- 1Department of Chemical Engineering, College of Engineering, Qatar University, Doha, Qatar
- 2Separation and Conversion Technology, Flemish Institute for Technological Research (VITO), Mol, Belgium
Changes in the environment due to multiple factors, such as combustion of fossil fuels, heating, transportation, deforestation, etc., have led to more greenhouse gases in the atmosphere, which eventually led to a rise in global temperatures. Carbon dioxide (CO2) is the major factor for the rapid rise in global temperature. One of the most encouraging technological advances to address global warming is to transform CO2 into value-added commodities that offer a win–win strategy. In this regard, intensive research has been pursued around the world for development of feasible systems in product recovery or product synthesis from CO2-rich industrial emissions. We envision that the biological CO2 reduction or conversion process can be beneficial for developing carbon-neutral technologies. The integration of CO2-emitting industrial technologies with CO2-converting biological systems can be helpful in achieving sustainable value-added products with no or minimal loss of energy and materials that are assuring for improved economics. The CO2-converting bioprocesses can be directly integrated with the processes emitting a high amount of CO2. This symbiotic integration can make the whole process carbon neutral. Herein, this review highlights an insight on research activities of biological CO2 mitigation using photo catalysts (algae and photo bacteria), an anaerobic biocatalyst (bacteria), gas fermentation, and an enzymatic catalyst. Perspectives and challenges of these technologies are discussed.
Introduction
Expansion of industries and world population are leading to an increase in the release of greenhouse gases, resulting in accelerated global warming (Kumar et al., 2016; Effendi and Ng, 2019; Youn et al., 2019). The higher levels of carbon dioxide (CO2) emissions into the environment have become a major contributor to global warming (Figure 1A). Both reduction in CO2 emission and capture of CO2 are critical to reduce global warming (Benhelal et al., 2013). In this regard, carbon capture, sequestration, and utilization have proven to be efficient options for decreasing the atmospheric CO2, which can be regarded as a waste-to-energy process (Liu et al., 2016). This energy process can be either fuel generation or chemical and material production. Several industrial technologies have been developed and are operational, such as the alkaline adsorption process (CO2 capture), mineralization process, landfill process (CO2 sequestration), urea synthesis, methanol production, phenol carboxylation, epoxide carboxylation, and pyrrole carboxylation (CO2 utilization) (Shi et al., 2015; Liu et al., 2016). Global research on biological CO2 conversion for renewable and sustainable energy generation is increasing at a rapid pace in diversified research disciplines (Figure 1B). In addition, many bench laboratory-scale or pilot-scale technologies are also being developed to increase the performance of CO2 capture, sequestration, and utilization toward value addition (Shi et al., 2015).
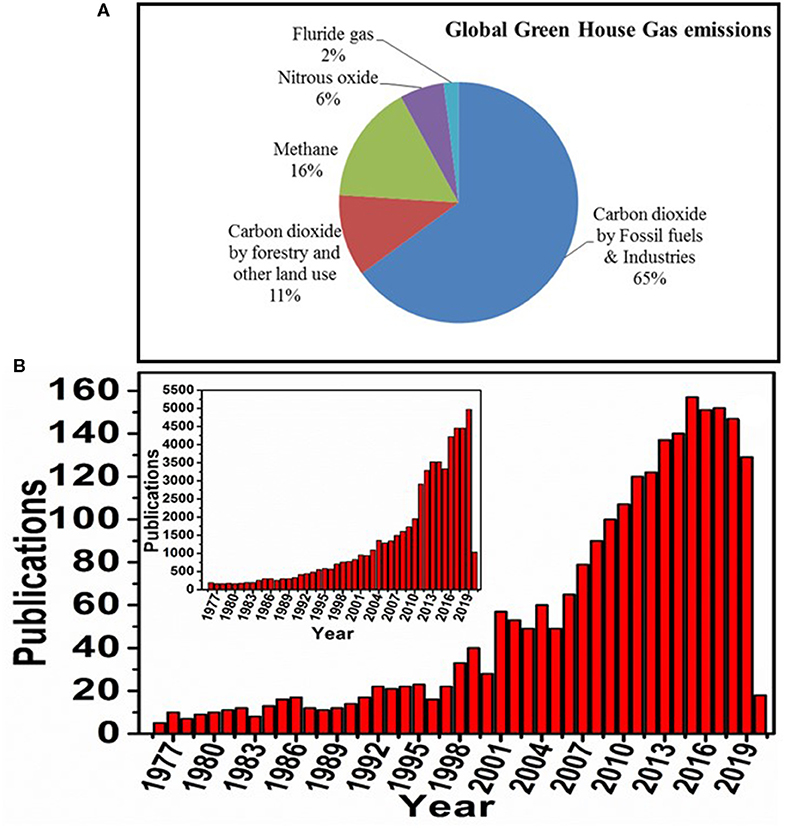
Figure 1. (A) Graphical representation of global greenhouse gas emissions (source: https://tinyurl.com/EPA-GHG-Data), (B) number of articles published on biological conversion of CO2 to value-added production (source: Scopus; key words: Biological carbon dioxide reduction or Biological carbon dioxide conversion, as on date: 21/04/2020). Inset figure exhibits the number of published articles on carbon dioxide reduction or carbon dioxide conversion irrespective of field.
Capture and transformation of CO2 to value-added products, such as biofuel, are of special interest, and several countries have been developing policies by providing subsidies and legislative incentives. For instance, the Renewable Energy Directive (RED) under the European Union (EU) is moving toward a vision of 2020, which is targeting the generation of 10% of the energy required for the transportation sector that should be replaced with renewable biofuels (Chang et al., 2016; Sadhukhan et al., 2016). Likewise, in the United States, the Renewable Fuel Standard is targeted at increasing biofuel generation by 36% with a vision of 2022 (Rana et al., 2011). About 58% of biofuel generation is mainly from second- (e.g., organic waste, plant waste) and third-generation (e.g., algae biomass) biofuels.
Biological, chemical, photo-chemical, electrochemical, and enzymatic technologies have been developed for fixing and conversion of CO2 to renewable energy generation (Benemann, 1997; Bond et al., 2001). However, a majority of the processes are linked with high energy input, which is making the process economically unfavorable. Among these methods, a biological system for CO2 utilization using bacteria as a catalyst, which is of low cost and self-regenerative, is of particular interest and importance (Benemann, 1993; Jiang et al., 2019). Hence, the potential of numerous biological CO2 utilization methods to decrease the atmospheric CO2 should be investigated further with an energy-neutral and biorefinery approach (Venkata Mohan et al., 2016; Hou et al., 2019). The petroleum refinery approach has been well-known for a long time for energy generation. However, the use of a CO2 biorefinery approach is exciting and offers a sustainable and eco-friendly process (Packer, 2009; Venkata Mohan et al., 2016). Nonetheless, this area of biological CO2 utilization research is obstructed with several questions regarding its efficiency, establishment cost, and fulfilling of current and future energy demands. Despite these criticisms, the research on utility of CO2 should be increased toward a carbon-neutral footprint and energy neutrality. Therefore, the major objective of this review is to highlight the biological CO2 utilization methods that can be implemented to generate bio-based products and elaborate on the biology of sequestration methods of different systems/microbes.
Biological Mitigation of CO2
Photosynthetic CO2 Reduction
Recently, several researchers have concentrated on sequestration of CO2 using light energy to generate value-added products, including fuels, chemicals, and other materials. Most of the photosynthetic biological systems include oxygenic or oxygenic photosynthesis routes (Hunt et al., 2010). Below, we discuss the CO2 reduction using algae and photosynthetic bacteria (Figure 2). Algae cultivation for CO2 conversion provides numerous advantages and liabilities that are documented in Table 1.
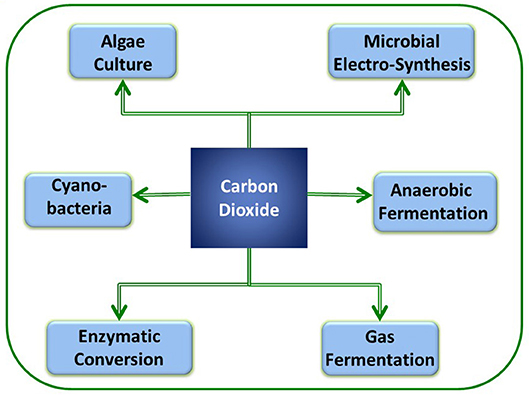
Figure 2. Various biological processes that have potential for CO2 sequestration along with sustainable bioenergy/chemical production.
CO2 Sequestration/Assimilation Using Algae
In the past decade, several studies have proven that CO2 mitigation with algae is found to be a sustainable process with simultaneous generation of high calorific products, such as biodiesel, pigments, fatty acids, etc. (Chisti, 2007; Demirbas, 2010) (Table 2). The potential utilization of algae is mainly attributed to its wide distribution, high biomass production, capability to adjust in adverse conditions, swift carbon uptake and utilization, and capability to generate value-added products (Figure 3). Both macro and micro algae have the capability to metabolize inorganic carbon by a photoautotrophic mechanism using carbonic anhydrase enzyme (CAE) (Clarens et al., 2010). The generated NADH2 from the electron transport chain combines with the RuBisCo (Ribulose-1,5-bisphosphate carboxylase/oxygenase, provided by CAE) and helps in carbohydrate generation from CO2 and provides the reducing power in the Calvin cycle for glucose synthesis (Shi et al., 2015).
Selection of suitable microalgae for CO2 fixation
It is known that a higher concentration (generally greater than 5%) of CO2 adversely impacts the growth and production rate in most microalgae (Clarens et al., 2010). Therefore, numerous research studies are being carried out to isolate a suitable algal strain for direct and efficient conversion of industrial CO2 into products such as agar and alginate, etc. (Table 3). The criteria for selection is not only tolerance of CO2, temperature, and toxic compounds, but also for attaining high growth rates and maximum cell densities (Kratz and Myers, 1955; Allen and Stanier, 1968).
Freshwater microalgae Chlorella HA-1 exhibited a maximum growth rate when it was evaluated at 5 and 10% of CO2 (Watanabe et al., 1992). However, the growth was inhibited with further increases in CO2 concentration up to 20%. This study proposed that Chlorella HA-1 can be used to treat industrial flue gas with a maximum CO2 concentration of 10% (Watanabe et al., 1992). This strain exhibited a variation in response with a change in temperature and pH. Chlorococcum littorale culture isolated from a saline water pond was able to show an almost similar growth rate from 5 to 60% of CO2, which is a much higher CO2 concentration than in flue gas (20%). When the CO2 concentration was boosted to 70%, a decrease in growth rate was identified. As this strain exhibits high growth at high CO2 concentrations, it can be readily applied for industrial flues gases (Kodama et al., 1993). There were also several other strains of micro algae that have exhibited a high growth rate in adverse conditions, such as extreme pH and temperature (Kumar et al., 2011). The most industrially applicable algal product is lipids, which can be used as a source for biofuel productions (e.g., biodiesel) (Silva et al., 2014). Therefore, the assortment of strains for generation of high lipid generation and augmenting the culture conditions, such as light, temperature, and pH, are vital to enhance the productivity (Csavina et al., 2011; Georgianna and Mayfield, 2012). The unoptimized pH, during algae growth, can affect the distribution of carbon species and carbon availability. Also, at extreme pH, it can directly affect the metabolic activity of algae.
Flue gases from fossil fuel power plants and industries discharge elevated strengths of CO2, varying from 10 to 20%, along with a biologically significant amount of NOx and SOx. The dosing of flue gases to algae ponds has shown to increase biomass yields by 3-fold and high energy generation (Hanagata et al., 1992; Maeda et al., 1995). Fortunately, the benefits in injecting the flue gas to algae growth can be more helpful than the growth impacts attributed in the photorespiration process at high CO2 concentrations. Recent studies have shown that direct submission of flue gases into algal ponds exhibited a 30% increase in biomass yields over using the equivalent concentration of CO2 (Tsuzuki et al., 1990; Singh and Singh, 2014). This might be due to the existence of additional nutrients (oxides of nitrogen and sulfur) present in flue gas. This competence of CO2 encapsulation by algae can vary according to the algae physiology, pond chemistry, and temperature. About 80 to 99% of CO2 capture can be obtained under optimized conditions with gas residence times as short as 2 s (Keffer and Kleinheinz, 2002; Lee and Lee, 2003). For a 200 MW/h power plant, it has been noted that 3,600 acres of algae pond would be required to capture 80% of the plant's CO2 emissions during the daylight hours, assuming a productivity rate of 20 g dry biomass/m2/d. Therefore, locating the algae ponds next to CO2 point suppliers provides numerous potential low-cost and energy-saving advantages (Sayre, 2010) with a minimal carbon footprint.
Challenges in using algae for CO2 capturing/sequestration
On an industrial scale, algae are typically growing in photo bioreactors, open ponds, or raceway ponds (Wang, 2014). The use of open ponds can be simpler in architecture and operation. However, these are limited with a large surface area necessity, elevated cultivation cost, high chance of contamination, and low productivity. Photo bioreactors with closed systems have been proposed to overcome the above limitations (Li et al., 2014). A detailed review study published by Kumar et al. (2011) provided great aspects in the design of photo bioreactors and challenges noticed in industrial algae growth. Flue gas compositions are made up of different types of gases along with CO2, which is viable for the growth of few algae. However, high temperatures, fluctuating flue gas composition, and the presence of sulfur and nitrogen can be toxic to some algal strains. Therefore, the selection of suitable algal processes and strains is needed. One of the other key aspects that play a crucial role in algae development is light intensity (Sorokin and Krauss, 1958). Insufficient light can lead to poor growth, and an increase in light intensity can also show adverse effects on the growth, known as photo inhibition (Xu et al., 2016). The mixing of cell cultures requires an extensive amount of energy input and constitutes a major portion of operational costs (Cloot, 1994). Stirring of cultures is important to reduce the mass transfer limitations occurring in photo bioreactors, which also favorably helps to eliminate oxygen accumulation. Several other advancements have been made to overcome the challenges that are discussed.
In general, the CO2 uptake/capture with algae is calculated based on carbon concentration in influent and effluent (Anbalagan et al., 2017). However, it is difficult to consider that whole CO2 uptake is carried out by algae and is utilized for its growth. But the carbon content in biomass can deliver a more precise value on the quantity of CO2 consumption by algae. This scenario can only be useful if the provided culture media does not have an additional organic carbon source, other than inorganic carbon, such as bicarbonate or CO2. Based on the following, CO2 capture by algae can be calculated as
where RCO2 is the fixation rate of CO2 concentration (g.l−1 d−1), P is the production of biomass concentration per day (g.l−1 d−1), CCO2 is increase in carbon content of algae biomass from CO2 utilization, MCO2 is the molecular weight of CO2, and MCis the molecular mass of carbon (de Morais and Costa, 2007a).
Tang et al. (2011) analyzed the carbon content and calculated the rate of CO2 biofixation for different algae species that were grown in modified Blue-Green 11 (BG11) medium. In this study, they calculated the carbon capture rate by varying the CO2 concentration from 0.03 to 50%. The carbon content in biomass was found to be around 50%, whereas the CO2 fixation was in the range of 0.105 to 0.288 g. l−1 d−1.
Commercialization of microalgal technologies
To commercialize products from microalgal technologies, a designed biorefinery in a circular loop was found to be a sustainable option. A self-sustainable biorefinery (SSB) model of microalgae maximizes resource utilization along with zero waste discharge (Venkata Mohan et al., 2020). Utilization of defatted or de-oiled microalgal biomass (DMB) for other processes also helps to develop sustainable biorefineries. DMB can be used as a substrate for other biofuel generation, such as biomethane, biohydrogen, and bioethanol (Maurya et al., 2016). Thermochemical liquefaction, pyrolysis, and gasification are non-biological processes in which DMB can be used as substrate to produce fuels and chemicals (Sarkar et al., 2015; Maurya et al., 2016). Compared to conventional methods, the microalgae industry is concerned about the two fundamental hurdles, which can be identified as low productivity and high costs (Maurya et al., 2016). Subjecting residual biomass or DMB (post lipid extraction) in various fields improves the economic feasibility and commercialization of algal bioprocesses (Singh et al., 2011; Rashid et al., 2013). The biomass of microalgae can be used as inoculum for new processes that reduce the maintenance of parent cultures. This helps in sustainable operation of microalgal bioreactors (Sarkar et al., 2015). Apart from utilizing DMB in fuel and chemical production, it can also be used as feed supplement and biofertilizer. DMB is rich in protein and essential amino acids that could be utilized as livestock and aquaculture feeds or as a dietary supplement. DMB has also been tested to replace fishmeal from wild fish caught in an ocean environment. Thus, it can mitigate ocean resource depletion (Zhang and Kendall, 2019). In wastewater treatment, DMB can be used as an active biosorbent to remove dyes/color and heavy metals (Maurya et al., 2016). During the extraction of lipids from algal biomass, the biomass used to rupture, which has a higher surface area than the raw biomass. This helps for the efficient functioning of DMB as an active biosorbent (Dong et al., 2016).
Bioenergy production with carbon capture and storage (CCS) plays a crucial role in CO2 mitigation. Combination of bioenergy production and CCS help to keep CO2 in geological reservoirs. Algae have high potential to store CO2 as biomass, which is due to high photosynthetic efficiencies and high biomass yields (Moreira and Pires, 2016). Microalgal cultivation was designated as a negative emission technology (NET), which can reduce the impacts of ocean acidification and anthropogenic climate change (Moreira and Pires, 2016). Since the scope of microalgae is versatile and possesses the expansive possibility of microalgae cultivation with a self-sustainability approach, it is designated as a blue-bioeconomy.
