemicals, γ-irradiation has been shown to have promising potential to produce fuel gas. Recent studies on the conversion of biomass and varying compositions of gas mixtures such as CO2 and CH4 showed that pure methane could be produced at 300°C with gamma irradiation as opposed to conventional pyrolysis at temperatures above 500°C (El Naggar et al., 2015). The promise of multifunctional processes for producing fuels and chemicals via irradiation can be realized by achieving controls on the underlying ultrafast propagation and termination reactions. The design of process configurations for producing fuels and chemicals via CO2 radiolysis is a significant knowledge gap. A mechanistic understanding of the desirable products and their kinetics is needed to develop the processes compatible with the use of nuclear energy. To realize the promising potential of harnessing nuclear energy for CO2 utilization, the challenges of nuclear waste disposal and safe and secure use of nuclear fuel need to be addressed at the scientific and societal levels.
As nuclear reactors become increasingly advanced, smaller and modular, the promise of using low carbon-emitting energy sources to drive distributed manufacturing of chemicals and fuels from anthropogenic CO2 can be realized. The activation and/or the conversion of CO2, a relatively inert molecule to produce fuels and chemicals of the future, can be achieved via irradiation. The relevant reactions are summarized in Table 1. The versatility of radiolytic pathways can be harnessed to direct carboxylation reactions with CO2 to produce amino acids or short chain proteins, salicylic acid, and malonic acid, for instance. Alternatively, these chemicals can be decarboxylated into their respective building blocks. This versatility can be utilized to direct the selectivity and yield of specific chemicals. One of the scientific challenges in converting CO2 to useful chemicals and fuels using irradiation lies in achieving predictive controls on the propagation and termination reactions. The irradiation of CO2 to produce highly reactive radicals is easily influenced by the presence of other compounds, even at very low concentrations. The potential for combining the radiolysis of CO2 with the water-gas-shift reaction for H2 production exists. The use of nuclear energy on renewable feedstocks and/or anthropogenic CO2 to produce H2 is a highly promising but less explored pathway. Designing chemical processes that can be coupled to nuclear reactors require the development of predictive controls on the radiolytic conversions of CO2 and the delineation of the underlying mechanisms. Toward this end, ultrafast characterization of the reactive radicals and the propagation and termination mechanisms is of significant interest. This approach will enable the design of multifunctional processes for producing fuels and chemicals using CO2 via irradiation. The directed synthesis of amino acids or short chain proteins, lactic acid, salicylic acid, and malonic acid through CO2 radiolysis is promising. Achieving mechanistic controls on the generation of CO from CO2 in the presence of varying additives will open up significant scientific opportunities in producing fuels via CO2 radiolysis. Lactic acid is an intermediate in the synthesis of many organic molecules and CO (with H2) is the precursor for synthetic fuels. In this context, nuclear energy presents a pathway to realize the concept of distributed fuel and chemical generation from CO2 captured from a wide range of dilute and distributed sources.
All the authors created the manuscript outline, realized the literature search and papers to be reviewed, determined the main contribution of each reviewed article to the manuscript, reviewed about 60% of the cited literature, and coordinated the participation of other co-authors.
The authors declare that the research was conducted in the absence of any commercial or financial relationships that could be construed as a potential conflict of interest.
One of the co-authors, MR-C acknowledges the support for the elaboration of this manuscript to the Laboratory Directed Research & Development (LDRD) Program of Battelle Energy Alliance, LLC under DOE Idaho Operations Office Contract No. DE-AC07-05ID14517. The United States Government retains and the publisher, by accepting the paper for publication, acknowledges that the United States Government retains a non-exclusive, paid-up, irrevocable, world-wide license to publish, or reproduce the published form of this manuscript, or allow others to do so, for United States Government purposes. STI Number: INL/JOU-20-57637.
Adamowicz, M. (2017). Bioeconomy - concept, application and perspectives' (‘biogospodarka - koncepcja, zastosowanie i perspektywy’). Zagadnienia Ekon. Rolnej 1, 29–49. doi: 10.5604/00441600.1232987
Ali, S. M., and Clay, P. G. (1976). The γ-radiolysis of CO2 in packed vessels. Int. J. Radiat. Phys. Chem. 8, 603–607. doi: 10.1016/0020-7055(76)90029-2
Anderson, A. R., Best, J. V., and Dominey, D. A. (1962). Radiolysis of carbon dioxide. J. Chem. Soc. 3498–3503. doi: 10.1039/jr9620003498
Bartoníček, B. (1973). Gamma radiolysis of solid succinic acid. Int. J. Radiat. Phys. Chem. 5, 361–369. doi: 10.1016/0020-7055(73)90061-2
Buchholz, O. S., Van Der Ham, A. G. J., Veneman, R., Brilman, D. W. F., and Kersten, S. R. A. (2014). “Power-to-gas: storing surplus electrical energy a design study,” in Proceedings of 12th International Conference on Greenhouse Gas Control Technologies, GHGT (Amsterdam: Elsevier Ltd.).
