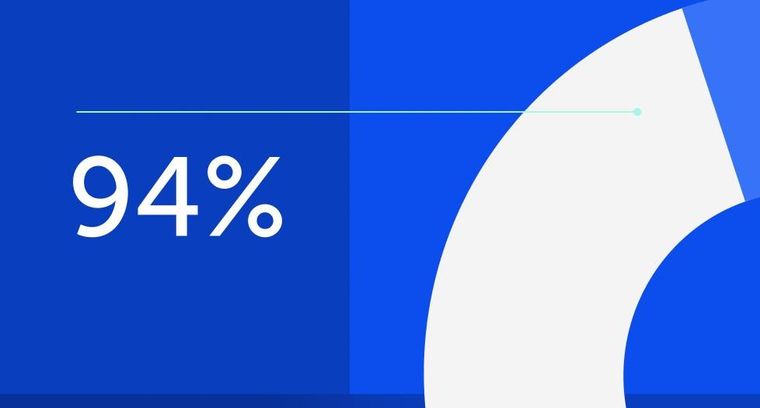
94% of researchers rate our articles as excellent or good
Learn more about the work of our research integrity team to safeguard the quality of each article we publish.
Find out more
SYSTEMATIC REVIEW article
Front. Endocrinol., 23 September 2020
Sec. Translational and Clinical Endocrinology
Volume 11 - 2020 | https://doi.org/10.3389/fendo.2020.573891
This article is part of the Research TopicEmerging Roles of the Gut Microbiota in the Pathogenesis of Metabolic DisordersView all 11 articles
Background: As growing evidence links gut microbiota with the therapeutic efficacy and side effects of anti-hyperglycemic drugs, this article aims to provide a systematic review of the reciprocal interactions between anti-hyperglycemic drugs and gut microbiota taxa, which underlie the effect of the gut microbiome on diabetic control via bug-host interactions.
Method: We followed the PRISMA requirements to perform a systematic review on human vs. animal gut microbiota data in PubMed, SCOPUS, and EMBASE databases, and used Cochrane, ROBIN-I, and SYRCLE tools to assess potential bias risks. The outcomes of assessment were trends on gut microbiota taxa, diversity, and associations with metabolic control (e.g., glucose, lipid) following anti-hyperglycemic treatment.
Results: Of 2,804 citations, 64 studies (17/humans; 47/mice) were included. In human studies, seven were randomized trials using metformin or acarbose in obese, pre-diabetes, and type 2 diabetes (T2D) patients. Treatment of pre-diabetes and newly diagnosed T2D patients with metformin or acarbose was associated with decreases in genus of Bacteroides, accompanied by increases in both Bifidobacterium and Lactobacillus. Additionally, T2D patients receiving metformin showed increases in various taxa of the order Enterobacteriales and the species Akkermansia muciniphila. Of seven studies with significant differences in beta-diversity, the incremental specific taxa were associated with the improvement of glucose and lipid profiles. In mice, the effects of metformin on A. muciniphila were similar, but an inverse association with Bacteroides was reported. Animal studies on other anti-hyperglycemic drugs, however, showed substantial variations in results.
Conclusions: The changes in specific taxa and β-diversity of gut microbiota were associated with metformin and acarbose in humans while pertinent information for other anti-hyperglycemic drugs could only be obtained in rodent studies. Further human studies on anti-hyperglycemic drugs other than metformin and acarbose are needed to explore gut microbiota's role in their therapeutic efficacies and side effects.
Gut microbiota plays a pivotal role in the pathogenesis of diabetes as significant alterations were found in the gut microbiome composition in type 2 diabetes (T2D) patients relative to healthy individuals (1). A metagenome-wide association study reported a moderate degree of dysbiosis associated with depletion in butyrate-producing bacteria, accompanied by increases in opportunistic pathogens among diabetic patients (2). These changes were echoed by a recent systematic review, which shows an inverse association of T2D with the genera Bifidobacterium, Akkermansia and butyrate-producing bacteria (e.g., Roseburia, Faecalibacterium), in conjunction with a positive association with Ruminococcus, Fusobacterium, and Blautia (1).
From a clinical perspective, these findings provide a rationale for targeting gut microbiota imbalance as a potential strategy for T2D treatment by restoring a healthy gut microbiome, including fecal microbiota transplant and probiotic supplements (3, 4). However, the efficiency and effectiveness of these treatments remain uncertain due to concerns over the invasive nature of fecal microbiota transplant and the dosage, species, and duration required for an effective probiotic treatment. Emerging evidence indicates that the therapeutic efficacy of anti-hyperglycemic drugs might, in part, be attributable to their ability to modulate the compositions of gut microbiota (1, 3, 5–9). This compositional change might lead to enrichments in bacterial species exhibiting beneficial effects to intestinal health via the production of health-promoting metabolites, such as short-chain fatty acids (SCFAs) and bile acids (8). Nevertheless, certain anti-hyperglycemic drugs were reported to cause increases in the abundance of Escherichia and Candidatus Arthromitus, which contribute to gastrointestinal side effects and weight gain, respectively (9–11).
Among various anti-hyperglycemic drugs in clinical use, metformin, acarbose, sitagliptin, and vildagliptin (5, 6) have been investigated for their reciprocal interplay with gut microbiota by assessing their effects on human and animal gut microbiota, and vice versa (1, 8, 11). From a translational perspective, animal models might help to explore the causality of complex host-microbiota interactions and possible mechanisms of action in a controlled experimental setting. However, it should be noted that differences in dietary habits, host metabolism, inflammatory states, and body anatomy contribute to great variations in gut microbiota compositions between humans and animals, and subsequently, the respective drug effects in disease control (12). A meta-analysis of published 16S rDNA sequencing data from mouse and human fecal microbiota showed that there were significant increases in Lactobacillus and Turicibacter genera in mouse gut microbiota while the genera of Streptococcus, Ruminococcus, Lachnospira, Faecalibacterium, Dialister, and Oscillospira were elevated in human gut microbiota (12). Moreover, age, mouse strains/populations, microbiota pools in laboratories, and other practical factors might have varied to a great extent among different studies (12). Previous reviews have suggested the effects of anti-hyperglycemic drugs on gut microbiota (8, 11, 13), however, the differences in results between human and animal studies have not been differentiated.
Reciprocal interplays between individual anti-hyperglycemic drugs and gut microbiota remain unexplored with respect to the contribution of specific bacterial taxa to drug's therapeutic efficacy in disease control (i.e., the clinical question). Thus, we conducted this systematic review aiming to shed light on the associations among anti-hyperglycemic agents, changes in specific taxonomic groups of gut microbiota, and host glucose control or metabolic profiles mainly in humans, as compared to those reported in animal studies.
