- 1Department of Chemical Engineering, South Tehran Branch, Islamic Azad University, Tehran, Iran
- 2Department of Microbial Biotechnology, Agricultural Biotechnology Research Institute of Iran, Agricultural Research, Extension, and Education Organization, Karaj, Iran
- 3Biofuel Research Team, Karaj, Iran
- 4Engineering and Technology, College of Agriculture and Natural Resources, University of Tehran, Karaj, Iran
- 5Department of Microbial Biotechnology, School of Biology and Centre of Excellence in Phylogeny of Living Organisms, College of Science, University of Tehran, Tehran, Iran
- 6Nanotechnology Research Center, Research Institute of Petroleum Industry, Tehran, Iran
- 7Department of Food Science and Technology, College of Agriculture, Isfahan University of Technology, Isfahan, Iran
- 8Department of Mechanics and Biosystems Engineering, Agricultural Faculty, Razi University, Kermanshah, Iran
- 9Center of Excellence in Environmental Studies, King Abdulaziz University, Jeddah, Saudi Arabia
Application of acid-activated bentonite and SO3H-functionlized multiwall carbon nanotubes (SO3H-MWCNTs) for lowering free fatty acids (FFAs) content of low-quality residual olive oil, prior to alkali-catalyzed transesterification was investigated. The used bentonite was first characterized by Scanning Electron Microscopy (SEM), Inductively Coupled Plasma mass spectrometry (ICP-MS), and X-ray fluorescence (XRF), and was subsequently activated by different concentrations of H2SO4 (3, 5, and 10 N). Specific surface area of the original bentonite was measured by Brunauer, Emmett, and Teller (BET) method at 45 m2/g and was best improved after 5 N-acid activation (95–98°C, 2 h) reaching 68 m2/g. MWCNTs was synthesized through methane decomposition (Co-Mo/MgO catalyst, 900°C) during the chemical vapor deposition (CVD) process. After two acid-purification (HCl, HNO3) and two deionized-water-neutralization steps, SO3H was grafted on MWCNTs (concentrated H2SO4, 110°C for 3 h) and again neutralized with deionized water and then dried. The synthesized SO3H-MWCNTs were analyzed using Fourier-Transform Infrared Spectroscopy (FTIR) and Transmission Electron Microscopy (TEM). The activated bentonite and SO3H-MWCNTs were utilized (5 wt.% and 3 wt.%, respectively), as solid catalysts in esterification reaction (62°C, 450 rpm; 15:1 and 12:1 methanol-to-oil molar ratio, 27 h and 8 h, respectively), to convert FFAs to their corresponding methyl esters. The results obtained revealed an FFA to methyl ester conversion of about 67% for the activated bentonite and 65% for the SO3H-MWCNTs. More specifically, the acid value of the residual olive oil was decreased significantly from 2.5 to 0.85 and 0.89 mg KOH/g using activated bentonite and SO3H-MWCNTs, respectively. The total FFAs in the residual olive oil after esterification was below 0.5%, which was appropriate for efficient alkaline-transesterification reaction. Both catalysts can effectively pretreat low-quality oil feedstock for sustainable biodiesel production under a biorefinery scheme. Overall, the acid-activate bentonite was found more convenient, cost-effective, and environment-friendly than the SO3H-MWCNTs.
Introduction
Clay, particularly bentonites (clay mineral with different colors) are very popular in various industries (Christidis, 2013). Bentonite, has been applied as adsorbent, bleaching earth, and catalyst, as well as in eutrophication management and remediation of pollutions (Önal, 2006; Jeenpadiphat and Tungasmita, 2014; Copetti et al., 2016; El Korashy et al., 2016; Rezende and Pinto, 2016). Smectites are major clay minerals in bentonite with a 2:1 structure, or in another word, two silica tetrahedral sheets sandwich, an aluminum octahedral sheet or, a three-layer structure in which an aluminum octahedral sheet is located between two silica tetrahedral sheets. Replacement of some trivalent ions such as Al3+, by some divalent ions like Fe2+ or Mg2+ in the octahedral layer, or substitution of Si4+ with Al3+ in the tetrahedral layer generates a net negative electric charge on the surface of clay. This negative charge is balanced by either Ca2+ or Na+ cations on the surface of clay in Na-type bentonite or Ca-type bentonite, respectively, (Shen, 2001; Önal, 2006; Önal and Sarıkaya, 2007).
Bentonites, by virtue of montmorillonite, have high absorption capacity for exchanging some certain cations from solutions with their own molecules. Bentonite quality and properties (i.e., clay minerals loading, cation exchange capacity (CEC), porosity, selectivity, surface acidity, surface area) are very important in industrial applications and could be modified by activation methods (Önal and Sarıkaya, 2007). In another word, prior to effective application of bentonite in industry, some of its properties should be improved through activation or treatment procedures. There are four major ways to activate bentonite and improve many of its properties. Those include (i) chemical, (ii) physical, (iii) pillaring, and (iv) thermal procedures. Bentonite is activated by the application of inorganic acids (such as H2SO4 or HCl) for replacing the exchangeable ions of bentonite during chemical procedure. Physical procedure involves mechanical improvement of bentonite's surface area by crushing it into smaller pieces. Similarly, pillaring procedure also improve bentonite's surface area as well as adsorption capacity. However, during pillaring procedure, these improvements are obtained through intercalating some elements or metal hydroxides between the lamellar structures of bentonite creating pillars. In the last procedure, crystalline structure, or chemical composition of bentonite is thermally modified by heating bentonite at appropriate temperatures depending on its type. Amongst the aforementioned procedures, chemical treatment is the most common, and convenient method. It is worth mentioning that acid-activated bentonite could be used as solid acid catalyst in various reactions, such as esterification of oil for biodiesel production.
