- 1IHE Delft Institute for Water Education, Delft, Netherlands
- 2Department of Civil, Environmental, Architectural Engineering and Mathematics, University of Brescia, Brescia, Italy
- 3Department of Chemistry, Mekelle University, Mekelle, Ethiopia
- 4Université de Paris, Institut de Physique du Globe de Paris, CNRS, UMR 7154, Paris, France
The main aim of this study was to evaluate the biomethane potential (BMP) of fruit waste by incorporating additives such as sewage sludge biochar at different inoculum-to-substrate ratios (ISRs) of 2, 1.5, and 1 (wt./wt.). The results showed an improvement in the maximum methane production by 13, 20, and 27% upon the addition of sewage sludge biochar produced at 350°C for ISR of 2, 1.5, and 1 (wt./wt.), respectively, and an increase 12, 18, and 22% for the added biochar produced at 550°C for ISR of 2, 1.5, and 1 (wt./wt.), respectively, compared to the BMP tests performed without the addition of biochar (i.e., the control). Biochar addition reduced volatile fatty acid (VFA) formation during the digestion of fruit waste as compared to the control reactor, which is a strong indication that the metabolic process of the system was streamlined to methanogenesis. The maximum methane yield for the tested fruit substrates was 285.7 mL CH4/g in the presence of biochar prepared at a low temperature (350°C). This value is higher than one obtained with same amount of biochar prepared at a higher temperature (550°C). The results from this study showed that the effectiveness of the anaerobic digestion process depends on the ISR, biochar dose, and the pyrolysis temperature used for the production of the sewage sludge digestate biochar.
Highlights
– Addition of biochar to an anaerobic fruit waste digestion process was evaluated.
– Addition of biochar increased the CH4 production rate and yield.
– Biochar shortened the lag phases of CH4 production.
– Biochar enhanced volatile fatty acid degradation during the CH4 production phase.
Introduction
The amount of municipal solid waste increases with economic development as well as population growth. Food waste (FW) is one of the municipal solid waste fractions, which adds pressure on the existing solid waste treatment facilities as well as on the waste management process (collection, treatment, landfilling), which in turn may potentially deteriorate the environment due to landfill leachates and greenhouse gases emissions. The unrestrained discharge of large amounts of FW may become the cause of severe environmental pollution especially in developing countries where solid waste management procedures are poorly implemented. Also, in developed countries such as the Netherlands (i.e., a relatively small country with a high population density), FW has become a challenging problem in terms of solid waste management (Chen et al., 2008; Zhang et al., 2014). Dutch people throw away an average of 135 kg of FW per person per year, which results in a total amount of nearly 2 billion kg of FW per total population per year (NOS Annual Report, 2016). The environmental impact (contribution to global warming and climate change) of FW is estimated to be 20–30% worldwide, whereas for the Netherlands it is >50% (NOS Annual Report, 2016). Therefore, one of the great challenges for governmental bodies is to reduce FW production by 20%, whereas the United Nations sustainable development goals set an objective of 50% reduction of FW production by 2030 (Milios and Reichel, 2013; Ariunbaatar et al., 2017). The traditional approaches for FW treatment are mainly landfilling and incineration, which are environmentally unfriendly and energy-intensive methods (Tiwary et al., 2015). Therefore, an effective and environmentally friendly approach is needed for FW treatment where resource recovery strategies are included.
Anaerobic digestion (AD) processes are employed for bioenergy production from organic waste streams including solid organic waste and high-strength wastewater. The application of AD for FW valorization has become an intensive field of research since the organic contents in FW are well-suited for anaerobic microorganism growth while the environmental concerns are tackled (Montecchio et al., 2017). During the AD process, FW is biologically degraded and converted into clean biogas/biomethane (Zhang and Jahng, 2012). Food waste contains different organic and inorganic materials. The critical parameters for optimal operation of AD on FW include the supply of well-balanced substrate composition (with respect to bioaccessibility and biodegradability, C/N (Carbon/nitrogen) ratio, and the supply of bioavailable trace elements), as well as the maintenance of stable parameters including pH, temperature, organic loading rate, the reactor type, and other related characteristics (Hagos et al., 2017). Among the FWs, common fruits and vegetables seem to have a composition suitable for AD, as investigated by Ji et al. (2017). However, properties such as TS, pH, volatile solid (VS), C/N ratio, and moisture content of such type of waste may pose challenges in the effective implementation of AD. This type of waste has >80% moisture content, ~10% total solid (TS), and ~6–18% VS content. Moreover, it has a pH value of 4.0 and a C/N ratio of 20 (Ji et al., 2017). The organic components for such wastes typically consist of 75% fructose, 5% cellulose, and 1% lignin (Ji et al., 2017), which is different from other FW types as reported by Mata-Alvarez et al. (2014). Because of the low content of cellulose, the substrate can easily be hydrolyzed and leads to acidic conditions in digesters, which results in the inhibition of the methanogenic activity and consequently to low biogas/biomethane production and system failure. To address these issues, the codigestion with other materials and/or the supply of additives such as biochar were reported (Ji et al., 2017). Biochar is a carbon-rich and porous solid produced during the pyrolysis of biomass (such as wood materials, crop residues, dairy manure, wastewater sludge, etc.), that is, the thermal degradation of biomass under inert atmosphere between 300 and 700°C. Depending on the heating rate, pyrolysis can be slow, fast, and flash with a heating rate (Yuan et al., 2011; Lehmann and Joseph, 2015).
The use of biochar in the AD process has recently received increased attention as it could enhance the recovery rate of the process during substrate-induced inhibition and decrease the loss of nutrients in the whole process (Sunyoto et al., 2016). It potentially improves the operation of monosubstrate AD. It also increases the availability of nutrients when the digestate is applied to the land, thereby minimizing the impact of pollutants and decreasing nutrient leaching to the environment (Fagbohungbe et al., 2017). By counteracting the process inhibitors, enhancing the quality of the digestate through the retention of nutrients, and improving the system's buffering capacity, biochar addition may provide another potential for AD processes operated on FW. Another advantage of biochar in AD is the improvement of the surface area for the colonization of the methanogenic microorganisms. Indeed, this would result in a decrease in the microbial lag phase (Fagbohungbe et al., 2016, 2017). Biochar added in the AD can also optimize and balance the trace minerals, micronutrients and macronutrients, buffering agents, compounds able to mitigate ammonia inhibition, and substances with high biomass immobilization capacity; remove the toxic materials; and enhance the degradation rate of dissolved organics and VFAs (Cai et al., 2016). Moreover, the direct addition of biochar would enhance significantly the production rate of H2, which favors the hydrogenotrophic methanogenic route of the AD process (Sunyoto et al., 2016). This is of high interest because the hydrogenotrophic methanogenic route is exothermic, thermodynamically more favorable, and a more stable process compared to the acetoclastic route. The hydrogenotrophic methanogenesis is the most common route of hydrogen (H2) in the anaerobic process. It has a high rate of growth, and the microorganisms are less sensitive to microbes (Wise et al., 1978; Luo and Angelidaki, 2012; Huang et al., 2015; Xu et al., 2015).
Recently, efforts have been made to produce biochar from sewage sludge. According to Agrafioti et al. (2013), the production of biochar from sewage sludge using pyrolytic process is a promising technique for waste management because it can be used for the remediation of contaminated soils, as well as to increase the productivity of the crops. In addition, the sludge from wastewater treatment plants can also serve as a source of nutrients (N, P), organic matter, and other micronutrients that can directly affect the properties of soil–plant interaction (Pathak et al., 2009). On the contrary, Lester et al. (1983) reported that sludge-derived biochar can have heavy metals such as copper, chromium, nickel, lead, and other metals, and their presence can restrict its application in soil amendment for crop productivity. Thus, it has been argued in the literatures that, in order to apply sewage sludge biochar for soil remediation, it requires more investigation about its environmental impact such as its mobility, solubility, and fate of the heavy metals in soils. Several authors have focused their research on sludge-derived biochar for soil application by performing vigorous laboratory tests to understand the leaching of heavy metals, soil–metal–biochar interactions, and their binding patterns. In some reports, it has been shown that the heavy metals present in biochar derived from sewage sludge are rather stable and immobile, and the leaching property of heavy metals to soil depends primarily on the pyrolysis temperature for the conversion of sewage sludge to biochar (Kistler et al., 1987; Hwang et al., 2007; He et al., 2010).
Hence, the main objective of this study was to evaluate the effectiveness of sewage sludge biochar prepared during slow pyrolysis at 350 and 550°C and its optimal dosage, as well as the effect of the inoculum-to-substrate ratio (ISR) on the AD of fruit wastes to enhance biogas production.
