- Department of Process, Energy and Environmental Technology, University of South-Eastern Norway, Porsgrunn, Norway
Hydraulic retention time (HRT) is one of the most important factors to be analyzed and optimized in continuous flow operations such as the integrated process of microbial electrosynthesis system (MES) and anaerobic digestion (AD). Highest methane production rate of 12.2 ± 0.1 mmol/L(feed)-d was obtained at 18-h HRT with reject water feed that was supplemented with acetic acid. Highest amount of COD removal of 23.4% was obtained at 18-h HRT operation with the reject water feed that was not supplemented with acetic acid. The pH of the effluent was 8.63 and 7.64 at 18-h HRT for both the feed types, respectively. This resulted in net alkalinity reduction implying conversion of bicarbonate to methane at 90% of biogas. It was also observed that the electrochemical methane production rates were higher in feeds that were not supplemented with acetic acid along with additional COD degradation via direct electro-oxidation of organics at anode.
Introduction
Microbial electrosynthesis systems (MES) have long been discussed as a tool for sustainable biochemical and biofuel production. One such application is to integrate MES with anaerobic digestion (AD) systems as a post-treatment unit for enhanced biomethane production and organic degradation (Nelabhotla and Dinamarca, 2019). One of most important aspects of a continuous flow reactors is the organic loading rate (OLR) which increases with shorter hydraulic retention time (HRT). These parameters have never been explored in the context of biomethane production via MES and integration of MES with AD.
It is important to differentiate between hydraulic retention time and solid retention time (SRT) in biochemical reactors when biofilm activity is an integral part of reactor design. HRT is defined as the average length of time that a soluble compound remains in a constructed bioreactor which in case of MES is the feed wastewater and its components. SRT on the other hand is defined as the average time the active-sludge solids are in the system which mainly implies the micro-organisms that carry out the metabolic activities in the bioreactor. In a continuous flow stirred tank reactor (CSTR) the SRT is equal to HRT whereas the presence of a surface within the bioreactor that is able to support biofilm growth the value of SRT can dramatically increase compared to HRT (Metcalf Eddy et al., 2014).
HRT influences the flow of nutrients, products and unreacted chemicals through reactor, whereas the SRT influences the rate of reactants converting into products. The biocathodes present in the MES can maintain a constant and indefinite SRT. Therefore, there is a need to optimize the HRT/OLR to exploit optimal and favorable productivity. HRT is a major factor to evaluate not only technical viability but most importantly economic viability. HRT can also influence biofilm thickness and biofilm efficiency in case of MES when strategized together with reactor design. The feed flow pattern and shear force exerted on the biofilm surface can impact biofilm structure and activity (Chang et al., 1991).
Previous articles have discussed other electrode material selection, parameters optimization, biocathode adaptation using control, and mass balance experiments and suggested a novel method to integrate MES with existing AD plants. In this article, two scenarios for optimizing HRT in MES are studied: (a) feed simulating food waste reject water (high acetic acid in feed) and (b) reject water from wastewater treatment plant (low acetic acid in feed). This study shows how HRT influences the two main pathways of methane production in MES i.e., the electrochemical and the acetoclastic pathways.
Materials and Methods
Reactor and Feed Preparation
The experiments were performed in a 135 mL electrochemical reactor designed according to materials and methods described in Nelabhotla et al. (2019). The cathodic surface area was 8 cm2 in the 135 mL reactor that gives an electrode surface area to reactor volume ratio of 6 m2/m3. The current density measured during the experiment was at an average 2 A/m2. The CSTR was operated in various conditions at 1-day hydraulic retention time (HRT) prior to the current study and discussed in previous publications (Nelabhotla and Dinamarca, 2019; Nelabhotla et al., 2019). The same reactor setup (Figure 1) was duplicated and were used to carry out HRT experiments with two different feed streams. The feed was prepared using reject water from AD treated sewage sludge and was obtained from Knarrdalstrand wastewater treatment plant, Porsgrunn, Norway. The reject water has a total solid concentration of 4,250 mg/L and volatile suspended solid concentration of 2,640 mg/L.