Our literature search strategies were designed to integrate the following PICOS (population, intervention, comparisons, outcomes, study design) based on the prior clinical question: Population: humans (e.g., healthy people or patients who were either obese, prediabetes, diabetes) or the corresponding animal models; Intervention: non-insulin anti-hyperglycemic drugs; Comparisons: post- vs. pre-intervention, with-vs. without-treatment, or on-vs. off-treatment; Outcomes: alteration of the gut microbial composition; Study design: clinical trials, observational studies or animal experiments, as that recommended for systematic reviews (14). We systematically searched PubMed, EMBASE, and SCOPUS databases from January 1, 2000, to November 13, 2019. The keywords and searching strategies based upon the PICOS were “anti-hyperglycemic drugs” and “gut microbiota” related terms (Supplementary Table 1). In addition, we searched manually the reference list of the review papers for additional publications of interest.
We followed the preferred reporting items for systematic reviews and meta-analysis (PRISMA) guidelines (15). The inclusion criteria for the published studies included: (i) any human studies or animal experiments reporting original data of gut microbiota after receiving anti-hyperglycemic drugs; (ii) gut microbiota data were analyzed from feces or colonic content specimens; (iii) must be written in English or Chinese. Studies were excluded if they did not provide data of individual bacterial taxa or were only available as conference abstracts or proceedings.
Initially, the abstracts and titles of potential articles were screened, followed by the evaluation of the full-text articles for eligibility. Two authors were responsible for screening and evaluating these papers independently. Disagreements were resolved by consensus between these two authors and, if necessary, discussed by additional two authors.
A standardized form in a Microsoft Excel file (e.g., characteristics of studies, participants, treatments and comparisons, methods to analyze the microbiome, and measures of outcomes) was used for data extraction. Data were extracted by one author and reviewed by a second one. All disagreements were resolved by consensus and a third or fourth author when necessary.
We used the Cochrane risk-of-bias tool to assess the risk of bias in selected randomized trials (16). For quasi-experimental and observational studies, we used the Risk of Bias in Non-randomized studies-of Interventions (ROBIN-I) to assess the risk of bias (17). Further, the SYRCLE's risk of bias tool for animal studies (18) was used to assess the risk of bias. The risks of bias data were extracted by four different authors and all disagreements were resolved by consensus made by the remaining authors.
Other than describing the characteristics of the evaluated human or animal studies, the primary outcome was the difference in relative abundance or change patterns of individual intestinal bacterial taxa, categorized based on six common taxonomic categories [Phylum (P)c, Class (C), Order (O), Family (F), Genus (G), Species (S)], in associations with the use of anti-hyperglycemic agents, among those available human or animal studies, respectively. Secondary outcomes were differences in microbial diversity, changes in intestinal or serum levels of SCFAs and/or bile acids in human or animal hosts, respectively, after taking/using individual drugs, associations between specific taxa and host metabolic parameters, e.g., glucose, body weight, and lipid profile.
We classified the primary and secondary outcomes into the following categories: significant increase, significant decrease, and no significant difference between comparison groups. Changes of each taxon were synthesized from at least 2 studies for human or animal studies, respectively. Specifically, the effects of different anti-hyperglycemic drugs on specific taxon among the evaluated human or animal studies were compared. Further, the corresponding effects of each individual drug on specific taxon were compared to explore its consistency, in terms of having the same trend of alteration on the specific taxon caused by the same specific anti-hyperglycemic drug, or not. These findings also were categorized by the target research populations (e.g., obese, pre-diabetic, newly T2D, prevalent T2D), individual treated drugs or different animal models (mice or rat models with various diets or genetic knockout). For gut microbial diversity, each study might use one or more measures to assess α- (richness and evenness) or β-diversity. We considered α-diversity as “Increase” if at least one measure showed an increase and no measure showed a decrease; “Decrease” if at least one measure showed a decrease and no measure showed an increase; “No difference” if all measures showed no difference. β-diversity was assessed as “Difference” if at least one measure showed a difference; “No difference” if all measures showed no difference. In terms of associations between specific taxa and host metabolic parameters, we collected data from specific taxa that increased or decreased significantly in participants receiving non-insulin anti-hyperglycemic drugs. Only data with statistical significance were extracted for analyses.
The following presented results were mainly focused on human studies, which are compared to those of animal studies.
Overall, 2,804 citations were retrieved, and the final analysis included 17 human studies (7, 19–34) and 47 mouse studies (5, 6, 35–79) from 64 papers (Figure 1). The majority of studies were published in and after 2017 and the duration of anti-hyperglycemic treatments varied from studies to studies (i.e., from few days to several months). Of 17 human studies, seven (41.2%) were randomized control trials (7, 19–21, 32–34). Thirteen studies (76.4%) enrolled either newly diagnosed or prevalent T2D patients (20, 21, 23, 24, 26–34), whereas the remaining four studies enlisted healthy participants (22, 25), obese individuals (19), and pre-diabetic patients (7) (Tables 1, 2).
Of 47 rodent studies, 30 (63.8%) studies were conducted in mice (35–41, 44–46, 48, 52, 53, 55, 56, 59–65, 68, 70, 74–79) and the others were in rats (5, 6, 42, 43, 47, 49–51, 54, 57, 58, 66, 67, 69, 71–73). Their characteristics, housing, acclimatization, and diet treatments were presented in Table 3 and Supplementary Table 2. Overall, 14 anti-hyperglycemic agents were used in these included studies, with metformin accounting for the most. There was only one human study focused on glipizide, while the other ten listed drugs (e.g., voglibose, miglitol, vildagliptin, sitagliptin, saxagliptin) were used only in rodent studies (Table 1).
Fecal specimens of all human studies were analyzed for the composition of gut microbiota. Of 47 mouse studies, 34 studies (72.3%) used fecal samples (5, 6, 35, 36, 38, 41, 42, 44–46, 49–56, 59–61, 63–73, 76, 78). 16S rRNA gene sequencing was the most common method used in human and animal studies (Table 1).
Among human studies, three randomized studies were at the high risk of bias in performance, detection, and attrition, while four studies were unclear risks in most domains (Supplementary Figure 1). Among quasi-experimental studies, three studies were at low risk of bias in all domains, whereas two studies were at serious risk in confounding, selection of participants, and classification of interventions (Supplementary Figure 2). All 5 cross-sectional studies were at serious risk in several domains, e.g., confounding, selection of participants, classification of intervention (Supplementary Figure 3). Almost all mouse studies were unclear risk across domains, even if some were at low risk of bias in selective outcome reporting (Supplementary Figure 4).
Importantly, the synthesized results from animal studies reported all common six taxonomic categories (P, C, O, F, G, S) of bacterial taxa but there were only three common taxonomic categories (F, G, S) of gut microbiota taxa were reported in those from human studies based on the available data (Tables 4–7). Glipizide and liraglutide were assessed in a single human study. No differences were found in patients treated with glipizide (32), while Wang et al. (34) found the association between liraglutide treatment and the increased abundance of genus Akkermansia in T2D patients. The assessments of the effects of metformin and acarbose on the human gut microbiota composition represented the foci of 14 studies (19–31, 34) and three studies (7, 32, 33), respectively (Tables 4, 5).