Materials and Methods
Substrates
Four different fruit waste substrates were used in this study, namely, mango, banana, tomato, and papaya at a ratio of 50, 25, 15, and 10 weight%, respectively. In order to choose the right composition, screening test for the feedstock was done. The results of the screening test are reported in the Supplementary Information. The fruit solid substrates were obtained from a fruit wholesale market in Delft (the Netherlands), and the sewage sludge digestate was obtained from an urban wastewater treatment plant in France. Biochar from sewage sludge digestate was industrially pyrolyzed at 350 or 550°C, for 15 min using the Biogreen® Technology [ETIA Ecotechnologies (Compiégne, France); Wongrod et al., 2018]. The physicochemical properties of the crushed biochar and the substrates, such as their TS concentration, pH, VSs concentration, and chemical oxygen demand (COD), were determined according to standard methods. The substrate was stored at −20°C before use.
The activated sludge digestate inoculum for this study was obtained from a wastewater treatment plant (Harnaschpolder, Delft, The Netherlands) operating at mesophilic temperature (38 ± 1°C). The inoculum was prepared for the experiments by conditioning at 25°C for 2 days for stabilization. The activated sludge digestate characteristics are shown in Table 1.
Experimental Design and Setup
The VS of the inoculum and the COD of the substrate were first determined. In all experiments, the volume of sludge added to the bottles was determined from the concentration of VS. A total volume of 400 mL in 650 mL digester bottles was used to prevent problems associated with foaming. The ISRs tested in this study were 2, 1.5, and 1 (based on the VS of the inoculum and COD values; Cai et al., 2016). Equations (1) and (2) were applied to calculate the volumes of inoculum and substrate required for the different tests (Table 2).
where ISR has a value of 2 or 1.5 or 1.
The experimental procedure was conducted according to the biochemical methane potential equipment (AMPTS II; Bioprocess Control AB, Lund, Sweden). The total volume was 650 mL with a working volume of 400 mL. All the reactors were placed in a thermostatic water bath maintained at 37 ± 0.5°C, at an agitation speed of 100 rpm and operated for ~50 h until gas production remained stable. The overhead space (250 mL) was flushed with 99.9% N2 to be prepared for AD. Thirty-two tests were performed and in triplicate bottles, according to the experimental design reported in Table 2. To evaluate the effect of biochar addition at various weight ratios with FW, treatment groups A, B, C, D, E, and F were prepared; 0, 0.5, 1, 1.5, and 2 g of biochars were added to the 400 mL working volume of the reactor, respectively. The initial pH solution was adjusted to be ~7.0 using 2 M sodium bicarbonate solutions as required in all biomethane potential (BMP) tests (Cai et al., 2016).
Analytical Methods
The TSs and VSs were measured according to the standard methods (APHA et al., 2012). The COD (mg/L) was determined using closed reflux, colorimetric method. The concentration of methane was determined using gas chromatography (GC) (SP-6890 gas chromatograph, Markham, ON, Canada), and the concentration of VFA was determined using a GC (Varian 430-GC, 25 m wax58FFAB column and FID detector). Total ammonia nitrogen -N of the sample was measured following the acid digestion method using an ultraviolet spectrophotometer (UV-vis lambda 365, Waltham, MA, USA; PerkinElmer, 2016). Nutrients and trace metals of the feedstock and biochar samples were measured by wet acid-extraction method followed by ICP-MS (Inductively Coupled Plasma Mass Spectroscopy, Thermo Scientific, PerkinElmer, Inc., Waltham, MA, USA) in accordance with EPA method 3050B and Toxicity Characteristic Leaching Procedure Protocol: Method 1311 by SW-846, respectively. The pH was measured using a pH meter (Xinksite Inc., Mainz, Germany).
Kinetic and Methane Yield Calculation
The AD process can be modeled using a first-order kinetic model (Mahmoud et al., 2004; Thangamani et al., 2010; Priyadarshini et al., 2015). According to this model, the rate of conversion of substrate to biogas/methane is directly proportional to the concentration of substrate, and the volume of gas produced is proportional to the mass of the substrate consumed, according to the following equations:
where k is the overall rate constant (day−1), G is the cumulative gas production (L), C is the yield constant or methane yield (L/g), V is the volume of the reactor (L), Bo is the initial substrate concentration (initial VS) (g/L), and B is the final substrate concentration (final VS) (g/L).
Integration of Equation (4) gives
where t0 is the lag time days and t > t0.
Substituting B in Equation (5) gives
Rearranging Equation (6), gives the following expression:
The methane yield (YCH4), C, was calculated from the experimental data (L/g), whereas the overall rate constant degradation “k” was calculated from Equation (7).
Theoretical Methane Production Potential
The maximum methane production potential can be calculated from the amount of material and the COD concentration using Equation (9), assuming that this equation is valid for any substance or product (Nielfa et al., 2015). This equation gives the theoretical value of methane at laboratory conditions:
where BMPthCOD is the theoretical production at laboratory conditions (L/g), R is the gas constant (R = 0.082 atm L/mol K), T is the temperature of the glass bottle (308 K), p is the atmospheric pressure (1 atm.), VSadded (g) are the VSs of the substrate, and nCH4 is the amount of molecular methane (mol) determined from Equation (10).
Statistical Analysis
The statistical difference of the methane production was determined using OriginLab Corporation, Northampton, MA, USA (version 8.0773) analysis. All treatment effects were considered to be significant at P < 0.05.
Results and Discussion
Characteristics of the Feedstock
The physicochemical characteristics of the substrate containing a mixture of the four fruit wastes are summarized in Table 3. The considered FW had >80% moisture content, ~10% TS, and ~90% VS content. Moreover, it had a pH value of 5.8 and a C/N ratio of 16. The total macronutrient and micronutrient contents in fruit waste (FW) samples are presented in Table 3.
A shortage of trace metals in the feedstock could cause slowness or instability of biogas production (Fermoso et al., 2008; Pobeheim et al., 2010). In general, anaerobic microorganisms also require macroelements (such as hydrogen, oxygen, nitrogen, carbon, phosphorus, sulfur, potassium, calcium, iron, and magnesium) at a concentration higher than 10−4 mol/L. A number of micronutrients such as Co and Ni must also be present at concentrations <10−4 mol/L. Some studies have indicated that supplementation of Ni and Co stimulates anaerobic processes (Speece et al., 1983; Frosteil, 1995).
In this study, as presented in Table 3, the FW has the only limited amount of macronutrients and micronutrients, indicating that additional macronutrients and micronutrients are needed for better biogas production. The feedstock used in this study contains 0.5 mg/g of Fe, but it did not contain detectable Co and Ni. It clearly shows that FW is deficient from key trace elements, which are essential to stabilize and maintain the efficiency of the system. Inhibition of the microbial activities and ultimately the failure of the digestion process may occur due to increased VFAs caused by the deficiency of some trace elements (Shen et al., 2016). The increase of VFA concentrations can also result from the high ammonia content of FW. This ultimately inhibits the digestion process and the activity of methanogenic microorganisms, finally leading to system failure (Shen et al., 2016).
Therefore, to obtain an efficient biogas production from FW, the FW must be rich in the specific elements such as Fe, Co, Ni, Se, and Mo to obtain an optimal bacterial growth (Table 4).
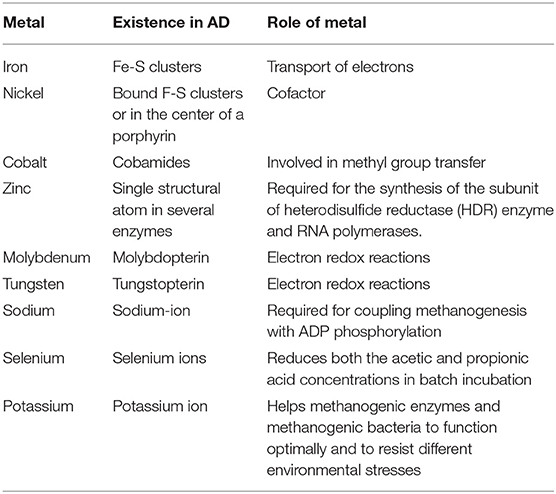
Table 4. Crucial role of important ion channels in the growth of methanogenic microorganisms (Somitsch, 2007; Fermoso et al., 2008; Pobeheim et al., 2010; Demirel and Scherer, 2011; Banks et al., 2012; Goswami et al., 2016).