The first MES, R1 was fed with reject water containing 17 mM acetic acid and 85 mM sodium bicarbonate. The second reactor setup, R2 was fed with reject water without any added acetic acid but was supplemented with 85 mM bicarbonate. 1M HCl was used to adjust the pH of the feed to 7.0. Both R1 and R2 were adapted to the electrochemical operation using the respective feed conditions for 2 months at 24-h HRT before recording data. All the other conditions such as temperature 35°C, cathode potential −0.65 V vs. Standard Hydrogen Electrode (SHE) was kept same for both R1 and R2. The hydraulic retention time was changed in steps of 24, 18, 12, 6, 3, 2, and 1 h as soon as 8–10 samples were obtained during each experimental period. The samples were collected at intervals of at least their respective hydraulic retention time.
Analytical Methods and Calculations
All the analytical methods used to measure chemical oxygen demand (COD), cathode potential, current, biogas composition including the calculations for several parameters have been previously described in Nelabhotla et al. (2019). Additionally, the below mentioned parameters were also calculated:
Where, Methaneelectrochem is the amount methane calculated by converting 8 electrons transferred to 1 mole of methane.
24,450 mL = standard volume of a gas at room temperature; eff = effluent; ne is the amount electrons consumed; F is the Faradaic constant 96,845 C/mol e−.
Results and Discussion
HRT Experiments With Acetic Acid in Feed
Methane production in Figure 2A is measured in terms of methane produced per day and per liter of reactor volume (MPR). The HRT operations of 6 and 3 h produce methane at the highest rate of 40.3 ± 0.4 and 40.2 ± 1.7 (mmol/L(reactor)-d) respectively. This is equivalent to a productivity of ~1.0 L/L(reactor)-d. However, it is important to note that the methane production rate [MPR(feed)] in terms of feed reduces by 50% (10.1–5.0 mmol/L(feed)-d: Table 1, Supplementary Table 1) when the feed rate is doubled from 6- to 3-h HRT. Optimum methane production is obtained at 18-h HRT with highest feed based MPR(feed) of about 12.2 ± 0.1 mmol/L(feed)-d at 90% methane concentration (Figures 2A,B). Other characteristics obtained at 18-h HRT operation that suggest optimum performance is the amount of COD consumed that is ~40.6% of the fed COD (Figure 2C). Other feed rate operations (at 24-, 12-, and 6-h HRT) show COD consumption of (38.7, 41.7, and 36.5%) of the fed quantity. Considering ~36–40% of fed COD is equivalent to the amount of supplemented acetic acid, it can be said that the lower HRT operations viz., 3-, 2-, and 1- h (25.6, 18.6, and 8.6% of COD consumed) are limited to produce methane from the available acetic acid. On the other hand, the higher HRT operations can consume maximum of the available acetic acid and produce methane at heterotrophic efficiencies of more than 90%. The 12-h HRT operation shows consumption of about 41.7% of fed COD which is marginally more than the COD equivalent of supplemented acetic acid. This suggests that electrochemical treatment of reject water could degrade COD that was not acetic acid and that was not digested in the AD reactor.
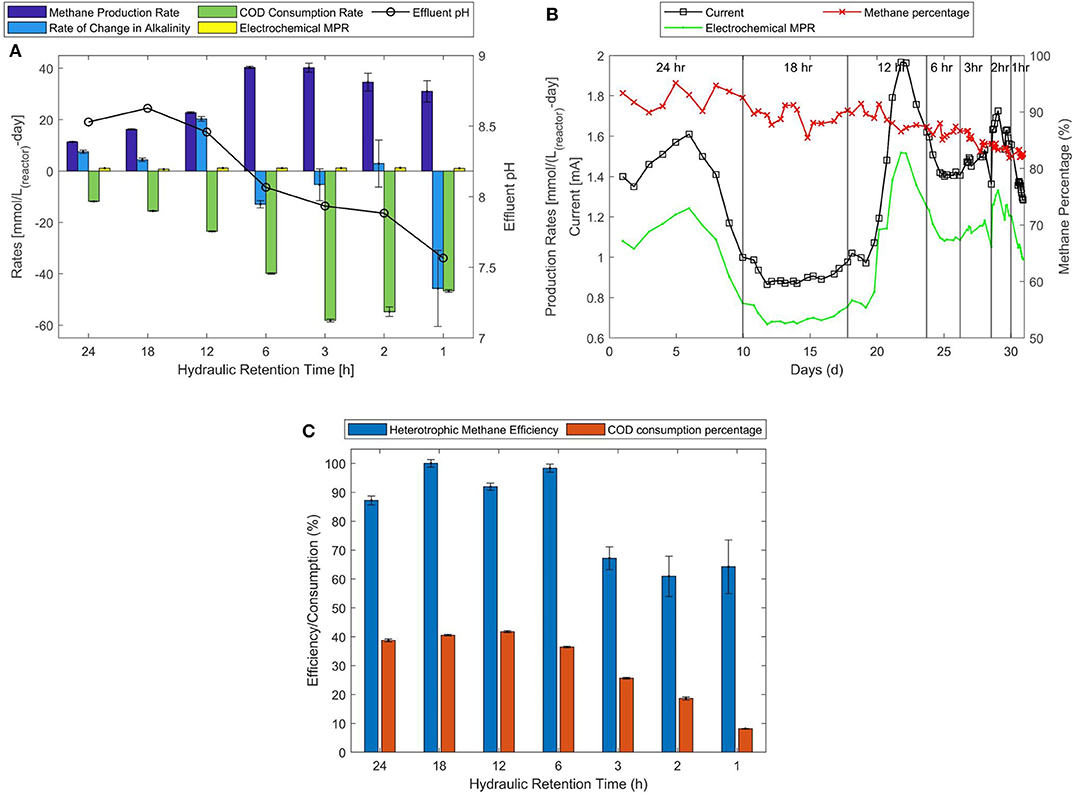
Figure 2. HRT experiments conducted with feed containing supplemented acetic acid. (A) Overall and electrochemical methane production rates, methane percentage in the biogas, and current measured. (B) Average overall and electrochemical methane production rates, rates of changes in COD and alkalinity. (C) Amount COD consumed and the heterotrophic efficiency to produce methane.
One of the primary reasons behind the HRT optimization experiments is the effect on effluent pH and on the concentration of alkalinity. It is clearly observed in Figure 2A that as HRT is decreased the pH decreases gradually. Most importantly, it is observed that when pH is close to and below 8.0 the concentration of alkalinity in the reactor/effluent is negatively affected. This implies that a lower pH in the reactor positively affects bicarbonate consumption. The negative signs in Figure 2A and represents “consumption” and positive sign represents “production,” the same applied to Figure 3A that is referred in the following section. It was not possible to obtain 100% COD to methane conversion efficiencies for many HRT operations except 18 and 6-h, which implies incomplete conversion of degraded COD to methane at other HRT operations.
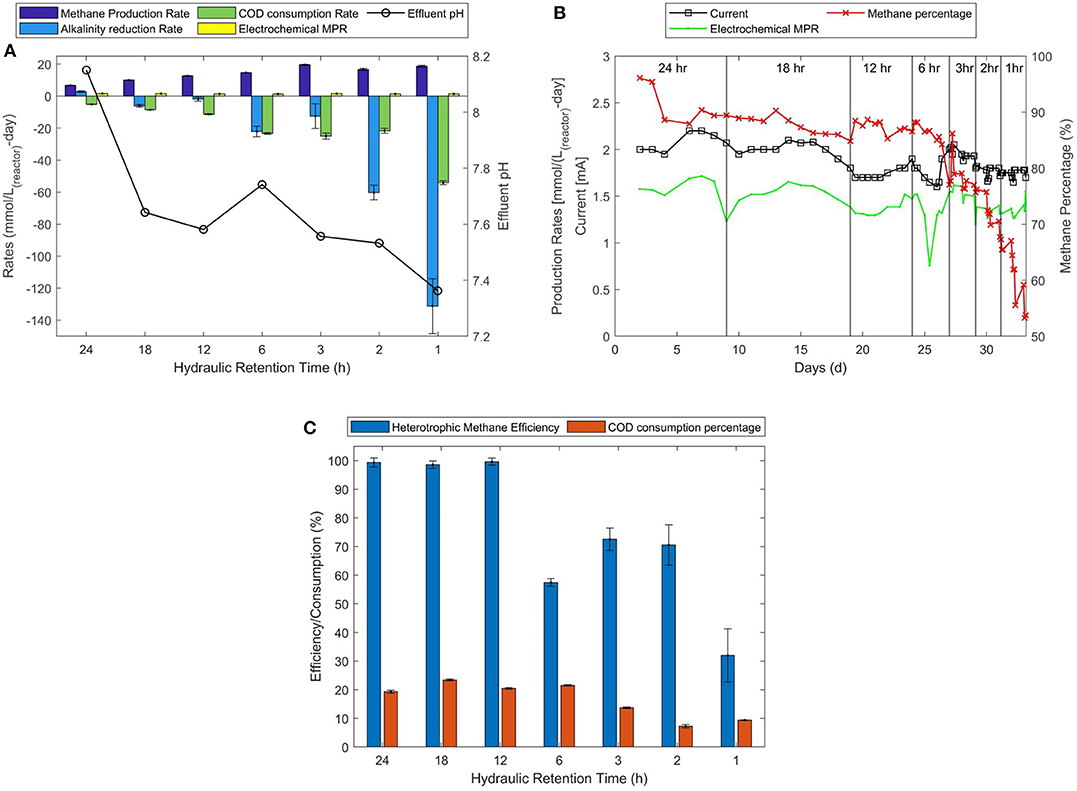
Figure 3. HRT experiments conducted with feed containing no acetic acid. (A) Overall and electrochemical methane production rates, methane percentage in the biogas and current measured. (B) Average overall and electrochemical methane production rates, rates of changes in COD and alkalinity. (C) Amount COD consumed and the heterotrophic efficiency to produce methane.