Table 4. Effects of anti-hyperglycemic drugs on specific taxa in human gut microbiota, categorized by the target research populationsa.
Table 5. Consistent and inconsistent effects of each anti-hyperglycemic drug on specific taxa in human gut microbiota, categorized by the target research populationsa.
Of the phylum Bacteroidetes, the genus Bacteroides decreased in two studies treated with metformin among newly diagnosed T2D patients (20, 24), and in two studies treated with acarbose among pre-diabetic and newly diagnosed T2D patients (7, 32). Additionally, one study treated with metformin (24) and one study treated with acarbose (32) in newly diagnosed T2D patients reported similar results of decreases in seven species (e.g., Bacteroides dorei, Bacteroides finegoldii). For the phylum Firmicutes, the genus Lactobacillus increased in two studies in pre-diabetic (7) and newly diagnosed T2D patients (32) receiving acarbose, and the species L. gasseri increased in one study treated with metformin (21), and with acarbose (32), respectively, among newly diagnosed T2D patients. Meanwhile, the genus Clostridium decreased in one study among healthy participants receiving metformin (22) which was also reported in newly diagnosed T2D patients treated with acarbose (32) (Table 4). Two species, i.e., C. bartlettii and C. botulinum, consistently decreased among T2D patients receiving metformin in two separate studies (21, 31). Three out of four studies showed a decrease in the genus Intestinibacter (21, 22, 30) among healthy participants and T2D patients treated with metformin (Table 5). With respect to the phylum Actinobacteria, the genus Bifidobacterium with the species B. adolescentis increased in one study with metformin (21) and another study with acarbose (32) among newly diagnosed T2D patients (Table 4), and B. longum consistently increased among T2D patients treated with acarbose in two studies (32, 33) (Table 5).
Concerning other phyla, two studies evaluated the genus Fusobacterium (phylum Fusobacteria) (20, 27) and the species Akkermansia muciniphila (phylum Verrucomicrobia) (21, 29) among T2D patients treated with metformin. Both showed increases in the abundance of these two taxa. In the phylum Proteobacteria, conflicting results were reported in two studies with respect to the family Enterobacteriaceae in healthy participants vs. T2D patients treated with metformin (25, 27). Overall, metformin might increase different taxa from the family Enterobacteriaceae and other families in the order of Enterobacteriales. Of six studies that evaluated the genus Escherichia (19–22, 25, 30) and four studies evaluating the genus Shigella (19, 20, 22, 25), the treatment of healthy participants, obese individuals, and T2D patients with metformin all led to increased abundance of these two genera. Another two studies on metformin in newly diagnosed and prevalent T2D patients (21, 31) reported consistently increases in eight species of the order Enterobacteriales, including Citrobacter koseri, Escherichia coli, Klebsiella pneumonia (family Enterobacteriaceae), Erwinia amylovora (family Erviniaceae), Pectobacterium wasabiae, and Dickeya dadantii (family Pectobacteriaceae) (Table 5).
The effects of 13 anti-hyperglycemic drugs on the compositions of gut microbiota were conducted in different rodent models. The results of pioglitazone was inconclusive (78), while the other 12 drugs were presented in Tables 6, 7. The Firmicutes/Bacteroidetes ratios were decreased in two studies treated with metformin in high fat diet (HDF)-fed mice (37, 48). This decrease was also noted in another two studies, in which liraglutide was given to in rat models induced by diets and gene knockout (66, 69).
Table 6. Effects of anti-hyperglycemic drugs on gut microbiota in mouse models, categorized by the treated, individual anti-hyperglycemic drug.
Table 7. Consistent and inconsistent effects of each anti-hyperglycemic drug on specific taxa in mouse gut microbiota, categorized by mice or rat models with three distinct animal models.
Responses of the phylum Bacteroidetes to different anti-hyperglycemic agents were investigated in 15 rodent studies. No difference was noted in studies treated with acarbose (5, 58). Five out of the seven studies on the genus Bacteroides after using metformin (37, 42, 48, 54, 56) revealed increased abundance of this genus in mice and rats.
Among 15 rodent studies (5, 6, 36, 37, 41, 47, 48, 54, 56, 58, 66, 69, 70, 73, 75) on the phylum Firmicutes, the results were inconclusive among those treated with metformin (5, 36, 37, 41, 47, 48, 54, 56), acarbose (5, 58), liraglutide (66, 69, 70), and sitagliptin (5, 73). The genus Lactobacillus was the focus of 13 studies (5, 35, 41–43, 47, 53, 54, 58, 62, 66, 70, 73). Six out of the eight studies treated with metformin (5, 35, 43, 53, 54, 62) saw an increase in this genus in mice and rats, while the results in studies treated with acarbose (5, 58, 62), liraglutide (66, 70), and sitagliptin (5, 73) were inconclusive.
With respect to other phyla, there was a trend of decrease in the phylum Proteobacteria in mice treated with metformin and liraglutide, while Verrucomicrobia and Tenericutes increased after treated with metformin and liraglutide, respectively. However, results for the phyla Actinobacteria, Cyanobacteria, Elusimicrobia, and Fusobacteria were conflicting. The genus Akkermanisa (phylum Verrucomicrobia) increased in eight studies treated with metformin using dietary or genetic models (35, 37, 38, 41, 44, 48, 54, 56). Three of the four studies with metformin (41, 53, 55) reported an increase in the species A. muciniphila, and another two studies reported a similar increase in this species after treating with liraglutide (65, 68).
Ten human studies treated with metformin (19, 20, 22–25, 27–30) and two studies treated with acarbose (7, 32) have provided the results of α-diversity. The results from those metformin studies, however, were conflicting, while both acarbose studies reported a decrease in the α-diversity among pre-diabetic and T2D patients. β-diversity was assessed in ten studies treated with metformin (19–22, 24, 25, 27–29, 34), of which six studies (20–22, 24, 27, 34) revealed a significant difference after the treatment in healthy participants and T2D patients. This difference was also noted in pre-diabetic patients treated with acarbose (32), and T2D patients treated with liraglutide (34) (Table 8).
Similar results with metformin and acarbose were reported in mouse studies. The effects of metformin on α-diversity were conflicting across different models, while the α-diversity decreased consistently in three studies treated with acarbose (5, 58, 59). Moreover, the results were inconsistent among those studies treated with liraglutide, sitagliptin, vildagliptin, and saxagliptin. In terms of β-diversity, there was higher cumulative evidence of significant difference after using metformin (5, 36–39, 41, 43–50, 54–56), and similar results were consistently reported among those studies treated with acarbose (5, 58–61), liraglutide (60, 65, 66, 69, 70), sitagliptin (5, 60, 73), and vildagliptin (6, 74) across different mouse models. Evidence for the effects of other drugs was limited (Table 9).