Many studies have shown that the addition of trace elements improved the AD process. It improves the digester stability; it enhances the degradation of organic matter, and it results in lower VFA generation and higher biogas yield (Rajagopal et al., 2013; Wang et al., 2016). An adequate amount of trace elements must be present to support the metabolism of microorganisms, thereby maintaining an effective digestion process. For instance, according to Demirel and Scherer (2011), in their research to improve the efficiency of food digestion mixed with cosubstrate of wine, wastewater shows that the trace elements that can be found in the cosubstrate of swine waste can decrease the accumulation of VFA, and this leads to enhanced biogas production when compared with reactor that contains only FW. In addition to this, the same result also reported by Zhang et al. (2011), in their research to compare the efficiency of the biogas production of FW mixed with piggery wastewater as codigestion in AD, shows that the reactor that contains FW mixed with piggery wastewater as codigestion resulted in high methane production and less accumulation of VFA as well as high stability of the process when compared with reactor that has FW with controlled pH. This is due to the piggery wastewater containing adequate trace elements such as Fe, Mo, and Ni that support the metabolism of the microorganism in the AD process. Moreover, they claimed that adding the trace elements as a supplement in the anaerobic codigestion was one of the main parameters in the methanogens stage to improve the methanogens. In a study by Banks et al. (2012), 0.16 and 0.22 mg/kg of Se and Co, respectively, were added to an AD with a VFA concentration of 5,000–6,100 mg/L and total ammonia concentration (TAN) concentration of 5,000 mg/L. However, the concentration of TAN in the reactors without these elements (control) was increased up to 6,100 mg/L. Trace elements, such as selenium (Se), molybdenum (Mo), and tungsten (W), are very important for methanogens as it helps in the oxidation of formate by enzyme formate dehydrogenase (Banks et al., 2012). Moreover, trace elements can enhance the metabolic and growth of microbes (Appels et al., 2008), as summarized in Table 4. In general, the fruit waste is rich in the conventional elements (e.g., K, Ca, and Mg), but it has a deficiency in the main trace elements such as Fe, Co, Ni, Se, and Mo. Hence, adding biochar as an additive to FW with other organic substances has been a promising way for improving the performance of AD, as confirmed by numerous studies (Luo et al., 2013; Zhao et al., 2015; Fagbohungbe et al., 2017). Many studies have shown that higher buffer capacity along with an optimum nutrient balance enhances the biogas/methane yields of the system.
Characteristics of the Biochar
Physicochemical Properties
The physicochemical properties of the sewage sludge digestate biochar prepared at two different pyrolysis temperatures are summarized in Table 5.

Table 5. Physico-chemical properties of sewage sludge digestate biochars produced at different temperatures (n = 3)*.
The physical and chemical properties of the biochar mostly depend on the type of feedstock and pyrolysis conditions such as the speed of temperature increase, pyrolysis pressure, pyrolysis temperature, residence time, feedstock type, and heat transfer rate (Ahmad et al., 2014). According to Fagbohungbe et al. (2017), the most important properties of biochar to be used as an additive in AD process are the ion-exchange capacity, the surface microstructure, the pore size, the pore volume, and the hydrophobicity, which are strongly influencing the immobilization of microorganisms, enhancing the direct interspecies electron transfer (DIET), contributing to the increase of alkalinity and cation exchange of AD and therefore the stability and performance of AD. As reported by Masebinu et al. (2019), the addition of biochar can mitigate the operation challenges of AD due to the following properties of biochar: (i) immobilization of bacterial cells, (ii) increasing the buffering capacity of AD, and (iii) sorption of inhibitors, but the amplitude of the mitigation is strongly affected by the properties of the biochar, which depends on the pyrolysis temperature and feedstock origin.
The results reported in Table 5 indicate that there is an increase in pH and a decrease in electrical conductivity (EC) with an increase in pyrolysis temperature. Similar observations were reported by Deressa (2015). The increase in pH at increased pyrolysis temperature was ascribed mainly to the minerals present such as Ca2+ that increased in concentration at higher pyrolysis temperature, and this led to an increase in pH value. Such a pH increase is induced by the alkalization effect of these cations. As a result, the pH value of sludge biochar, which was pyrolyzed at 550°C (9.5), was higher than that of sludge biochar pyrolyzed at 350°C (6.4). The increase in the ash content with the increase in pyrolysis temperature results from the progressive concentration increase of inorganic constituents with more organic material decomposition.
The fixed carbon content at the two pyrolysis temperatures (350 and 550°C) was 13.2–15.3%, respectively, indicating a slightly increasing trend with an increase in temperature. Higher ash content and lower VSs were obtained at the highest pyrolysis temperature. Higher ash content and lower VSs were obtained at the highest pyrolysis temperature, which leads to the volatilization of inorganic oxides such as Si, Al, and Fe (Lu et al., 1995). Another factor that affects the properties of biochar is EC values, which relate to the concentration of total dissolved salts that could be used to describe the variation in the organic and inorganic ions. The decrease in EC at increased pyrolysis temperature could be due to the decrease in the concentration of leached minerals such as K+ and Na+ (Zhang et al., 2015) as shown in Table 7.
Amount of Trace Elements in the Digestate Biochar
As can be seen from Table 6, there is an increase in the total concentration of trace metals with an increase in pyrolysis temperature. This can be explained by the fact that organic material decomposition is more complete when temperature increases, while metals remain stable in the solid. However, the biochar with low pyrolysis temperature (350°C) can release more trace metals by leaching than that produced at higher pyrolysis temperature (550°C) (Table 7).
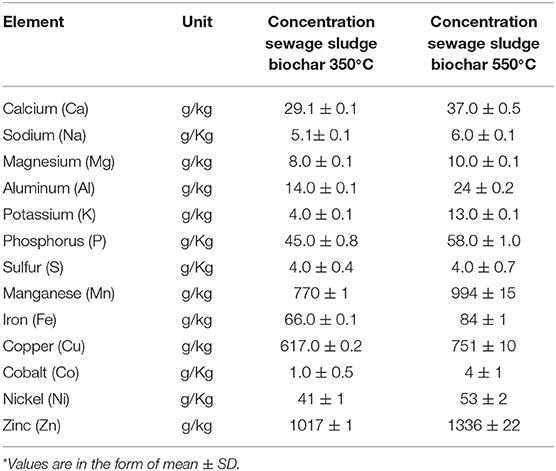
Table 6. Total element composition of sewage sludge biochars produced at 350 and 550°C using wet acid-extract method followed by ICP-OES in accordance with EPA method 3050B (n = 3)*.
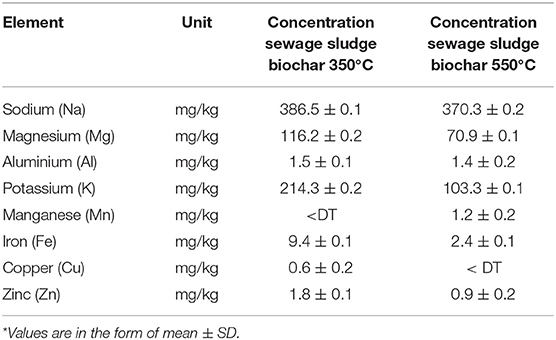
Table 7. Amount of trace elements leached from sewage sludge biochars at 350 and 550°C in the water extract according to the Toxicity Characteristic Leaching Procedure (TCLP) Protocol: Method 1311 by SW-846 (n = 3)*.
In general, the pyrolysis process brought a major variation in the sludge-derived biochar fixed carbon content and influenced the changes in the apparent nutrient concentration such as sodium, potassium, iron, copper, and zinc. Particularly, the leaching of nutrients into the AD system was found to decrease with biochar pyrolysis temperature.
Effect of Biochar Addition on Methane Production
The current study shows that the AD of fruit wastes was enhanced by the additions of sewage sludge biochar. Figures 1A–C show the cumulative methane production over 50 h of operation in the first phase with and without the different ratios of biochar addition. A comparison between experimental results and estimated maximum biodegradability methane yields for the fruit waste shows that the maximum biodegradability for the tested fruit substrates was 285.7 mL CH4/g COD, and this value is comparable to the experimental result that was 249.3 mL. This result indicated that fruit waste subjected to AD can be a useful source for biogas production.