Carbon nanotubes (CNTs), first synthesized in 1991, have many applications as fillers, chemical sensors, hydrogen storage, electronic devices, catalyst supports, and etc., due to their unique properties including chemical stability, electrical, and thermal properties, high surface area, mechanical characteristic, etc. (Ham et al., 2004). CNTs are divided into single-wall CNTs and multi-wall CNTs (MWCNTs), arranged like a single rolled-up graphite sheet and like multiple layers of graphite rolled-up on themselves to form a tube shape, respectively (Kalamkarov et al., 2006). These structures can provide good support for various functional groups of specific features.
Oil feedstocks could be converted into biodiesel through the transesterification reaction (Aghbashlo et al., 2018b,c); however, food vs. fuel debate prevents the application of edible oils for this purpose (Hasheminejad et al., 2011; Aghbashlo et al., 2015, 2017b; Sahafi et al., 2018). On the other hand, ~70–88% of the total biodiesel production cost arises from the cost of raw material (Haas et al., 2006). Therefore, biodiesel may be synthesized under a waste-oriented biorefinery scheme to address the aforementioned issues (Aghbashlo et al., 2018a, 2019). Under this concept, non-edible oils, such as waste cooking oils, and vegetable oil refinery waste oil that are either generated during processing oily crops into edible oil or their subsequent residuals from food processing sectors could be recycled into biodiesel (Aghbashlo et al., 2017a; Hajjari et al., 2017). For example, 0.11–0.22 billion liters a year residual olive oil is recovered from olive cake (Pütün et al., 2005). Therefore, one prominent feedstock for the reduction of biodiesel production cost is such waste/residual oil resources such as residual olive oil. The main drawbacks of such oil feedstock are; however, their high water, and free FFA contents, lowering the yield of direct alkaline-catalyzed transesterification of oil into biodiesel due to the consumption of high amounts of catalyst and the formation of soap.
Alternatively, biodiesel may be produced from high FFA oil feedstock through acid-catalyzed (trans) esterification reaction, in which FFAs and triglyceride simultaneously react with alcohol to produce methyl ester (biodiesel) (Pan et al., 2017). The main disadvantage of this strategy is the lengthy process that requires high methanol-to-oil molar ratios. Therefore, often acids are only used as a pretreatment (esterification) method only to lower FFA or acid value to the acceptable points (0.5% or 1 mg KOH/g, respectively), prior to the commencement of the alkaline-catalyzed transesterification reaction (Montefrio et al., 2010; Hasheminejad et al., 2011). Homogeneous acids (such as HCl, H2SO4, H3PO4) have been widely applied as catalyst for esterification of high FFA oil feedstocks (Photaworn et al., 2017; Murad et al., 2018). Overall, the main shortcomings of using homogeneous acids include high quantity of alcohol consumed, and that the acid used could not be recovered and should be neutralized as well. Moreover, the corrosive nature of some of these acids, for example H2SO4, could increase the maintenance costs by damaging the equipment.
Hydrophobic solid acid catalysts could be used to overcome the above-mentioned drawbacks of the homogeneous acid catalysts. Carbonized vegetable oil asphalt, ferric sulfate supported on silica, and tolune-4-sulfonic monohydrate acid are some solid acid catalysts investigated for pretreating low-quality oil feedstock for biodiesel production (Hayyan et al., 2010; Shu et al., 2010; Dokic et al., 2012). Unfortunately, despite their comparatively high catalytic activity, they suffer from high production cost, complexity of preparation methods, or harsh operating conditions.
Having considered the disadvantages of the acid pretreatment methods discussed above, searching for more efficient, less expensive, and easier to operate strategies is inevitable. Considering that, this study was set to investigate the catalytic performance of acid-activated bentonite in converting FFAs of low-quality, high FFA-containing residual olive oil into methyl esters. This could be regarded as a pretreatment step for FFA removal prior to basic-catalyzed transesterification reaction. To achieve that, bentonite was characterized, acid-activated, and its performance in decreasing FFA content of residual olive oil through conversion to methyl esters was evaluated. Moreover, MWCNTs were also synthesized, SO3H functionalized, and their performance in FFA removal from residual olive oil was evaluated and compared with that of the acid-activated bentonite.
Materials and Methods
Chemicals
Raw bentonite with the following physicochemical properties was used: surface area of 45 m2.g−1, mean pore diameter of 7.7 nm, total pore volume of 0.0898 cm3.g−1, swelling index of 15 mL/2 g, and CEC of 58 meq/100 g. Residual olive oil was purchased from an olive oil refinery and its FFA profiles and physical properties were investigated. All the chemicals used in this study were purchased either from Merck (Germany) or Sigma-Aldrich (Germany).
Bentonite Preparation, Activation, and Characterization
A uniform sample of bentonite with the size of about 130 μm was obtained by passing it through different sieves with appropriate mesh sizes. Then, aliquots of 50 g were weighted for the acid activation step.
Three different concentrations of H2SO4 (i.e., 3, 5, and 10 N) were used for the activation of 50 g bentonite. The treatment was conducted in a water bath (95–98°C, atmospheric pressure) and 250 ml H2SO4 was slowly added while the mixture was agitated at 400 rpm using a magnetic stirrer. After 2 h, samples were washed (i.e., pH 7) with deionized water until reaching a neutral pH. The remaining slurry was subsequently dried in an oven at 130°C for 2 h. The dried bentonites were kept in a desiccator until further use. The acid-activated bentonite was characterized by Scanning Electron Microscopy (SEM) (Tescan Vega3, Czech Republic), Inductively Coupled Plasma Mass Spectrometry (ICP-MS) (Agilent, United States), and X-ray fluorescence (XRF) (Spectro, Germany). The specific area of the bentonite was measured by BET technique with N2 adsorption, at 77 K, using BELSORB Mini instrument (Bel Japan, Inc.) based on the ASTM 4567.