Budzianowski, W. M. (2012). Negative carbon intensity of renewable energy technologies involving biomass or carbon dioxide as inputs. Renew. Sust. Energy Rev. 16, 6507–6521. doi: 10.1016/j.rser.2012.08.016
Caputo, G., Galia, A., Scrò, F., Spadaro, G., and Filardo, G. (2002). Gamma radiation induced polymerization of vinyl monomers in dense CO2. Radiat. Phys. Chem. 63, 45–51. doi: 10.1016/S0969-806X(01)00480-7
Chen, X., Mcelroy, M. B., Wu, Q., Shu, Y., and Xue, Y. (2019). Transition towards higher penetration of renewables: an overview of interlinked technical, environmental and socio-economic challenges. J. Mod. Power Syst. Clean Energy 7, 1–8. doi: 10.1007/s40565-018-0438-9
Cheng, J., Lu, H., Huang, Y., Li, K., Huang, R., Zhou, J., et al. (2016). Enhancing growth rate and lipid yield of chlorella with nuclear irradiation under high salt and CO2 stress. Bioresour. Technol. 203, 220–227. doi: 10.1016/j.biortech.2015.12.032
Couceiro, I. B., Zanon, R. A. D., Huang, Y. K., and Massone, C. A. (1990). Experimental analysis of the γ-ray ionization effects of a dc discharge CO2 laser. Optics Laser Technol. 22, 348–350. doi: 10.1016/0030-3992(90)90079-J
Crowell, R. A., Gosztola, D. J., Shkrob, I. A., Oulianov, D. A., Jonah, C. D., and Rajh, T. (2004). Ultrafast processes in radiation chemistry. Radiat. Phys. Chem. 70, 501–509. doi: 10.1016/j.radphyschem.2003.12.028
Cruz-Castañeda, J., Negrón-Mendoza, A., Frías, D., Colín-García, M., Heredia, A., Ramos-Bernal, S., et al. (2015). Chemical evolution studies: the radiolysis and thermal decomposition of malonic acid. J. Radioanal. Nucl. Chem. 304, 219–225. doi: 10.1007/s10967-014-3711-z
Dispenza, C., Filardo, G., Silvestri, G., and Spadaro, G. (1997). Carboxylation of linear low density polyethylene through gamma irradiation in presence of supercritical carbon dioxide. Grafted groups analysis via derivatization procedures. Colloid Polymer Sci. 275, 390–395. doi: 10.1007/s003960050096
Domae, M., Hojo, K., and Sugino, W. (2014). “Water chemistry technology of methanol addition in pwr primary systems - radiolysis of methanol solution at 320°C,” in Proceedings of 2014 22nd International Conference on Nuclear Engineering, ICONE 2014 [Prague: American Society of Mechanical Engineers (ASME)], 1.
Dominey, D. A., and Palmer, T. F. (1963). Radiolytic exchange of carbon-14 between carbon monoxide and carbon dioxide. Discuss. Faraday Soc. 36, 35–45. doi: 10.1039/df9633600035
Dominey, D. A., and Wickham, A. J. (1971). γ-radiation induced isotope exchange in the carbon monoxide-carbon dioxide system. Studies in silica vessels. Trans. Faraday Soc. 67, 2598–2606. doi: 10.1039/tf9716702598
Dondi, D., Merli, D., Albini, A., Zeffiro, A., and Serpone, N. (2012). Chemical reaction networks as a model to describe uvc- and radiolytically-induced reactions of simple compounds. Photochem. Photobiol. Sci. 11, 835–842. doi: 10.1039/c2pp00005a
Dougherty, P. L., and Gottschall, W. C. Jr. (1976). Gamma radiolysis of oxalic acid and selected metal oxalates. Radiat. Res. 68, 229–233. doi: 10.2307/3574473
Dyer, A., and Moores, G. E. (1984). The radiolysis of simple gas mixtures-ii. The production of lower alkanes and alkenes. Radiat. Phys. Chem. 23, 515–522. doi: 10.1016/0146-5724(84)90153-5
Dyer, A., and Moores, G. E. (1985). The radiolysis of simple gas mixtures-iii. The production of “trace organics”. Radiat. Phys. Chem. 26, 267–272. doi: 10.1016/0146-5724(85)90063-9