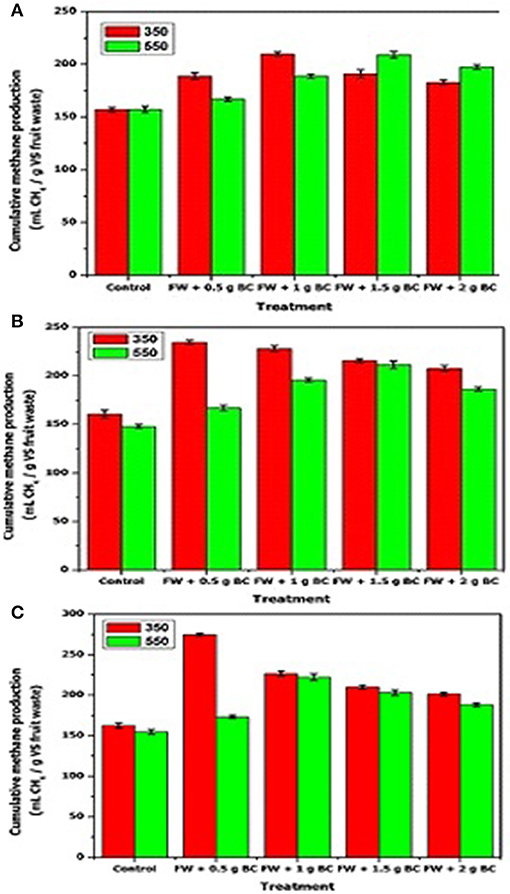
Figure 1. Cumulative methane production in different AD treatments upon addition of different amounts of sludge biochar produced at: 350°C and 550°C, for (A) ISR = 2; (B) ISR = 1.5; (C) ISR = 1. Data are given as the arithmetic mean ± standard deviation.
In all treatments under every ISR scenario, the addition of biochar enhanced methane production, but at different yields. When the ISR was 2 (Figure 1A), the p-value of paired t-tests was lower than 0.01, showing a significant effect of biochar addition on digestion between 0 and 0.5 g biochar, 0 and 1 g biochar, 0 and 1.5 g biochar, and 0 and 2 g of biochar pyrolyzed at 350 and 550°C treatment, respectively. Biochar additions of 1 and 1.5 g pyrolyzed at 350 and 550°C enhanced methane production by 25 and 20.6%, respectively, when compared to the control. However, for ISR = 2, biochar has less impact on methane production when compared to other for ISR (i.e., 1 and 1.5).
For treatment with ISR 1 (Figure 1C), biochar addition of 0.5 and 1.5 g of biochar that was pyrolyzed at 350 and 550°C could be regarded as an optimal dose to enhance methane production by 44 and 33%, respectively, when compared to the control. Considering the p-value of each paired t-test (p < 0.002), the results showed a statistically significant difference between treatments on the methane production of 0 and 0.5 g biochar, 0 and 1.5 g biochar, and 0 and 2 g of biochar pyrolyzed at 350 and 550°C biochar dose, respectively. However, there was only a slight change in 0.5 and 1 g and 0.5 and 1.5 g for digesters treated with biochar (550°C). Therefore, for ISR = 1, the amount of biochar added in the reactor had a higher impact on biochar efficiency than the one reported for ISR = 2.
When the ISR was 1.5 (or FW 237 mL and 163 mL inoculum) (Figure 1C), the p-value of each paired t-test was < 0.01 (p < 0.01). Therefore, the difference in the production of methane of 0 and 0.5 g biochar, 0 and 1.5 g biochar, 0 and 1.5 g biochar, and 0 and 2 g of biochar pyrolyzed at 350 and 550°C, respectively, was significant. For ISR 1.5, biochar pyrolyzed at 350 and 550°C displayed a significantly positive effect only when its dose reached 0.5 and 1.5 g, and it improved the methane maximum production by 42 and 30%, respectively, as compared to the treatment without biochar.
The results shown in Figures 1A–C indicate that the reactor containing biochar pyrolyzed at a lower temperature (350°C) produced more methane than the reactor containing biochar pyrolyzed at a higher temperature (550°C). One of the reasons could be that the trace elements contained in sludge biochars were in a poorly leachable chemical form (Table 7) so that they cannot be released in the AD reactor, leading to a decrease in methane production. This statement is in agreement with the data reported by Mukherjee and Zimmerman (2013). These results showed that the amount and pyrolysis temperature of biochar added as well as the inoculum amount added affect biochar efficiency. In the case of low ISR, the addition of biochar was effective. But the addition of biochar was not necessarily efficient for high ISR. It was expected that a small biochar amount has more impact, and as the ISR decreases, the biochar (350°C) demand decreases, and it was the opposite for the biochar (550°C) demand. Therefore, in the BMP test, the addition of biochar varies with the amount of inoculum added in the BMP tests.
Effect of Biochar Addition on pH in the Digester
One of the important parameters that can control as well as influence the microbial activity of the AD process was pH (Ward et al., 2008; Weiland, 2010; Franke-Whittle et al., 2014; Gomez-Romero et al., 2014; Shi et al., 2014; Sawatdeenarunat et al., 2015).
In this study, it was expected that the pH of the digester could increase with the addition of biochar owing to the alkaline nature of biochar samples. For all treatments (ISR 2, 1.5, and 1) in AD, despite the pH decrease after the AD of FW, the initial pH was maintained in a slightly neutral range by the biochar-amended digesters (6.8–7.2 for all treatments). There was no significant difference among the different treatment groups with regard to the initial pH (p > 0.01 for all conditions). The highest final pH was found in the ISR treatment with FW + 2 g BC (8.6 ± 0.1), and the lowest pH (4.5 ± 0.1) was in the control treatment without biochar. The result showed no statistically significant difference according to the ISR and types of biochar (Figure 2). This is in agreement with the results reported by Mumme et al. (2014), Shen et al. (2015), and Wang et al. (2017). Moreover, they claimed that digesters without supplement of the biochar can seriously inhibit the methanogenic activity at a pH lower than 6.6, and this leads to a decline in the methane yield. Therefore, the alkaline nature of biochar may enhance methane production by an increase in pH resulting from the conversion of CO2 to or . Improved buffering capacity can improve reactor stability through alleviation of pH drop caused by the accumulation of VFAs.
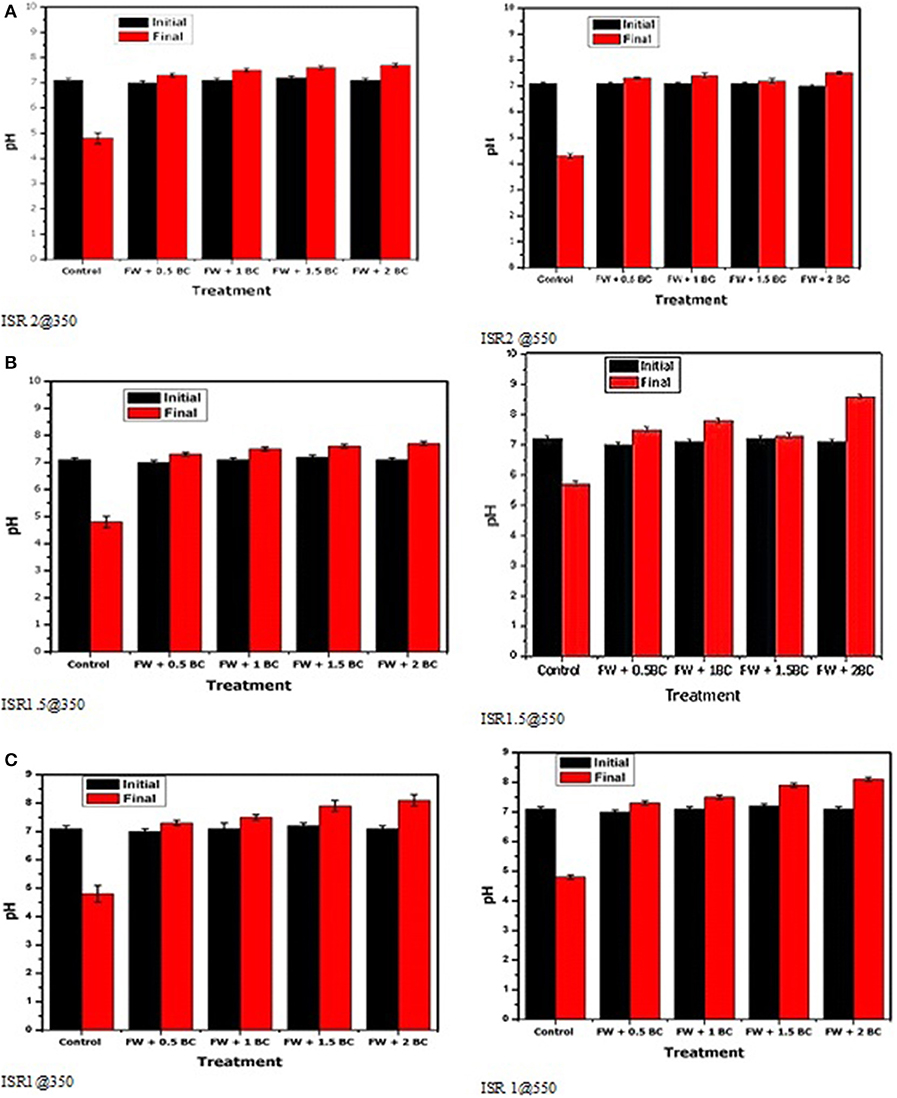
Figure 2. Initial and final pH of the different AD treatments upon addition of different amounts of sludge biochar produced at: 350°C and 550°C, for ISR 2 (A), ISR 1.5 (B), ISR 1 (C) experiments. Averages of triplicate measurements are displayed, and error bars correspond to standard deviations.