MWCNTs Synthesis and Characterization
MWCNTs were synthesized through methane decomposition (900°C, atmospheric pressure, 20–50 min) over cobalt-molybdenum nanoparticles supported by nanoporous magnesium oxide during a chemical vapor deposition (CVD) process as described previously (Rashidi et al., 2007). Briefly, the reaction was performed using a flow of methane (50 mL/min) as carbon source and a flow of hydrogen (250 mL/min) as career gas at 900°C for 30 min. Upon the completion of the reaction, the furnace was cooled under a nitrogen atmosphere and then the product was purified. To achieve that, the resultant material was first dissolved in HCl solution (18%) for a duration of 16 h at ambient temperature (25°C ± 1) followed by filtration and several rounds of rinsing by distilled water. Subsequently, the product was dissolved in a nitric acid solution (6 M) for 6 h at 70°C. The slurry was then filtered, dried, and heated in a furnace at 400°C for 30 min. Then, the synthesized MWCNTs were subsequently sulfonated with 98% H2SO4 (110°C for 3 h), and neutralized (pH 7) by washing with deionized water. The sulfonated MWCNTs (SO3H-MWCNTs) were dried and kept until further use.
The prepared MWCNTs were characterized by transmission electron microscopy (TEM, CM30, Philips, Netherland) for morphology determination. For FTIR analysis, the samples were milled with KBr to form a very fine powder, followed by their compression into pellets. FTIR spectra were recorded on a Thermo Nicolet Nexus 670 FT-IR ESP (Thermo Nicolet Corp., Madison, WI, United States). The specific area of the MWCNTs was measured by same BET technique used for bentonite, but was analyzed by an ASAP 2010 (Micromeritics, United States).
Catalytic Activity of the Catalysts
Catalytic activities of the acid-activated bentonite (5 wt.% of oil) and SO3H-MWCNTs (3 wt.% of oil) were calculated in respect to the esterification reaction with methanol-to-oil molar ratio of 15:1 and 12:1, respectively. The reaction was conducted in a magnetic stirred tank reactor (450 rpm) equipped with an alcohol reflux system at 62°C. The acid value was determined at certain intervals and the pretreatment step was continued until the value reached about 0.5 mg KOH/g. Samples were withdrawn and immediately placed in an ice bath to stop the reaction, followed by centrifugation (3,500 rpm, 5 min). Then, these samples were used for FFA reduction measurements.
Determination of FFA Conversion
The composition of the methyl esters produced by esterification of residual olive oil and methanol in the presence of acid-activated bentonite or SO3H-MWCNTs as solid acid catalyst was determined using gas chromatography (GC; Claus 580 GC model, Perkin Elmer Co., United States). The conversion of the waste oil into methyl esters was determined by using the following equation:
where ∑A is the total area of the peaks, AIS is the peak of the internal standard (C17:0), m is weight of internal standard, and M is the sample weight.
Acid value of the samples was also measured according to the method of Cd 3d-63 provided by American Oil Chemists' Society.
Results and Discussion
Characterization of the Acid-Activated Bentonite
The morphology of the natural bentonite as shown by SEM are presented in Figure 1A. The CEC of natural bentonite (58 meq/100 g) was, to a certain extent, lower than the expected range for smecite (80–150 meq/100), showing the presence of some impurities. The characterizations of the acid-activated bentonite synthesized in this study are presented in Table 1. The results of ICP-MS revealed that the bentonite used was of Na-type. Moreover, XRF data indicated that the bentonite structure was mostly composed of Al2O3 and SiO2, providing good structure stability. It was expected that H3O+ ions replaced some of these elements (such as Na and calcium) as a result of acid pretreatment (Rezende and Pinto, 2016). Bentonite activation was performed with three different concentration of H2SO4 (3, 5, and 10 N), in order to investigate the impact of acid concentration on the surface area. Compared with natural bentonite, acid-activated bentonite displayed about 38–51% larger surface area (Table 1). It can be deduced from the BET data tabulated in Table 1, that the activation of bentonite with 5 N solution of H2SO4 triggered the largest surface area. In another word, increasing acid concentration from 3 to 5 N had a positive impact on the surface area, and consequently, on the number of hydrogen ions anchored on bentonite‘s surface. However, further elevation of acid concentration to 10 N must have damaged bentonite structure by collapsing some of its layers by dissolving them. In fact, the lower surface area obtained through the activation by 10 N H2SO4 solution signifies that fewer hydrogen ions were anchored, and thus, less catalytic activity was achieved. On this basis, bentonite sample activated by 5 N acid solution was selected for the FFA reduction experiment.
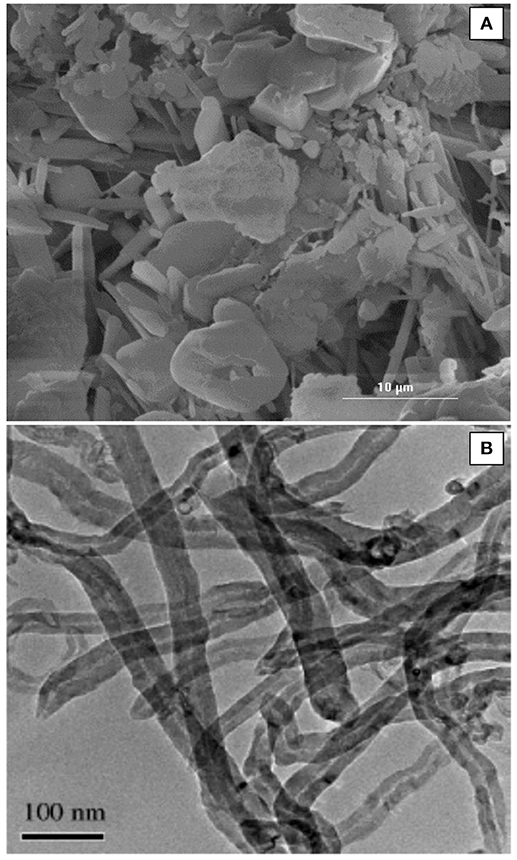
Figure 1. (A) SEM micrograph of the bentonite used and (B) TEM micrograph of the synthesized MWCNTs.