Autotrophic lipid/biodiesel generation using algae
World energy is mainly dependent on electricity and fuel segments. Still, both segments are needed to implement substantial carbon-neutral techniques to reach international legislated standards in regard to the CO2 emissions target (International Energy Agency, 2019). Currently, 38% of the world's energy is generated in the form of electricity from fossil fuels, and still, these sectors are pursuing research in development of low-range carbon emission technologies, such as solar, wind, geothermal, hydroelectricity, etc. On the contrary, transportation fuels account for larger consumption (Dudley, 2018). Despite the significance of fuels, CO2-neutral biodiesel, bioethanol, and biohydrogen are still being less used due to several concerns in biofuel production and environmental limitations. In this aspect, second-generation biomass, such as using lignocellulose and algae for fuels, have tremendous potential in a clean energy market with a value of US$500 billion by 2050 (Schenk et al., 2008). Furthermore, based on several research studies, it is well-known that microalgae biofuel production systems exhibit high net energy balance, high water efficiency, and less land requirement in comparison to first-generation biofuels (plant biomass) (Benedetti et al., 2018).
In consideration of oil extracted from plant biomass vs. algal biomass, palm oil can generate a maximum of 4–5 vs. 30 ton/ha/yr, thus yielding a 5-fold higher product in comparison to a plant crop during similar posttreatment of biomass (Benedetti et al., 2018). In terms of area, algae are known to generate about 15–300 times more oil for biodiesel (Schenk et al., 2008). In contrast to crop plants' single harvesting process, algae allows multiple harvesting cycles in the same duration with high yield and greater light and CO2 capture efficiencies.
Different oil concentrations are noted with various algae species, which is due to differences in the amount of protein/carbohydrates/fats. For instance, S. maxima has about 60~70% w/w proteins, Porphyidium cruentum generates around 57% proteins, and Scenedesmus dimorphus generates up to 40% w/w of lipids (Nigam and Singh, 2011; Venkata Mohan et al., 2011) (Table 4). In a few species of algae, such as Botryococcus braunii and Chlorella protothecoides, terpenoid hydrocarbons and lipids are noted, which can be further converted to short-chain hydrocarbons, similar to that of crude oil (Carlsson and Bowles, 2007). On the basis of prevalence of lipids or sugars, algae can be a wonderful substrate for biodiesel or bioalcohol, respectively (Kondaveeti et al., 2014a). Therefore, algae can be a great resource as a biofuel substrate under certain conditions. Several studies have employed different strategies for increasing lipid concentration of algae, such as temperature, CO2 concentration, light strength/intensity, nutrient starvation, metal, and salinity stress. It is well-known that adequate light intensity helps in overproduction of microalgae lipids. This might be because a sufficient amount of light is beneficial for storing an excess of photo assimilates, which can be further transformed into chemical energy (Zhu et al., 2016). The Nannochloropsis species of algae experienced the maximum accumulation of lipids (47% of dry weight) under light intensity of 51,800 lux (Zhu et al., 2016). In another study with Scenedesmus abundans by Mandotra et al. (2016) and Zhu et al. (2016), they noticed a 32.7% increase in lipid accumulation with an upsurge in light intensity from 3,000 to 6,000 lux. When light intensity of 5,000 and 3,000 lux were maintained, the lipid content was found to be 27 and 21%, respectively. It is recognized that higher lipid concentration is noted at maximum photosynthetic efficiency, which occurs at the light saturation point (Zhu et al., 2016). Hence, the extreme light intensity can cause photo inhibition, leading to damage of algal photo systems and, thus, reducing lipid accumulation. In the case of temperature, the optimal value for higher lipid generation achieved does vary from species to species. C. vulgaris, a microalgal species, achieved a maximum lipid concentration at 25°C. The decrease in external temperature led to an obvious decrease in lipids (Converti et al., 2009). Microalgae species S. obliquus had a lipid concentration of 18 and 40% of dry weight when the temperature was at 20 and 27.5°C, respectively (Xin et al., 2011). This suggests that an increase in temperature can increase total lipid content. However, this does not mean that all types of lipids experience an increase in concentration. A recent review by Zhu et al. (2016) provides detailed strategies for lipid enhancement.
Biodiesel is being developed as one of the most vital biofuels as basically all industrialized vehicles used for agriculture, transportation, and trade are diesel/fossil fuel–based. With an increase in algae biodiesel production, several studies had endeavored to calculate the cost of oilgae (algae oil) from large farms. Studies by Benemann and Oswald (1996) reported that the cost of oilgae would ranging from US$39 to US$69 per barrel from 4 Km2 open ponds either by using pure CO2 or flue gas from coal power stations with productivity in a range of 30 to 60 g/m2/day with a 50% algal lipid yield (Benemann and Oswald, 1996). In recent studies, it is noted that the production of oil from algae would cost around US$66–153 per barrel (Nagarajan et al., 2013). Companies generating oilgae are not optimistic in terms of production cost. Seambiotic Ltd. calculated that the cost of dried algae would be US$0.34 per kg with a productivity and total lipid content of 20 g/m2/d and 8~40%, respectively. Presuming the standard yield of lipid to be 24% without any additional cost, this would be equals to US$1.42 per kg for lipid extractions, which is equal to US$209 per barrel. With an increase in yield of lipids to 40%, the lipid extraction would cost around US$0.85 per kg, which is equal to US$126 per barrel, which points to an increase in lipid concentration decreasing oil prices. Accounting for inflation in crude oil prices in 2017, the oil prices seem to be stabilizing around US$51 per barrel (https://www.eia.gov/todayinenergy/prices.php, 2017). These estimates suggest that oilgae diesel production needs to be further improved to be economically viable and to compete with conventional fuels. However, the industrial production of biodiesel using algae is at early stages, and there is much scope for decrease in cost and increase in performance.
Reduction of CO2 Using Photosynthetic Cyanobacteria
Most types of photosynthetic bacteria derive energy from ATP, which helps in the conversion of CO2 to biomass and other products (Hatch and Slack, 1970). In comparison to eukaryotic plants and algae, photosynthetic bacteria have simpler photosystems due to the presence of pigments on their cytoplasm rather than specialized organelles (Pfennig, 1967). Photosynthetic bacteria are classified as five phyla: cyanobacteria, proteobacteria, chlorobi, choloflexi, and firmicutes (Pfennig, 1967). Cyanobacteria are capable of fixing atmospheric nitrogen and carbon. Similar to algae, they are distinct and broadly distributed and exist as biofilms or as suspended planktonic cells. Several scientists believe that cyanobacteria played a decisive role in atmospheric formation by decreasing CO2 concentration and increasing oxygen (Figure 1) (Kasting and Siefert, 2002). In the present days also, cyanobacteria are found to be a key player by accounting for 20~30% of Earth's photosynthetic activity. Owing to their simpler nature, cyanobacteria are the most efficient in atmospheric carbon utilization over algae (Berman-Frank et al., 2003). However, cyanobacteria are still limited in biomass yield as compared to algae without any structural or genetic modifications.
In recent years, the production of ethanol using microbes has gained considerable attention. In general, the biomass from photosynthetic microbes is collected and subsequently converted to biofuel by solventogenesis. This process was found to be inefficient due to lower conversion efficiency (Lau et al., 2015). Thus, intense research is being carried out on direct conversion of CO2 to fuel by photosynthetic bacteria. Although few cyanobacterial species are capable of generating ethanol in smaller concentrations as a product in biological fermentation, it is required to boost production rates as an economically viable process. Dexter and Fu (2009) made an attempt to generate ethanol by introducing the genes of pyruvate decarboxylate (pdc) and alcohol dehydrogenase II (adh) from Z. mobilis into the chromosomes of Synechocuystis sp. PCC6803, which achieved 550 mg/l of ethanol. Acetyl Co A-dependent 1-butanol production by Synechococcus elongates demonstrates its capability in generation of butanol through an autotrophic mechanism (Kusakabe et al., 2013). This species was metabolically engineered to generate isopropanol by using CO2 and light to generate 26.5 mg/l of product following 9 days of cultivation (Kusakabe et al., 2013), whereas Clostridium beijerinckii generated 1.5 g/l through a heterotrophic mechanism (Chen and Hiu, 1986). These studies suggest that usage of genetically modified bacteria can be a viable advancement to increase product formation by employing CO2 as an electron source rather than organics. Butanol is considered to be a better alternative to gasoline in contrast to ethanol as it exhibits higher energy density, less volatility, and low corrosivity (García et al., 2011; Zhang et al., 2018). Current advances in metabolic engineering of cyanobacteria for the generation of solvents from CO2 were critically reviewed elsewhere (Quintana et al., 2011).
Cyanobacterial species, such as Anabaena, Aphanocapsa, Calothrix, Microcystis, Nostoc, and Oscillatoria, are equipped for the generation of hydrogen by using a photosynthetic autotrophic mechanism (Lambert and Smith, 1977; Lau et al., 2015). Filamentous cyanobacteria generate H2 as a by-product during nitrogen fixation under N2-limiting conditions. Additionally, cyanobacteria can generate H2 by reversible activity of the hydrogenases enzyme. In general, these species are equipped to use bidirectional or reversible hydrogenase enzymes to oxidize oxygen to generate hydrogen. The enhancement in hydrogen generation can be achieved by blocking the pathways that use the hydrogenases for a reduction reaction (Tamagnini et al., 2002).
Cyanobacteria are also known to produce alkanes and alkenes, which have a desirable property for industrial application (Lau et al., 2015). Among these enzymes, acyl-acyl carrier protein reductase and aldehyde decarbonylases are the key enzymes noted for efficient conversion of fatty acids of metabolic intermediates to alkanes and alkenes (Schirmer et al., 2010). Additionally, due to similarity to algae metabolism, cyanobacteria are well-known for their effluent treatment of wastewaters that are rich in nitrogen and phosphorus contaminants.
Value-Added Products Using Algae and Cyanobacteria
Apart from the biodiesel and fuels, algae are well-known for their usage in the food industry. The commercial products of algal powders are sold in the health and food industries. Algal products, such as unsaturated fatty acids and polysaccharides from microalgae and seaweed provide a dietary fiber and health benefits. The carotenoids that present in algae serve as antioxidants and vitamin A. In the textile industry, several algal products (pigments) are used as coloring agents. In aquatic fields, algae are used as feedstock in fish, shrimp, and other seafood that are grown in enclosed environments. Carotenoids, such as astaxanthin, beta-carotene, bixin, and fucoxanthin, are used in the cosmetic and pharmaceutical industries (Hudek et al., 2014).
Anaerobic Fermentation of CO2 for Value Addition
The increase in fossil fuel consumption is leading to a shortage of fuels and increase in energy prices. In order to reimburse energy consumption from the past few decades, research has been gaining attention on renewable energy sources with a prime focus on using inorganic carbon such as CO2 as a substrate (Chang et al., 2016). The policy is to replace the fossil fuel with renewable energy sources and simultaneously decrease global carbon emissions. One of these renewable products includes CH4 generation, which can be an assurance of chemical storage of energy (Villano et al., 2010). In several cases, anaerobic methane generation is productive and is frequently used in electricity generation and as a heat energy source (combustion process). Additionally, it can be used in the generation of biodiesel, methanol, and a few other hydrocarbons, such as ethanol and butanol (Ge et al., 2014; Zakaria and Kamarudin, 2016; Wang et al., 2017). In general, methane is generated in two ways: through biotic and abiotic pathways. Abiotic pathways include the catalytic conversion or thermal split of kerogen (Lawton and Rosenzweig, 2016). Biogenic methane generation is abundantly noticed from anaerobic digestion and from dairy farms. In fact, 20% of global natural gas is being produced from methanogens (Wang et al., 2017).
Methanogenic microbes are obligate anaerobes, which are under the Euryarchaeota phyla belonging to the Archaea domain. These obligate anaerobes have the capability of sequestering the CO2 under optimized specific conditions (Zeikus, 1977; Strong et al., 2016). In gas fermentation, CO2 sequestration is carried out by hydrogenotrophic methanogens with the help of gamma- and zeta-type carbonic anhydrase enzymes. The CO2 can be digested with different forms of chemicals, such as bicarbonate, carboxylic acids, alcohols, and biogas with their respective enzymes. However, acetyl CoA is the central mediator molecule in several microbial processes for product synthesis. Methanogenic and acetogenic bacteria are frequently found in a pair when CO2 is the reduction medium (Kuramochi et al., 2013; Shi et al., 2015). In conventional anaerobic digestion, the difference in operational conditions affects the behavior and fate of both acetogenic and hydrogenotrophic methanotrophs. Under optimized conditions, the acetogenic bacterium transforms the acetic acid to H2 and CO2, which is followed by the conversion to methane via acetate (Equation 1) or the indirect conversion of H2 and CO2 (Equation 2) by hydrogenotrophic methanogenic bacterial cells (Jiang et al., 2013; Bian et al., 2018).
For efficient and stable performance in the transformation of CO2 and H2 to CH4 by hydrogenotrophic methanogens, a continuous supplement of molecular hydrogen is required. Based on several studies, the key parameters that control hydrogenotrophic methanogenic activity were identified as pressure, redox conditions, temperature, headspace gas composition, hydraulic retention time, and pH (Rabaey et al., 2009; Lee et al., 2012). Scherer et al. (2000) analyzed the degradation of organics in municipal gray water under thermophilic and mesophilic environments using a lab-scale continuous mode of operation for biogas generation. The most probable number (MPN) technique illustrated that hydrogenotrophic methanogens (H2-CO2 utilizers) were in a range of 108 to 1010/g total solids (TS), which seems to dominate the acetogenic methanotrophs by a factor of 10 to 10,000, due to short hydraulic retention time (HRT). Similarly, Ahring et al. (2001) noted that hydrogenotrophic methanogens exhibited higher specific methanogenic activity at 65°C. Although these studies prove that the presence of hydrogenotrophic methanogens can enhance methane formation, it is unclear whether enhanced methane formation is by direct or indirect conversion under practical conditions. Thus, future research in methane production should be encouraged toward the CO2-utilizing metabolic pathways that control the symbiotic relationship among acetoclastic and hydrogenotrophic methanotrophs along with acidogenic bacteria.
Gas Fermentation of CO2 to Products
The preceding advances in bio-energy generation have raised a feed vs. fuel debate. In this regard, gas fermentation looks to be a promising technology, in which gaseous substrates (CO2) are converted to biochemicals. Also, many industrial processes generate large amounts of carbon gases, which are left unused. Thereby, utilization of CO2 as a feedstock toward value-added products can shut down the feed vs. fuel debate. As aforementioned, conversion scenarios indicate that CO2-rich waste gases can be a suitable substrate for gas fermentation. Several microbes have the ability to utilize CO2 as a carbon source. Among these several microbes, acetogenic bacterium is mostly being studied and shows a capability for industrial applications. These bacterial species are equipped to convert CO2 and CO by making use of the Wood–Ljungdahl pathway (WLP). Different acetogenic bacteria can naturally generate ethanol or butanol and some other goods of industrial interest, such as 2-oxobutyrate, hexanol, PHA, vitamin B12, polymers, and their precursors for other products.
In a recent study by Kim et al. (2016) acetate was noted as an end product in a multistage gas fermentation process by using steel mill off-gas as a feedstock. In the initial stage of fermentation, CO was transformed to CO2 and H2 by Thermococcus onnurineus. Later, the mixture of CO, CO2, and H2 were converted to acetate by Thermoanaerobacter kivui and resulted in a maximum generation rate of 0.33 mmol/l/h. When the initial step was absent, T. kivui was unable to generate acetate due to CO inhibition and lack of CO2 as a substrate. Acetate formations in the multistep process also can be employed for the production of ethanol and other value-added products. Richter et al. (2013) operated a two-stage system for generation of ethanol with CO2 as a feedstock by using C. ljungdahlii. The first stage of the reactor was employed for acetate; then the broth containing acetate was transferred to a second fermenter by lowering the pH for efficient conversion of acetate to ethanol. The production rate and maximum concentrations of ethanol were noted as 0.37 g/l/h and 450 mM, respectively. Different types of clostridium strains were tested in similar systems, and it was noted that C. ljungdahlii PETC was the most optimal producer for ethanol (Martin et al., 2016). Hu et al. (2016) developed a two-stage system for generation of longer chain fatty acids (C16-C18). This was carried out by M. thermoacetica in the first stage for acetate formation with syngas as a feedstock. The effluent broth was subjected to an aerobic reactor containing genetically modified Yarrowia lipolytica. The system could generate up to 18 g/l of C16-C18 lipids at a rate of 0.19 g/l/h. The system proves the conversion of carbon in syngas to complex substrates with a multistage system.
To generate value-added products by an integration approach along with biological processes, chemical processes are also feasible. Lanzatech, in association with invista and SK innovation (http://greenchemicalsblog.com/2014/06/05/invista-and-lanzatech-expand-collaboration/; http://www.invista.com/en/news/pr-invista-and-lanzatech-make-breakthrough-for-bio-derived-butadiene-production.html), proposed production of 1, 3-butadiene in a two-step process, which is combination of biological and chemical steps. The recent review published by Liew et al. (2016) gives an excellent overview on the advances of synthetic biology on syngas-fermenting bacteria. However, we speculate that these technologies are limited by low concentration of substrate uptake, productivity, and selectivity in terms of product formation in comparison to current technologies.