Effect of Biochar Addition on VFA Concentration in the Digester
Another most essential intermediate product of AD during the production of methane was VFAs. However, high accumulation of the VFAs in the digester can lead to the failure of the digestion process so that controlling and evaluation of the amount of VFAs in the anaerobic reactor process were considered as important parameters in the AD process (Zamanzadeh et al., 2016). The VFA concentration is displayed in Figures 3A–C. In all cases, VFA concentration increased quickly during the treatment with no biochar and gradually decreased to very low values afterward in the treatment with biochar, in accordance with the changing trend of pH as shown in Figures 3A–C.
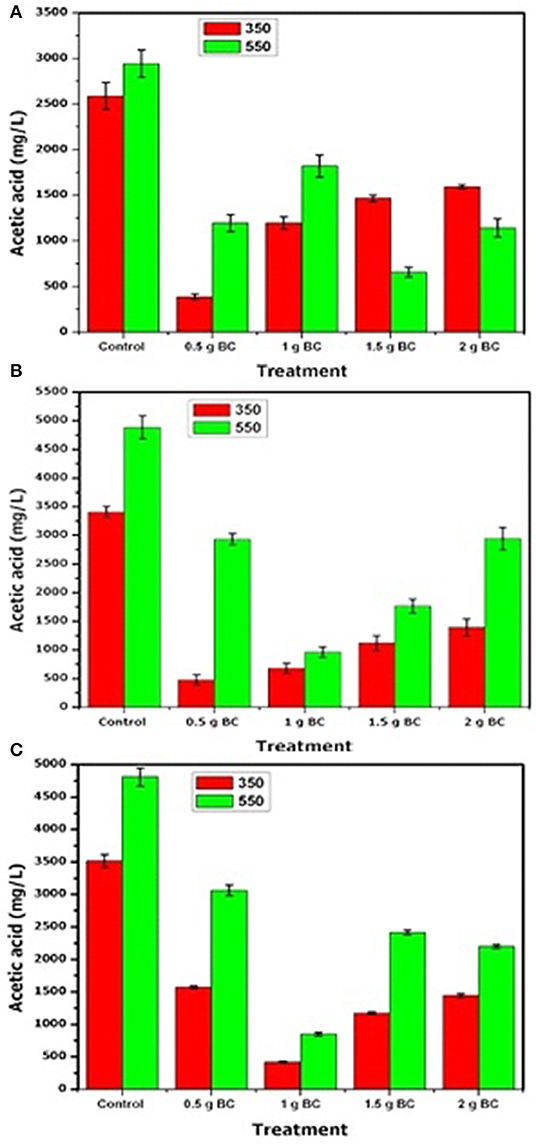
Figure 3. Volatile fatty acid (acetic acid) concentration in anaerobic digestion for ISR 2 (A), ISR 1.5 (B), ISR 1 (C) and the addition of different amounts of sludge biochar obtained at 350 and 550°C. Data are means of triplicates and error bars show standard deviations.
For ISR = 2 (Figure 3A), only acetic acid was detected. The maximum VFA concentrations were 2,587, 387, 1,196, 1,465, and 1,594 mg/L for sewage sludge biochar (350°C) additions of 0, 0.5, 1, 1.5, and 2 g, respectively, and 2,943, 1,196, 1,821, 646, and 1,142 mg/L for sewage sludge biochar (550°C) additions of 0, 0.5, 1, 1.5, and 2 g, respectively. In the experiments having ISR 0.5 and 1.5 g of biochar prepared at 350 and 550°C, the VFA concentrations decreased faster than that for the other digesters.
For ISR = 1 (Figure 3C), acetic acid was detected (as for ISR 2). The maximum VFA concentration were 3,407, 473, 678, 1,120, and 1,390 mg/L for sewage sludge biochar (350°C) additions of 0, 0.5, 1, 1.5, and 2 g, respectively, and 4,885, 2,932, 959, 1,767, and 1,943 mg/L for sewage sludge biochar (550°C) additions of 0, 0.5, 1, 1.5, and 2 g, respectively, and in accord with the change trend of pH showed in Figure 2 with biochar than in the control (no biochar added). However, for the dose of the biochar treatment with 0.5 and 1 g of biochar prepared at 350 and 550°C pyrolysis conditions, respectively, the VFA concentrations decreased faster than for the other digesters, and this shows that the degradation of VFAs in the treatment with biochar was faster than that in the control (without biochar).
For ISR = 1.5 (Figure 3B), only acetic acid was detected as well. The maximum VFA concentration was 3,515 mg/L in the control without the biochar, whereas in the digester mixed with the biochar it was 1,573, 419, 1,174, and 1,444 mg/L for sewage sludge biochar (350°C) additions of 0.5, 1, 1.5, and 2 g, respectively, and 4,809, 3,062, 851, 2,415, and 2,199 mg/L for sewage sludge biochar (550°C) additions of 0, 0.5, 1, 1.5, and 2 g, respectively. For this ISR(1.5), the dose of biochar with 1 and 1.5 g of biochar prepared at 350°C and 550°C pyrolysis conditions, respectively, the VFA concentrations decreased is lower than for the other digesters.
In this study, only one VFA compound was detected and that is acetic acid. Acetate is an important intermediate product for methane production and has a crucial impact on acetotrophic methanogens. However, the growth of Methanosaeta bacteria favored the low concentration of acetate. Therefore, with the rapid accumulation of VFAs, AD of fruit waste usually results in process inhibition, and an increase of the VFA concentration occurred while decreasing the ISR. However, the inhibition can be alleviated by adding biochar.
Impact of the Biochar Dose on Methane Production Rate and Yield of Anaerobic Digestion Fruit Waste
The performance of the codigestion process with sewage sludge biochar produced at 350 and 550°C was evaluated by determining the methane yield and the first-order rate constant of the kinetic model. The comparison of methane yield and rate parameters with and without biochar of cosubstrate after the AD retention time of 50 h is reported in Table 8.
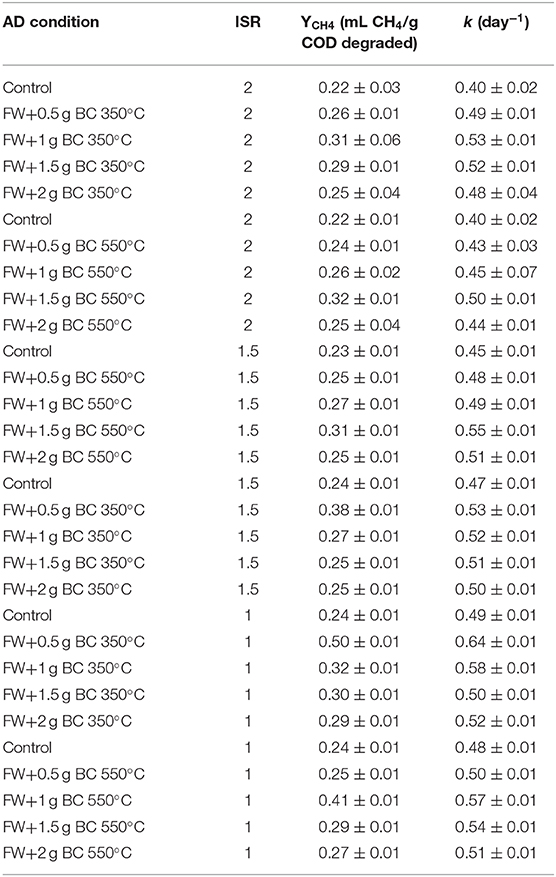
Table 8. Methane yield (YCH4) and rate constant (k) for different AD conditions for ISR 2, 1.5, and 1.