HRT Experiments Without Acetic Acid in Feed
The MPR depicted in Figure 3A shows that highest rate of methane production of about 19.6 ± 0.2 mmol/L(reactor)-d occurs at 3-h HRT operation. However, at 3-h HRT, MPR(feed) is as low as 2.5 mmol/L(feed)-d. Twelve- and six-hours HRT operations show MPRs of about 12.6 ± 0.2 and 14.7 ± 0.1 mmol/L(reactor)-d, respectively but the MPR(feed) of about 6.3 ± 0.1 and 3.7 ± 0.0 mmol/L(feed)-d were obtained, respectively. However, the methane percentages obtained are 90 and 87% for 12 and 6-h HRT operations, respectively (Figure 3B). Therefore, it can be said that the 18-h HRT operation that produces highest feed based MPR of about 7.4 ± 0.1 mmol/L(feed)-d at methane concentration of 90% shows optimum HRT for MES operation (Figures 3A,B). Further observing the amount of COD consumed i.e., 23.4% of fed COD shows highest COD degradation at 18-h HRT operation (Figure 3C). It is important to note that the feed in these experiments was not supplemented with additional acetic acid, which implies that a large portion of 23.4% of COD consumed belongs to undigested COD from the AD. It can be speculated that this non-acetic COD was degraded via anodic oxidation of organics. It can also be observed that a mass balance is achieved between COD and electrons consumed with total methane production at 18-h HRT operation. This implies that the additional degraded COD was also converted to methane. However, this was not the case at lower HRTs where the flow rate was too high to convert degraded COD to methane. The multiple pathways involved in COD degradation and methane production is explained in the following section.
The pH of effluent in these experiments reduced below 8.00 at 18-h and all lower HRT operations. At 6-h HRT operation the effluent pH was around 7.74 and the alkalinity was reduced at a rate of −22.1 ± 3.3 mmol/L(reactor)-d implying high bicarbonate conversion to methane and CO2. Even higher reductions in alkalinity were observed at lower HRT operations but resulted in unreacted CO2 gas and lower methane percentages in biogas (Figure 3B; 12 h = 87%, 6 h = 85%, 3 h = 80%, 2 h = 70%, and 1 h = 60%). While, higher methane production rates imply that bicarbonate was converted to methane, the lower COD to methane conversion efficiencies imply that anodic oxidation of organics lead to release of unreacted CO2 gas and is explained in the section Electro-Oxidation of Organics. It is speculated that the high flow rates at lower HRT operations could damage the cathodic biofilm resulting in decreased CO2 reduction rates. This can be avoided by adapting the biofilms for higher flow rates and by designing a reactor with high cathodic surface area to reactor volume ratio.
Electro-Oxidation of Organics
Typically, two pathways are described for anodic oxidation of organics: (a) direct and (b) indirect. The direct anodic oxidation depends on direct contact between the organics and electrode surface and the final product released is CO2 and protons (Ghimire et al., 2019). The indirect organic anodic-oxidation is possible in the presence of large concentrations of chloride ions (>3 g/L) (Chen, 2004). Both the methods for anodic oxidation are possible in the MES experiments discussed in the current article. A recent publication states that direct oxidation of COD is characterized by the generation of hydroxide radical (OH*) that is capable destroying wide range of pollutants (Ghanim and Hamza, 2018). The authors demonstrated 86–87% of COD removal with two different electrode materials at high temperatures of 50–55°C. The current article demonstrates 23.5% COD removal at 18-h HRT combined with methane percentage of 90% albeit at low production rates of 10.0 mmol/L(reactor)-d.