Bioelectrochemical Conversion of CO2 to Fuels and Chemicals
Bioelectrochemical systems (BES), such as microbial electrosynthesis (MES), microbial electrolysis cells (MECs), and microbial fuel cells (MFCs), are gaining importance in the field of renewable energy due to their promising approach for converting waste to energy (Kondaveeti et al., 2017; Mohanakrishna et al., 2020). In general, MFCs generate electricity from organics, whereas MES synthesizes value-added chemicals from CO2 via electrogenic fermenting cathode microbes (biocathode) by using the cathode electrode as a sole electron source (Figure 4) (Logan, 2008). Nonetheless, MES is more beneficial in comparison to MFCs due to its capability in taking CO2 and slashing the greenhouse gases. In addition, MES is interesting because of energy storage in the form of chemical production (e.g., hydrogen, alcohols, and C1-C6 medium chain fatty acids) for sustainability and decrease in dependency on non-renewable sources, such as fossil fuels (Rabaey et al., 2009).
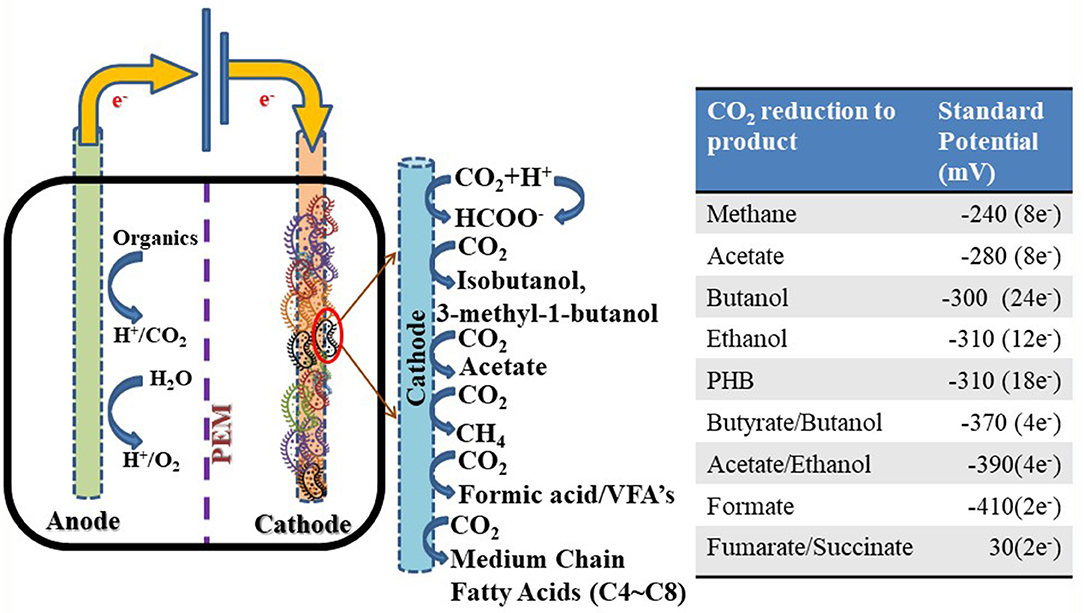
Figure 4. Schematic representation of microbial electrosynthesis system toward CO2 reduction for value-added product formation and their corresponding standard potentials.
The bioelectrochemical conversion of organics, such as acetate, in the anode of MFCs generates CO2, protons, and electrons. The generated electrons move toward the cathode through an external circuit. The protons are diffused toward the cathode through a separator, typically a proton exchange membrane (PEM, e.g., Nafion-117) or cation exchange membrane (CEM). On the cathode, the diffused protons and electrons are combined together to form water by using O2 as an electron acceptor (cathodic reduction reaction). The potential disparity between the anode and cathode are captured as voltage. When an external electron acceptor, such as O2, is absent, the diffused protons are converted to H2 with the help of minimal external voltage (0.2 V), which was termed as MEC. The initial discovery for generation of hydrogen by modifying MFC to MEC was reported by the B.E. Logan group at Penn State University (Logan, 2008). MEC operations are more economic over MFC due to the ecologically beneficial production of valuable products, such as H2. By the same process, the microbes at biocathodes can shuttle the e− from the electrode for the reduction of CO2 to value-added products. This can be achieved by using an anaerobic biotic anode with an electrogenic biocatalyst using organics or with an abiotic anode with an electrolysis process by applying additional potential. The bioprocess for value-added product formation was carried out by numerous metabolic pathways, such as the Calvin Benson cycle or the WLP (Rabaey and Rozendal, 2010; ElMekawy et al., 2016).
MES is capable of generating basic value-added products, such as H2, acetate, and oxobutyrate (Kadier et al., 2020). These products can be directly used as fuels or can be used further to generate high carbon fuels or bioplastics. Initially, Nevin et al. (2010) stated reduction of CO2 to acetate in small concentrations by operating MES at −0.6 V by using an acetogenic Sporomusaovata ascathodic biocatalyst (Nevin et al., 2010) and renewable energy (solar energy) as an external power source. This study also evidenced smaller amounts of oxobutyrate production (Table 5). Further, in 2011, acetate at a production rate of 0.05 mM/d was achieved using mixed cultures (Nevin et al., 2011). In other studies using S. ovata, the production rates were 2.3-fold higher using a nickel nanoparticle-coated cathode over a plain graphite cathode (1.13 mM/d) (Zhang et al., 2013). Mohanakrishna et al. (2015) reported an acetate generation rate of 4.1g/l by using enriched single chamber MES with VITO-activated carbon electrodes. Similarly, in other studies from the same group, they analyzed acetate formation by using bicarbonate and CO2 as a substrate by varying the poised cathode potentials (−0.6 and −0.8 V vs. Ag/AgCl). Interestingly, in this study, they noted that MES operation with CO2 at −0.8 V generated a higher acetate concentration of around 5.1 g/l. However, decreasing poised potentials (−0.6 V) during MES operation with bicarbonate exhibited maximum acetate concentrations of around 4.1 g/l (Mohanakrishna et al., 2016). Batlle-Vilanova et al. (2016) operated continuous mode MES for CO2 reduction and noted an acetate production rate of 0.98 mM.l/d. Marshall et al. (2013) reported the highest acetate generation (17.25 mM/d) from CO2 by using MES (−0.79 V) with a carbon bed cathode and enriched cultures from methonogenic bacteria and H2. MES operation with a simultaneous production and extraction mechanism for acetate from CO2 were operated by Gildemyn et al. (2015). In this study, the maximum concentration of acetate formation was noted as 13.5 g/l, which is found to be the highest to date, whereas the highest production rate of 0.78 g/l/h was registered by LaBelle and May (2017). This might be due to the ceasing of acetate oxidation at the cathode by employing simultaneous production and extraction techniques. All these studies pointed out that, during long-term operation, the oxidation of acetate to methane is one of the major limitations. This can be controlled by employing a chemical treatment process, such as by using BESA (2-Bromoethanesulfonic acid) or employing pH shock treatment (Mohanakrishna et al., 2015, 2016; ElMekawy et al., 2016). The variation in acetate production might be due to differences in applied voltage, operational environment, reactor configuration, electrode type, biofilm activity, and type of bio-electrocatalyst at the cathode.
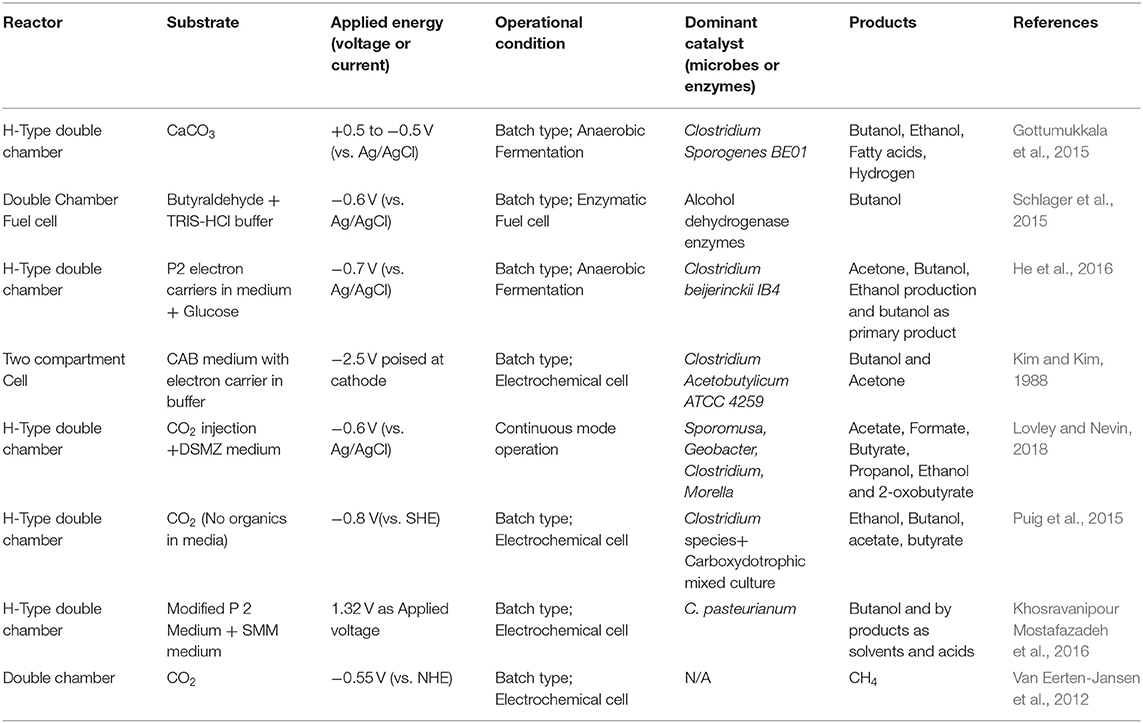
Table 5. Bio electrochemical generation of solvents and biofuels from CO2 under various operational and nutritional conditions.
Compared to pure culture of C. ljungdahlii, enriched mixed culture results showed a higher acetate (1.3 mM/d) production rate at −1.1 V, which has hydrogen-generating potential (Bajracharya et al., 2015). This study also evidenced methane production and suggests an increase in CO2 reduction by using mixed cultures by supplementing H2. The supplementation of H2 can be obtained by maintaining higher negative cathode potentials for the reduction of protons to H2. However, generated H2 can leak from the reactors, thereby restricting the CO2-reduction process, which, in turn, decreases current and product formation. This complication can be overcome by using high gas absorption electrodes (e.g., platinum single crystal electrode) in order to decrease H2 leakage (Will, 1965; Wang Q. et al., 2015). Several pure cultures, such as Clostridia, Moorella, and Sporomusa, are proven for direct conversion of CO2 by accepting electrons from a poised cathode electrode at −0.6 V (Nevin et al., 2010; Bajracharya et al., 2015); however, these are still limited for practical applications due to strict operational conditions.
In MES, acetate is one of the primary products noticed from the reduction of CO2 (Mohanakrishna et al., 2016; Modestra and Mohan, 2017). However, its autotrophic production is not economically desirable due to lower yields and little commercial value. In this aspect, researchers have further explored the possibility of higher value-added product generation (Rabaey and Rozendal, 2010; Arends et al., 2017). In this facet, acetic and butyric acids were converted to bioalcohols using a poised biocathode at −0.65 V (Steinbusch et al., 2010; Sharma et al., 2013b). In this study, total alcohol production of 13.5 mM was achieved. Ethanol, butanol, propanol, and acetone were noticed as major alcohols along with propionic and caproic acids (Sharma et al., 2013a). Similarly, Kondaveeti et al. operated a BES and transformed the VFAs from anaerobic digester effluent to bioalcohols by varying applied voltage. Electron mediators, such as neutral red, helped for increasing product formation at higher redox potentials (Kondaveeti and Min, 2015). Ethanol and butanol are the major alcohols noticed at the applied voltage of 2 V (Cathode potential, −0.9 V). Likewise, Steinbusch et al. (2010) operated a microbial electrosynthesis system with various mediators [methyl viologen (MV), neutral red (NR), anthraquinone-2,6-disulfonate (AQDS)] and noted a maximum yield of 13.5 mM of ethanol by using MV. In other studies pursued by Van Eerten-Jansen et al. (2013), a reduction in acetate to Caproate (6.8 mM) and butyrate (3 mM) were noticed in MES with cathode poised potential of −0.9 V. Recently, Vassilev et al. (2019) operated a dual cathode MES system for concurrent acetogenesis and solventogenesis followed by carbon chain elongation. This type of system can assist in operational cost reduction with a simultaneous increase in productivity due to the utilization of off-gas. All previous studies are found to be interesting in terms of product formation; however, these systems are still limited by selectivity of product formation and extraction of product using cheaper processes (del Pilar Anzola Rojas et al., 2018; Li et al., 2018; Vassilev et al., 2018). Most of the product extraction studies of MES include integrated membrane separation with an anion and cation exchange membrane or sequential operation. However, the operation of such processes can be expensive as they often need to be changed because of fouling during longer operation. The research in this regard needs to be pursued to increase the production rate and decrease operational costs for product extraction and selectivity of product formation.
In the past few years, research on generation of methane from CO2 using bioelectrochemical techniques has increased significantly. It has been noted that the optimized electrode potential (direct electron donor) for direct conversion of CO2 to methane was found to be around −0.6 to −0.7 V vs. Ag/AgCl (Cheng et al., 2009; Villano et al., 2010; ElMekawy et al., 2016; Bajracharya et al., 2017). Villano et al. (2010) reported that, using −1.0 V of applied external potential, 96% of the obtained current was captured in the form of methane. Based on current and obtained abiotic hydrogen, it was noted that CH4 was produced directly from electrons derived from a cathode electrode rather than using the hydrogen gas. Alternatively, they also have suggested that some amount of methane generation is by hydrogenophilic methanogens by consuming H2 from abiotic water reduction. The performances of biocathodes were further tested by inoculating with methanogenic culture. These cultures were greatly able to reduce the inorganic carbon to methane (0.055 mmol/d for mg/VSS) with 80% electron capture efficiency (Villano et al., 2010). The standard theoretical potential for the reduction of CO2 to CH4 is −0.44 V vs. Ag/AgCl. The required energy for this cathodic reaction can be achieved from the bioanode (organic oxidation) process. However, in practical operation, these can be greatly limited with over-potentials. These over-potentials can be reduced by implementing biocathode systems. In another study, Villano et al. (2013) demonstrated a double chamber MEC for methane generation with anode potential of −0.397 V vs. Ag/AgCl by using acetate as an energy source. Simultaneously, a cathode was constantly supplemented along with CO2 and N2 for pH correction and carbonate supply. It was reported that a 94% reduction in acetate at the anode chamber with 91% of coulombic efficiency, which proves that the generated current was mainly from methane production. Yasin et al. operated an enriched activated sludge system to generate methane from CO2. In this system, in the presence of CO2 and H2, they noticed a 70-fold increase in methane generation after 72 h of operation. The complete utilization of CO2 was noticed after 96 h of incubation under supplementation of CO2 and H2 as carbon and electron sources, respectively (Mohd Yasin et al., 2015). Based on a recent study by Xenia et al., the microbial electrosynthesis cells were proven to be economical and sustainable with CO2 as a substrate for product formation, such as methanol, ethanol, and formate (Christodoulou et al., 2017).
Other CO2-Utilizing Bacteria
β-Proteobacteria
Proteobacteria are Gram-negative bacteria, mainly composed of lipopolysaccharides in their outer membrane. These are found to be a geologically, environmentally, and evolutionarily important group of microorganisms. The members of this bacterial phylum exhibit an enormous metabolic diversity. Most of these bacteria have an industrial, medical, and agricultural significance (Sezenna, 2011). The photosynthetic proteobacteria are known as purple bacteria, concerning their reddish pigments. One of the most common and well-known photosynthetic β-proteobacteria is Ralstonia eutropha. This bacterium can grow on variable carbon environments, such as heterotrophic, autrotrophic, and mixotrophic. In the absence of a heterotrophic carbon environment as an electron source, this bacterium has proven to utilize hydrogen as an energy source for fixing CO2 via the Calvin–Bassham cycle (CBB) (Figure 5) (Jajesniak et al., 2014).
R. eutrohpa is a convenient photosynthetic bacterium to handle because of its aerobic H2 oxidizing capabilities over obligate anaerobes to generate chemicals and other value-added products. This fascinating interest in R. eutrohpa has led to generation of bioplastics (PHAs/poly hydroxyalkanoates) by storing carbon in cytoplasm. PHAs are generally composed of PHB (poly 3-hydroxybutyrate) and PHBV (poly 3-hydroxybutyrate-co-3-hydroxyvalerate) (Luengo et al., 2003; Jajesniak et al., 2014). These bioplastics are gaining high importance due to credentials such as biodegradability over conventional plastics and their green synthesis with CO2 sink. These polymers with desired length can be pursued with genetic modifications (Reinecke and Steinbüchel, 2009). Few studies are pursued in genetic modification of R. eutropha for the generation of products with variable properties (mechanical strength) and production yield. Voss et al. modified R. eutropha and generated a cyanophycin, a protein-like polymer, with an increased production quantity (Voss and Steinbüchel, 2006). A few other studies have synthesized several useful compounds, such as ferulic acid (precursor to vanillin biotransformation) and 2 methyl citric acid, by genetic modification (Overhage et al., 2002; Ewering et al., 2006). The limitation in these studies is use of organic carbon as an energy source rather than inorganic CO2. Beller et al. engineered R. eutropha and generated methyl ketones of diesel range with productivity in a range of 50–180 mg/l by using CO2 and H2 as energy and electron sources, respectively (Müller et al., 2013). In a previous study, Li et al. (2012) described an integrated approach for production of isobutanol and 3-methyl-1-butanol by using a microbial electrochemical process. In this system, initially, CO2 was converted to formate and tailed by isobutanol and 3-methyl-1-butanol generation. This system achieved a final concentration of 850 and 570 mg/l of isobutanol and 3-methyl-1-butanol, respectively.