In human studies, changes in the levels of three main SCFAs (acetate, propionate, and butyrate) in feces and sera were reported in three studies treated with metformin (19, 21, 23) (Supplementary Table 3). Wu et al. (21) found that the levels of fecal butyrate and propionate increased in T2D male patients. However, no difference in fecal levels of these two SCFAs was noted among obese women in Ejtahed's study (19). In contrast, fecal acetate levels decreased in obese women (19) did not change among T2D patients in Wu's study (21). Huang et al. (23) reported that the serum levels of all three SCFAs remained unchanged after treating with metformin in T2D patients.
In mouse studies, fecal levels of SCFAs after metformin interventions were assessed in db/db mice (35) and OLETF rats (50) (Supplementary Table 3). It was found that levels of acetate and butyrate increased, but propionate levels remained unchanged. The effects of acarbose on these SCFAs levels were also assessed in dietary models (59, 61, 62). These studies showed consistent results of increased levels of butyrate in feces and ceca, but the levels of acetate and propionate varied in a diet-dependent manner.
In human studies, three clinical trials treated with metformin (21, 24, 26) and one randomized trial with acarbose and glipizide (32) were carried out in T2D patients to assess the respective effects on the fecal and serum levels of bile acids (Supplementary Table 4). Regarding metformin, one study (24) showed increases in the fecal level of conjugated secondary bile acids, while no difference was reported in the other two studies (21, 26). Two of these three studies (21, 24) reported increases in the blood level of secondary bile acids, while the other one (26) revealed an inverse trend. Concerning changes in total and primary bile acids, their levels in feces were unchanged among these three trials, but results in blood levels were conflicting (21, 26). The random trial assessing the effects of acarbose and glipizide on bile acid levels in newly diagnosed T2D patients (32) showed that acarbose might increase the plasma and fecal levels of primary bile acids, accompanied by decreases in secondary bile acids. In contrast, no significant changes in bile acid levels were found in patients treated with glipizide.
As for rodent studies, one study in rats (51) revealed that the fecal level of total bile acids increased while the levels in liver tissues were decreased after metformin intervention. One study in mice (63) found that voglibose treatment was associated with increases in serum levels of primary bile acids, accompanied by decreases in serum levels of secondary bile acids (Supplementary Table 4).
Among pre-diabetic and T2D patients treated with metformin (20, 21) or acarbose (7, 32, 33), alterations in certain specific taxa in human gut microbiota were associated with improvement in HbA1C and fasting blood glucose values, body weights, and lipid profiles (Table 10). For instance, increments in the genera Escherichia, Shigella, Subdoligranulum, and Dialister, and the species Bifidobacterium adolescentis, Bifidobacterium longum, and Lactobacillus gasseri were inversely associated with HbA1C after treating with metformin or acarbose (7, 20, 21, 32). In addition, there were inverse associations between increases in the genus Blautia and fasting blood glucose after treating with metformin (20).
Mouse studies treated with metformin (44, 50, 55, 57), liraglutide (65, 66, 70), and saxagliptin (70) also explored the relationship between changes in the compositions of gut microbiota and improvement in various metabolic parameters (Table 11). It was found that some related specific taxa after treating with metformin in mice (i.e., Bacteroides spp., Blautia) were different from that in humans.
Our study provides a comprehensive review to report human and animal data separately about reciprocal interactions between anti-hyperglycemic drugs and specific taxonomic groups of gut microbiota. While other reviews suggest the effects of anti-hyperglycemic drugs on gut microbiota without discerning findings from either human or animal studies (8, 11, 13), this systematic review attempts to fill the gap of these reviews to try to explore the associations among anti-hyperglycemic agents, specific taxonomic patterns of gut microbiota, and glucose control or metabolic profiles mainly in humans, as compared to those reported in mouse studies. Further, the fact that three-quarters of included studies were published in and after 2017 implies a growing interest in this clinical question for an up-to-date systematic review.
Of the 17 human studies selected, the majority of these studies focus on either newly diagnosed or prevalent T2D patients, and directed toward investigating the interplay of metformin, and to a lesser extent, acarbose, with gut microbiota. Our results suggest that these two drugs mediate their glucose-lowering effect, in part, by stimulating beneficial gut bacteria that could produce metabolites to promote intestinal homeostasis (3, 9). We rationalize that alterations in gut microbiota compositions might also underlie the gastrointestinal side effects known to metformin, i.e., diarrhea and fecal incontinence (10, 25, 80). In contrast, results from other anti-hyperglycemic drugs analyzed in this study showed inconsistency with respect to their effects on the compositions of gut microbiota, which might be attributable to small numbers of studies and, equally important, differences in animal models and experimental conditions used among these studies.
Further, treatment durations of anti-hyperglycemic drugs in available studies, regardless of human or animal, varied to a great extent (i.e., few days to few months). Thus, the reported drug effects on the gut microbiota structure were diverse. Indeed, the anti-hyperglycemic drugs, i.e., metformin, could affect the intestinal bacterial compositions after 1 or several days of treatment (24, 25, 40, 43), or after prolonged periods of treatments (21). For instance, Wu et al. found that gut microbiota compositions after a 2- and 4-month treatment of metformin in newly T2D patients were not identical (21). In contrast, Wang et al. did not find significant changes in the gut microbiota compositions among T2D patients after different periods of metformin or liraglutide treatment, given their baseline gut microbiota compositions were unknown (34). Thus, there are no consistent findings on gut microbiota after various treatment durations of anti-hyperglycemic drugs, and further studies are warranted to explore the treatment duration of anti-hyperglycemics required for emergence of beneficial gut bacteria.
Evidence indicates that the use of metformin or acarbose in T2D patients was associated with increases in the abundance of beneficial bacteria, including the genera Bifidobacterium (phylum Actinobacteria) and Lactobacillus (phylum Firmicutes), and the species A. muciniphila (phylum Verrucomicrobia). The increase in the genus Bifidobacterium was positively associated with diabetes control, which is consistent with that reported in the review by Gurung et al. (1). In addition, two included studies showed an increase in two specific species of the genus Bifidobacterium (B. adolescentis and B. longum), which was inversely associated with HbA1C levels or body weights, and positively associated with HDL cholesterol levels among newly diagnosed T2D patients (21, 32).
A number of human studies have reported positive associations between the abundance of the genus Lactobacillus (phylum Firmicutes) and improved T2D control (81, 82). For example, T2D patients treated with acarbose showed increased L. gasseri levels, accompanied by lower HbA1C and body weights (32). In addition, as several species in the genus Lactobacillus have been used as probiotics, administration of these Lactobacillus strains showed beneficial effects on glycemic control and lipid profiles in T2D patients (4). Moreover, almost all animal studies that tested the efficacy of several species from the genus as probiotics for T2D reported improvements of glucose parameters (1).