Higher feed flow rates during the lower HRT might wash away weaker biofilm networks on the anode that bring direct contact between the feed and the electrode allowing direct electro-oxidation. Since, the pH of the feed in R2 was adjusted using diluted 1M HCl solution, indirect electro-oxidation is also a possibility. These pathways have not been quantified in this study. However, the results show increase in CO2 percentage (Figure 3A; decrease in methane percentage from 87 to 52%) in the biogas against decreasing HRT suggests the dominance of direct electro-oxidation of organics in MES. Although HRT does not directly improve methane production, it is revealed that HRT can affect COD degradation, which can also be beneficial in terms of lower discharge concentrations.
A study conducted by Sangeetha et al. (2017) discusses the influence of HRT on an integrated ME-AD system. It reports COD removal rates of about 92% at 36 h HRT from the whole reactor compared to 20-25% COD removal in the current study from only MES reactor placed post-AD. Furthermore, the MPRs achieved in the Sangeetha et al., study report 304.5 mLCH4/Lreactor/day which is comparable to the MPR from MES operation of feeds F1 and F2 (396.1 and 244.5 mLCH4/Lreactor/day equivalent to 16.2 and 10 mmol/L(reactor)-d, respectively at 18 h HRT) in the current study. Some studies on MFCs have reflected higher coulombic efficiencies achieved in relation to oxidation of simpler substrates (Thygesen et al., 2009; Velasquez-Orta et al., 2011). On the other hand, oxidation of complex organics at anode reduce the overall coulombic efficiencies. This hypothesis also can be used to deduce anodic oxidation of highly complex residual COD as the current production obtained in the current study (2 mA with feed F2) are lower than current densities in Sangeetha et al. (2017) ~10 mA.
It is important to consider that the CO2/ required for electrochemical methane production can be obtained from four sources. (1) Heterotrophic conversion of residual acetic acid to methane and CO2/ (2) CO2/ dissolved in the feed (3) CO2/ produced via electro-oxidation of COD and (4) CO2 supplied as biogas for upgrading. The third pathway although results in COD consumption for methane production, cannot be accounted for heterotrophic methane production pathway. The COD degradation in this case is as a result of electrochemical activity and thus contributes to electrochemical methane production pathway. This residual COD degradation at anode would also account for proton source required for electrochemical activity. Thus, the process avoids splitting of water that produces oxygen along with protons which would hinder methanogenic activity.
Conclusion
The article studies the impact of hydraulic retention time (HRT) on MES operation as a post-AD treatment integration with anaerobic digestion systems. It can be concluded that HRT can be used as a tool to affect the final outcome of an integrated AD-MES system. An HRT of 18-h operation offers optimized methane production rates [12.2 ± 0.1, 7.4 ± 0.1 mmol/L(feed)-d] and COD reductions (40.6 and 23.4%) with both feed types that mimic reject water from food waste and wastewater treatments, respectively. pH of the effluent was 8.63 and 7.64 for feed with and without acetic acid, implying low reductions in alkalinity, which in turn could be due to conversion of autotrophic CO2 to bicarbonate. HRTs lower than 6 h decrease in both COD removal and methane production efficiencies. The MES is able to degrade an additional 20–25% of COD when there is no freely available acetic acid in the feed at HRTs maintained above 3-h. It was identified that the additional COD degradation was possible via direct electro-oxidation of organics at anode and contributed indirectly to electrochemical methane production. It is also to be noted that placement of cathode with respect to the inlet feed flow will influence methane/biogas generation. This is due to difference in electron transfer via diffusion with change in flow profile across cathode. Although, conclusive evidence could not be drawn in these set of experiments; still, future experimental studies can also focus on this aspect of reactor configuration and electrode design.
Data Availability Statement
All datasets generated for this study are included in the article/Supplementary Material.
Author Contributions
AN and CD: conceptualization, methodology, validation, resources, writing—review, and editing. AN, MK, and NC: formal analysis and investigation. MK and NC: data curation. AN: writing—original draft preparation and visualization. CD: supervision, project administration, and funding acquisition.