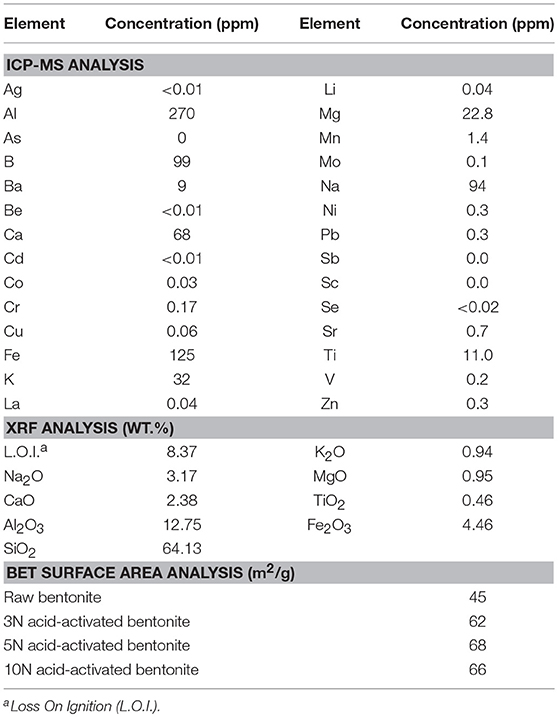
Table 1. Inductively coupled plasma mass spectrometry (ICP-MS) and X-ray fluorescence (XRF) results for the used bentonite as well as surface area data obtained using BET technique for bentonite samples activated by different concentration of H2SO4.
Characterization of the Synthesized SO3H-MWCNTs
The BET surface area of the synthesized SO3H-MWCNTs was measured at 230 m2/g, with the pore volume and diameter recorded at 0.76 cm3/g and 11 nm, respectively. This high BET surface area was because of inducing the repulsion force between SO3H and COOH groups on the MWCNTs surface (Shuit et al., 2015), The morphology of the SO3H-MWCNTs as shown by TEM are presented in Figure 1B. As it can be observed, the sidewalls of SO3H-MWCNTs are not smooth due to the oxidizing effect of HNO3. FTIR data confirmed successful functionalization of the MWCNTs with SO3H (Figure 2). As revealed in the figure, a strong peak representing the stretch vibration of the SO3H functional group anchored on the MWCNTs appeared at around 1,000–1,080 cm−1. Moreover, a broad band in the region 3,100–3,400 cm−1 was assigned to OH group caused by the purification step implemented. In addition, the strong band observed at 1,709 cm−1, and the weak band at 1,338 cm−1 could be attributed to the stretching modes of sulfate groups.
Impacts of the Synthesized Catalysts on FFA Conversion
The characteristics of the residual olive oil used in this study, i.e., FFA profiles and physicochemical properties are presented in Table 2. Respectively, the applications of 5 N acid-activated bentonite and SO3H-MWCNTs efficiently decreased the FFA content of residual olive oil to 0.43 and 0.47% that were less than the maximum acceptable value of 0.5% (Table 3). Intriguingly, original bentonite sample used as control did not show any positive impacts on FFA reduction. The results related to the FFA content reduction as determined by GC analysis (Figure 3), through their conversion to methyl esters are shown in Table 3 and Figure 4. These findings well demonstrate the catalytic activity of the acid-activated bentonite and the SO3H-MWCNTs.
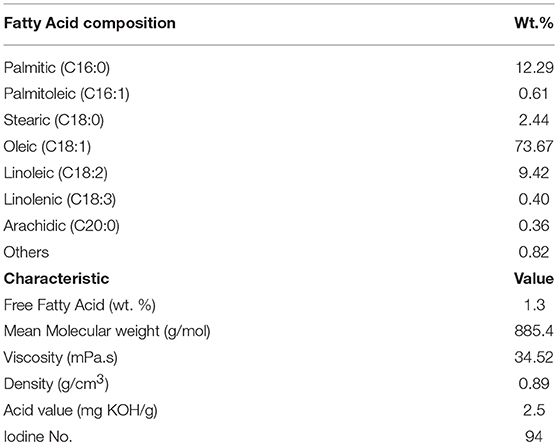
Table 2. Free fatty acid profile and physical properties of residual olive oil used in the present study.
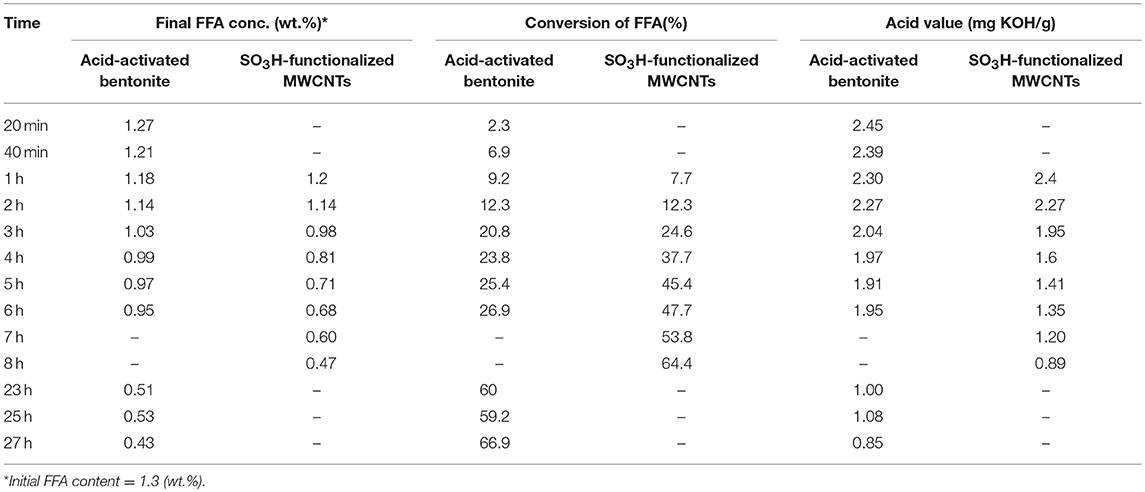
Table 3. The impact of 5 N acid-activated bentonite and SO3H-functionalized MWCNT as catalyst in the esterification reaction (methanol-to-oil molar ratio of 15:1 and 12:1, respectively), on FFA removal through its conversion into methyl esters and acid value over time.