A previous report found decreased abundance in the mucin-degrading bacterium A. municiphila in patients with metabolic disorders, including obesity, impaired glucose tolerance, and diabetes, which were associated with insulin resistance, dyslipidemia, and overweight (83). Two other studies showed increased amounts of A. municiphila in newly diagnosed and prevalent T2D patients treated with metformin, which, however, did not provide pertinent information on metabolic parameters. The potential role of this mucin-degrading bacterium in ameliorating metabolic disorders was further confirmed by a series of animal experiments. For example, mice treated with metformin and liraglutide showed increased levels of A. municiphila in association with improved control of blood glucose and body weight (55, 65). More importantly, HFD-fed mice treated with A. municiphila exhibited similar improvements in glucose tolerance and goblet cell production and inflammatory regulations as compared to the metformin treatment group (56).
The effect of metformin and acarbose on the abundance of different species of the genus Bacteroides (phylum Bacteroidetes) is interesting. The genus Bacteroides seem to play a beneficial role in glucose metabolism where B. intestinalis and B. vulgatus were decreased in T2D patients, and B. stercoris was enriched in patients with diabetes remission (1). The same phenomenon was also noted in experimental animals (1). However, decreased abundance of some Bacteroides species, including B. pleibeius, B. dorei, B. vulgatus, after using acarbose in newly diagnosed T2D patients was reported to be positively associated with body weight in one study (32). As for rodent studies, colonization of B. fragilis was associated with more severe glucose intolerance in HFD-fed mice (24). A recent study, which compared fecal microbiota compositions between T2D patients and non-diabetic individuals, showed that Bacteroides was an independent risk factor of the disease by diminishing insulin sensitivity (84).
The effects of metformin on A. muciniphila were similar in both human and rodent studies. However, there was an inverse association of Bacteroides and metformin use in human and mouse studies in this review. Alterations of many other taxa in humans treated with metformin or acarbose were not the same as in mouse studies, and vice versa. The diverse dietary habits, metabolism or inflammatory statuses of host, body sizes and organs in these human and mouse studies might contribute to inconsistent findings of gut microbiota compositions (12, 85). Although the gut microbiota of human and mice are dominated by two major phyla, i.e., Bacteroidetes and Firmicutes, approximately 85% of the representative gut microbiota sequences in mice were not found in humans (86).
Further, the genera Escherichia and Shigella, belonging to the order of Enterobacterales in phylum Proteobacteria, were found to increase consistently after metformin treatment in T2D patients. Certain bacteria belonging to the phylum Proteobacteria, including the order Enterobacteriales, was found to be overly present in patients with metabolic disorders and T2D, and were positively related to intestinal permeability and endotoxemia in the pathophysiology of these metabolic diseases (87, 88). Enrichment in the order Enterobacterales, especially Escherichia coli, was demonstrated to play an important role in gut inflammation in patients with inflammatory bowel disease and also in various mouse model of colitis (88). Elbere et al. (25) observed an association between the severity of gastrointestinal side effects and increased abundance of the genera Escherichia and Shigella. Thus, the enrichment of the order Enterobacteriales might contribute to gastrointestinal side effects of metformin.
In this review, the results of β-diversity indicate significant changes in gut microbiome structure related to metformin or acarbose treatment. The findings on α-diversity among those treated with metformin were inconsistent while the richness and evenness were decreased after treating with acarbose. For healthy human subjects, the reference microbiome list and abundance profile showed various ratios of Bacteroidetes and Firmicutes, as well as the other phyla, e.g., Acinobacteria, Proteobacteria (89). This might reflect sufficient α- and β-diversities in healthy individuals due to significant regional heterogeneity at the species level and consistency at the higher taxonomic level (89). With regard to T2D patients, the associations between the disease and the diversities of microbiota were inconclusive (1). In the other words, there is no consensus or simple way to make a conclusion on the relationship between diversity and gut microbiota compositions among T2D patients treated with metformin or acarbose.
While metformin and acarbose have been shown to stimulate the growth of SCFA-producing bacteria, e.g., Lactobacillus and Bifidobacterium, information on the effects of these drugs on the fecal levels of various SCFAs in humans is lacking in the literature. Thus, this review entailed data from mouse studies, which are more informative. These mouse studies showed an increase trend in fecal and cecal levels of acetate, propionate, and butyrate in response to treatments of metformin, acarbose, voglibose, dapagliflozin or canagliflozin, of which the impacts on other physiological functions, other than that in gastrointestinal track, warrant further evaluations (90). With respect to bile acids, information on the effects of anti-hyperglycemic agents on their levels is limited and often conflicting in human vs. rodent studies, which merits further investigations to understand the role of other confounding factors, such as diets, antibiotic therapy, and disease states (91).
Again, the most critical limitation is lack of consistency among human and rodent studies. In humans, differences in the health status of participants, disease type or staging, ethnicity, drug dosage, and duration of treatment might directly impact gut microbiota compositions. Furthermore, it becomes difficult to come to a conclusion due to the small number of participants as well as differences in study design in each study. The risk of bias of studies needs to be taken into considerations, in which overall bias of randomized trials was high risk and unclear risk, and two out of five quasi-experimental studies and all of observational studies were at serious risk. Also, there was a high degree of heterogeneity in rodent studies due to differences in species used and environmental factors, as alluded above. Differences in microbiota analysis methods could also be a cause of deviation.
Another major limitation is lack of human studies on anti-hyperglycemic drugs beyond metformin and acarbose. Thus, no conclusion could be reached regarding the associations between human gut microbiota and these drugs. Because results from rodent models might not always be translatable to humans, conclusions should be made with cautions. Although findings from rodent studies included in this review suggest potential positive effects of other anti-hyperglycemic drugs besides metformin and acarbose on human gut microbiota, additional human studies on these drugs are needed to clarify the role of gut microbiota in their therapeutic efficacies.
In light of the enormous amounts of published data, this systematic review aims to provide readers a comprehensive view of this emerging area by taking an integrated approach through an all-inclusive literature search in conjunction with vigorous data extraction and validation, and assessment of risk bias. Moreover, this systematic review has tried to differentiate various aspects of the anti-hyperglycemic drug-gut microbiome-host axis, thereby filling the gap of merging all available data from human or animal studies relevant to the inter-dependence between anti-hyperglycemic drugs and the specific taxon of gut microbiota. Nevertheless, more investigations are warranted to support the positive contribution of metformin and acarbose to the health of gut microbiome (e.g., A. muciniphila, Lactobacillus, Bifidobacterium longum). In addition, given the limited information available in the literature, more studies are needed to shed light onto the roles of other anti-hyperglycemic drugs (e.g., miglitol, voglibose) in modulating human host taxa of gut microbiome.
This review highlights that changes in specific taxa and β-diversity of gut microbiota were associated with metformin and acarbose in humans while pertinent information for other anti-hyperglycemic drugs could be only obtained in rodent studies. These results support the possible action mechanisms of these drugs, which may have translational potential to foster new approaches for the treatment of diseases related to gut dysbiosis in the future. Mouse studies on the other anti-hyperglycemics suggested the links between these drugs and gut microbiota were inconclusive. Therefore, additional human studies are needed to explore the role of gut microbiota in their therapeutic efficacies or side effects.
The original data presented in the study are included in the article/Supplementary Material, further inquiries can be directed to the corresponding author.