Dyer, A., and Moorse, G. E. (1982). The radiolysis of simple gas mixtures-i. Rates of production and destruction of methane in mixtures with carbon dioxide as a major constituent. Radiat. Phys. Chem. 20, 315–321. doi: 10.1016/0146-5724(82)90121-2
Dyer, J., Blau, W. J., Coates, C. G., Creely, C. M., Gavey, J. D., George, M. W., et al. (2003). The photophysics of fac-[re(CO)3(dppz)(py)]+ in CH3CN: a comparative picosecond flash photolysis, transient infrared, transient resonance raman and density functional theoretical study. Photochem. Photobiol. Sci. 2, 542–554. doi: 10.1039/b212628a
EIA (2019). Short-Term Energy Outlook. Washington, DC: US Energy Information Administration. Available online at: www.eia.gov/outlooks/steo/report/renew_co2.php
El Naggar, A. M. A., El Sayed, H. A., Elsalamony, R. A., and Elrazak, A. A. (2015). Biomass to fuel gas conversion through a low pyrolysis temperature induced by gamma radiation: an experimental and simulative study. RSC Adv. 5, 77897–77905. doi: 10.1039/C5RA10621D
El-Shobaky, G. A., El-Nabarawy, T., and Dessouki, A. M. (1987). Adsorption properties of gamma-irradiated carbons. Radiat. Phys. Chem. 30, 161–163. doi: 10.1016/1359-0197(87)90072-5
El-Shobaky, H. G., and Shaheen, W. M. (2003). Effect of γ-irradiation and doping with MoO3 and V2O5 on surface and catalytic properties of manganese oxides. Radiat. Phys. Chem. 66, 55–65. doi: 10.1016/S0969-806X(02)00258-X
European Commission (2014). “General union environment action programme to 2020 ‘living well, within the limits of our planet’,” in 1386/2013/EU. 2013, The 7th Environment Action Programme: European Union, 30. Available online at: https://eur-lex.europa.eu/legal-content/EN/TXT/?uri=CELEX:32013D1386
European Technology Platforms (ETP) (2011). The European Bioeconomy in 2030. Report No. KBBE-2008-226526. Brussels: European Union. Available online at: https://www.greengrowthknowledge.org/resource/european-bioeconomy-2030-delivering-sustainable-growth-addressing-grand-societal-challenges
Fujita, N., Fukuda, Y., Matsuura, C., and Hiroishi, D. (1995). The effect of radiation and metal ion addition on the CO2 reducing reaction in iron containing water. Radiat. Phys. Chem. 46, 345–352. doi: 10.1016/0969-806X(94)00133-5
Fujita, N., Fukuda, Y., Matsuura, C., and Saigo, K. (1996a). Changes in ph and redox potential during radiation-induced CO2 reduction in an aqueous solution containing iron powder. Radiat. Phys. Chem. 47, 543–549. doi: 10.1016/0969-806X(95)00102-4
Fujita, N., Fukuda, Y., Matsuura, C., and Saigo, K. (1996b). Radiation-enhanced h+ generation in iron-containing solution saturated with CO2. Radiat. Phys. Chem. 48, 297–304. doi: 10.1016/0969-806X(95)00446-5
Fujita, N., and Matsuura, C. (1994). Radiation induced reduction of CO2 in iron containing solution. Radiat. Phys. Chem. 43, 205–213. doi: 10.1016/0969-806X(94)90180-5
Fujita, N., Matsuura, C., and Saigo, K. (1997). Radiation-induced potential difference between electrodes with and without gamma rays. Radiat. Phys. Chem.49, 357–362. doi: 10.1016/S0969-806X(96)00063-1
Fujita, N., Morita, H., Matsuura, C., and Hiroishi, D. (1994). Radiation induced CO2 reduction in an aqueous medium suspended with iron powder. Radiat. Phys. Chem. 44, 349–357. doi: 10.1016/0969-806X(94)90072-8
Fumizawa, M., Kosuge, Y., and Horiuchi, H. (2009). “Thermal evaluation for a ultra high temperature reactor with pebble type fuels,” in Proceedings of 17th International Conference on Nuclear Engineering, ICONE17 (Brussels), 865–872.