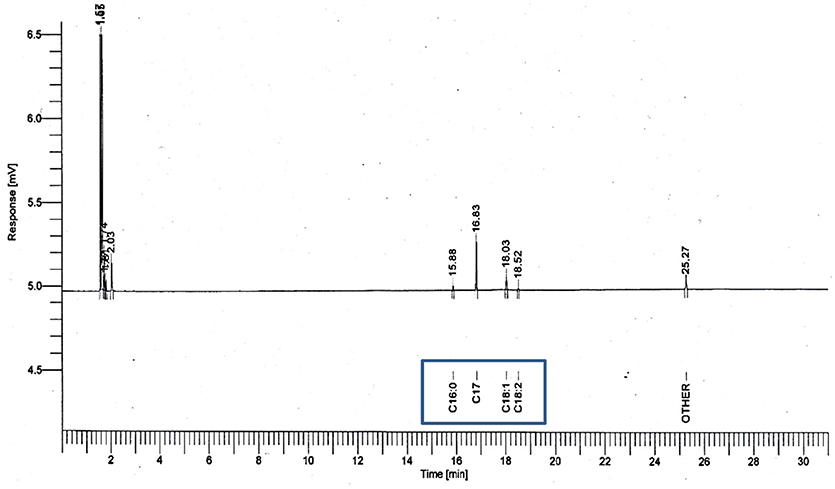
Figure 3. GC chromatograms used for calculating methyl ester yield through the esterification reaction, with 5 N acid-activated bentonite and the SO3H-MWCNTs as catalyst.
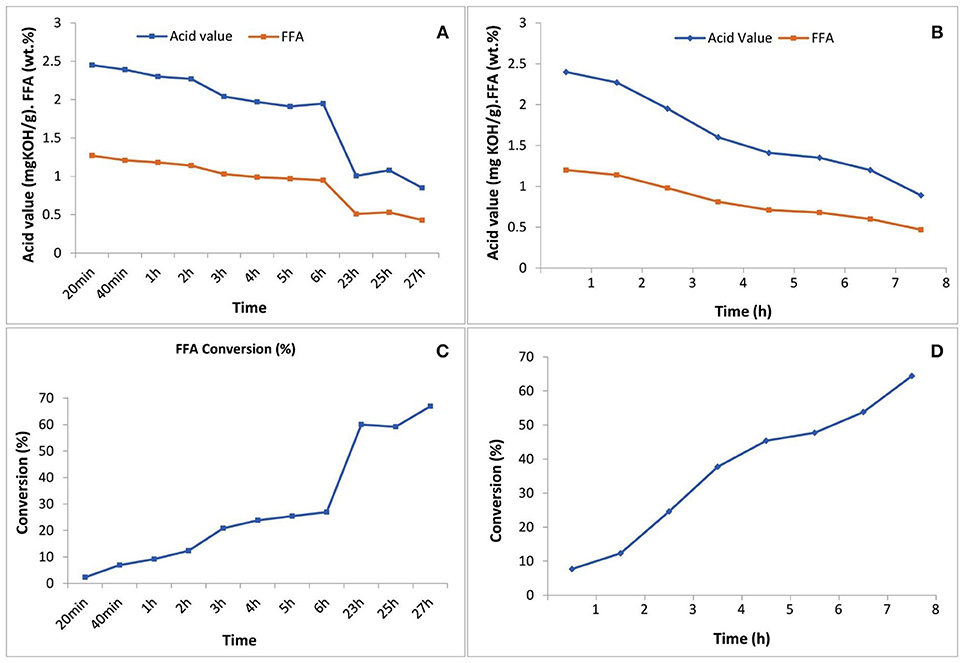
Figure 4. Acid value and FFA content reduction through the esterification reaction (A) with 5 N acid-activated bentonite and (B) with SO3H-MWCNTs, as catalyst vs. time; and diagrams of FFA conversion to methyl esters (C) using 5N acid-activated bentonite and (D) using SO3H-MWCNTs.
The acid-activated bentonite acted as a solid acid catalyst due to the hydrogen ions already anchored among its smectite's layers during the activation process. In another word, acid-activated bentonite acted as a proton donor catalyst expediting the esterification reaction. As seen in Figure 4, ~67% of the FFAs contained in the residual olive oil were converted to methyl esters within the 27 h experimental period. It is worth mentioning that a methyl ester yield of 1.3% would be achieved from 100% conversion of the total FFA. However, the total methyl ester measured was 2.06% from 67% conversion of total available FFAs. This unexpected difference implies that some of the methyl ester yield (~1.1 out of 2.06%) arose from acid-transesterification of triglycerides and not from the esterification of FFAs. This indicates that, although very minor, the active sites present on the surface of acid-activated bentonites could also attack the huge triglyceride molecules, and converted them into methyl esters.