TC, P-CL, H-WL, CC, C-SC, K-CW, J-LH, and L-YY collected, screened, and extracted the data and analyzed the results. TC, H-WL, CC, and L-YY wrote the first draft of the manuscript. All authors contributed to conception, design of the study, manuscript revision, read, and approved the submitted version.
This research was partially supported by China Medical University under Grant CMU107-Z-03, CMU108-N-03, CMU107-Z-03, CMU-108-Z-07, and 1075955A; Ministry of Science and Technology under Grant MOST 109-2320-B-039-023, 109-2622-8-039-002-TB1, and 108-2911-I-039-301; Ministry of Education Center-of-Excellence under Grant for Drug Development Center, China Medical University, Taichung, Taiwan. The funding agencies played no role in the study implementation, analysis or interpretation of data, or preparation and review or approval of the manuscript.
The authors declare that the research was conducted in the absence of any commercial or financial relationships that could be construed as a potential conflict of interest.
We would like to appreciate Shu-Han Yang, Fang-Chu Lin, Yu-Ching Wang, Yu-Tong Wang, Shang-Hua Wu, Po-Chen Chu, and those who were not listed but helped to search and review the literature in this project.
The Supplementary Material for this article can be found online at: https://www.frontiersin.org/articles/10.3389/fendo.2020.573891/full#supplementary-material
Supplementary Figure 1. Risk of bias of randomized trials. (A) Each risk of bias item for each study. (B) Each risk of bias item across studies.
Supplementary Figure 2. Risk of bias of quasi-experimental studies. (A) Each risk of bias item for each study. (B) Each risk of bias item across studies.
Supplementary Figure 3. Risk of bias of observational studies. (A) Each risk of bias item for each study. (B) Each risk of bias item across studies.
Supplementary Figure 4. Risk of bias of animal studies. (A) Each risk of bias item for each study. (B) Each risk of bias item across studies.
Supplementary Table 1. Searching strategy.
Supplementary Table 2. Characteristics of housing and dietary of studied animals.
Supplementary Table 3. Effects of anti-hyperglycemia drugs on level of short-chain fatty acids (SCFAs).
Supplementary Table 4. Effects of anti-hyperglycemia on bile acid levels.
1. Gurung M, Li Z, You H, Rodrigues R, Jump DB, Morgun A, et al. Role of gut microbiota in type 2 diabetes pathophysiology. EBioMedicine. (2020) 51:102590. doi: 10.1016/j.ebiom.2019.11.051
2. Qin J, Li Y, Cai Z, Li S, Zhu J, Zhang F, et al. A metagenome-wide association study of gut microbiota in type 2 diabetes. Nature. (2012) 490:55–60. doi: 10.1038/nature11450
3. Adeshirlarijaney A, Gewirtz AT. Considering gut microbiota in treatment of type 2 diabetes mellitus. Gut microbes. (2020) 11:253–64. doi: 10.1080/19490976.2020.1717719
4. Tiderencel KA, Hutcheon DA, Ziegler J. Probiotics for the treatment of type 2 diabetes: a review of randomized controlled trials. Diabetes Metab Res Rev. (2020) 36:e3213. doi: 10.1002/dmrr.3213
5. Zhang M, Feng R, Yang M, Qian C, Wang Z, Liu W, et al. Effects of metformin, acarbose, and sitagliptin monotherapy on gut microbiota in zucker diabetic fatty rats. BMJ Open Diabetes Res Care. (2019) 7:e000717. doi: 10.1136/bmjdrc-2019-000717
6. Zhang Q, Xiao X, Li M, Yu M, Ping F, Zheng J, et al. Vildagliptin increases butyrate-producing bacteria in the gut of diabetic rats. PLoS ONE. (2017) 12:e0184735. doi: 10.1371/journal.pone.0184735
7. Zhang X, Fang Z, Zhang C, Xia H, Jie Z, Han X, et al. Effects of acarbose on the gut microbiota of prediabetic patients: a randomized, double-blind, controlled crossover trial. Diabetes Ther. (2017) 8:293–307. doi: 10.1007/s13300-017-0226-y
8. Whang A, Nagpal R, Yadav H. Bi-directional drug-microbiome interactions of anti-diabetics. EBioMedicine. (2019) 39:591–602. doi: 10.1016/j.ebiom.2018.11.046
9. Kyriachenko Y, Falalyeyeva T, Korotkyi O, Molochek N, Kobyliak N. Crosstalk between gut microbiota and antidiabetic drug action. World J Diabetes. (2019) 10:154–68. doi: 10.4239/wjd.v10.i3.154
10. Stein SA, Lamos EM, Davis SN. A review of the efficacy and safety of oral antidiabetic drugs. Expert Opin Drug Saf. (2013) 12:153–75. doi: 10.1517/14740338.2013.752813
11. Montandon SA, Jornayvaz FR. Effects of antidiabetic drugs on gut microbiota composition. Genes. (2017) 8:250. doi: 10.3390/genes8100250
12. Nguyen TL, Vieira-Silva S, Liston A, Raes J. How informative is the mouse for human gut microbiota research? Dis Model Mech. (2015) 8:1–16. doi: 10.1242/dmm.017400
13. Pascale A, Marchesi N, Govoni S, Coppola A, Gazzaruso C. The role of gut microbiota in obesity, diabetes mellitus, and effect of metformin: new insights into old diseases. Curr Opin Pharmacol. (2019) 49:1–5. doi: 10.1016/j.coph.2019.03.011
14. Scells H, Zuccon G, CSIRO BK, Deacon A, Azzopardi L, Geva S. Integrating the framing of clinical questions via pico into the retrieval of medical literature for systematic reviews. In: Proceedings of the 2017 ACM on Conference on Information and Knowledge Management; 2017 November 6-10; Singapore. Association for Computing Machinery, New York, NY (2017). p. 2291–94.