Fütterer, M. A., Fu, L., Sink, C., De Groot, S., Pouchon, M., Kim, Y. W., et al. (2014). Status of the very high temperature reactor system. Prog. Nucl. Energy 77, 266–281. doi: 10.1016/j.pnucene.2014.01.013
Garibov, A. A., Velibekova, G. Z., and Agayev, T. N. (1987). Heterogeneous radiolysis of CO2 in the presence of zeolites. Int. J. Radiat. Appl. Instrum. Part C Radiat. Phys. Chem. 29, 71–73. doi: 10.1016/1359-0197(87)90064-6
Getoff, N. (1965). Carboxylation of formic acid in aqueous solutions under the influence of uv-light. Photochem. Photobiol. 4, 433–438. doi: 10.1111/j.1751-1097.1965.tb09756.x
Getoff, N. (1999). Radiation chemistry and the environment. Radiat. Phys. Chem. 54, 377–384. doi: 10.1016/S0969-806X(98)00266-7
Getoff, N. (2006). Control of greenhouse gases emission by radiation-induced formation of useful products. Utilization of CO2. Radiat. Phys. Chem. 75, 514–523. doi: 10.1016/j.radphyschem.2005.09.014
Getoff, N., and Schenck, G. O. (1967). On the formation of amino acids by gamma-ray-induced carboxylation of amines in aqueous solutions. Radiat. Res. 31, 486–505. doi: 10.2307/3572367
Getoff, N., Solar, S., and Quint, R. M. (1997). One-electron oxidation of mitomycin c and its corresponding peroxyl radicals. A steady-state and pulse radiolysis study. Radiat. Phys. Chem. 50, 575–583. doi: 10.1016/S0969-806X(97)00099-6
Getoff, N., Solar, S., Richter, U. B., and Haenel, M. W. (2003). Pulse radiolysis of pyrene in aprotic polar organic solvents: simultaneous formation of pyrene radical cations and radical anions. Radiat. Phys. Chem. 66, 207–214. doi: 10.1016/S0969-806X(02)00392-4
Global Carbon Project (2020). Global Carbon Atlas: CO2 Emissions. Available online at: http://www.globalcarbonatlas.org/en/CO2-emissions (accessed February 2018).
Gomberg, H. J., and Gordus, A. A. (1982). Hydrogen for synthetic fuels via nuclear energy. J. Fus. Energy 2, 319–339. doi: 10.1007/BF01063684
Götz, M., Lefebvre, J., Mörs, F., Mcdaniel Koch, A., Graf, F., Bajohr, S., et al. (2016). Renewable power-to-gas: a technological and economic review. Renew. Energy 85, 1371–1390. doi: 10.1016/j.renene.2015.07.066
Graf, F., Götz, M., and Bajohr, S. (2011). Injection of biogas, SnG and hydrogen into the gas grid. GWF Gas Erdgas 2, 30–40.
Grills, D. C., Farrington, J. A., Layne, B. H., Lymar, S. V., Mello, B. A., Preses, J. M., et al. (2014). Mechanism of the formation of a mn-based CO2 reduction catalyst revealed by pulse radiolysis with time-resolved infrared detection. J. Am. Chem. Soc. 136, 5563–5566. doi: 10.1021/ja501051s
Grills, D. C., Farrington, J. A., Layne, B. H., Preses, J. M., Bernstein, H. J., and Wishart, J. F. (2015). Development of nanosecond time-resolved infrared detection at the leaf pulse radiolysis facility (#044102). Rev. Sci. Instrum. 86:044102. doi: 10.1063/1.4918728
Grills, D. C., and Lymar, S. V. (2018). Radiolytic formation of the carbon dioxide radical anion in acetonitrile revealed by transient ir spectroscopy. Phys. Chem. Chem. Phys. 20, 10011–10017. doi: 10.1039/C8CP00977E
Gütlbauer, F., and Getoff, N. (1965). Radiation chemical carboxylation of hydroxycompounds. Int. J. Appl. Radiat. Isotopes 16, 673–680. doi: 10.1016/0020-708X(65)90067-0
Harteck, P., and Dondes, S. (1955). Decomposition of carbon dioxide by ionizing radiation. Part I. J. Chem. Phys. 23, 902–908. doi: 10.1063/1.1742145
Harteck, P., and Dondes, S. (1957). Decomposition of carbon dioxide by ionizing radiation. Part II. J. Chem. Phys. 26, 1734–1737. doi: 10.1063/1.1743612
Harth, R. E., and Boltendahl, U. (1981). The chemical heat pipe eva and adam. Interdiscipl. Sci. Rev. 6, 221–228. doi: 10.1179/isr.1981.6.3.221
Hashimoto, K., Kumagai, N., Izumiya, K., Takano, H., and Kato, Z. (2014). The production of renewable energy in the form of methane using electrolytic hydrogen generation. Energy Sust. Soc. 4, 1–9. doi: 10.1186/s13705-014-0017-5
Hashimoto, K., Yamasaki, M., Fujimura, K., Matsui, T., Izumiya, K., Komori, M., et al. (1999). Global CO2 recycling - novel materials and prospect for prevention of global warming and abundant energy supply. Mater. Sci. Eng. A 267, 200–206. doi: 10.1016/S0921-5093(99)00092-1