Idenolla species is another type of photosynthetic bacteria similar to R. eutropha in generation of polymers by using CO2. An additional superiority of Idenolla over R. eutropha is the generation of products using carbon monoxide (CO) as an energy source. These bacterial species have an additional advantage by synthesizing products from exhaust gas (Tanaka et al., 2011).
Clostridia
Most clostridia are anaerobic and Gram-positive bacteria. They are found to have great importance in (i) human and animal health and physiology, (ii) anaerobic mortification of simple and complex carbohydrates, (iii) the carbon cycle, and (iv) bioremediation/degradation of complex organic chemicals (Kondaveeti et al., 2014b). Many strains of clostridia are capable of autotrophic fixation of CO2 or CO by using H2 as an electron donor (Willey et al., 2014). Clostridia also can use simple chain organic carbon molecules, such as formate or methanol, as an energy source. They can achieve this by the WLP pathway in which two molecules of CO2 are scaled down to generate one molecule of Acetyl-CoA with CO or H2 being used as a reducing proportionate (Tracy et al., 2012).
Additionally, Clostridium species provide many fascinating characteristics for biotechnological utilization: (i) ability to use simple as well as complex substrates such as H2, CO2, and CO; (ii) equipped with diversified pathways for generation of value-added products; and (iii) tolerant to toxic metabolites and substrates. However, as they are strictly anaerobic, the cultivation of Clostridium is very difficult (Willey et al., 2014). Normal atmospheric and facultative conditions are lethal to Clostridium species. Early work with Clostridium was mostly carried out for the production of acetic acid and other related products. The synthetic biology/genetic modification of Clostridium are being pursued at a slow pace due to their difficulty in lack of genetic tools as found in R. eutropha. However, the repertoires of genetic tools are applicable for Clostridium with development in plasmid DNA and chromosomal manipulation technologies (Tracy et al., 2012). The uses of mobile group II introns are generally targeted for gene disruption and genetic engineering of Clostridium. Cooksley et al. (2012) demonstrated a ClosTron application through genetic engineering in Clostridium acetobutylicum for acetone-butanol-ethanol (ABE) fermentation and for expression of cellulosome. Leang et al. (2012) employed double crossover homologous recombination technology and an improved electroporation protocol to the process in a proof-of-concept gene deletion study on the species of C. ljundahlii. Likewise, recent studies by the D.R. Lovley group demonstrated introduction of foreign genes and deletion of certain genes in C. ljungdahlii to enhance the production of biocommodities. Here, deletion of the ethanol-production pathway led to an increase in acetate production. The homolog recombination method described in their study can be applicable for the introduction of desired metabolic genes or reporters into the chromosomes of C. Ljungdahlii (Leang et al., 2012; Ueki et al., 2014).
Archaea
Archaea are generally found in abnormal ecological niches, such as high and low temperatures, acidic and high-saline environments, and anaerobic atmospheres. The hydrogenotrophic methanogens of Archaea use H2 as an energy carrier and CO2 as a carbon source for CH4 generation. Furthermore, several strains of Archaea belonging to the haloarchael group are equipped with a metabolic pathway for accumulation of PHA from CO2. As most of the Archaea are thermophilic in nature, this becomes an excellent source of the carbonic anhydrases (CA) enzyme, which is thermo stable and convenient for industrial CO2 capture and a platform for gas fermentation (Willey et al., 2014). The recognition of genetic tools for Archaea might enhance the product yield in the gas fermentation process. Recently, genetic reengineering of Archaea for valuable product formation has been rising. Keller et al. (2013) reported a heterologous expression of five genes from a carbon fixation pathway in Metallosphaera sedula into hyper theromophilic Pyrococcus furious, which grows on organic carbon at 100°C. Engineered P. furious is capable of incorporating CO2 for generation of 3-hydroxypropionte-4-hydroxybutyrate (3BHP) at an optimum condition of M. sedula; i.e., at 70°C. This temperature-dependent strategy for generation of valuable product formations is established in the work of Basen et al. (2012).
Key Role of Enzymes in Microbial Cells for Conversion of CO2
Adaptation of CO2 to value-added products in the microbial kingdom has been found with six different pathways, such as WLP, Calvin Benson Bassham cycle, reductive tricarboxylic acid cycle (reductive/reverse TCA cycle), dicarboxylate 4-hydroxybutyrate cycle, 3-hydroxypropionate bicycle, and 3-hydroxypropionate 4-hydroxybutyrate cycle. These pathways are not found in every species, but specific pathways are found in specific organisms (Figure 5). Among these, the first three routes were found to be dominant for CO2 conversion, and these are discussed in detail.
Calvin Cycle for the Phototrophic Reduction of CO2
The Calvin cycle is one of the important biochemical cycles in photosynthetic organisms, which are capable of incorporating CO2 into carbon metabolism. Thus, this metabolic function is dominant for CO2 reduction in nature. In general, three key enzymes and three vital steps of the CO2 process are noticed in the Calvin cycle (Venkata Mohan et al., 2016). The steps are known for CO2 fixation, CO2 reduction, and regeneration of CO2. In the first step, the 1-5, bisphosphate ribulose bis-phosphate carboxylase (RuBisCo) catalyzes the reaction between CO2 and 1-5, bisphosphate ribulose, and this process generates 3-phosphoglycerate (Shi et al., 2015). Later, 3-phosphoglycerate is further converted to 1,3-diphosphoglycerate by accepting inorganic phosphate (Pi) from ATP and further reduced to 3-phosphate glyceraldehyde with the help of the phosphoglyceraldehyde dehydrogenase enzyme. In the final step, phosphate glyceraldehyde is further transformed to 5-phosphate ribulose via a series of enzymatic reactions following further with its activation by phosphoribulokinase in ATP-dependent condensation to synthesize 1-5, bisphosphate ribulose for CO2 collection for further cycles (Willey et al., 2014). One sixth of 3-phosphate glyceraldehyde is transformed as sugars, fatty acids, amino acids, etc.
Reverse TCA (Tricarboxylic Acid Cycle) Cycle
The reverse TCA (rTCA) cycle is known as the reductive citric acid cycle (reverse Krebs cycle), which basically runs in the reverse pathway. In this cycle, CO2 and water are converted to carbon compounds (Lehninger et al., 2005). The reductive TCA cycle was first identified in anaerobic Chloorbium limicola, a green photoautotrophic sulfur-reducing bacteria. It was also identified in another thermophilic bacterium that uses hydrogen and sulfur as an energy source. The reverse TCA cycle contains four steps of carboxylation, in which, initially, succinyl-CoA is reductively carboxylated with CO2 by α-Ketoglutarate synthase/2-oxoglutarate synthase (ΔGo, +19 kJ/mol; first step) to generate α-Ketoglutarate/2-oxoglutarate by utilizing two equivalents of reduced ferrodoxin. Next, α-Ketoglutarate/2-oxoglutarate along with CO2 is converted to isocitrate (ΔGo, +8 kJ/mol; second step) by using isocitrate dehydrogenase and NADPH. Further, isocitrate is converted to citrate by a cleavage mechanism, followed by oxaloacetate and acetate-CoA by ATP citrate lyase. The terminal compounds are carboxylated with CO2, which requires a pyruvate synthase enzyme (ΔGo, +19 kJ/mol). The synthesized pyruvate is activated by pyruvate kinase to yield phosphoenolpyruvate (PEP), which is further converted to bicarbonate by a carboxylation mechanism (ΔGo, −24 kJ/mol). As an outcome, oxaloacetate is generated and finally converted to succinyl-CoA by a series of enzymes (Venkata Mohan et al., 2016).
Reverse Acetyl-CoA Cycle
The reverse acetyl-CoA pathway is predominantly found in strict acetogenic (Eubacteria) and methanogenic (Euryarchaeota) bacteria. This route was suggested by Ljungdahl and Wood (2013), and it was named after them (Garrett and Grisham, 2008). Unlike the Calvin and reductive TCA cycles, the reductive acetyl-CoA is a non-cyclic route. In this route, initially, CO2 is converted to formate by using NADH-dependent formate dehydrogenase (FateDH) (ΔGo +22 kJ/mol). Later, formate is apprehended by tetrahydrofolate and reduced into a methyl group by generating methyl-H4 folate. Methyltransferase transfers the methyl group of methyl-H4 folate to the cobalt center of hetero dimeric corrinoid iron sulfur protein, thus generating methylated corrinoid protein. Further, CO dehydrogenase (CODH) functions to convert CO2 into CO via acetyl CoA synthase accepting the methyl group from CH3-CoIII and converts CO, CoASH, and methyl groups to acetyl CoA (Mohanakrishna et al., 2015; Shi et al., 2015). As noted in the above enzymatic reaction, the autotrophic mechanism is by either CO2 or bicarbonate as the carbon source. This mainly depends on the specific enzyme in a specific CO2 fixation/conversion reaction. The attribute difference is noted in carbon source, and a fast-reliable conversion of CO2 to bicarbonate is required.
Synthetic Biology in the Conversion of CO2
Direct use of microbes in generation of value-added products is typically hindered by several characteristics/properties, such as low product yield, expensive cultivation condition, and inadequate growth rate. In this aspect, synthetic biology has gained importance due to its interest in product formation by altering biochemical pathways or by introduction of heterologous pathways into microbes for favorable features (Ferry et al., 2012). With consideration of recent advances in metabolic and protein engineering, the synthetic biology proves to be an excellent tool for boosting up biological process and to improve economic viability. In this section, E. coli is given special attention as it is the most often used microbe in the field of synthetic biology (Sawitzke et al., 2007).
Genetic Engineering for CO2 Conversion
Despite being heterotrophic in nature, the Gram-negative E. coli gained its place at the table of genetic engineering due to its simple cultivation, low cost, easy transformation, fermentation, and high production for new technologies, which include using CO2 as a carbon source (Willey et al., 2014). Zhuang et al. utilized different fermentation conditions for heterologous expression of RuBisCo and phosphoribulokinase (PRK) in E. coli (Zhuang and Li, 2013). In this study, they noticed a 67-mg CO2/mole arabinose/l/h as the CO2 fixation rate by a 15% decrease in CO2 reduction during the fermentation process. Heterologous expression of carbonic anhydrase (CA) in E. coli is extensively reported. CA expression of Methanobacterium thermoautotrophicum in periplasm of E. coli resulted in a whole cell biocatalyst for CO2 hydration. These systems successfully hydrated the CO2 with a catalytic efficiency and productivity similar to free enzymes (Patel et al., 2013). The smaller difference observed in productivity might be due to mass transport limitations. In the presence of CaCl2 and CaCO3, a 50~70% increase in carbon precipitation is noticed in comparison to operation without calcium (carbonate and chloride) components (Jo et al., 2013). Despite using free enzymes, whole cell utilization provides additional advantages such as (i) an increase in stability (100% in mortality activity after 24 h of use) and (ii) by passing the protein purification (whole cells are isolated and recycled). Similarly, as M. thermoautotrophicum, the periplasmic expression of CA is noticed in Neisseria gonorrhoeae (Jo et al., 2013). These advances noted in E. coli are not confined in the expression of individual enzymes. Bonacci et al. (2012) studied the expression of efficient carboxysomes from Halothiobacillus neapolitanus and encoded 10 genes in E. coli and noted that these bacteria are capable of carbon fixation. Further, they noticed an in vitro CO2 fixation mechanism by using expressed carboxysomes with a sucrose gradient centrifugation as a purification step.
Synthetic biology of E. coli has provided a possibility of encoding photo bacterial genetics for the generation of value-added products. For instance, brown algae are well-known feedstock for the generation of carbon-neutral biofuel. However, from the point of economic industrial viability, these are limited by their inability to utilize alginate. Wargacki et al. (2012) reported the DNA fragments in Vibrio splendidus that are responsible for secretion of enzymes that are capable of utilization of alginate. This enzyme expression in E. coli resulted in a bacterial platform that is capable of metabolizing alginate with a yield of 0.281 weight ethanol/dry weight of microalgae that is equivalent to 80% of the theoretical basis of total polysaccharide composition. Along with several bacterial species, Saccharomyces cerevisiae also gained attention in conversion of CO2 to value-added products. However, it has been limited with low productivity as compared to E. coli.
Future Prospects
The concepts of green and biological energy and/or chemical generation have been settling firmly in academia and industrial aspects, especially in utilizing CO2 (Kuk et al., 2019). These processes guide the development of environmentally friendly products by optimized design concepts. The existing carbon capture technologies require huge land areas, large volumes, high energy, and expensive catalysts, and they also consume chemicals (ElMekawy et al., 2016). Therefore, based on reviewing the biological processes here, we suggest that biological systems align perfectly with green chemistry platforms for sustainable carbon capture and sequestration. The importance of biological processes for chemical generation using CO2 as a carbon source is increasing rapidly (Venkata Mohan et al., 2016). From an economic point of view, all the biological processes for chemical/fuel production cannot be an industrially viable process. However, in consideration of carbon-neutral green energy generation, challenges, and their improvements, the biological adaptation of CO2 utilization seems to be promising. The integration of a biological CO2-mitigation process with conventional wastewater treatment technologies can minimize the economics related to waste remediation (Sadhukhan et al., 2016). Concurrently, process integration can be beneficial in generating sustainable energy or fuels with a minimum carbon footprint. Yet the conversion of low concentrated waste organics into fuels or chemical commodities is required to achieve selective product formation with use of biocatalytic technology by economic evaluation, mathematical modeling, optimization, and integration of energy-efficient processes (ElMekawy et al., 2016; Sadhukhan et al., 2016). In this regard, the MES technology has proven to be a potential approach for conversion of CO2 to chemicals and fuels.
Moreover, the scaling up of MES is an advancement to MFC that produces high-value products. Likewise, it has the capability to become an alternative to fossil fuels and also to eliminate the food vs. fuel debate. However, there are few constraints that should be solved, for instance, the low electron intake by bacteria at the cathode and low productivity, stability, selectivity, etc. The synergistic effects of the substrate, electrode materials, and bacterial cell should be studied and well-understood for up-scaling. The conventional anaerobic fermentation system necessitates ~3 kg of glucose for production of 1 kg of butanol, which seems to be non-optimal in spite of existing large-scale processes (He et al., 2016). In this regard, alcohol generation in MES using organic-rich wastewater seems to be a promising choice (Rabaey and Rozendal, 2010). More specifically, influence of current and electrical potential on the microbial metabolism and microbial strains related to electro fermentation or MES are required to consider for industrial application (Mohanakrishna et al., 2016). Microbial carbon capture is likely to be a standalone process that could be competitive to conventional technologies. Therefore, coupling of biological CO2 processes with chemical processes, such as gasification and water gas shift reaction, could be beneficial. Complex biomass sources, such as cellulosic, wood, and straw materials, cannot be easily degraded or fermented. A good alternative is biomass gasification, which converts carbonaceous compounds into syngas through partial oxidation. H2 and CO2 in syngas also can be utilized by microbes for product formation. The conversion of syngas to products with a higher productivity rate can be achieved with bioelectrochemical and photobiological processes (Jiang et al., 2013). Further research would be required in order to enhance biofuel generation with low energy utilization and a minimal amount of toxic by-product generation (Rabaey and Rozendal, 2010; Mohanakrishna et al., 2015; ElMekawy et al., 2016; Venkata Mohan et al., 2016).
In terms of biotechnology of CO2 utilization, algae and cyanobacteria have gained importance due to enhanced biomass generation for a feedstock and transesterification process. However, β-proteobacteria, clostridia, archaea, and other bacterial species can overtake the photosynthetic microbes due to rigorous research and efforts (Wang et al., 2012). Nonetheless, in utilization of various microbes for successful bioprocess development, one needs to pay attention to vital necessities, such as (i) suitable and appropriate strain selection for higher lipid generation (e.g., cell growth, autotrophic carbon fixation rate, product type and yield), (ii) supplementation minerals/chemical in CO2 fixation, (iii) operation parameters (gas concentration, composition, etc.), (iv) type of bioprocess operation (batch or continuous), and (v) reactor configuration (CSTR, fixed bed reactor, membrane reactor) (Li et al., 2014). A few decisive factors in biological processes and engineering of carbon capture and utilization include (i) safety (especially during the H2 utilization as electron donor/source), (ii) type of product selectivity and formation (concerning the purification and extraction economy), (iii) optimized operational conditions, and (iv) an increase in productivity by amplifying the cell density, etc.