15. Liberati A, Altman DG, Tetzlaff J, Mulrow C, Gotzsche PC, Ioannidis JP, et al. The PRISMA statement for reporting systematic reviews and meta-analyses of studies that evaluate healthcare interventions: explanation and elaboration. BMJ. (2009) 339:b2700. doi: 10.1136/bmj.b2700
16. Higgins JP, Altman DG, Gotzsche PC, Juni P, Moher D, Oxman AD, et al. The cochrane collaboration's tool for assessing risk of bias in randomised trials. BMJ. (2011) 343:d5928. doi: 10.1136/bmj.d5928
17. Sterne JA, Hernan MA, Reeves BC, Savovic J, Berkman ND, Viswanathan M, et al. ROBINS-I: a tool for assessing risk of bias in non-randomised studies of interventions. BMJ. (2016) 355:i4919. doi: 10.1136/bmj.i4919
18. Hooijmans CR, Rovers MM, de Vries RB, Leenaars M, Ritskes-Hoitinga M, Langendam MW. SYRCLE's risk of bias tool for animal studies. BMC Med Res Methodol. (2014) 14:43. doi: 10.1186/1471-2288-14-43
19. Ejtahed HS, Tito RY, Siadat SD, Hasani-Ranjbar S, Hoseini-Tavassol Z, Rymenans L, et al. Metformin induces weight loss associated with gut microbiota alteration in non-diabetic obese women: a randomized double-blind clinical trial. Eur J Endocrinol. (2019) 180:165–76. doi: 10.1530/EJE-18-0826
20. Tong X, Xu J, Lian F, Yu X, Zhao Y, Xu L, et al. Structural alteration of gut microbiota during the amelioration of human type 2 diabetes with hyperlipidemia by metformin and a traditional Chinese herbal formula: a multicenter, randomized, open label clinical trial. mBio. (2018) 9:e02392–17. doi: 10.1128/mBio.02392-17
21. Wu H, Esteve E, Tremaroli V, Khan MT, Caesar R, Mannerås-Holm L, et al. Metformin alters the gut microbiome of individuals with treatment-naive type 2 diabetes, contributing to the therapeutic effects of the drug. Nat Med. (2017) 23:850–8. doi: 10.1038/nm.4345
22. Bryrup T, Thomsen CW, Kern T, Allin KH, Brandslund I, Jørgensen NR, et al. Metformin-induced changes of the gut microbiota in healthy young men: results of a non-blinded, one-armed intervention study. Diabetologia. (2019) 62:1024–35. doi: 10.1007/s00125-019-4848-7
23. Huang F, Nilholm C, Roth B, Linninge C, Höglund P, Nyman M, et al. Anthropometric and metabolic improvements in human type 2 diabetes after introduction of an okinawan-based nordic diet are not associated with changes in microbial diversity or SCFA concentrations. Int J Food Sci Nutr. (2018) 69:729–40. doi: 10.1080/09637486.2017.1408059
24. Sun L, Xie C, Wang G, Wu Y, Wu Q, Wang X, et al. Gut microbiota and intestinal FXR mediate the clinical benefits of metformin. Nat Med. (2018) 24:1919–29. doi: 10.1038/s41591-018-0222-4
25. Elbere I, Kalnina I, Silamikelis I, Konrade I, Zaharenko L, Sekace K, et al. Association of metformin administration with gut microbiome dysbiosis in healthy volunteers. PLoS ONE. (2018) 13:e0204317. doi: 10.1371/journal.pone.0204317
26. Napolitano A, Miller S, Nicholls AW, Baker D, Van Horn S, Thomas E, et al. Novel gut-based pharmacology of metformin in patients with type 2 diabetes mellitus. PLoS ONE. (2014) 9:e100778. doi: 10.1371/journal.pone.0100778
27. Zhang F, Wang M, Yang J, Xu Q, Liang C, Chen B, et al. Response of gut microbiota in type 2 diabetes to hypoglycemic agents. Endocrine. (2019) 66:485–93. doi: 10.1007/s12020-019-02041-5
28. Barengolts E, Green SJ, Eisenberg Y, Akbar A, Reddivari B, Layden BT, et al. Gut microbiota varies by opioid use, circulating leptin and oxytocin in African American men with diabetes and high burden of chronic disease. PLoS ONE. (2018) 13:e0194171. doi: 10.1371/journal.pone.0194171
29. De La Cuesta-Zuluaga J, Mueller NT, Corrales-Agudelo V, Velásquez-Mejía EP, Carmona JA, Abad JM, et al. Metformin is associated with higher relative abundance of mucin-degrading akkermansia muciniphila and several short-chain fatty acid-producing microbiota in the gut. Diabetes Care. (2017) 40:54–62. doi: 10.2337/dc16-1324
30. Forslund K, Hildebrand F, Nielsen T, Falony G, Le Chatelier E, Sunagawa S, et al. Disentangling type 2 diabetes and metformin treatment signatures in the human gut microbiota. Nature. (2015) 528:262–6. doi: 10.1038/nature15766
31. Karlsson FH, Tremaroli V, Nookaew I, Bergström G, Behre CJ, Fagerberg B, et al. Gut metagenome in European women with normal, impaired and diabetic glucose control. Nature. (2013) 498:99–103. doi: 10.1038/nature12198
32. Gu Y, Wang X, Li J, Zhang Y, Zhong H, Liu R, et al. Analyses of gut microbiota and plasma bile acids enable stratification of patients for antidiabetic treatment. Nat Commun. (2017) 8:1785. doi: 10.1038/s41467-017-01682-2
33. Su B, Liu H, Li J, Sunli Y, Liu B, Liu D, et al. Acarbose treatment affects the serum levels of inflammatory cytokines and the gut content of bifidobacteria in Chinese patients with type 2 diabetes mellitus. J Diabetes. (2015) 7:729–39. doi: 10.1111/1753-0407.12232
34. Wang Z, Saha S, Van Horn S, Thomas E, Traini C, Sathe G, et al. Gut microbiome differences between metformin- and liraglutide-treated T2DM subjects. Endocrinol Diabetes Metab. (2018) 1:e00009. doi: 10.1002/edm2.9
35. Zhang W, Xu JH, Yu T, Chen QK. Effects of berberine and metformin on intestinal inflammation and gut microbiome composition in db/db mice. Biomed Pharmacother. (2019) 118:109131. doi: 10.1016/j.biopha.2019.109131
36. Xue J, Li X, Liu P, Li K, Sha L, Yang X, et al. Inulin and metformin ameliorate polycystic ovary syndrome via anti-inflammation and modulating gut microbiota in mice. Endocr J. (2019) 66:859–70. doi: 10.1507/endocrj.EJ18-0567
37. Ryan PM, Patterson E, Carafa I, Mandal R, Wishart DS, Dinan TG, et al. Metformin and dipeptidase peptidyl-4 inhibitor differentially modulate the intestinal microbiota and plasma metabolome of metabolically dysfunctional mice. Can J Diabetes. (2019) 44:146–55.e2. doi: 10.1016/j.jcjd.2019.05.008
38. Ji S, Wang L, Li L. Effect of metformin on short-term high-fat diet-induced weight gain and anxiety-like behavior and the gut microbiota. Front Endocrinol. (2019) 10:704. doi: 10.3389/fendo.2019.00704