For ISR 2, the yield of methane and the kinetic behavior of the sewage sludge biochar at 350 and 550°C in AD are summarized in Table 8. The biochar (350°C) amendment raised the methane yield (YCH4) of the digesters by 7.7, 22.6, 17.2, and 4% with FW and 0.5, 1, 1.5, and 2 g of BC treatment, respectively, compared to the control. However, the methane yield with the biochar (550°C) amendment raised the methane yield (YCH4) of the digesters by 8.3, 15.4, 31.3, and 12% with 0.5, 1, 1.5, and 2 g of BC treatment, respectively, compared to the control. These results were in accordance with the CH4 volume production data. The 1 and 1.5 g doses of biochar 350 and 500°C lead to the highest cumulative CH4 volume production, respectively.
For ISR 1 (Table 8), the biochar (350°C) amendment raised the methane yield (YCH4) of the digesters by 52, 25, 20, and 17% with FW and 0.5, 1, 1.5, and 2 g of BC treatment, respectively, compared to the control. However, the biochar (550°C) amendment raised the YCH4 of the digesters by 16, 41, 17, and 11% with 0.5, 1, 1.5, and 2 g of BC treatment, respectively, compared to the control. These results were consistent with the CH4 volume production data. The 0.5 and 1 g doses of 350 and 550°C digester lead to the maximum cumulative CH4 volume production, respectively.
At ISR 1.5 (Table 8), the sewage sludge biochar (550°C pyrolysis) raised the methane yield (YCH4) of the digesters by 4.9, 14.6, 23.8, and 6.8% with FW + 0.5, 1, 1.5, and 2 g of BC treatment, respectively, compared to the control. At 350°C pyrolysis, the sewage sludge biochar raised the methane yield (YCH4) of the digesters by 38.7, 13.9, 6.3, and 4.1% with FW + 0.5, 1, 1.5, and 2 g of BC treatment, respectively, compared to the control. The reactor flask mixed with a lower biochar dosage (0.5 g) led to significantly higherYCH4 values than the control for 350°C pyrolysis temperature, whereas 1.5 g dose for 550°C pyrolysis of biochar led to the maximum methane production. This result corresponds to the finding reported by Shen et al. (2016) that showed the kinetic yield and methane production are dependent on the dose of the biochar and its pyrolysis temperature. In contrast, according to Bai et al. (2013), in their research to compare the kinetic degradation of hydrochar and pyrolytic biochar in AD, the digester that contains hydrochar can easily degrade, whereas the digester that contains pyrolytic biochar was more stable, and this shows less degradation kinetics than hydrochar and moreover, they claimed that the type of biochar can also affect the kinetics degradation in AD.
The reaction rate coefficient (k) values for ISR 2, the biochar pyrolyzed at 350 and 550°C with doses of 0.5, 1, 1.5, and 2 g were in the range of 0.4–0.53 d−1. However, the differences between biochar (350 and 550°C) amended digester and the control with regard to the k values were not significant (p > 0.01 in all conditions). For ISR 1, the kinetic values (k) of the biochar (350°C) with doses of 0.5, 1, 1.5, and 2 g achieved significantly higher k values (0.50–0.64 d−1) than the control (0.49 d−1). However, the differences between biochar (550°C) treatment reactors and the control with regard to the k values were not significant (p > 0.2 for all conditions).
At ISR 1.5, the sewage sludge biochar at 350°C pyrolysis mixed faster degradation k values 0.55, 0.49, 0.48, and 0.47 d−1 for FW + 0.5, 1, 1.5, and 2 g BC treatment, respectively. Digesters FW + 0.5 g BC, FW + 1 g BC achieved significant difference higher than the control (p < 0.001). Similarly, in a batch test in a digester blended with 550°C sludge biochar, the degradation k values of 0.53, 0.52, 0.51, and 0.50 d−1 for the reactor flask containing FW + 0.5, 1, 1.5, and 2 g BC treatment, respectively. Treatment with FW + 0.5, 1, 1.5, and 2 g BC therefore showed significant difference, namely, higher than the control (p < 0.001).
In this study, the biochar at a higher dose increases the stability of the system by increasing alkalinity; however, it leads to inhibitory effect for kinetic degradation and methane yield in both types of biochar; therefore, that microbial activity and kinetics may be inhibited by the addition of a high amount of biochar in all treatment conditions and at both pyrolysis temperatures of the biochar. Shen et al. (2016) reported when monitoring the methane production using woody biochar under thermophilic and mesophilic conditions, the addition of biochar increases degradability of sludge biomass generated from the municipal wastewater treatment plants to a certain level. However, the study came up with a conclusion that adding the biochar beyond a certain limit does not contribute to improving further the degradability. Rather, it constrains the growth of microorganisms and makes the biomass be inhibited, which is in agreement with the present study.
Conclusions
The effects of supplementing sewage sludge biochar pyrolyzed at 350 or 550°C on the AD of fruit wastes with 2, 1.5, and 1 ISR were investigated. The first-order kinetic analysis model was used to determine the kinetic parameters of the process. In addition, the concentration of macro and micronutrients of the biochars was measured to analyze the nutrient leaching from the system to the AD. The digestion performances in the treatments at ISR = 2 with an added concentration of biochar of 1 and 1.5 g/g VS-fruit waste at a temperature of 350 and 550°C, respectively, gave the best results. In treatments with biochar concentrations 0.5 and 1.5 g/g VS fruit waste for 350 and 550°C correspondingly, a decrease in ISR to 1.5 resulted in good digester performance. In treatments with biochar concentrations 0.5 and 1 g/g VS fruit waste for 350 and 550°C, respectively, the use of a very low ISR value (ISR = 1) results in a better digestive performance. The addition of biochar prepared at a low pyrolysis temperature (350°C) slightly increased the methane production as compared to high temperature (550°C) likely due to a better release of micronutrients into digestate. The kinetic analysis models revealed that the degradation rate of dissolved organics was inhibited as a result of adding biochar beyond the optimum level. It was observed that biochars reduced VFA formation upon the digestion of fruit waste as compared to the control reactor, which indicated that the metabolic process of the system was improved. This study suggested that the utilization of biochar in the AD for CH4 production is a promising technology, which needs further investigation for its economic feasibility for large-scale biogas plants.
Data Availability Statement
All datasets generated for this study are included in the article/Supplementary Material.
Author Contributions
TA and SW performed the laboratory experiments and data analysis. TA wrote the manuscript. ER, EH, and CD assisted in designing the laboratory experiments, as well as in writing the manuscript. ER and CD offered technical guidance, supported in data analysis and worked on the completed manuscript. EH provided overall guidance, supervision, and scientific knowledge support for this research work.
Funding
This study is based on a thesis submitted by the first author to IHE Delft Institute for Water Education (Delft, The Netherlands), to obtain the MSc degree in Environmental Science. TA thanks the Netherlands Fellowship Programmes (NFP) for providing a scholarship.
Conflict of Interest
The authors declare that the research was conducted in the absence of any commercial or financial relationships that could be construed as a potential conflict of interest.
Acknowledgments
Additionally, the authors would like to thank Franck, Ferdi, Lyzette, Berend, Peter, and all laboratory staff members for their constant support and help in running experiments and analyses.
Supplementary Material
The Supplementary Material for this article can be found online at: https://www.frontiersin.org/articles/10.3389/fenrg.2020.00031/full#supplementary-material
References
Agrafioti, E., Bouras, G., Kalderis, D., and Diamadopoulos, E. (2013). Biochar production by sewage sludge pyrolysis. J. Anal. Appl. Pyrolysis. 101, 72–78. doi: 10.1016/j.jaap.2013.02.010
Ahmad, M., Rajapaksha, A. U., Lim, J. E., Zhang, M., Bolan, N., Mohan, D., et al. (2014). Biochar as a sorbent for contaminant management in soil and water: a review. Chemosphere 99, 19–33. doi: 10.1016/j.chemosphere.2013.10.071
APHA, AWWA, WEF. (2012). Standard Methods for the Examination of Water and Wastewater, (22nd ed.). Washington, DC: American Public Health Association; American Water Works Association; Water Environment Federation.