In overview, the earlier described bioelectrochemical reaction pathways using CO2 as the feedstock are based on H2 as a reaction companion, whereas algae can transform CO2 into value-added products, such as omega fatty acids, proteins, and amino acids and can simultaneously reduce pollutants. Likewise, other technologies, such as enzymes (e.g., formate dehydrogenase) can utilize/transform CO2 by providing necessary energy. Figure 6 provides an overview of different CO2-based product formation pathways and their current status of employment. Apart from urea, the leading CO2-based product formations from other industrial processes involve the production of cyclic carbonates and salicylic acids, which are being produced at about 0.1 million tons/year (technology readiness level (TRL): 9) (Bazzanella and Ausfelder, 2017). The poly (propylene) carbonate and polycarbonate etherols are extensively produced polymers in industries using CO2 as a building block. Novomer incorporation from the United States and Covestro (former Bayer Material Science) are dynamic in this area with large-scale operations (TRL7-9). DNV (Det Norske Veritas) from Norway has constructed a pilot-scale operation plant for formic acid generation with a capability of 1 kg/day via electrochemical CO2 reduction. Similarly, Mantra Energy Alternative Limited from Vancouver, British Columbia, Canada, is constructing a plant for 100 kg/day formic acid generation (TRL7) by electro-reduction of CO2 (Bazzanella and Ausfelder, 2017). Mineral carbonation, also known as carbon mineralization, is already being used in smaller and semi-commercial plants for various purposes, such as treatment of industrial waste, polluted soil, and generation of cement-like building materials (TRL7-9). There are several other processes being researched at lab scale with significantly lower TRL. Among these, one approach is noteworthy to point out because of its huge potential in the direct synthesis of dimethyl ether (DME) from CO2. This method allows a CO2 reduction of 0.125 t CO2 /t DME, which is known to be higher in comparison to the state-of-the-art process with an intermediation of methanol equivalent to a 30% reduction. There is more production pathways research that involves direct production of sodium acrylate from ethylene and CO2 or electrocatalytic conversion of CO2 to ethylene (Alexis Bazzanella, 2017).
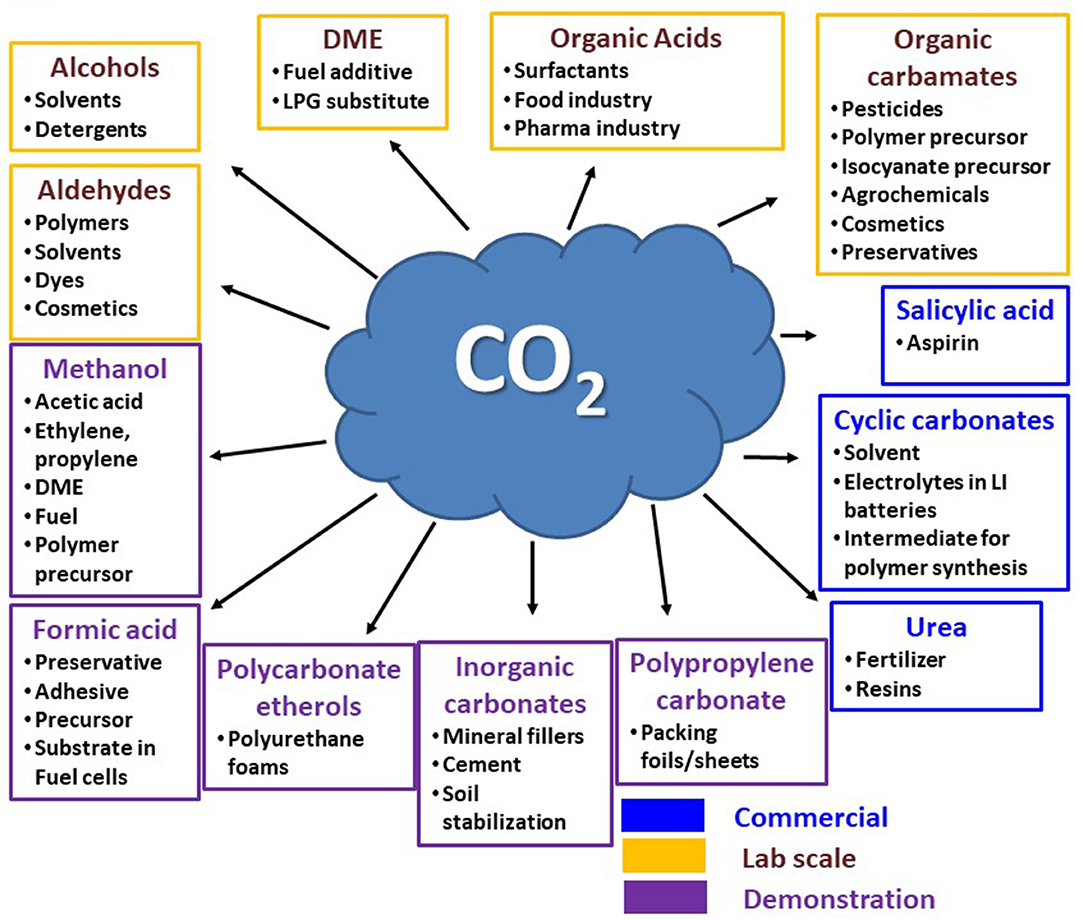
Figure 6. CO2 conversion to target products and their position of implementation. Redrawn from (Bioenergy, IEA – 2020; ISBN 978-1-910154-69-4).
One of the crucial parameters to be considered for commercialization of CO2 reduction via biological routes is the CO2 that is stoichiometrically converted per ton of product. Based on earlier studies, acetic acid, ethanol, succinic acid, and caproic acid have stoichiometric production of 1.47, 1.91, 1.49, and 2.28 t CO2/t, respectively. These chemicals (acetic acid, ethanol, succinic acid, and caproic acid) have a projected market value of 16.4 (Acumen Research Consulting, 2019), 54.63 (Coherent Market Insights, 2017), 0.237 (Markets Markets, 2019), and 0.27 (Market Study Report, 2019) billion dollars by 2026. Moreover, these products, such as acetate, can be used as building blocks for higher valued product generation, such as ethyl acetate, with a projected market value of 95 billion dollars by 2024 (Global Market Insights, 2018). Also, the combination of ethanol and other compounds (e.g., volatile fatty acids) can be used for a carbon chain elongation reaction. On the basis of these projected market values, the mitigation of CO2 to product formation can be beneficial. However, these biological approaches need a further life cycle assessment (LCA) in order to compare benchmarks with the state-of-the-art production, considering energy aspects, etc.
In order to implement innovative biological ideas on a commercial scale and to reach the visionary target, consideration of the following aspects can be beneficial: (i) high-performing conversion technology on the level of CO2 upstream: The technology should ideally cope with given impurities and/or CO2 gas concentrations of their client's CO2 waste streams at minimal pretreatment costs. CO2 conversion process: convert CO2 in an efficient way (total CO2 conversion and selectivity toward product of interest) at an appreciable productivity (space–time yield) toward product in order to minimize investment and operational costs and obtain a techno-economic feasible route. Downstream: obtain high product titers and high product selectivity in order to reduce further downstream processing steps or costs. (ii) Local value chains fit for given CO2-to-product pathway via biological route. (iii) Legislation for the end product in case of high-volume application (ethanol, fuels) (Carr, 2005).
Indeed, some of the major challenges of CO2 reduction are the availability of renewable energy to sufficiently supply the potential biological CO2 conversion strategies (via green H2 production), location, and transport of product. In this regard, low potential photovoltaic energy prices in the Middle East, Southern Europe, and North Africa would enable the production of liquid CO2 fuels that can be easily transported to Europe, for instance. This enables the creation of new value chains and business cases of interest. As energy prices are high today and scales are small, we need high-value product routes or high volume that is supported by proper legislation. In the future, at low energy prices and larger scale, the challenge would be the energy supply in the most ambitious scenario, in which the full potential for given chemical/fuels is valorized via the CO2 route (Tahvonen et al., 1994; Illing et al., 2018).
Conclusions
This report has overviewed and discussed potential applications of biological processes for CO2 fixation with generation of value-added products. Despite achieving some progress, there is still a need for finding suitable processes or microbial strains for CO2 mitigation. For instance, the productivity of microbial CO2 sequestration should be more than 3-fold higher than traditional CO2 technologies, which seems to be a daunting goal with current methods. Thus, the combination of industrial processes with microbial CO2 mitigation can be sustainable and economically viable for reduction of CO2. The metabolism of CO2 conversion by algae and photosynthetic processes can be equally viable as chemical and electrochemical technologies. Furthermore, market analysis and commercialization need to be further discussed by considering the real field application. Bioelectrochemical CO2 conversion and photo biological processes for value-added product synthesis seems to be an economical and sustainable approach toward future technological development and commercialization. However, integration of suitable CO2-converting processes with CO2-generating processes helps for the development of a CO2-based bio-refinery that may lead to carbon-neutral industrialization.
Author Contributions
SK: inception of review concept, major write-up part of the review. IA-R: editing of review and supervision and Co-Lead PI of the project. GM: involved in writing two sections of the manuscript, editing of review and supervision. MB: involved in writing future prospective of the CO2 transformation/reduction section of the review. DP: editing of review and supervision. All authors contributed to the article and approved the submitted version.
Funding
This publication was made possible by NPRP grant # 6-289-2-125 from the Qatar national research fund (a member of Qatar Foundation). The statements made herein are solely the responsibility of the authors.
Conflict of Interest
The authors declare that the research was conducted in the absence of any commercial or financial relationships that could be construed as a potential conflict of interest.
References
Acumen Research Consulting (2019). Acetic Acid Market Value to Reach US 16.4 Billion by 2026. Available online at: https://www.acumenresearchandconsulting.com/acetic-acid-market (accessed February 15, 2020).
Ahring, B. K., Ibrahim, A. A., and Mladenovska, Z. (2001). Effect of temperature increase from 55 to 65°C on performance and microbial population dynamics of an anaerobic reactor treating cattle manure. Water Res. 35, 2446–2452. doi: 10.1016/S0043-1354(00)00526-1
Alexis Bazzanella, D. K. (2017). Technologies for Sustainabilityand Climate Protection –Chemical Processes and Use of CO2. Available online at: https://dechema.de/en/energyandclimate_CO2_Buch_engl.pdf (accessed February 15, 2020).
Allen, M. M., and Stanier, R. Y. (1968). Selective isolation of blue-green algae from water and soil. Microbiology 51, 203–209. doi: 10.1099/00221287-51-2-203
Anbalagan, A., Toledo-Cervantes, A., Posadas, E., Rojo, E. M., Lebrero, R., González-Sánchez, A., et al. (2017). Continuous photosynthetic abatement of CO2 and volatile organic compounds from exhaust gas coupled to wastewater treatment: evaluation of tubular algal-bacterial photobioreactor. J. CO2 Util. 21, 353–359. doi: 10.1016/j.jcou.2017.07.016
Arends, J. B. A., Patil, S. A., Roume, H., and Rabaey, K. (2017). Continuous long-term electricity-driven bioproduction of carboxylates and isopropanol from CO2 with a mixed microbial community. J. CO2 Util. 20, 141–149. doi: 10.1016/j.jcou.2017.04.014
Bajracharya, S., Ter Heijne, A., Dominguez Benetton, X., Vanbroekhoven, K., Buisman, C. J. N., Strik, D. P. B. T. B., et al. (2015). Carbon dioxide reduction by mixed and pure cultures in microbial electrosynthesis using an assembly of graphite felt and stainless steel as a cathode. Bioresour. Technol. 195, 14–24. doi: 10.1016/j.biortech.2015.05.081
Bajracharya, S., Yuliasni, R., Vanbroekhoven, K., Buisman, C. J. N., Strik, D. P. B. T. B., and Pant, D. (2017). Long-term operation of microbial electrosynthesis cell reducing CO2 to multi-carbon chemicals with a mixed culture avoiding methanogenesis. Bioelectrochemistry 113, 26–34. doi: 10.1016/j.bioelechem.2016.09.001
Basen, M., Sun, J., and Adams, M. W. W. (2012). Engineering a hyperthermophilic archaeon for temperature-dependent product formation. MBio 3, e00053–e00012. doi: 10.1128/mBio.00053-12
Batlle-Vilanova, P., Puig, S., Gonzalez-Olmos, R., Balaguer, M. D., and Colprim, J. (2016). Continuous acetate production through microbial electrosynthesis from CO2 with microbial mixed culture. J. Chem. Technol. Biotechnol. 91, 921–927. doi: 10.1002/jctb.4657
Bazzanella, A., and Ausfelder, F. (2017). Low Carbon Energy and Feedstock for the European Chemical Industry. Frankfurt am Main: DECHEMA; Gesellschaft für Chemische Technik und Biotechnologie eV.
Benedetti, M., Vecchi, V., Barera, S., and Dall'Osto, L. (2018). Biomass from microalgae: the potential of domestication towards sustainable biofactories. Microb. Cell Fact. 17:173. doi: 10.1186/s12934-018-1019-3
Benemann, J. R. (1993). Utilization of carbon dioxide from fossil fuel-burning power plants with biological systems. Energy Convers. Manage. 34, 999–1004. doi: 10.1016/0196-8904(93)90047-E
Benemann, J. R. (1997). CO2 mitigation with microalgae systems. Energy Convers. Manage. 38(Suppl.), S475–S479. doi: 10.1016/S0196-8904(96)00313-5
Benemann, J. R., and Oswald, W. J. (1996). Systems and Economic Analysis of Microalgae Ponds for Conversion of CO {sub 2} to Biomass. Final report. Berkeley, CA: California Univ., Dept. of Civil Engineering. doi: 10.2172/493389
Benhelal, E., Zahedi, G., Shamsaei, E., and Bahadori, A. (2013). Global strategies and potentials to curb CO2 emissions in cement industry. J. Clean. Prod. 51, 142–161. doi: 10.1016/j.jclepro.2012.10.049
Berman-Frank, I., Lundgren, P., and Falkowski, P. (2003). Nitrogen fixation and photosynthetic oxygen evolution in cyanobacteria. Res. Microbiol. 154, 157–164. doi: 10.1016/S0923-2508(03)00029-9
Bhola, V., Desikan, R., Santosh, S. K., Subburamu, K., Sanniyasi, E., and Bux, F. (2011). Effects of parameters affecting biomass yield and thermal behaviour of Chlorella vulgaris. J. Biosci. Bioeng. 111, 377–382. doi: 10.1016/j.jbiosc.2010.11.006
Bian, B., Alqahtani, M. F., Katuri, K. P., Liu, D., Bajracharya, S., Lai, Z., et al. (2018). Porous nickel hollow fiber cathodes coated with CNTs for efficient microbial electrosynthesis of acetate from CO2 using Sporomusa ovata. J. Mater. Chem. A 6, 17201–17211. doi: 10.1039/C8TA05322G
Bonacci, W., Teng, P. K., Afonso, B., Niederholtmeyer, H., Grob, P., Silver, P. A., et al. (2012). Modularity of a carbon-fixing protein organelle. Proc. Natl. Acad. Sci. U.S.A. 109, 478–483. doi: 10.1073/pnas.1108557109
Bond, G. M., Stringer, J., Brandvold, D. K., Simsek, F. A., Medina, M-G., and Egeland, G. (2001). Development of integrated system for biomimetic CO2 sequestration using the enzyme carbonic anhydrase. Energy Fuels 15, 309–316. doi: 10.1021/ef000246p
Bulgariu, D., and Bulgariu, L. (2016). Potential use of alkaline treated algae waste biomass as sustainable biosorbent for clean recovery of cadmium(II) from aqueous media: batch and column studies. J. Clean. Prod. 112, 4525–4533. doi: 10.1016/j.jclepro.2015.05.124
Carlsson, A. S., and Bowles, D. J. (2007). Micro- and Macro-algae: Utility for Industrial Applications : Outputs from the EPOBIO Project. Speen; Newbury, UK: CPL Press.
Carr, M. (2005). Energy bill boosts industrial biotechnology; sweeping new legislation looks to bioproducts and biofuels to cut peak oil-dependency. Ind. Biotechnol. 1, 142–143. doi: 10.1089/ind.2005.1.142
Chae, S. R., Hwang, E. J., and Shin, H. S. (2006). Single cell protein production of Euglena gracilis and carbon dioxide fixation in an innovative photo-bioreactor. Bioresour. Technol. 97, 322–329. doi: 10.1016/j.biortech.2005.02.037
Chang, X., Wang, T., and Gong, J. (2016). CO2 photo-reduction: insights into CO2 activation and reaction on surfaces of photocatalysts. Energy Environ. Sci. 9, 2177–2196. doi: 10.1039/C6EE00383D
Chen, J-S., and Hiu, S. F. (1986). Acetone-butanol-isopropanol production by Clostridiumbeijerinckii (synonym,clostridiumbutylicum). Biotechnol. Lett. 8, 371–376. doi: 10.1007/BF01040869
Chen, W., Zhang, C., Song, L., Sommerfeld, M., and Hu, Q. (2009). A high throughput Nile red method for quantitative measurement of neutral lipids in microalgae. J. Microbiol. Methods 77, 41–47. doi: 10.1016/j.mimet.2009.01.001
Cheng, S., Xing, D., Call, D. F., and Logan, B. E. (2009). Direct biological conversion of electrical current into methane by electromethanogenesis. Environ. Sci. Technol. 43, 3953–3958. doi: 10.1021/es803531g
Chinnasamy, S., Ramakrishnan, B., Bhatnagar, A., and Das, K. C. (2009). Biomass production potential of a wastewater alga Chlorella vulgaris ARC 1 under elevated levels of CO(2) and temperature. Int. J. Mol. Sci. 10, 518–532. doi: 10.3390/ijms10020518
Chisti, Y. (2007). Biodiesel from microalgae. Biotechnol. Adv. 25, 294–306. doi: 10.1016/j.biotechadv.2007.02.001
Chiu, S-Y., Kao, C-Y., Tsai, M-T., Ong, S-C., Chen, C-H., and Lin, C-S. (2009). Lipid accumulation and CO2 utilization of Nannochloropsis oculata in response to CO2 aeration. Bioresour. Technol. 100, 833–838. doi: 10.1016/j.biortech.2008.06.061
Christodoulou, X., Okoroafor, T., Parry, S., and Velasquez-Orta, S. B. (2017). The use of carbon dioxide in microbial electrosynthesis: advancements, sustainability and economic feasibility. J. CO2 Util. 18(Suppl. C), 390–399. doi: 10.1016/j.jcou.2017.01.027
Clarens, A. F., Resurreccion, E. P., White, M. A., and Colosi, L. M. (2010). Environmental life cycle comparison of algae to other bioenergy feedstocks. Environ. Sci. Technol. 44, 1813–1819. doi: 10.1021/es902838n
Cloot, A. (1994). Effect of light intensity variations on the rate of photosynthesis of algae: a dynamical approach. Math. Comput. Model. 19, 23–33. doi: 10.1016/0895-7177(94)90038-8
Coherent Market Insights (2017). Global Ethanol Market to Surpass US 54.63 Billion by 2026, Attributed to Increasing Production of Renewable Fuels. Available online at: https://www.coherentmarketinsights.com/press-release/global-ethanol-market-to-surpass-us-531-billion-by-2025-fuelled-by-increased-production-of-renewable-fuels-314. (accessed February 15, 2020).