Hirschfelder, J. O., and Taylor, H. S. (1938). The alpha-particle reactions in carbon monoxide, oxygen and carbon dioxide systems. J. Chem. Phys. 6, 783–790. doi: 10.1063/1.1750171
Hoekman, S. K., Broch, A., Robbins, C., and Purcell, R. (2010). CO2 recycling by reaction with renewably-generated hydrogen. Int. J. Greenh. Gas Control 4, 44–50. doi: 10.1016/j.ijggc.2009.09.012
Höhlein, B., Menzer, R., and Range, J. (1981). High temperature methanation in the long-distance nuclear energy transport system. Appl. Catal. 1, 125–139. doi: 10.1016/0166-9834(81)80001-2
Honig, R. E., and Sheppard, C. W. (1946). An experimental comparison of the chemical effects of deuterons and of alpha particles on methane and n-butane. J. Phys. Chem. 50, 119–143. doi: 10.1021/j150446a004
Hosokawa, Y., Kajiya, S., Ohshima, A., Ishida, N., Washio, M., and Usuki, A. (2019). CO2 conversion by high-dose rate electron beam irradiation: one-step, metal-free and simultaneous production of H2, CO, CH4, C2H6 and organic acids from an acid-decomposed CaCO3/additive etoh mixture. Green Chem. 21, 3091–3098. doi: 10.1039/C9GC00525K
Hunt, J., Ferrari, A., Lita, A., Crosswhite, M., Ashley, B., and Stiegman, A. E. (2013). Microwave-specific enhancement of the carbon–carbon dioxide (boudouard) reaction. J. Phys. Chem. C 117, 26871–26880. doi: 10.1021/jp4076965
Ikezoe, Y., and Sato, S. (1976). Radiation chemical reactions in carbon dioxide-propane system formation of carbon monoxide by fission fragments. J. Nucl. Sci. Technol. 13, 503–507. doi: 10.1080/18811248.1976.9734064
Ikezoe, Y., Sato, S., Shimizu, S., and Matsuoka, S. (1981). Effect of water on the radiolysis of carbon dioxide. Radiat. Phys. Chem. 17, 69–70. doi: 10.1016/0146-5724(81)90002-9
Ikezoe, Y., Sato, S., Shimizu, S., and Nakajima, H. (1982). Potential of carbon dioxide radiolysis for hydrogen production. Int. J. Hydrogen Energy 7, 539–543. doi: 10.1016/0360-3199(82)90034-9
Kalashnikov, N. A., Kalinichenko, B. S., and Shvetsov, I. K. (1992a). Influence of reagent phase state, form and dose rate irradiation on efficiency of radiolytic decomposition of water and carbon dioxide. Atomnaya Energiya 72, 47–53. doi: 10.1007/BF01121317
Kalashnikov, N. A., Kalinichenko, B. S., and Shvetsov, I. K. (1992b). Influence of temperature pressure and inorganic gases admixtures on efficiency of water vapour and carbon dioxide radiolysis. Atomnaya Energiya 72, 54–59. doi: 10.1007/BF01121318
Kalinichenko, B. S., Kalashnikov, N. A., Kulazhko, V. G., and Shvetsov, I. K. (1989). Method of Producing Carbon Monoxide. Patent No. SU1490074. National Research Centre “Kurchatov Institute.”
Kalinichenko, B. S., Kalashnikov, N. A., Kulazhko, V. G., and Shvetsov, I. K. (1993). Method of Producing Hydrogen by Radiolysis of Water Vapor. Patent No. RU1824375. National Research Centre “Kurchatov Institute.”
Kalmykova, Y., Sadagopan, M., and Rosado, L. (2018). Circular economy - from review of theories and practices to development of implementation tools. Resour. Conserv. Recycl. 135, 190–201. doi: 10.1016/j.resconrec.2017.10.034
Kaltschmitt, M., Hartmann, H., and Hofbauer, H. (2009). Energie Aus Biomasse: Grundlagen, Techniken und Verfahren. Springer.
Krapfenbauer, K. F., and Getoff, N. (1997). Radiation-and photo-induced formation of salicylic acid from phenol and CO2 in aqueous solution. World Resour. Rev. 9, 421–433.
Kuimova, M. K., Alsindi, W. Z., Dyer, J., Grills, D. C., Jina, O. S., Matousek, P., et al. (2003). Using picosecond and nanosecond time-resolved infrared spectroscopy for the investigation of excited states and reaction intermediates of inorganic systems. J. Chem. Soc. Dalton Trans. 3996–4006. doi: 10.1039/b306303h
Kummler, R., Leffert, C., Im, K., Piccirelli, R., Kevan, L., and Willis, C. (1977). A numerical model of carbon dioxide radiolysis. J. Phys. Chem. 81, 2451–2463. doi: 10.1021/j100540a023
Lewis, J. G., Martin, A. J., Meinke, W. W., Ricker, C. W., and Teital, R. J. (1979). Radiolytic-Chemical Method for Production of Gases. Patent No. US4175016. Texas Gas Transmission Corp.