Moreover, SO3H-MWCNTs was shown to possess favorable catalytic activity as 65% of the contained FFAs were converted, reaching the final concentration of 0.47%, in just 8 h of esterification reaction (Figure 4). From the performance point of view, SO3H-MWCNTs were found as a better choice in comparison with acid-activated bentonite, as it led to slightly higher FFA reduction within 3-fold shorter time period (Table 3). This could be ascribed to its stronger acidic functional group (SO3H).
Table 4 compares some studies in which acid-activated bentonites and SO3H-MWCNTs were used as catalysts in FFA esterification process. These studies differ in terms of the procedures used for catalyst preparation though. Shuit et al. (2015) used 10 wt.% (NH4)2SO4 solution and ultrasonication (10 min), followed by a heating step (°C, 30 min) to synthesize SO3H-MWCNTs for biodiesel production. This process was more advantageous than the procedure used in the present study in terms of synthesis time and being H2SO4 free; however, about 2.1-time higher temperature was required. Moreover, the synthesized SO3H-MWCNTs had very lower surface area (92.37 vs. 230 m2/g, respecively) and pore volume (0.25 vs. 0.76 cm3/g, respectiely) making it a less efficient catalyst. The acid-activated bentonite produced herein showed about 62% higher surface area than that prepared by Jeenpadiphat and Tungasmita (2014). Both catalysts prepared in the current study, especially SO3H-MWCNTs, could decrease mass transfer limitation due to their high surface area. Nevertheless, and despite their characteristic advantages, lower FFA conversion yields were obtained by these catalysts in comparison with those listed in Table 4. This could be attributed to the fact that esterification process was not optimized in this study. Instead, a common mild-esterification reaction was targeted to only evaluate the catalytic performance of prepared catalysts.
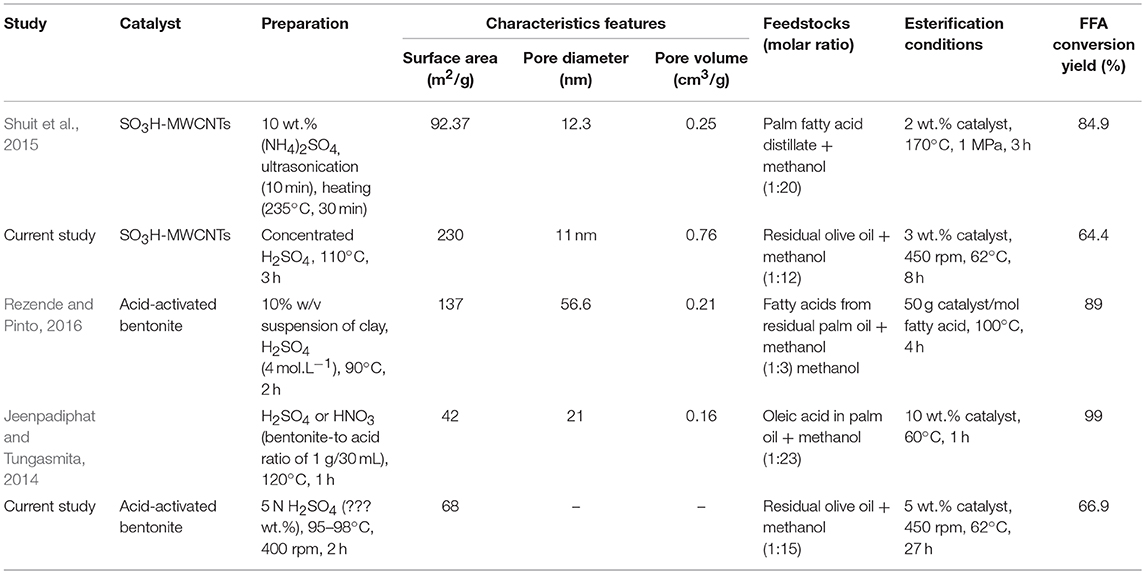
Table 4. Comparison of some studies in which acid-activated bentonites and SO3H-functionlized multiwall carbon nanotubes (MWCNT) were used as catalysts in FFA esterification process.
Concluding Remarks and Future Prospects
This study showed that bentonite could be applied as an efficient raw material for synthesizing solid acid catalyst, through a simple chemical (i.e., acid) activation method, for using in solid acid catalyzed-esterification of high FFA oils. The application of this natural clay may encourage the production of sustainable fuels, as there is no requirement for sophisticated methods, and environmental hazardous chemicals as well. Acid activation of benonite using 5 N H2SO4 was found to considerably improve its acidity as well as its specific surface area (more than half-time), and in turn, its catalytic activity for FFA content reduction of residual olive oil to <0.5 wt.%. It should also be noted that bentonite is very abundant and cheap (<20 USD/ton) and therefore, 5 N acid-activated bentonite could serve as a promising pre-treatment process for esterification of low-quality oil feedstock prior to alkali-catalyzed transesterification. Moreover, SO3H-MWCNTs were also synthesized and their catalytic performance was characterized. It showed excellent specific surface area (230 m2/g), and good pore diameter (11 nm) and pore volume (0.76 cm3/g), enhancing mass transfer of reaction. Compared with the acid-activated bentonite, SO3H-MWCNTs provided better catalytic performance. Despite more favorable economic preparation of the 5 N acid-activated bentonite and its relatively similar performance in terms of FFA reduction with the SO3H-MWCNTs, the latter catalyst proved more feasible for industrial application. This feasibility can be attributed to its considerably shorter reaction time due to more than 3.3 times higher specific surface area, significantly lowering mass transfer limitation. Further optimization of the esterification reaction conditions would be necessary for full exploitation of these two catalysts for FFA conversion into biodiesel. Overall, both of the prepared catalysts could be used for effective pretreatment of low-quality residual olive oil for sustainable biodiesel production under a biorefinery scheme.
Author Contributions
HR performed the bentonite catalyst production, SG and MM assisted with GC analyses, MeA assisted with some characterizations, MT and AR developed the idea and led the research. MT, MoA, and A-SN wrote the manuscript. HP as of substantial help during the revision process.