Converti, A., Casazza, A. A., Ortiz, E. Y., Perego, P., and Del Borghi, M. (2009). Effect of temperature and nitrogen concentration on the growth and lipid content of Nannochloropsis oculata and Chlorella vulgaris for biodiesel production. Chem. Eng. Process. Process Intensific. 48, 1146–1151. doi: 10.1016/j.cep.2009.03.006
Cooksley, C. M., Zhang, Y., Wang, H., Redl, S., Winzer, K., and Minton, N. P. (2012). Targeted mutagenesis of the Clostridium acetobutylicum acetone–butanol–ethanol fermentation pathway. Metab. Eng. 14, 630–641. doi: 10.1016/j.ymben.2012.09.001
Csavina, J. L., Stuart, B. J., Guy Riefler, R., and Vis, M. L. (2011). Growth optimization of algae for biodiesel production. J. Appl. Microbiol. 111, 312–318. doi: 10.1111/j.1365-2672.2011.05064.x
de Morais, M. G., and Costa, J. A. V. (2007a). Biofixation of carbon dioxide by Spirulina sp. and Scenedesmus obliquus cultivated in a three-stage serial tubular photobioreactor. J. Biotechnol. 129, 439–445. doi: 10.1016/j.jbiotec.2007.01.009
de Morais, M. G., and Costa, J. A. V. (2007b). Carbon dioxide fixation by Chlorella kessleri, C. vulgaris, Scenedesmus obliquus and Spirulina sp. cultivated in flasks and vertical tubular photobioreactors. Biotechnol. Lett. 29, 1349–1352. doi: 10.1007/s10529-007-9394-6
de Morais, M. G., and Costa, J. A. V. (2007c). Isolation and selection of microalgae from coal fired thermoelectric power plant for biofixation of carbon dioxide. Energy Convers. Manage. 48, 2169–2173. doi: 10.1016/j.enconman.2006.12.011
del Pilar Anzola Rojas, M., Zaiat, M., Gonzalez, E. R., De Wever, H., and Pant, D. (2018). Effect of the electric supply interruption on a microbial electrosynthesis system converting inorganic carbon into acetate. Bioresour. Technol. 266, 203–210. doi: 10.1016/j.biortech.2018.06.074
Demirbas, A. (2010). Use of algae as biofuel sources. Energy Convers. Manage. 51, 2738–2749. doi: 10.1016/j.enconman.2010.06.010
Dexter, J., and Fu, P. (2009). Metabolic engineering of cyanobacteria for ethanol production. Energy Environ. Sci. 2, 857–864. doi: 10.1039/b811937f
Dong, T., Knoshaug, E. P., Pienkos, P. T., and Laurens, L. M. L. (2016). Lipid recovery from wet oleaginous microbial biomass for biofuel production: a critical review. Appl. Energy 177, 879–895. doi: 10.1016/j.apenergy.2016.06.002
Effendi, S. S. W., and Ng, I. S. (2019). The prospective and potential of carbonic anhydrase for carbon dioxide sequestration: a critical review. Process Biochem. 87, 55–65. doi: 10.1016/j.procbio.2019.08.018
El Gamal, A. A. (2010). Biological importance of marine algae. Saudi Pharm. J. 18, 1–25. doi: 10.1016/j.jsps.2009.12.001
ElMekawy, A., Hegab, H. M., Mohanakrishna, G., Elbaz, A. F., Bulut, M., and Pant, D. (2016). Technological advances in CO2 conversion electro-biorefinery: a step toward commercialization. Bioresour. Technol. 215, 357–370. doi: 10.1016/j.biortech.2016.03.023
Ewering, C., Heuser, F., Benölken, J. K., Brämer, C. O., and Steinbüchel, A. (2006). Metabolic engineering of strains of Ralstonia eutropha and Pseudomonas putida for biotechnological production of 2-methylcitric acid. Metab. Eng. 8, 587–602. doi: 10.1016/j.ymben.2006.05.007
Fan, L-H., Zhang, Y-T., Zhang, L., and Chen, H-L. (2008). Evaluation of a membrane-sparged helical tubular photobioreactor for carbon dioxide biofixation by Chlorella vulgaris. J. Membr. Sci. 325, 336–345. doi: 10.1016/j.memsci.2008.07.044
Fernando, I. P. S., Nah, J-W., and Jeon, Y-J. (2016). Potential anti-inflammatory natural products from marine algae. Environ. Toxicol. Pharmacol. 48, 22–30. doi: 10.1016/j.etap.2016.09.023
Ferry, M. S., Hasty, J., and Cookson, N. A. (2012). Synthetic Biology Approaches to Biofuel Production. Biofuels 3, 9–12. doi: 10.4155/bfs.11.151
Foo, S. C., Yusoff, F. M., Ismail, M., Basri, M., Yau, S. K., Khong, N. M. H., et al. (2017). Antioxidant capacities of fucoxanthin-producing algae as influenced by their carotenoid and phenolic contents. J. Biotechnol. 241, 175–183. doi: 10.1016/j.jbiotec.2016.11.026
García, V., Päkkilä, J., Ojamo, H., Muurinen, E., and Keiski, R. L. (2011). Challenges in biobutanol production: how to improve the efficiency? Renew. Sustain. Energy Rev. 15, 964–980. doi: 10.1016/j.rser.2010.11.008
Ge, X., Yang, L., Sheets, J. P., Yu, Z., and Li, Y. (2014). Biological conversion of methane to liquid fuels: status and opportunities. Biotechnol. Adv. 32, 1460–1475. doi: 10.1016/j.biotechadv.2014.09.004
Georgianna, D. R., and Mayfield, S. P. (2012). Exploiting diversity and synthetic biology for the production of algal biofuels. Nature 488, 329–335. doi: 10.1038/nature11479
Gildemyn, S., Verbeeck, K., Slabbinck, R., Andersen, S. J., Prévoteau, A., and Rabaey, K. (2015). Integrated production, extraction, and concentration of acetic acid from CO2 through microbial electrosynthesis. Environ. Sci. Technol. Lett. 2, 325–328. doi: 10.1021/acs.estlett.5b00212
Global Market Insights (2018). Ethyl Acetate Market size, Industry Analysis Report, Regional Outlook, Application Development Potential, Price Trends, Competitive Market Share and Forecast, 2019 - 2025. Available online at: https://www.gminsights.com/industry-analysis/ethyl-acetate-market (accessed February 15, 2020).
González López, C. V., Acién Fernández, F. G., Fernández Sevilla, J. M., Sánchez Fernández, J. F., Cerón García, M. C., and Molina Grima, E. (2009). Utilization of the cyanobacteria Anabaena sp. ATCC 33047 in CO2 removal processes. Bioresour. Technol. 100, 5904–5910. doi: 10.1016/j.biortech.2009.04.070
Gottumukkala, L. D., Sukumaran, R. K., Mohan, S. V., Valappil, S. K., Sarkar, O., and Pandey, A. (2015). Rice straw hydrolysate to fuel and volatile fatty acid conversion by Clostridium sporogenes BE01: bio-electrochemical analysis of the electron transport mediators involved. Green Chem. 17, 3047–3058. doi: 10.1039/C5GC00310E
Hanagata, N., Takeuchi, T., Fukuju, Y., Barnes, D. J., and Karube, I. (1992). Tolerance of microalgae to high CO2 and high temperature. Phytochemistry 31, 3345–3348. doi: 10.1016/0031-9422(92)83682-O
Hatch, M., and Slack, C. (1970). Photosynthetic CO2-fixation pathways. Annu. Rev. Plant Physiol. 21, 141–162. doi: 10.1146/annurev.pp.21.060170.001041
He, A. Y., Yin, C. Y., Xu, H., Kong, X. P., Xue, J. W., Zhu, J., et al. (2016). Enhanced butanol production in a microbial electrolysis cell by Clostridium beijerinckii IB4. Bioprocess Biosyst. Eng. 39, 245–254. doi: 10.1007/s00449-015-1508-2
Holkar, C. R., Jadhav, A. J., Pinjari, D. V., Mahamuni, N. M., and Pandit, A. B. (2016). A critical review on textile wastewater treatments: possible approaches. J. Environ. Manage. 182, 351–366. doi: 10.1016/j.jenvman.2016.07.090
Hou, X., Huang, L., Zhou, P., Tian, F., Tao, Y., and Li Puma, G. (2019). Electrosynthesis of acetate from inorganic carbon (HCO3–) with simultaneous hydrogen production and Cd(II) removal in multifunctional microbial electrosynthesis systems (MES). J. Hazard Mater. 371, 463–473. doi: 10.1016/j.jhazmat.2019.03.028
Hu, P., Chakraborty, S., Kumar, A., Woolston, B., Liu, H., Emerson, D., et al. (2016). Integrated bioprocess for conversion of gaseous substrates to liquids. Proc. Natl. Acad. Sci. U.S.A. 113, 3773–3778. doi: 10.1073/pnas.1516867113
Hudek, K., Davis, L. C., Ibbini, J., and Erickson, L. (2014). “Commercial products from algae,” in Algal Biorefineries: Volume 1: Cultivation of Cells and Products, eds R. Bajpai, A. Prokop, and M. Zappi (Dordrecht: Springer Netherlands), 275–295. doi: 10.1007/978-94-007-7494-0_11
Hunt, A. J., Sin, E. H. K., Marriott, R., and Clark, J. H. (2010). Generation, capture, and utilization of industrial carbon dioxide. ChemSusChem 3, 306–322. doi: 10.1002/cssc.200900169
Illing, L., Natelson, R., Resch, M., Rowe, I., and Babson, D. (2018). Rewiring the Carbon Economy: Engineered Carbon Reduction Listening Day Summary Report. USDOE Office of Energy Efficiency and Renewable Energy (EERE), Washington, DC. doi: 10.2172/1419624
International Energy Agency (2019). CO2 Status Report. Paris, France: IEA (International Energy Agency).
Jajesniak, P., Ali, H. E., and Wong, T. S. (2014). Carbon dioxide capture and utilization using biological systems: opportunities and challenges. J. Bioprocess. Biotech. 4:1. doi: 10.4172/2155-9821.1000155
Jiang, Y., May, H. D., Lu, L., Liang, P., Huang, X., and Ren, Z. J. (2019). Carbon dioxide and organic waste valorization by microbial electrosynthesis and electro-fermentation. Water Res. 149, 42–55. doi: 10.1016/j.watres.2018.10.092
Jiang, Y., Su, M., Zhang, Y., Zhan, G., Tao, Y., and Li, D. (2013). Bioelectrochemical systems for simultaneously production of methane and acetate from carbon dioxide at relatively high rate. Int. J. Hydrogen Energy 38, 3497–3502. doi: 10.1016/j.ijhydene.2012.12.107
Jin, H-F., Lim, B-R., and Lee, K. (2006). Influence of nitrate feeding on carbon dioxide fixation by microalgae. J. Environ. Sci. Health A Toxicol. Hazard Substain. Environ. Eng. 41, 2813–2824. doi: 10.1080/10934520600967928
Jo, B. H., Kim, I. G., Seo, J. H., Kang, D. G., and Cha, H. J. (2013). Engineered Escherichia coli with periplasmic carbonic anhydrase as a biocatalyst for CO2 sequestration. Appl. Environ. Microbiol. 79, 6697–6705. doi: 10.1128/AEM.02400-13
Kadier, A., Jain, P., Lai, B., Kalil, M. S., Kondaveeti, S., Alabbosh, K. F. S., et al. (2020). Biorefinery perspectives of microbial electrolysis cells (MECs) for hydrogen and valuable chemicals production through wastewater treatment. Biofuel Res. J. 7, 1128–1142. doi: 10.18331/BRJ2020.7.1.5
Kaewkannetra, P., Enmak, P., and Chiu, T. (2012). The effect of CO2 and salinity on the cultivation of scenedesmus obliquus for biodiesel production. Biotechnol. Bioprocess Eng. 17, 591–597. doi: 10.1007/s12257-011-0533-5
Kasting, J. F., and Siefert, J. L. (2002). Life and the evolution of earth's atmosphere. Science 296, 1066–1068. doi: 10.1126/science.1071184
Keffer, J. E., and Kleinheinz, G. T. (2002). Use of Chlorella vulgaris for CO2 mitigation in a photobioreactor. J. Ind. Microbiol. Biotechnol. 29, 275–280. doi: 10.1038/sj.jim.7000313
Keller, M. W., Schut, G. J., Lipscomb, G. L., Menon, A. L., Iwuchukwu, I. J., Leuko, T. T., et al. (2013). Exploiting microbial hyperthermophilicity to produce an industrial chemical, using hydrogen and carbon dioxide. Proc. Natl. Acad. Sci. U.S.A. 110, 5840–5845. doi: 10.1073/pnas.1222607110
Kerdudo, A., Burger, P., Merck, F., Dingas, A., Rolland, Y., Michel, T., et al. (2016). Development of a natural ingredient – natural preservative: a case study. Comptes Rendus Chimie 19, 1077–1089. doi: 10.1016/j.crci.2016.06.004
Khosravanipour Mostafazadeh, A., Drogui, P., Brar, S. K., Tyagi, R. D., Le Bihan, Y., Buelna, G., et al. (2016). Enhancement of biobutanol production by electromicrobial glucose conversion in a dual chamber fermentation cell using C. pasteurianum. Energy Convers. Manage. 130, 165–175. doi: 10.1016/j.enconman.2016.10.050
Kim, T. S., and Kim, B. H. (1988). Electron flow shift in Clostridium acetobutylicum fermentation by electrochemically introduced reducing equivalent. Biotechnol. Lett. 10, 123–128. doi: 10.1007/BF01024638
Kim, T. W., Bae, S. S., Lee, J. W., Lee, S-M., Lee, J-H., Lee, H. S., et al. (2016). A biological process effective for the conversion of CO-containing industrial waste gas to acetate. Bioresour. Technol. 211, 792–796. doi: 10.1016/j.biortech.2016.04.038
Kishimoto, M., Okakura, T., Nagashima, H., Minowa, T., Yokoyama, S-Y., and Yamaberi, K. (1994). CO2 fixation and oil production using micro-algae. J. Ferment. Bioeng. 78, 479–482. doi: 10.1016/0922-338X(94)90052-3
Kodama, M., Ikemoto, H., and Miyachi, S. (1993). A new species of highly CO2 tolerant fast growing marine microalga suitable for high-density culture. J. Mar. Biotechnol. 1, 21–25.