Lind, S. C., and Bardwell, D. C. (1925). The chemical action of gaseous ions produced by alpha particles. VI. Reactions of the oxides of carbon. J. Am. Chem. Soc. 47, 2675–2697. doi: 10.1021/ja01688a009
Lind, S. C., and Bardwell, D. C. (1926). The chemical action of gaseous ions produced by alpha particles. IX. Saturated hydrocarbons. J. Am. Chem. Soc. 48, 2335–2351. doi: 10.1021/ja01420a012
Lohan, C., and Kylä-Harakka-Ruonala, T. (2018). Eesc opinion: Monitoring Framework for the Circular Economy. Report No. 2018/C 367/19. The European Economic and Social Committee, Brussels.
Mccormick, K., and Kautto, N. (2013). The bioeconomy in europe: an overview. Sustainability 5, 2589–2608. doi: 10.3390/su5062589
Mejía, C., Bender, M., Severin, D., Trautmann, C., Boduch, P., Bordalo, V., et al. (2015). Radiolysis and sputtering of carbon dioxide ice induced by swift Ti, Ni, and Xe ions. Nucl. Instrum. Methods Phys. Res. B Beam Interact. Mater. Atoms 365, 477–481. doi: 10.1016/j.nimb.2015.09.039
Meyer, W., La-Belle, D. W., and Rassoul, S. A. (1973). The effects of co-60 gamma radiation on the thermal decomposition of carbonate compounds. Int. J. Appl. Radiat. Isotopes 24, 315–318. doi: 10.1016/0020-708X(73)90071-9
Milko, J., Allen, T., and Fitzpatrick, R. (2018). Keeping Up With the Advanced Nuclear Industry. Available online at: https://www.thirdway.org/graphic/keeping-up-with-the-advanced-nuclear-industry (accessed December 2018).
Moraga, G., Huysveld, S., Mathieux, F., Blengini, G. A., Alaerts, L., Van Acker, K., et al. (2019). Circular economy indicators: what do they measure? Resour Conserv. Recycl. 146, 452–461. doi: 10.1016/j.resconrec.2019.03.045
Mustafaev, I. I., Gurbanov, M. A., and Hajiev, H. M. (1988). Gasification of different types of carbon in steam and carbon dioxide in the presence of γ-radiation. Carbon 26, 125–128. doi: 10.1016/0008-6223(88)90028-0
Nagai, T., and Suzuki, N. (1978). The radiation-induced degradation of lignin in aqueous solutions. Int. J. Appl. Radiat. Isotopes 29, 255–259. doi: 10.1016/0020-708X(78)90051-0
Ngo, K. T., Mckinnon, M., Mahanti, B., Narayanan, R., Grills, D. C., Ertem, M. Z., et al. (2017). Turning on the protonation-first pathway for electrocatalytic CO2 reduction by manganese bipyridyl tricarbonyl complexes. J. Am. Chem. Soc. 139, 2604–2618. doi: 10.1021/jacs.6b08776
Olabi, A. G. (2019). Circular economy and renewable energy. Energy 181, 450–454. doi: 10.1016/j.energy.2019.05.196
Oulianov, D. A., Crowell, R. A., Gosztola, D. J., Shkrob, I. A., Korovyanko, O. J., and Rey-De-Castro, R. C. (2007). Ultrafast pulse radiolysis using a terawatt laser wakefield accelerator. J. Appl. Phys. 101:053102. doi: 10.1063/1.2696204
Perkins, C., Jovanovic, Z., and Laska, T. E. (2014). Various Methods and Apparatuses for a Radiant-Heat Driven Chemical Reactor. Patent No. US8814961 (also published as US20120241677). Sundrop Fuels Inc.