Conflict of Interest Statement
The authors declare that the research was conducted in the absence of any commercial or financial relationships that could be construed as a potential conflict of interest.
Acknowledgments
We are thankful to Biofuel Research Team (BRTeam) for supporting this study.
Abbreviations
BET, Brunauer, Emmett and Teller; CEC, Cation exchange capacity; CNT, Carbon nanotube; CVD, Chemical vapor deposition, FFA, Free fatty acid; FTIR, Fourier-Transform Infrared Spectroscopy; ICP-MS, Inductively Coupled Plasma mass spectrometry; SEM, Scanning Electron Microscopy, SO3H-MWCNT, SO3H-functionlized multiwall carbon nanotube, TEM, Transmission Electron Microscopy; XRF, X-ray fluorescence.
References
Aghbashlo, M., Hosseinpour, S., Tabatabaei, M., and Dadak, A. (2017a). Fuzzy modeling and optimization of the synthesis of biodiesel from waste cooking oil (WCO) by a low power, high frequency piezo-ultrasonic reactor. Energy 132, 65–78. doi: 10.1016/j.energy.2017.05.041
Aghbashlo, M., Hosseinpour, S., Tabatabaei, M., and Soufiyan, M. M. (2019). Multi-objective exergetic and technical optimization of a piezoelectric ultrasonic reactor applied to synthesize biodiesel from waste cooking oil (WCO) using soft computing techniques. Fuel 235, 100–112. doi: 10.1016/j.fuel.2018.07.095
Aghbashlo, M., Tabatabaei, M., and Hosseinpour, S. (2018a). On the exergoeconomic and exergoenvironmental evaluation and optimization of biodiesel synthesis from waste cooking oil (WCO) using a low power, high frequency ultrasonic reactor. Energy Convers. Manage. 164, 385–398. doi: 10.1016/j.enconman.2018.02.086
Aghbashlo, M., Tabatabaei, M., Jazini, H., and Ghaziaskar, H. S. (2018b). Exergoeconomic and exergoenvironmental co-optimization of continuous fuel additives (acetins) synthesis from glycerol esterification with acetic acid using Amberlyst 36 catalyst. Energy Convers. Manage. 165, 183–194. doi: 10.1016/j.enconman.2018.03.054
Aghbashlo, M., Tabatabaei, M., Mohammadi, P., Khoshnevisan, B., Rajaeifar, M. A., and Pakzad, M. (2017b). Neat diesel beats waste-oriented biodiesel from the exergoeconomic and exergoenvironmental point of views. Energy Convers. Manage. 148, 1–15. doi: 10.1016/j.enconman.2017.05.048
Aghbashlo, M., Tabatabaei, M., Mohammadi, P., Pourvosoughi, N., Nikbakht, A. M., and Goli, S. A. H. (2015). Improving exergetic and sustainability parameters of a DI diesel engine using polymer waste dissolved in biodiesel as a novel diesel additive. Energy Convers. Manage. 105, 328–337. doi: 10.1016/j.enconman.2015.07.075
Aghbashlo, M., Tabatabaei, M., Rastegari, H., Ghaziaskar, H. S., and Shojaei, T. R. (2018c). On the exergetic optimization of solketalacetin synthesis as a green fuel additive through ketalization of glycerol-derived monoacetin with acetone. Renew. Energy 126, 242–253. doi: 10.1016/j.renene.2018.03.047
Christidis, G. (2013). “Assessment of industrial clays,” in Handbook of Clay Science. Part B. Techniques and Applications, eds F. Bergaya and G. Lagaly (Amsterdam: Elsevier), 425–449.
Copetti, D., Finsterle, K., Marziali, L., Stefani, F., Tartari, G., Douglas, G., et al. (2016). Eutrophication management in surface waters using lanthanum modified bentonite: a review. Water Res. 97, 162–174. doi: 10.1016/j.watres.2015.11.056
Dokic, M., Kesic, Z., Krstic, J., Jovanovic, D., and Skala, D. (2012). Decrease of free fatty acid content in vegetable oil using silica supported ferric sulfate catalyst. Fuel 97, 595–602. doi: 10.1016/j.fuel.2012.03.039
El Korashy, S. A., Elwakeel, K. Z., and El Hafeiz, A. A. (2016). Fabrication of bentonite/thiourea-formaldehyde composite material for Pb (II), Mn (VII) and Cr (VI) sorption: a combined basic study and industrial application. J. Cleaner Prod. 137, 40–50. doi: 10.1016/j.jclepro.2016.07.073
Haas, M. J., McAloon, A. J., Yee, W. C., and Foglia, T. A. (2006). A process model to estimate biodiesel production costs. Bioresour. Tech. 97, 671–678. doi: 10.1016/j.biortech.2005.03.039
Hajjari, M., Tabatabaei, M., Aghbashlo, M., and Ghanavati, H. (2017). A review on the prospects of sustainable biodiesel production: a global scenario with an emphasis on waste-oil biodiesel utilization. Renew. Sustain. Energy Rev. 72, 445–464. doi: 10.1016/j.rser.2017.01.034
Ham, H. T., Koo, C. M., Kim, S. O., Choi, Y. S., and Chung, I. J. (2004). Chemical modification of carbon nanotubes and preparation of polystyrene/carbon nanotubes composites. Macromol. Res. 12, 384–390. doi: 10.1007/BF03218416
Hasheminejad, M., Tabatabaei, M., Mansourpanah, Y., and Javani, A. (2011). Upstream and downstream strategies to economize biodiesel production. Bioresour. Tech. 102, 461–468. doi: 10.1016/j.biortech.2010.09.094