Kondaveeti, S., Choi, K. S., Kakarla, R., and Min, B. (2014a). Microalgae Scenedesmus obliquus as renewable biomass feedstock for electricity generation in microbial fuel cells (MFCs). Front. Environ. Sci. Eng. 8, 784–791. doi: 10.1007/s11783-013-0590-4
Kondaveeti, S., Kakarla, R., Kim, H. S., Kim, B-G., and Min, B. (2017). The performance and long-term stability of low-cost separators in single-chamber bottle-type microbial fuel cells. Environ. Technol. 39, 288–297. doi: 10.1080/09593330.2017.1299223
Kondaveeti, S., Lee, S-H., Park, H-D., and Min, B. (2014b). Bacterial communities in a bioelectrochemical denitrification system: the effects of supplemental electron acceptors. Water Res. 51, 25–36. doi: 10.1016/j.watres.2013.12.023
Kondaveeti, S., and Min, B. (2015). Bioelectrochemical reduction of volatile fatty acids in anaerobic digestion effluent for the production of biofuels. Water Res. 87, 137–144. doi: 10.1016/j.watres.2015.09.011
Kratz, W. A., and Myers, J. (1955). Nutrition and growth of several blue-green algae. Am. J. Bot. 42, 282–287. doi: 10.1002/j.1537-2197.1955.tb11120.x
Kuk, S. K., Ham, Y., Gopinath, K., Boonmongkolras, P., Lee, Y., Lee, Y. W., et al. (2019). CO2 reduction: continuous 3D titanium nitride nanoshell structure for solar-driven unbiased biocatalytic CO2 reduction. Adv. Energy Mater. 9:1970097. doi: 10.1002/aenm.201970097
Kumar, B., Brian, J. P., Atla, V., Kumari, S., Bertram, K. A., White, R. T., et al. (2016). New trends in the development of heterogeneous catalysts for electrochemical CO2 reduction. Catal. Today 270, 19–30. doi: 10.1016/j.cattod.2016.02.006
Kumar, K., Dasgupta, C. N., Nayak, B., Lindblad, P., and Das, D. (2011). Development of suitable photobioreactors for CO2 sequestration addressing global warming using green algae and cyanobacteria. Bioresour. Technol. 102, 4945–4953. doi: 10.1016/j.biortech.2011.01.054
Kuramochi, Y., Fu, Q., Kobayashi, H., Ikarashi, M., Wakayama, T., Kawaguchi, H., et al. (2013). Electromethanogenic CO2 conversion by subsurface-reservoir microorganisms. Energy Procedia 37, 7014–7020. doi: 10.1016/j.egypro.2013.06.636
Kurano, N., Ikemoto, H., Miyashita, H., Hasegawa, T., Hata, H., and Miyachi, S. (1995). Fixation and utilization of carbon dioxide by microalgal photosynthesis. Energy Convers. Manage. 36, 689–692. doi: 10.1016/0196-8904(95)00099-Y
Kusakabe, T., Tatsuke, T., Tsuruno, K., Hirokawa, Y., Atsumi, S., Liao, J. C., et al. (2013). Engineering a synthetic pathway in cyanobacteria for isopropanol production directly from carbon dioxide and light. Metab. Eng. 20, 101–108. doi: 10.1016/j.ymben.2013.09.007
LaBelle, E. V., and May, H. D. (2017). Energy efficiency and productivity enhancement of microbial electrosynthesis of acetate. Front. Microbiol. 8:756. doi: 10.3389/fmicb.2017.00756
Lambert, G. R., and Smith, G. D. (1977). Hydrogen formation by marine blue—green algae. FEBS Lett. 83, 159–162. doi: 10.1016/0014-5793(77)80664-9
Lau, N-S., Matsui, M., and Abdullah, A. A-A. (2015). Cyanobacteria: photoautotrophic microbial factories for the sustainable synthesis of industrial products. Biomed. Res. Int. 2015:754934. doi: 10.1155/2015/754934
Lawton, T. J., and Rosenzweig, A. C. (2016). Biocatalysts for methane conversion: big progress on breaking a small substrate. Curr. Opin. Chem. Biol. 35, 142–149. doi: 10.1016/j.cbpa.2016.10.001
Leang, C., Ueki, T., Nevin, K. P., and Lovley, D. R. (2012). A genetic system for Clostridium ljungdahlii: a chassis for autotrophic production of biocommodities and a model homoacetogen. Appl. Environ. Microbiol. 79, 1102–1109. doi: 10.1128/AEM.02891-12
Lee, J. C., Kim, J. H., Chang, W. S., and Pak, D. (2012). Biological conversion of CO2 to CH4 using hydrogenotrophic methanogen in a fixed bed reactor. J. Chem. Technol. Biotechnol. 87, 844–847. doi: 10.1002/jctb.3787
Lee, J-S., and Lee, J-P. (2003). Review of advances in biological CO2 mitigation technology. Biotechnol. Bioprocess Eng. 8:354. doi: 10.1007/BF02949279
Lee, W-K., Lim, Y-Y., Leow, A. T-C., Namasivayam, P., Ong Abdullah, J., and Ho, C-L. (2017). Biosynthesis of agar in red seaweeds: a review. Carbohydr. Polym. 164, 23–30. doi: 10.1016/j.carbpol.2017.01.078
Lehninger, A. L., Nelson, D. L., and Cox, M. M. (2005). Lehninger Principles of Biochemistry. New York, NY: W. H. Freeman.
Li, H., Opgenorth, P. H., Wernick, D. G., Rogers, S., Wu, T-Y., Higashide, W., et al. (2012). Integrated electromicrobial conversion of CO2 to higher alcohols. Science 335:1596. doi: 10.1126/science.1217643
Li, J., He, J., and Huang, Y. (2017). Role of alginate in antibacterial finishing of textiles. Int. J. Biol. Macromol. 94, 466–473. doi: 10.1016/j.ijbiomac.2016.10.054
Li, K., An, X., Park, K. H., Khraisheh, M., and Tang, J. (2014). A critical review of CO2 photoconversion: catalysts and reactors. Catal. Today 224, 3–12. doi: 10.1016/j.cattod.2013.12.006
Li, X., Angelidaki, I., and Zhang, Y. (2018). Salinity-gradient energy driven microbial electrosynthesis of value-added chemicals from CO2 reduction. Water Res. 142, 396–404. doi: 10.1016/j.watres.2018.06.013
Liew, F., Martin, M. E., Tappel, R. C., Heijstra, B. D., Mihalcea, C., and Köpke, M. (2016). Gas fermentation—a flexible platform for commercial scale production of low-carbon-fuels and chemicals from waste and renewable feedstocks. Front. Microbiol. 7:694. doi: 10.3389/fmicb.2016.00694
Liu, M., Pang, Y., Zhang, B., De Luna, P., Voznyy, O., Xu, J., et al. (2016). Enhanced electrocatalytic CO2 reduction via field-induced reagent concentration. Nature 537, 382–386. doi: 10.1038/nature19060
Ljungdahl, L., and Wood, H. G. (1965). Incorporation of C14 From Carbon Dioxide into Sugar Phosphates, Carboxylic Acids, and Amino Acids by Clostridium thermoaceticum. J. Bacteriol. 89, 1055–1064.
Lovley, D. R., and Nevin, K. (2018). Microbial Production of Multi-carbon Chemicals and Fuels from Water and Carbon Dioxide Using Electric Current. U.S. Patent, 9856449. Available online at: https://tinyurl.com/yahnawoc
Lowe, R. L., and LaLiberte, G. D. (2017). “Chapter 11 - benthic stream algae: distribution and structure,” in Methods in Stream Ecology, 3rd Edn, Vol. 1. (Boston, MA: Academic Press), 193–221. doi: 10.1016/B978-0-12-416558-8.00011-1
Luengo, J. M., Garcia, B., Sandoval, A., Naharro, G., and Olivera, E. A. R. (2003). Bioplastics from microorganisms. Curr. Opin. Microbiol. 6, 251–260. doi: 10.1016/S1369-5274(03)00040-7
Maeda, K., Owada, M., Kimura, N., Omata, K., and Karube, I. (1995). CO2 fixation from the flue gas on coal-fired thermal power plant by microalgae. Energy Convers. Manage. 36, 717–720. doi: 10.1016/0196-8904(95)00105-M
Mandotra, S., Kumar, P., Suseela, M., Nayaka, S., and Ramteke, P. (2016). Evaluation of fatty acid profile and biodiesel properties of microalga Scenedesmus abundans under the influence of phosphorus, pH and light intensities. Bioresour. Technol. 201, 222–229. doi: 10.1016/j.biortech.2015.11.042
Mark Ibekwe, A., Murinda, S. E., Murry, M. A., Schwartz, G., and Lundquist, T. (2017). Microbial community structures in high rate algae ponds for bioconversion of agricultural wastes from livestock industry for feed production. Sci. Total Environ. 580, 1185–1196. doi: 10.1016/j.scitotenv.2016.12.076
Market Study Report (2019). Capric Acid Market Size is Expected to Exhibit 270 million USD by 2024. Available online at: https://www.marketwatch.com/press-release/capric-acid-market-size-is-expected-to-exhibit-270-million-usd-by-2024-2019-09-05 (accessed February 15, 2020).
Markets Markets (2019). Succinic Acid Market worth $237.8 million by 2027. Available online at: https://www.marketsandmarkets.com/PressReleases/succinic-acid.asp (accessed February 15, 2020).
Marshall, C. W., LaBelle, E. V., and May, H. D. (2013). Production of fuels and chemicals from waste by microbiomes. Curr. Opin. Biotechnol. 24, 391–397. doi: 10.1016/j.copbio.2013.03.016
Martin, M. E., Richter, H., Saha, S., and Angenent, L. T. (2016). Traits of selected Clostridium strains for syngas fermentation to ethanol. Biotechnol. Bioeng. 113, 531–539. doi: 10.1002/bit.25827
Maurya, R., Paliwal, C., Ghosh, T., Pancha, I., Chokshi, K., Mitra, M., et al. (2016). Applications of de-oiled microalgal biomass towards development of sustainable biorefinery. Bioresour. Technol. 214, 787–796. doi: 10.1016/j.biortech.2016.04.115
Modestra, J. A., and Mohan, S. V. (2017). Microbial electrosynthesis of carboxylic acids through CO2 reduction with selectively enriched biocatalyst: microbial dynamics. J. CO2 Util. 20, 190–199. doi: 10.1016/j.jcou.2017.05.011
Mohanakrishna, G., Abu Reesh, I. M., Vanbroekhoven, K., and Pant, D. (2020). Microbial electrosynthesis feasibility evaluation at high bicarbonate concentrations with enriched homoacetogenic biocathode. Sci. Total Environ. 715:137003. doi: 10.1016/j.scitotenv.2020.137003
Mohanakrishna, G., Seelam, J. S., Vanbroekhoven, K., and Pant, D. (2015). An enriched electroactive homoacetogenic biocathode for the microbial electrosynthesis of acetate through carbon dioxide reduction. Faraday Discuss. 183, 445–462. doi: 10.1039/C5FD00041F
Mohanakrishna, G., Vanbroekhoven, K., and Pant, D. (2016). Imperative role of applied potential and inorganic carbon source on acetate production through microbial electrosynthesis. J. CO2 Util. 15(Suppl. C), 57–64. doi: 10.1016/j.jcou.2016.03.003
Mohd Yasin, N. H., Maeda, T., Hu, A., Yu, C-P., and Wood, T. K. (2015). CO2 sequestration by methanogens in activated sludge for methane production. Appl. Energy 142, 426–434. doi: 10.1016/j.apenergy.2014.12.069
Moreira, D., and Pires, J. C. M. (2016). Atmospheric CO2 capture by algae: negative carbon dioxide emission path. Bioresour. Technol. 215, 371–379. doi: 10.1016/j.biortech.2016.03.060
Müller, J., MacEachran, D., Burd, H., Sathitsuksanoh, N., Bi, C., Yeh, Y-C., et al. (2013). Engineering of Ralstonia eutropha H16 for autotrophic and heterotrophic production of methyl ketones. Appl. Environ. Microbiol. 79, 4433–4439. doi: 10.1128/AEM.00973-13
Murakami, M., and Ikenouchi, M. (1997). The biological CO2 fixation and utilization project by rite (2) — screening and breeding of microalgae with high capability in fixing CO2 —. Energy Convers. Manage. 38(Suppl.), S493–S497. doi: 10.1016/S0196-8904(96)00316-0
Nagarajan, S., Chou, S. K., Cao, S., Wu, C., and Zhou, Z. (2013). An updated comprehensive techno-economic analysis of algae biodiesel. Bioresour. Technol. 145, 150–156. doi: 10.1016/j.biortech.2012.11.108
Nautiyal, P., Subramanian, K. A., and Dastidar, M. G. (2016). Adsorptive removal of dye using biochar derived from residual algae after in-situ transesterification: alternate use of waste of biodiesel industry. J. Environ. Manage. 182, 187–197. doi: 10.1016/j.jenvman.2016.07.063
Negoro, M., Shioji, N., Miyamoto, K., and Micira, Y. (1991). Growth of microalgae in high CO2 gas and effects of SOX and NOX. Appl. Biochem. Biotechnol. 28, 877–886. doi: 10.1007/BF02922657
Nevin, K. P., Hensley, S. A., Franks, A. E., Summers, Z. M., Ou, J., Woodard, T. L., et al. (2011). Electrosynthesis of organic compounds from carbon dioxide is catalyzed by a diversity of acetogenic microorganisms. Appl. Environ. Microbiol. 77, 2882–2886. doi: 10.1128/AEM.02642-10
Nevin, K. P., Woodard, T. L., Franks, A. E., Summers, Z. M., and Lovley, D. R. (2010). Microbial electrosynthesis: feeding microbes electricity to convert carbon dioxide and water to multicarbon extracellular organic compounds. mBio 1:e00103–10. doi: 10.1128/mBio.00103-10
Nigam, P. S., and Singh, A. (2011). Production of liquid biofuels from renewable resources. Prog. Energy Combust. Sci. 37, 52–68. doi: 10.1016/j.pecs.2010.01.003
Overhage, J., Steinbüchel, A., and Priefert, H. (2002). Biotransformation of eugenol to ferulic acid by a recombinant strain of Ralstonia eutropha H16. Appl. Environ. Microbiol. 68, 4315–4321. doi: 10.1128/AEM.68.9.4315-4321.2002
Packer, M. (2009). Algal capture of carbon dioxide; biomass generation as a tool for greenhouse gas mitigation with reference to New Zealand energy strategy and policy. Energy Policy 37, 3428–3437. doi: 10.1016/j.enpol.2008.12.025
Patel, T. N., Park, A-H. A., and Banta, S. (2013). Periplasmic expression of carbonic anhydrase in Escherichia coli: a new biocatalyst for CO2 hydration. Biotechnol. Bioeng. 110, 1865–1873. doi: 10.1002/bit.24863
Petersen, H. I., Rosenberg, P., and Nytoft, H. P. (2008). Oxygen groups in coals and alginite-rich kerogen revisited. Int. J. Coal Geol. 74, 93–113. doi: 10.1016/j.coal.2007.11.007
Pfennig, N. (1967). Photosynthetic bacteria. Annu. Rev. Microbiol. 21, 285–324. doi: 10.1146/annurev.mi.21.100167.001441
Puig, S., Batlle-Vilanova, R. G. P., Dolors Balaguer, M., and Colprim, J. (2015). Microbial electrosynthesis of C2-C4 platform chemicals from carbon dioxide. ACS Sustain. Chem. Eng. 6, 8485–8493.
Quintana, N., Van der Kooy, F., Van de Rhee, M. D., Voshol, G. P., and Verpoorte, R. (2011). Renewable energy from cyanobacteria: energy production optimization by metabolic pathway engineering. Appl. Microbiol. Biotechnol. 91, 471–490. doi: 10.1007/s00253-011-3394-0
Rabaey, K., Angenent, L., Schroder, U., and Keller, J. (2009). Bioelectrochemical Systems. London: IWA Publishing.
Rabaey, K., and Rozendal, R. A. (2010). Microbial electrosynthesis — revisiting the electrical route for microbial production. Nat. Rev. Microbiol. 8, 706–716. doi: 10.1038/nrmicro2422
Ramanan, R., Kannan, K., Deshkar, A., Yadav, R., and Chakrabarti, T. (2010). Enhanced algal CO2 sequestration through calcite deposition by Chlorella sp. and Spirulina platensis in a mini-raceway pond. Bioresour. Technol. 101, 2616–2622. doi: 10.1016/j.biortech.2009.10.061
Rana, D., Kim, Y., Matsuura, T., and Arafat, H. A. (2011). Development of antifouling thin-film-composite membranes for seawater desalination. J. Membr. Sci. 367, 110–118. doi: 10.1016/j.memsci.2010.10.050
Rashid, N., Rehman, M. S. U., and Han, J-I. (2013). Recycling and reuse of spent microalgal biomass for sustainable biofuels. Biochem. Eng. J. 75, 101–107. doi: 10.1016/j.bej.2013.04.001
Reinecke, F., and Steinbüchel, A. (2009). Ralstonia eutropha strain H16 as model organism for PHA metabolism and for biotechnological production of technically interesting biopolymers. J. Mol. Microbiol. Biotechnol. 16, 91–108. doi: 10.1159/000142897
Rengasamy, K. R. R., Kulkarni, M. G., Papenfus, H. B., and Van Staden, J. (2016). Quantification of plant growth biostimulants, phloroglucinol and eckol, in four commercial seaweed liquid fertilizers and some by-products. Algal Res. 20, 57–60. doi: 10.1016/j.algal.2016.09.017
Richter, H., Martin, M. E., and Angenent, L. T. (2013). A two-stage continuous fermentation system for conversion of syngas into ethanol. Energies 6, 3987–4000. doi: 10.3390/en6083987
Sadhukhan, J., Lloyd, J. R., Scott, K., Premier, G. C., Yu, E. H., Curtis, T., et al. (2016). A critical review of integration analysis of microbial electrosynthesis (MES) systems with waste biorefineries for the production of biofuel and chemical from reuse of CO2. Renew. Sustain. Energy Rev. 56, 116–132. doi: 10.1016/j.rser.2015.11.015
Sakai, N., Sakamoto, Y., Kishimoto, N., Chihara, M., and Karube, I. (1995). Chlorella strains from hot springs tolerant to high temperature and high CO2. Energy Convers. Manage. 36, 693–696. doi: 10.1016/0196-8904(95)00100-R
Samarakoon, K., and Jeon, Y-J. (2012). Bio-functionalities of proteins derived from marine algae — a review. Food Res. Int. 48, 948–960. doi: 10.1016/j.foodres.2012.03.013
Sarkar, O., Agarwal, M., Naresh Kumar, A., and Venkata Mohan, S. (2015). Retrofitting hetrotrophically cultivated algae biomass as pyrolytic feedstock for biogas, bio-char and bio-oil production encompassing biorefinery. Bioresour. Technol. 178, 132–138. doi: 10.1016/j.biortech.2014.09.070
Sawitzke, J. A., Thomason, L. C., Costantino, N., Bubunenko, M., Datta, S., and Court, D. L. (2007). Recombineering: in vivo genetic engineering in E. coli, S. enterica, and Beyond. Methods Enzymol. 421, 171–199. doi: 10.1016/S0076-6879(06)21015-2
Sayre, R. (2010). Microalgae: the potential for carbon capture. Bioscience 60, 722–727. doi: 10.1525/bio.2010.60.9.9
Schenk, P. M., Thomas-Hall, S. R., Stephens, E., Marx, U. C., Mussgnug, J. H., Posten, C., et al. (2008). Second generation biofuels: high-efficiency microalgae for biodiesel production. Bioenergy Res. 1, 20–43. doi: 10.1007/s12155-008-9008-8
Scherer, P. A., Vollmer, G-R., Fakhouri, T., and Martensen, S. (2000). Development of a methanogenic process to degrade exhaustively the organic fraction of municipal “grey waste” under thermophilic and hyperthermophilic conditions. Water Sci. Technol. 41, 83–91. doi: 10.2166/wst.2000.0059
Schirmer, A., Rude, M. A., Li, X., Popova, E., and Del Cardayre, S. B. (2010). Microbial biosynthesis of alkanes. Science 329, 559–562. doi: 10.1126/science.1187936
Schlager, S., Neugebauer, H., Haberbauer, M., Hinterberger, G., and Sariciftci, N. S. (2015). Direct electrochemical addressing of immobilized alcohol dehydrogenase for the heterogeneous bioelectrocatalytic reduction of butyraldehyde to butanol. ChemCatChem 7, 967–971. doi: 10.1002/cctc.201402932
Scragg, A. H., Illman, A. M., Carden, A., and Shales, S. W. (2002). Growth of microalgae with increased calorific values in a tubular bioreactor. Biomass Bioenergy 23, 67–73. doi: 10.1016/S0961-9534(02)00028-4
Sezenna, M. L. (2011). Proteobacteria: Phylogeny, Metabolic Diversity and Ecological Effects. New York, NY: Nova Science.