Pilling, S., Seperuelo Duarte, E., Domaracka, A., Rothard, H., Boduch, P., and Da Silveira, E. F. (2010). Radiolysis of H2O:CO2 ices by heavy energetic cosmic ray analogs. Astron. Astrophys. 523:A77. doi: 10.1051/0004-6361/201015123
Ponomarev, A. V., and Ershov, B. G. (2014). Radiation-induced high-temperature conversion of cellulose. Molecules 19, 16877–16908. doi: 10.3390/molecules191016877
Ryazantsev, S. V., and Feldman, V. I. (2015). Matrix-isolation studies on the radiation-induced chemistry in H2O/CO2 systems: reactions of oxygen atoms and formation of HOCO radical. J. Phys. Chem. A 119, 2578–2586. doi: 10.1021/jp509313n
Sadło, J., Pajchel, L., Michalik, J., and Kolodziejski, W. (2012). Epr studies of radicals generated by γ-radiation in nanocrystalline hydroxyapatites prepared by dry milling. J. Mol. Struct. 1022, 61–67. doi: 10.1016/j.molstruc.2012.04.062
Schmeda-Lopez, D., Mcconnaughy, T. B., and Mcfarland, E. W. (2018). Radiation enhanced chemical production: improving the value proposition of nuclear power. Energy 162, 491–504. doi: 10.1016/j.energy.2018.07.208
Shkrob, I. A. (2002). Ionic species in pulse radiolysis of supercritical carbon dioxide. 2. Ab initio studies on the structure and optical properties of (CO2)n+, (CO2)2−−, and CO3−− ions. J. Phys. Chem. A 106, 11871–11881. doi: 10.1021/jp0214918
Shkrob, I. A., Dimitrijevic, N. M., Marin, T. W., He, H., and Zapol, P. (2012b). Heteroatom-transfer coupled photoreduction and carbon dioxide fixation on metal oxides. J. Phys. Chem. C 116, 9461–9471. doi: 10.1021/jp300123z
Shkrob, I. A., Marin, T. W., He, H., and Zapol, P. (2012a). Photoredox reactions and the catalytic cycle for carbon dioxide fixation and methanogenesis on metal oxides. J. Phys. Chem. C 116, 9450–9460. doi: 10.1021/jp300122v
Shkrob, I. A., and Sauer, M. C. Jr. (2001). Solvent anions in supercritical carbon dioxide: formation of complexes with polar solutes. J. Phys. Chem. B 105, 7027–7032. doi: 10.1021/jp011139e
Shkrob, I. A., Sauer, M. C. Jr., Jonah, C. D., and Takahashi, K. (2002). Ionic and neutral species in pulse radiolysis of supercritical CO2. 1. Transient absorption spectroscopy, electric field effect, and charge dynamics. J. Phys. Chem. A 106, 11855–11870. doi: 10.1021/jp021494k
Shkrob, I. A., Sauer, M. C. Jr., and Trifunac, A. D. (1996a). High-mobility ions in the viscous hydrocarbon squalane. J. Phys. Chem. 100, 5993–6002. doi: 10.1021/jp9532374
Shkrob, I. A., Sauer, M. C. Jr., and Trifunac, A. D. (1996b). High-mobility ions in cyclohexane. A transient absorption study. J. Phys. Chem. 100, 7237–7245. doi: 10.1021/jp953255l
Shkrob, I. A., Sauer, M. C. Jr., and Trifunac, A. D. (2001). Radiation chemistry of organic liquids: saturated hydrocarbons. Stud. Phys. Theor. Chem. 87, 175–221. doi: 10.1016/S0167-6881(01)80011-2
Shkrob, I. A., Sauer, M. C. Jr., Yan, J., and Trifunac, A. D. (1996c). Transient absorption spectroscopy of high-mobility ions in decalin. J. Phys. Chem. 100, 6876–6878. doi: 10.1021/jp960387l
Shkrob, I. A., and Schlueter, J. A. (2006). Can a single molecule trap the electron? Chem. Phys. Lett. 431, 364–369. doi: 10.1016/j.cplett.2006.09.106
Shkrob, I. A., and Trifunac, A. D. (1995a). Pulse radiolysis of alkanes: a time-resolved epr study-part I. Alkyl radicals. Radiat. Phys. Chem. 46, 83–96. doi: 10.1016/0969-806X(94)00118-4
Shkrob, I. A., and Trifunac, A. D. (1995b). Pulse radiolysis of alkanes: a time-resolved EPR study-part II. Phenolic additives. Radiat. Phys. Chem. 46, 97–104. doi: 10.1016/0969-806X(94)00119-5
Shkrob, I. A., and Trifunac, A. D. (1995c). Free d atoms in pulse radiolysis of methane in cryogenic fluids. J. Phys. Chem. 99, 11122–11130. doi: 10.1021/j100028a012
Shkrob, I. A., and Trifunac, A. D. (1996). Electron transfer reactions and mobile holes in radiolysis of squalane. Time-resolved fdmr study. J. Phys. Chem. 100, 14681–14687. doi: 10.1021/jp9532475
Simmons, W. W., Perkins, C., Jovanovic, Z., Hilton, C., Popp, P., Schramm, B. J., et al. (2015). Various Methods and Apparatuses for Ultra-High Heat Flux Chemical Reactor. Patent No. US9011560 (also published as US2012145965, CN102985195, WO2011155962, CA2799827, AU2010355257). Sundrop Fuels Inc.