Hayyan, A., Alam, M. Z., Mirghani, M. E., Kabbashi, N. A., Hakimi, N. I. N. M., Siran, Y. M., et al. (2010). Sludge palm oil as a renewable raw material for biodiesel production by two-step processes. Bioresour. Tech. 101, 7804–7811. doi: 10.1016/j.biortech.2010.05.045
Jeenpadiphat, S., and Tungasmita, D. N. (2014). Esterification of oleic acid and high acid content palm oil over an acid-activated bentonite catalyst. Appl. Clay Sci. 87, 272–277. doi: 10.1016/j.clay.2013.11.025
Kalamkarov, A. L., Georgiades, A., Rokkam, S., Veedu, V., and Ghasemi Nejhad, M. (2006). Analytical and numerical techniques to predict carbon nanotubes properties. Int. J. Solids Struct. 43, 6832–6854. doi: 10.1016/j.ijsolstr.2006.02.009
Montefrio, M. J., Xinwen, T., and Obbard, J. P. (2010). Recovery and pre-treatment of fats, oil and grease from grease interceptors for biodiesel production. Appl. Energy 87, 3155–3161. doi: 10.1016/j.apenergy.2010.04.011
Murad, P. C., Hamerski, F., Corazza, M. L., Luz, L. F., and Voll, F. A. (2018). Acid-catalyzed esterification of free fatty acids with ethanol: an assessment of acid oil pretreatment, kinetic modeling and simulation. React. Kinet. Mech. Catal. 123, 505–515. doi: 10.1007/s11144-017-1335-3
Önal, M. (2006). Physicochemical properties of bentonite: an overview. Commun. Fac. Sci. Univ. Ankara Seri. B 52, 7–21. doi: 10.1501/Commub_0000000349
Önal, M., and Sarıkaya, Y. (2007). Preparation and characterization of acid-activated bentonite powders. Powder Technol. 172, 14–18. doi: 10.1016/j.powtec.2006.10.034
Pan, H., Zhang, H., and Yang, S. (2017). “Production of Biodiesel via Simultaneous Esterification and Transesterification,” in Production of Biofuels and Chemicals with Bifunctional Catalysts, eds Z. Fang, J.R. Smith, and H. Li (Singapore: Springer), 307–326. doi: 10.1007/978-981-10-5137-1_10
Photaworn, S., Tongurai, C., and Kungsanunt, S. (2017). Process development of two-step esterification plus catalyst solution recycling on waste vegetable oil possessing high free fatty acid. Chem. Eng. Process. 118, 1–8. doi: 10.1016/j.cep.2017.04.013
Pütün, A. E., Uzun, B. B., Apaydin, E., and Pütün, E. (2005). Bio-oil from olive oil industry wastes: pyrolysis of olive residue under different conditions. Fuel Process. Tech. 87, 25–32. doi: 10.1016/j.fuproc.2005.04.003
Rashidi, A. M., Akbarnejad, M. M., Khodadadi, A. A., Mortazavi, Y., and Ahmadpourd, A. (2007). Single-wall carbon nanotubes synthesized using organic additives to Co-Mo catalysts supported on nanoporous MgO. Nanotechnology 18:315605. doi: 10.1088/0957-4484/18/31/315605
Rezende, M. J., and Pinto, A. C. (2016). Esterification of fatty acids using acid-activated Brazilian smectite natural clay as a catalyst. Renew. Energy 92, 171–177. doi: 10.1016/j.renene.2016.02.004
Sahafi, S. M., Ahmadibeni, A., Talebi, A. F., Goli, S. A. H., Aghbashlo, M., and Tabatabaei, M. (2018). Seed oils of Sisymbrium irio and Sisymbrium sophia as a potential non-edible feedstock for biodiesel production. Biofuels 1–9. doi: 10.1080/17597269.2018.1457315
Shen, Y. H. (2001). Preparations of organobentonite using nonionic surfactants. Chemosphere 44, 989–995. doi: 10.1016/S0045-6535(00)00564-6
Shu, Q., Nawaz, Z., Gao, J., Liao, Y., Zhang, Q., Wang, D., et al. (2010). Synthesis of biodiesel from a model waste oil feedstock using a carbon-based solid acid catalyst: reaction and separation. Bioresour. Technol. 101, 5374–5384. doi: 10.1016/j.biortech.2010.02.050
Keywords: acid-activated bentonite, SO3H-functionlized multiwall carbon nanotube (SO3H-MWCNTs), waste olive oil, high free fatty acid, biodiesel production, biorefinery
Citation: Rahimzadeh H, Tabatabaei M, Aghbashlo M, Panahi HKS, Rashidi A, Goli SAH, Mostafaei M, Ardjmand M and Nizami A-S (2018) Potential of Acid-Activated Bentonite and SO3H-Functionalized MWCNTs for Biodiesel Production From Residual Olive Oil Under Biorefinery Scheme. Front. Energy Res. 6:137. doi: 10.3389/fenrg.2018.00137
Received: 22 September 2018; Accepted: 28 November 2018;
Published: 17 December 2018.
Edited by:
Saurabh Dhiman, South Dakota School of Mines and Technology, United StatesReviewed by:
Jaime Puna, Instituto Superior de Engenharia de Lisboa, PortugalKonstantinos Moustakas, National Technical University of Athens, Greece
Copyright © 2018 Rahimzadeh, Tabatabaei, Aghbashlo, Panahi, Rashidi, Goli, Mostafaei, Ardjmand and Nizami. This is an open-access article distributed under the terms of the Creative Commons Attribution License (CC BY). The use, distribution or reproduction in other forums is permitted, provided the original author(s) and the copyright owner(s) are credited and that the original publication in this journal is cited, in accordance with accepted academic practice. No use, distribution or reproduction is permitted which does not comply with these terms.
*Correspondence: Meisam Tabatabaei, meisam_tab@yahoo.com; meisam_tabatabaei@abrii.ac.ir
Mortaza Aghbashlo, maghbashlo@ut.ac.ir