Funding
The research carried out in this article was funded by Norwegian Ministry of Education and Research through the University of South-Eastern Norway Ph.D. Programme.
Conflict of Interest
The authors declare that the research was conducted in the absence of any commercial or financial relationships that could be construed as a potential conflict of interest.
Acknowledgments
The acknowledge the Norwegian Ministry of Education and Research for funding the Ph.D. program in Process, Energy and Automation Engineering at University of South-Eastern Norway.
Supplementary Material
The Supplementary Material for this article can be found online at: https://www.frontiersin.org/articles/10.3389/fenrg.2020.00087/full#supplementary-material
Table S1. Experimental and calculated dataset.
References
Chang, H. T., Rittmann, B. E., Amar, D., Heim, R., Ehlinger, O., and Lesty, Y. (1991). Biofilm detachment mechanisms in a liquid-fluidized bed. Biotechnol. Bioeng. 38, 499–506. doi: 10.1002/bit.260380508
Chen, G. (2004). Electrochemical technologies in wastewater treatment. Sep. Purif. Technol. 38, 11–41. doi: 10.1016/j.seppur.2003.10.006
Ghanim, A. N., and Hamza, A. S. (2018). Evaluation of direct anodic oxidation process for the treatment of petroleum refinery wastewater. J. Environ. Eng. 144, 4018–4047. doi: 10.1061/(ASCE)EE.1943-7870.0001389
Ghimire, U., Jang, M., Jung, S. P., Park, D., Park, S. J., Yu, H., et al. (2019). Electrochemical removal of ammonium nitrogen and cod of domestic wastewater using platinum coated titanium as an anode electrode. Energies 12:883. doi: 10.3390/en12050883
Metcalf Eddy, Inc., Tchobanoglous, G., Stensel, H., Tsuchihashi, R., and Burton, F. (2014). Wastewater Engineering: Treatment and Resource Recovery, Vol. 2. Boston, MA: McGraw-Hill.
Nelabhotla, A. B. T., Bakke, R., and Dinamarca, C. (2019). Performance analysis of biocathode in bioelectrochemical CO2 reduction. Catalysts 9:683. doi: 10.3390/catal9080683
Nelabhotla, A. B. T., and Dinamarca, C. (2019). Bioelectrochemical CO2 reduction to methane: MES integration in biogas production processes. Appl. Sci. 9:1056. doi: 10.3390/app9061056
Sangeetha, T., Guo, Z., Liu, W., Gao, L., Wang, L., Cui, M., et al. (2017). Energy recovery evaluation in an up flow microbial electrolysis coupled anaerobic digestion (ME-AD) reactor: role of electrode positions and hydraulic retention times. Appl. Energy 206, 1214–1224. doi: 10.1016/j.apenergy.2017.10.026
Thygesen, A., Poulsen, F. W., Min, B., Angelidaki, I., and Thomsen, A. B. (2009). The effect of different substrates and humic acid on power generation in microbial fuel cell operation. Bioresour. Technol. 100, 1186–1191. doi: 10.1016/j.biortech.2008.07.067
Keywords: hydraulic retention time, microbial electrosynthesis system, biogas, CO2 reduction, methane
Citation: Nelabhotla ABT, Khoshbakhtian M, Chopra N and Dinamarca C (2020) Effect of Hydraulic Retention Time on MES Operation for Biomethane Production. Front. Energy Res. 8:87. doi: 10.3389/fenrg.2020.00087
Received: 24 February 2020; Accepted: 27 April 2020;
Published: 27 May 2020.
Edited by:
Ao Xia, Chongqing University, ChinaReviewed by:
Sureewan Sittijunda, Mahidol University, ThailandPensri Plangklang, Khon Kaen University, Thailand
Yang Yang, Northwestern Polytechnical University, China
Copyright © 2020 Nelabhotla, Khoshbakhtian, Chopra and Dinamarca. This is an open-access article distributed under the terms of the Creative Commons Attribution License (CC BY). The use, distribution or reproduction in other forums is permitted, provided the original author(s) and the copyright owner(s) are credited and that the original publication in this journal is cited, in accordance with accepted academic practice. No use, distribution or reproduction is permitted which does not comply with these terms.
*Correspondence: Carlos Dinamarca, Y2FybG9zLmRpbmFtYXJjYSYjeDAwMDQwO3Vzbi5ubw==