Spadaro, G., De Gregorio, R., Galia, A., Valenza, A., and Filardo, G. (2000). Gamma radiation induced maleation of polypropylene using supercritical CO2: preliminary results. Polymer 41, 3491–3494. doi: 10.1016/S0032-3861(99)00588-1
Sterner, M. (2009). Bioenergy and renewable power methane in integrated 100% renewable energy systems: limiting global warming by transforming energy systems, Vol. 14 (Doktor der Ingenieurwissenschaften Thesis), Faculty of Electrical Engineering and Computer Science of the University of Kassel, Kassel University Press GmbH, Kassel, 230. Available online at: http://www.uni-kassel.de/upress/online/frei/978-3-89958-798-2.volltext.frei.pdf
Sullivan, K. K., Boamah, M. D., Shulenberger, K. E., Chapman, S., Atkinson, K. E., Boyer, M. C., et al. (2016). Low-energy (<20 ev) and high-energy (1000 ev) electron-induced methanol radiolysis of astrochemical interest. Mon. Not. Royal Astron. Soc. 460, 664–672. doi: 10.1093/mnras/stw593
Trifunac, A. D., Sauer, M. C. Jr., Shkrob, I. A., and Werst, D. W. (1997). Radical cations in radiation chemistry of liquid hydrocarbons. Acta Chem. Scand. 51, 158–166. doi: 10.3891/acta.chem.scand.51-0158
US Department of Energy (2002). Nuclear Energy Research Advisory Committe and Generation IV International Forum A Technology Roadmap for Generation IV Nuclear Energy Systems. Report No. GIF-002-00. USA Department of Energy.
Velasco, F. G., Luzardo, F. H. M., Guzman, F., Rodriguez, O., Coto Hernandez, I., Barroso, S., et al. (2014). Gamma radiation effects on molecular characteristic of vegetable tannins. J. Radioanal. Nucl. Chem. 299, 1787–1792. doi: 10.1007/s10967-014-2921-8
Wang, W. H., Himeda, Y., Muckerman, J. T., Manbeck, G. F., and Fujita, E. (2015). CO2 hydrogenation to formate and methanol as an alternative to photo- and electrochemical CO2 reduction. Chem. Rev. 115, 12936–12973. doi: 10.1021/acs.chemrev.5b00197
Watanabe, D., Yoshida, T., Allen, C., and Tanabe, T. (2007). Enhancement of gamma-ray radiolysis of carbon dioxide with the assistance of solid materials. J. Radioanal. Nucl. Chem. 272, 461–465. doi: 10.1007/s10967-007-0604-4
Wickham, A. J., Best, J. V., and Wood, C. J. (1977). Recent advances in the theories of carbon dioxide radiolysis and radiolytic graphite corrosion. Radiat. Phys. Chem. 10, 107–117. doi: 10.1016/0146-5724(77)90016-4
Wigmans, T., Van Dam, F., and Moulijn, J. A. (1981). Comparison of a block-flow reactor and thermogravimetric analysis in the steam gasification of different types of carbon. Carbon 19, 309–320. doi: 10.1016/0008-6223(81)90078-6
Willis, C., and Boyd, A. W. (1976). Excitation in the radiation chemistry of inorganic gases. Int. J. Radiat. Phys. Chem. 8, 71–111. doi: 10.1016/0020-7055(76)90061-9
Keywords: CO2 radiolysis, CO2 capture and conversion, irradiation, free radicals, CO2 utilization
Citation: Ramirez-Corredores MM, Gadikota G, Huang EE and Gaffney AM (2020) Radiation-Induced Chemistry of Carbon Dioxide: A Pathway to Close the Carbon Loop for a Circular Economy. Front. Energy Res. 8:108. doi: 10.3389/fenrg.2020.00108
Received: 16 March 2020; Accepted: 11 May 2020;
Published: 09 June 2020.
Edited by:
Youngjune Jason Park, Gwangju Institute of Science and Technology, South KoreaReviewed by:
Hannu-Petteri Mattila, Independent Researcher, Parainen, FinlandCopyright © 2020 Ramirez-Corredores, Gadikota, Huang and Gaffney. This is an open-access article distributed under the terms of the Creative Commons Attribution License (CC BY). The use, distribution or reproduction in other forums is permitted, provided the original author(s) and the copyright owner(s) are credited and that the original publication in this journal is cited, in accordance with accepted academic practice. No use, distribution or reproduction is permitted which does not comply with these terms.
*Correspondence: Maria M. Ramirez-Corredores, bWFyaWFtYWdkYWxlbmEucmFtaXJlemNvcnJlZG9yZXNAaW5sLmdvdg==; Greeshma Gadikota, Z2c0NjRAY29ybmVsbC5lZHU=
Disclaimer: All claims expressed in this article are solely those of the authors and do not necessarily represent those of their affiliated organizations, or those of the publisher, the editors and the reviewers. Any product that may be evaluated in this article or claim that may be made by its manufacturer is not guaranteed or endorsed by the publisher.
Research integrity at Frontiers
Learn more about the work of our research integrity team to safeguard the quality of each article we publish.