Appels, L., Baeyens, J., Degrève, J., and Dewil, R. (2008). Principles and potential of the anaerobic digestion of waste activated sludge. Prog. Energy Combust. Sci. 34, 755–781. doi: 10.1016/j.pecs.2008.06.002
Ariunbaatar, J., Ozcan, O., Bair, R., Esposito, G., Ball, R., Lens, P. N., et al. (2017). Bioaugmentation of the anaerobic digestion of food waste by dungs of herbivore, carnivore, and omnivore zoo animals. Environ. Technol. 39, 516–526. doi: 10.1080/09593330.2017.1305002
Bai, M., Wilske, B., Buegger, F., Esperschütz, J., Kammann, C. I., Eckhardt, C., et al. (2013). Degradation kinetics of biochar from pyrolysis and hydrothermal carbonization in temperate soils. Plant Soil 372, 375–387. doi: 10.1007/s11104-013-1745-6
Banks, C. J., Zhang, Y., Jiang, Y., and Heaven, S. (2012). Trace element requirements for stable food waste digestion at elevated ammonia concentrations. Bioresour. Technol. 104, 127–135. doi: 10.1016/j.biortech.2011.10.068
Cai, J., He, P., Wang, Y., Shao, L., and Lu, F. (2016). Effects and optimization of the use of biochar in anaerobic digestion of food wastes. Waste Manag. Res. 34, 409–416. doi: 10.1177/0734242X16634196
Chen, Y., Cheng, J. J., and Creamer, K. S. (2008). Inhibition of anaerobic digestion process: a review. Bioresour. Technol. 99, 4044–4064. doi: 10.1016/j.biortech.2007.01.057
Demirel, B., and Scherer, P. (2011). Trace element requirements of agricultural biogas digesters during biological conversion of renewable biomass to methane. Biomass Bioenergy. 35, 992–998. doi: 10.1016/j.biombioe.2010.12.022
Deressa, A. (2015). Effects of soil moisture and temperature on carbon and nitrogen mineralization in grassland soils fertilized with improved cattle slurry manure with and without manure additive. J. Environ. Hum. 2, 2373–8332. doi: 10.15764/EH.2015.01001
Fagbohungbe, M. O., Herbert, B. M., Hurst, L., Ibeto, C. N., Li, H., Usmani, S. Q., et al. (2017). The challenges of anaerobic digestion and the role of biochar in optimizing anaerobic digestion. Waste Manag. 61, 236–249. doi: 10.1016/j.wasman.2016.11.028
Fagbohungbe, M. O., Herbert, B. M., Hurst, L., Li, H., Usmani, S. Q., and Semple, K. T. (2016). Impact of biochar on the anaerobic digestion of citrus peel waste. Bioresour. Technol. 216, 142–149. doi: 10.1016/j.biortech.2016.04.106
Fermoso, F. G., Collins, G., Bartacek, J., O'Flaherty, V., and Lens, P. (2008). Role of nickel in high rate methanol degradation in anaerobic granular sludge bioreactors. Biodegradation 19, 725–737. doi: 10.1007/s10532-008-9177-3
Franke-Whittle, I. H., Confalonieri, A., Insam, H., Schlegelmilch, M., and Körner, I. (2014). Changes in the microbial communities during co-composting of digestates. Waste Manag. 34, 632–641. doi: 10.1016/j.wasman.2013.12.009
Frosteil, B. (1995). Process control in anaerobic wastewater treatment. Water Sci. Technol. 17, 173–189. doi: 10.2166/wst.1985.0014
Gomez-Romero, J., Gonzalez-Garcia, A., Chairez, I., Torres, L., and García-Peña, E. I. (2014). Selective adaptation of an anaerobic microbial community: biohydrogen production by co-digestion of cheese whey and vegetables fruit waste. Int. J. Hydrogen Energy 39, 12541–12550. doi: 10.1016/j.ijhydene.2014.06.050
Goswami, R., Chattopadhyay, P., Shome, A., Banerjee, S. N., Chakraborty, A. K., Mathew, A. K., et al. (2016). An overview of physicochemical mechanisms of biogas production by microbial communities: a step towards sustainable waste management. 3 Biotech. 6:72. doi: 10.1007/s13205-016-0395-9
Hagos, K., Zong, J., Li, D., Liu, C., and Lu, X. (2017). Anaerobic co-digestion process for biogas production: progress, challenges and perspectives. Renew. Sustain. Energy Rev. 76, 1485–1496. doi: 10.1016/j.rser.2016.11.184
He, Y. D., Zhai, Y. B., Li, C. T., Yang, F., Chen, L., Fan, X. P., et al. (2010). The fate of Cu, Zn, Pb and Cd during the pyrolysis of sewage sludge at different temperatures. Environ. Technol. 31, 567–574. doi: 10.1080/09593330903514466
Huang, W., Wang, Z., Zhou, Y., and Ng, W. J. (2015). The role of hydrogenotrophic methanogens in an acidogenic reactor. Chemosphere 140, 40–46. doi: 10.1016/j.chemosphere.2014.10.047
Hwang, I. H., Ouchi, Y., and Matsuto, T. (2007). Characteristics of leachate from pyrolysis residue of sewage sludge. Chemosphere 68, 1913–1919. doi: 10.1016/j.chemosphere.2007.02.060
Ji, C., Kong, C.-X., Mei, Z.-L., and Li, J. (2017). A review of the anaerobic digestion of fruit and vegetable waste. Appl. Biochem. Biotechnol. 183, 906–922. doi: 10.1007/s12010-017-2472-x
Kistler, R. C., Widmer, F., and Brunner, P. H. (1987). Behavior of chromium, nickel, copper, zinc, cadmium, mercury, and lead during the pyrolysis of sewage sludge. Environ. Sci. Technol. 21, 704–708. doi: 10.1021/es00161a012
Lehmann, J., and Joseph, S. (2015). “Biochar for environmental management: an introduction,” in Biochar for Environmental Management, eds J. Lehmann and S. Joseph (London: Routledge), 33–46. doi: 10.4324/9780203762264
Lester, J. N., Sterrit, R. M., and Kirk, P. W. W. (1983). Significance and behaviour of heavy metals in wastewater treatment process. II. Sludge treatment and disposal. Sci. Total Environ. 30, 45–83. doi: 10.1016/0048-9697(83)90003-7
Lu, G. Q., Low, J. C. F., Liu, C. Y., and Lua, A. C. (1995). Surface area development of sewage sludge during pyrolysis. Fuel 74, 344–348. doi: 10.1016/0016-2361(95)93465-P
Luo, G., and Angelidaki, I. (2012). Integrated biogas upgrading and hydrogen utilization in an anaerobic reactor containing enriched hydrogenotrophic methanogenic culture. Biotechnol. Bioeng. 109, 2729–2736. doi: 10.1002/bit.24557
Luo, G., Wang, W., and Angelidaki, I. (2013). Anaerobic digestion for simultaneous sewage sludge treatment and CO biomethanation: process performance and microbial ecology. Environ. Sci. Technol. 47, 10685–10693. doi: 10.1021/es401018d
Mahmoud, N., Zeeman, G., Gijzen, H., and Lettinga, G. (2004). Anaerobic stabilisation and conversion of biopolymers in primary sludge, effect of temperature and sludge retention time. Water Res. 38, 983–991. doi: 10.1016/j.watres.2003.10.016
Masebinu, S. O., Akinlabi, E. T., Muzenda, E., and Aboyade, A. O. (2019). A review of biochar properties and their roles in mitigating challenges with anaerobic digestion. Renewable Sustain. Energy Rev. 103, 291–307. doi: 10.1016/j.rser.2018.12.048
Mata-Alvarez, J., Dosta, J., Romero-Güiza, M. S., Fonoll, X., Peces, M., and Astals, S. (2014). A critical review on anaerobic co-digestion achievements between 2010 and 2013. Renew. Sustain. Energy Rev. 36, 412–427. doi: 10.1016/j.rser.2014.04.039
Milios, L., and Reichel, A. (2013). Municipal Waste Management in the Netherlands. European Environment Agency. Available online at: https://www.eea.europa.eu/publications/managing-municipal-solid-waste/netherlands-municipal-waste-management (accessed March 2020).
Montecchio, D., Gallipoli, A., Gianico, A., Mininni, G., Pagliaccia, P., and Braguglia, C. M. (2017). Biomethane potential of food waste: modeling the effects of mild thermal pretreatment and digestion temperature. Environ. Technol. 38, 1452–1464. doi: 10.1080/09593330.2016.1233293
Mukherjee, A., and Zimmerman, A. R. (2013). Organic carbon and nutrient release from a range of laboratory-produced biochars and biochar-soil mixtures. Geoderma 193, 122–130. doi: 10.1016/j.geoderma.2012.10.002
Mumme, J., Srocke, F., Heeg, K., and Werner, M. (2014). Use of biochars in anaerobic digestion. Bioresour. Technol. 164, 189–197. doi: 10.1016/j.biortech.2014.05.008
Nielfa, A., Cano, R., and Fdz-Polanco, M. (2015). Theoretical methane production generated by the co-digestion of organic fraction municipal solid waste and biological sludge. Biotechnol. Rep. 5, 14–21. doi: 10.1016/j.btre.2014.10.005
NOS Annual Report (2016). https://www.government.nl/topics/food/cutting-down-on-food-waste (accessed March 2020).