Sharma, M., Aryal, N., Sarma, P. M., Vanbroekhoven, K., Lal, B., Benetton, X. D., et al. (2013a). Bioelectrocatalyzed reduction of acetic and butyric acids via direct electron transfer using a mixed culture of sulfate-reducers drives electrosynthesis of alcohols and acetone. Chem. Commun. 49, 6495–6497. doi: 10.1039/c3cc42570c
Sharma, M., Jain, P., Varanasi, J. L., Lal, B., Rodríguez, J., Lema, J. M., et al. (2013b). Enhanced performance of sulfate reducing bacteria based biocathode using stainless steel mesh on activated carbon fabric electrode. Bioresour. Technol. 150, 172–180. doi: 10.1016/j.biortech.2013.09.069
Shi, J., Jiang, Y., Jiang, Z., Wang, X., Wang, X., Zhang, S., et al. (2015). Enzymatic conversion of carbon dioxide. Chem. Soc. Rev. 44, 5981–6000. doi: 10.1039/C5CS00182J
Silva, C., Soliman, E., Cameron, G., Fabiano, L. A., Seider, W. D., Dunlop, E. H., et al. (2014). Commercial-scale biodiesel production from algae. Ind. Eng. Chem. Res. 53, 5311–5324. doi: 10.1021/ie403273b
Singh, A., Nigam, P. S., and Murphy, J. D. (2011). Mechanism and challenges in commercialisation of algal biofuels. Bioresour. Technol. 102, 26–34. doi: 10.1016/j.biortech.2010.06.057
Singh, S. P., and Singh, P. (2014). Effect of CO2 concentration on algal growth: a review. Renew. Sustain. Energy Rev. 38, 172–179. doi: 10.1016/j.rser.2014.05.043
Sorokin, C., and Krauss, R. W. (1958). The effects of light intensity on the growth rates of green algae. Plant Physiol. 33, 109–113. doi: 10.1104/pp.33.2.109
Steinbusch, K. J. J., Hamelers, H. V. M., Schaap, J. D., Kampman, C., and Buisman, C. J. N. (2010). Bioelectrochemical ethanol production through mediated acetate reduction by mixed cultures. Environ. Sci. Technol. 44, 513–517. doi: 10.1021/es902371e
Strong, P. J., Kalyuzhnaya, M., Silverman, J., and Clarke, W. P. (2016). A methanotroph-based biorefinery: potential scenarios for generating multiple products from a single fermentation. Bioresour. Technol. 215, 314–323. doi: 10.1016/j.biortech.2016.04.099
Sung, K. D., Lee, J. S., Shin, C. S., and Park, S. C. (1999). Isolation of a new highly CO2 tolerant fresh water microalga Chlorella SP. KR-1. Renew. Energy 16, 1019–1022. doi: 10.1016/S0960-1481(98)00362-0
Sydney, E. B., Sturm, W., de Carvalho, J. C., Thomaz-Soccol, V., Larroche, C., Pandey, A., et al. (2010). Potential carbon dioxide fixation by industrially important microalgae. Bioresour. Technol. 101, 5892–5896. doi: 10.1016/j.biortech.2010.02.088
Tahvonen, O., Von Storch, H., and Storch, J-S. (1994). Economic efficiency of CO2 reduction programs. Clim. Res. 4, 127–141. doi: 10.3354/cr004127
Tamagnini, P., Axelsson, R., Lindberg, P., Oxelfelt, F., Wünschiers, R., and Lindblad, P. (2002). Hydrogenases and hydrogen metabolism of cyanobacteria. Microbiol. Mol. Biol. Rev. 66, 1–20. doi: 10.1128/MMBR.66.1.1-20.2002
Tanaka, K., Miyawaki, K., Yamaguchi, A., Khosravi-Darani, K., and Matsusaki, H. (2011). Cell growth and P(3HB) accumulation from CO2 of a carbon monoxide-tolerant hydrogen-oxidizing bacterium, Ideonella sp. O-1. Appl. Microbiol. Biotechnol. 92, 1161–1169. doi: 10.1007/s00253-011-3420-2
Tang, D., Han, W., Li, P., Miao, X., and Zhong, J. (2011). CO2 biofixation and fatty acid composition of Scenedesmus obliquus and Chlorella pyrenoidosa in response to different CO2 levels. Bioresour. Technol. 102, 3071–3076. doi: 10.1016/j.biortech.2010.10.047
Tracy, B. P., Jones, S. W., Fast, A. G., Indurthi, D. C., and Papoutsakis, E. T. (2012). Clostridia: the importance of their exceptional substrate and metabolite diversity for biofuel and biorefinery applications. Curr. Opin. Biotechnol. 23, 364–381. doi: 10.1016/j.copbio.2011.10.008
Trivedi, J., Aila, M., Bangwal, D. P., Kaul, S., and Garg, M. O. (2015). Algae based biorefinery—how to make sense? Renew. Sustain. Energy Rev. 47, 295–307. doi: 10.1016/j.rser.2015.03.052
Tsuzuki, M., Ohnuma, E., Sato, N., Takaku, T., and Kawaguchi, A. (1990). Effects of CO2 concentration during growth on fatty acid composition in microalgae. Plant Physiol. 93, 851–856. doi: 10.1104/pp.93.3.851
Ueki, T., Nevin, K. P., Woodard, T. L., and Lovley, D. R. (2014). Converting carbon dioxide to butyrate with an engineered strain of Clostridium ljungdahlii. mBio 5:e01636-14. doi: 10.1128/mBio.01636-14
Van Eerten-Jansen, M. C. A. A., Heijne, A. T., Buisman, C. J. N., and Hamelers, H. V. M. (2012). Microbial electrolysis cells for production of methane from CO2: long-term performance and perspectives. Int. J. Energy Res. 36, 809–819. doi: 10.1002/er.1954
Van Eerten-Jansen, M. C. A. A., Ter Heijne, A., Grootscholten, T. I. M., Steinbusch, K. J. J., Sleutels, T. H. J. A., Hamelers, H. V. M., et al. (2013). Bioelectrochemical production of caproate and caprylate from acetate by mixed cultures. ACS Sustain. Chem. Eng. 1, 513–518. doi: 10.1021/sc300168z
Vassilev, I., Hernandez, P. A., Batlle-Vilanova, P., Freguia, S., Krömer, J. O., Keller, J., et al. (2018). Microbial electrosynthesis of isobutyric, butyric, caproic acids, and corresponding alcohols from carbon dioxide. ACS Sustain. Chem. Eng. 6, 8485–8493. doi: 10.1021/acssuschemeng.8b00739
Vassilev, I., Kracke, F., Freguia, S., Keller, J., Krömer, J. O., Ledezma, P., et al. (2019). Microbial electrosynthesis system with dual biocathode arrangement for simultaneous acetogenesis, solventogenesis and carbon chain elongation. Chem. Commun. 55, 4351–4354. doi: 10.1039/C9CC00208A
Vassilev, S. V., and Vassileva, C. G. (2016). Composition, properties and challenges of algae biomass for biofuel application: an overview. Fuel 181, 1–33. doi: 10.1016/j.fuel.2016.04.106
Venil, C. K., Zakaria, Z. A., and Ahmad, W. A. (2013). Bacterial pigments and their applications. Process Biochem. 48, 1065–1079. doi: 10.1016/j.procbio.2013.06.006
Venkata Mohan, S., Hemalatha, M., Chakraborty, D., Chatterjee, S., Ranadheer, P., and Kona, R. (2020). Algal biorefinery models with self-sustainable closed loop approach: trends and prospective for blue-bioeconomy. Bioresour. Technol. 295:122128. doi: 10.1016/j.biortech.2019.122128
Venkata Mohan, S., Modestra, J. A., Amulya, K., Butti, S. K., and Velvizhi, G. (2016). A circular bioeconomy with biobased products from CO2 sequestration. Trends Biotechnol. 34, 506–519. doi: 10.1016/j.tibtech.2016.02.012
Venkata Mohan, S., Prathima Devi, M., Mohanakrishna, G., Amarnath, N., Lenin Babu, M., and Sarma, P. N. (2011). Potential of mixed microalgae to harness biodiesel from ecological water-bodies with simultaneous treatment. Bioresour. Technol. 102, 1109–1117. doi: 10.1016/j.biortech.2010.08.103
Vigani, M., Parisi, C., Rodríguez-Cerezo, E., Barbosa, M. J., Sijtsma, L., Ploeg, M., et al. (2015). Food and feed products from micro-algae: market opportunities and challenges for the EU. Trends Food Sci. Technol. 42, 81–92. doi: 10.1016/j.tifs.2014.12.004
Villano, M., Aulenta, F., Ciucci, C., Ferri, T., Giuliano, A., and Majone, M. (2010). Bioelectrochemical reduction of CO2 to CH4 via direct and indirect extracellular electron transfer by a hydrogenophilic methanogenic culture. Bioresour. Technol. 101, 3085–3090. doi: 10.1016/j.biortech.2009.12.077
Villano, M., Scardala, S., Aulenta, F., and Majone, M. (2013). Carbon and nitrogen removal and enhanced methane production in a microbial electrolysis cell. Bioresour. Technol. 130, 366–371. doi: 10.1016/j.biortech.2012.11.080
Voss, I., and Steinbüchel, A. (2006). Application of a KDPG-aldolase gene-dependent addiction system for enhanced production of cyanophycin in Ralstonia eutropha strain H16. Metab. Eng. 8, 66–78. doi: 10.1016/j.ymben.2005.09.003
Wang, B., Albarracín-Suazo, S., Pagán-Torres, Y., and Nikolla, E. (2017). Advances in methane conversion processes. Catal. Today 285, 147–158. doi: 10.1016/j.cattod.2017.01.023
Wang, B., Wang, J., Zhang, W., and Meldrum, D. R. (2012). Application of synthetic biology in cyanobacteria and algae. Front. Microbiol. 3:344. doi: 10.3389/fmicb.2012.00344
Wang, C., Scott Buchanan, J., Kliewer, W. R., and Qian, K. (2015). Water-washing to reduce metals in oils extracted from nannochloropsis algae for potential FCC feedstock. Fuel 155, 63–67. doi: 10.1016/j.fuel.2015.03.074
Wang, H-M. D., Chen, C-C., Huynh, P., and Chang, J-S. (2015). Exploring the potential of using algae in cosmetics. Bioresour. Technol. 184, 355–362. doi: 10.1016/j.biortech.2014.12.001
Wang, Q., Dong, H., Yu, H., and Yu, H. (2015). Enhanced performance of gas diffusion electrode for electrochemical reduction of carbon dioxide to formate by adding polytetrafluoroethylene into catalyst layer. J. Power Sources 279, 1–5. doi: 10.1016/j.jpowsour.2014.12.118
Wang, W-N. (2014). Comparison of CO2 photoreduction systems: a review. Aerosol Air Qual. Res. 14, 533–549. doi: 10.4209/aaqr.2013.09.0283
Wargacki, A. J., Leonard, E., Win, M. N., Regitsky, D. D., Santos, C. N. S., Kim, P. B., et al. (2012). An engineered microbial platform for direct biofuel production from brown macroalgae. Science 335, 308–313. doi: 10.1126/science.1214547
Watanabe, Y., Ohmura, N., and Saiki, H. (1992). Isolation and determination of cultural characteristics of microalgae which functions under CO2 enriched atmosphere. Energy Convers. Manage. 33, 545–552. doi: 10.1016/0196-8904(92)90054-Z
Will, F. G. (1965). Hydrogen adsorption on platinum single crystal electrodes I. Isotherms and heats of adsorption. J. Electrochem. Soc. 112, 451–455. doi: 10.1149/1.2423567
Willey, J. M., Sherwood, L. M., and Woolverton, C. J. (2014). Prescott's Microbiology. New York, NY: McGraw-Hill Education.
Xia, A., Jacob, A., Tabassum, M. R., Herrmann, C., and Murphy, J. D. (2016). Production of hydrogen, ethanol and volatile fatty acids through co-fermentation of macro- and micro-algae. Bioresour. Technol. 205, 118–125. doi: 10.1016/j.biortech.2016.01.025
Xin, L., Hong-Ying, H., and Yu-Ping, Z. (2011). Growth and lipid accumulation properties of a freshwater microalga Scenedesmus sp. under different cultivation temperature. Bioresour. Technol. 102, 3098–3102. doi: 10.1016/j.biortech.2010.10.055
Xu, Y., Ibrahim, I. M., and Harvey, P. J. (2016). The influence of photoperiod and light intensity on the growth and photosynthesis of Dunaliella salina (chlorophyta) CCAP 19/30. Plant Physiol. Biochem. 106, 305–315. doi: 10.1016/j.plaphy.2016.05.021
Yoo, C., Jun, S-Y., Lee, J-Y., Ahn, C-Y., and Oh, H-M. (2010). Selection of microalgae for lipid production under high levels carbon dioxide. Bioresour. Technol. 101(Suppl. 1), S71–S74. doi: 10.1016/j.biortech.2009.03.030
Youn, M. H., Park, K. T., Lee, Y. H., Kang, S-P., Lee, S. M., Kim, S. S., et al. (2019). Carbon dioxide sequestration process for the cement industry. J. CO2 Util. 34, 325–334. doi: 10.1016/j.jcou.2019.07.023
Zakaria, Z., and Kamarudin, S. K. (2016). Direct conversion technologies of methane to methanol: an overview. Renew. Sustain. Energy Rev. 65, 250–261. doi: 10.1016/j.rser.2016.05.082
Zeikus, J. (1977). The biology of methanogenic bacteria. Bacteriol. Rev. 41, 514–541. doi: 10.1128/MMBR.41.2.514-541.1977
Zhang, L., Singh, R. D. S., Guo, Z., Li, J., Chen, F., et al. (2018). An artificial synthetic pathway for acetoin, 2,3-butanediol, and 2-butanol production from ethanol using cell free multi-enzyme catalysis. Green Chem. 20, 230–242. doi: 10.1039/C7GC02898A
Zhang, T., Nie, H., Bain, T. S., Lu, H., Cui, M., Snoeyenbos-West, O. L., et al. (2013). Improved cathode materials for microbial electrosynthesis. Energy Environ. Sci. 6, 217–224. doi: 10.1039/C2EE23350A
Zhang, Y., and Kendall, A. (2019). Consequential analysis of algal biofuels: benefits to ocean resources. J. Clean. Prod. 231, 35–42. doi: 10.1016/j.jclepro.2019.05.057
Zhu, L. D., Li, Z. H., and Hiltunen, E. (2016). Strategies for lipid production improvement in microalgae as a biodiesel feedstock. Biomed. Res. Int. 2016:8792548. doi: 10.1155/2016/8792548
Keywords: CO2 capture and utilization, algae, bioenergy, greenhouse gases (GHG), gas fermentation, microbial electrosynthesis (MES), CO2 reduction pathways
Citation: Kondaveeti S, Abu-Reesh IM, Mohanakrishna G, Bulut M and Pant D (2020) Advanced Routes of Biological and Bio-electrocatalytic Carbon Dioxide (CO2) Mitigation Toward Carbon Neutrality. Front. Energy Res. 8:94. doi: 10.3389/fenrg.2020.00094
Received: 24 February 2020; Accepted: 01 May 2020;
Published: 12 June 2020.
Edited by:
Michele Aresta, IC2R srl, ItalyReviewed by:
Nicolaas Vermeulen, Northwestern University, PhilippinesEduardo René Perez Gonzalez, São Paulo State University, Brazil
Copyright © 2020 Kondaveeti, Abu-Reesh, Mohanakrishna, Bulut and Pant. This is an open-access article distributed under the terms of the Creative Commons Attribution License (CC BY). The use, distribution or reproduction in other forums is permitted, provided the original author(s) and the copyright owner(s) are credited and that the original publication in this journal is cited, in accordance with accepted academic practice. No use, distribution or reproduction is permitted which does not comply with these terms.
*Correspondence: Ibrahim M. Abu-Reesh, YWJ1cmVlc2hAcXUuZWR1LnFh