Pathak, A., Dastidar, M. G., and Sreekrishnan, T. R. (2009). Bioleaching of heavy metals from sewage sludge: a review. J. Environ. Manage. 90, 2343–2353. doi: 10.1016/j.jenvman.2008.11.005
Pobeheim, H., Munk, B., Johansson, J., and Guebitz, G. M. (2010). Influence of trace elements on methane formation from a synthetic model substrate for maize silage. Bioresour. Technol. 101, 836–839. doi: 10.1016/j.biortech.2009.08.076
Priyadarshini, R., Vaishnavi, L., Murugan, D., Sivarajan, M., Sivasamy, A., Saravanan, P., et al. (2015). Kinetic studies on anaerobic co-digestion of ultrasonic disintegrated feed and biomass and its effect substantiated by microcalorimetry. Int. J. Environ. Sci. Technol. 12, 3029–3038. doi: 10.1007/s13762-014-0688-7
Rajagopal, R., Massé, D. I., and Singh, G. (2013). A critical review on inhibition of anaerobic digestion process by excess ammonia. Bioresour. Technol. 143, 632–641. doi: 10.1016/j.biortech.2013.06.030
Sawatdeenarunat, C., Surendra, K. C., Takara, D., Oechsner, H., and Khanal, S. K. (2015). Anaerobic digestion of lignocellulosic biomass: challenges and opportunities. Bioresour. Technol. 178, 178–186. doi: 10.1016/j.biortech.2014.09.103
Shen, Y., Linville, J. L., Ignacio-de Leon, P. A. A., Schoene, R. P., and Urgun-Demirtas, M. (2016). Towards a sustainable paradigm of the waste-to-energy process: enhanced anaerobic digestion of sludge with woody biochar. J. Clean. Prod. 135, 1054–1064. doi: 10.1016/j.jclepro.2016.06.144
Shen, Z., Jin, F., Wang, F., McMillan, O., and Al-Tabbaa, A. (2015). Sorption of lead by Salisbury biochar produced from British broadleaf hardwood. Bioresour. Technol. 193, 553–556. doi: 10.1016/j.biortech.2015.06.111
Shi, J., Xu, F., Wang, Z., Stiverson, J. A., Yu, Z., and Li, Y. (2014). Effects of microbial and non-microbial factors of liquid anaerobic digestion effluent as inoculum on solid-state anaerobic digestion of corn stover. Bioresour. Technol. 157, 188–196. doi: 10.1016/j.biortech.2014.01.089
Somitsch, W. (2007). “Prozesstechnische und biochemische Wirkungsweise von Betriebhilfsmitteln in der Methangärung,” in OTTI-Symposium Bioenergie. (Germany: Kloster Banz).
Speece, R. E., Parkin, G. F., and Gallagher, D. (1983). Nickel stimulation of anaerobic digestion. Water Res. 17, 677–683. doi: 10.1016/0043-1354(83)90237-3
Sunyoto, N. M. S., Zhu, M., Zhang, Z., and Zhang, D. (2016). Effect of biochar addition on hydrogen and methane production in two-phase anaerobic digestion of aqueous carbohydrates food waste. Bioresour. Technol. 219, 29–36. doi: 10.1016/j.biortech.2016.07.089
Thangamani, A., Rajakumar, S., and Ramanujam, R. A. (2010). Anaerobic co-digestion of hazardous tannery solid waste and primary sludge: biodegradation kinetics and metabolite analysis. Clean Technol. Environ. Policy 12, 517–524. doi: 10.1007/s10098-009-0256-x
Tiwary, A., Williams, I. D., Pant, D. C., and Kishore, V. V. N. (2015). Assessment and mitigation of the environmental burdens to air from land applied food-based digestate. Environ. Pollut. 203, 262–270. doi: 10.1016/j.envpol.2015.02.001
Wang, B., Jiang, Y. S., Li, F. Y., and Yang, D. Y. (2017). Preparation of biochar by simultaneous carbonization, magnetization, and activation for norfloxacin removal in water. Bioresour. Technol. 233, 159–165. doi: 10.1016/j.biortech.2017.02.103
Wang, L., Liu, J., Zhao, Q., Wei, W., and Sun, Y. (2016). Comparative study of wastewater treatment and nutrient recycle via activated sludge, microalgae and combination systems. Bioresour. Technol. 211, 1–5. doi: 10.1016/j.biortech.2016.03.048
Ward, A. J., Hobbs, P. J., Holliman, P. J., and Jones, D. L. (2008). Optimisation of the anaerobic digestion of agricultural resources. Bioresour. Technol. 99, 7928–7940. doi: 10.1016/j.biortech.2008.02.044
Weiland, P. (2010). Biogas production: current state and perspectives. Appl. Microbiol. Biotechnol. 85, 849–860. doi: 10.1007/s00253-009-2246-7
Wise, D. L., Cooney, C. L., and Augenstein, D. C. (1978). Biomethanation: Anaerobic fermentation of CO2, H2 and CO to methane. Biotechnol. Bioeng. 20, 1153–1172. doi: 10.1002/bit.260200804
Wongrod, S., Simon, S., Guibaud, G., Lens, P. N. L., Pechaud, Y., Huguenot, D., et al. (2018). Lead sorption by biochar produced from digestates: consequences of chemical modification and washing. J. Environ. Manag. 219, 277–284. doi: 10.1016/j.jenvman.2018.04.108
Xu, H., Gong, S., Sun, Y., Ma, H., Zheng, M., and Wang, K. (2015). High-rate hydrogenotrophic methanogenesis for biogas upgrading: the role of anaerobic granules. Environ. Technol. 36, 529–537. doi: 10.1080/09593330.2014.979886
Yuan, J. H., Xu, R. K., and Zhang, H. (2011). The forms of alkalis in the biochar produced from crop residues at different temperatures. Bioresour. Technol. 102, 3488–3497. doi: 10.1016/j.biortech.2010.11.018
Zamanzadeh, M., Hagen, L. H., Svensson, K., Linjordet, R., and Horn, S. J. (2016). Anaerobic digestion of food waste-effect of recirculation and temperature on performance and microbiology. Water Res. 96, 246–254. doi: 10.1016/j.watres.2016.03.058
Zhang, C., Su, H., Baeyens, J., and Tan, T. (2014). Reviewing the anaerobic digestion of food waste for biogas production. Renew. Sustain. Energy Rev. 38, 383–392. doi: 10.1016/j.rser.2014.05.038
Zhang, J., Lü, F., Zhang, H., Shao, L., Chen, D., and He, P. (2015). Multiscale visualization of the structural and characteristic changes of sewage sludge biochar oriented towards potential agronomic and environmental implication. Sci. Rep. 5:9406. doi: 10.1038/srep09406
Zhang, L., and Jahng, D. (2012). Long-term anaerobic digestion of food waste stabilized by trace elements. Waste Manag. 32, 1509–1515. doi: 10.1016/j.wasman.2012.03.015
Zhang, L., Lee, Y. W., and Jahng, D. (2011). Anaerobic co-digestion of food waste and piggery wastewater: focusing on the role of trace elements. Bioresour. Technol. 102, 5048–5059. doi: 10.1016/j.biortech.2011.01.082
Keywords: food waste, anaerobic digestion, biomethane potential, digestate biochar, additives
Citation: Ambaye TG, Rene ER, Dupont C, Wongrod S and van Hullebusch ED (2020) Anaerobic Digestion of Fruit Waste Mixed With Sewage Sludge Digestate Biochar: Influence on Biomethane Production. Front. Energy Res. 8:31. doi: 10.3389/fenrg.2020.00031
Received: 17 November 2019; Accepted: 19 February 2020;
Published: 31 March 2020.
Edited by:
Baharak Sajjadi, University of Mississippi, United StatesReviewed by:
Obulisamy Parthiba Karthikeyan, University of Houston, United StatesCheng Tung Chong, Shanghai Jiao Tong University, China
Copyright © 2020 Ambaye, Rene, Dupont, Wongrod and van Hullebusch. This is an open-access article distributed under the terms of the Creative Commons Attribution License (CC BY). The use, distribution or reproduction in other forums is permitted, provided the original author(s) and the copyright owner(s) are credited and that the original publication in this journal is cited, in accordance with accepted academic practice. No use, distribution or reproduction is permitted which does not comply with these terms.
*Correspondence: Teklit Gebregiorgis Ambaye, teklitgeb@gmail.com; Eldon R. Rene, e.raj@un-ihe.org