39. Dong TS, Chang HH, Hauer M, Lagishetty V, Katzka W, Rozengurt E, et al. Metformin alters the duodenal microbiome and decreases the incidence of pancreatic ductal adenocarcinoma promoted by diet-induced obesity. Am J Physiol Gastrointest Liver Physiol. (2019) 317:G763–72. doi: 10.1152/ajpgi.00170.2019
40. Brandt A, Hernández-Arriaga A, Kehm R, Sánchez V, Jin CJ, Nier A, et al. Metformin attenuates the onset of non-alcoholic fatty liver disease and affects intestinal microbiota and barrier in small intestine. Sci Rep. (2019) 9:6668. doi: 10.1038/s41598-019-43228-0
41. Adeshirlarijaney A, Zou J, Tran H, Chassaing B, Gewirtz AT. Amelioration of metabolic syndrome by metformin associates with reduced indices of low-grade inflammation independently of the gut microbiota. Am J Physiol Endocrinol Metab. (2019) 317:E1121–30. doi: 10.1152/ajpendo.00245.2019
42. Xu M, Yue RS, Yang MY, Yang X, Wu TC, Li JN. Effects of banxia xiexin decoction on intestinal flora and inflammatory factors of diabetic gastroparesis rats. Chin Trad Herbal Drugs. (2018) 49:3056–61. doi: 10.7501/j.issn.0253-2670.2018.13.015
43. Bauer PV, Duca FA, Waise TMZ, Rasmussen BA, Abraham MA, Dranse HJ, et al. Metformin alters upper small intestinal microbiota that impact a glucose-SGLT1-sensing glucoregulatory pathway. Cell Metab. (2018) 27:101–17.e5. doi: 10.1016/j.cmet.2017.09.019
44. Zheng J, Li H, Zhang X, Jiang M, Luo C, Lu Z, et al. Prebiotic mannan-oligosaccharides augment the hypoglycemic effects of metformin in correlation with modulating gut microbiota. J Agric Food Chem. (2018) 66:5821–31. doi: 10.1021/acs.jafc.8b00829
45. Wang JH, Bose S, Shin NR, Chin YW, Choi YH, Kim H. Pharmaceutical impact of houttuynia cordata and metformin combination on high-fat-diet-induced metabolic disorders: link to intestinal microbiota and metabolic endotoxemia. Front Endocrinol. (2018) 9:620. doi: 10.3389/fendo.2018.00620
46. Ma W, Chen J, Meng Y, Yang J, Cui Q, Zhou Y. Metformin alters gut microbiota of healthy mice: implication for its potential role in gut microbiota homeostasis. Front Microbiol. (2018) 9:1336. doi: 10.3389/fmicb.2018.01336
47. Liu G, Bei J, Liang L, Yu G, Li L, Li Q. Stachyose improves inflammation through modulating gut microbiota of high-fat diet/streptozotocin-induced type 2 diabetes in rats. Mol Nutr Food Res. (2018) 62:e1700954. doi: 10.1002/mnfr.201700954
48. Lee H, Lee Y, Kim J, An J, Lee S, Kong H, et al. Modulation of the gut microbiota by metformin improves metabolic profiles in aged obese mice. Gut Microbes. (2018) 9:155–65. doi: 10.1080/19490976.2017.1405209
49. Shin NR, Bose S, Wang JH, Ansari A, Lim SK, Chin YW, et al. Flos lonicera combined with metformin ameliorates hepatosteatosis and glucose intolerance in association with gut microbiota modulation. Front Microbiol. (2017) 8:2271. doi: 10.3389/fmicb.2017.02271
50. Wang JH, Bose S, Lim SK, Ansari A, Chin YW, Choi HS, et al. Houttuynia cordata facilitates metformin on ameliorating insulin resistance associated with gut microbiota alteration in OLETF rats. Genes. (2017) 8:239. doi: 10.3390/genes8100239
51. Han K, Bose S, Wang JH, Lim SK, Chin YW, Kim YM, et al. In vivo therapeutic effect of combination treatment with metformin and scutellaria baicalensis on maintaining bile acid homeostasis. PLoS ONE. (2017) 12:e0182467. doi: 10.1371/journal.pone.0182467
52. Salomäki-Myftari H, Vähätalo LH, Ailanen L, Pietilä S, Laiho A, Hänninen A, et al. Neuropeptide Y overexpressing female and male mice show divergent metabolic but not gut microbial responses to prenatal metformin exposure. PLoS ONE. (2016) 11:e0163805. doi: 10.1371/journal.pone.0163805
53. Zhou ZY, Ren LW, Zhan P, Yang HY, Chai DD, Yu ZW. Metformin exerts glucose-lowering action in high-fat fed mice via attenuating endotoxemia and enhancing insulin signaling. Acta Pharmacol Sin. (2016) 37:1063–75. doi: 10.1038/aps.2016.21
54. Zhang X, Zhao Y, Xu J, Xue Z, Zhang M, Pang X, et al. Modulation of gut microbiota by berberine and metformin during the treatment of high-fat diet-induced obesity in rats. Sci Rep. (2015) 5:14405. doi: 10.1038/srep14405
55. Lee H, Ko G. Effect of metformin on metabolic improvement and gut microbiota. Appl Environ Microbiol. (2014) 80:5935–43. doi: 10.1128/AEM.01357-14
56. Shin NR, Lee JC, Lee HY, Kim MS, Whon TW, Lee MS, et al. An increase in the Akkermansia spp. population induced by metformin treatment improves glucose homeostasis in diet-induced obese mice. Gut. (2014) 63:727–35. doi: 10.1136/gutjnl-2012-303839
57. Pyra KA, Saha DC, Reimer RA. Prebiotic fiber increases hepatic acetyl CoA carboxylase phosphorylation and suppresses glucose-dependent insulinotropic polypeptide secretion more effectively when used with metformin in obese rats. J Nutr. (2012) 142:213–20. doi: 10.3945/jn.111.147132
58. Zhao J, Li Y, Sun M, Xin L, Wang T, Wei L, et al. The Chinese herbal formula shenzhu tiaopi granule results in metabolic improvement in type 2 diabetic rats by modulating the gut microbiota. Evid Based Complement Altern Med. (2019) 2019:6976394. doi: 10.1155/2019/6976394
59. Smith BJ, Miller RA, Ericsson AC, Harrison DC, Strong R, Schmidt TM. Changes in the gut microbiome and fermentation products concurrent with enhanced longevity in acarbose-treated mice. BMC Microbiol. (2019) 19:130. doi: 10.1186/s12866-019-1494-7
60. Liao X, Song L, Zeng B, Liu B, Qiu Y, Qu H, et al. Alteration of gut microbiota induced by DPP-4i treatment improves glucose homeostasis. EBioMedicine. (2019) 44:665–74. doi: 10.1016/j.ebiom.2019.03.057
61. Baxter NT, Lesniak NA, Sinani H, Schloss PD, Koropatkin NM. The glucoamylase inhibitor acarbose has a diet-dependent and reversible effect on the murine gut microbiome. mSphere. (2019) 4:e00528–18. doi: 10.1128/mSphere.00528-18
62. Xu GD, Cai L, Ni YS, Tian SY, Lu YQ, Wang LN, et al. Comparisons of effects on intestinal short-chain fatty acid concentration after exposure of two glycosidase inhibitors in mice. Biol Pharm Bull. (2018) 41:1024–33. doi: 10.1248/bpb.b17-00978
63. Do HJ, Lee YS, Ha MJ, Cho Y, Yi H, Hwang YJ, et al. Beneficial effects of voglibose administration on body weight and lipid metabolism via gastrointestinal bile acid modification. Endocr J. (2016) 63:691–702. doi: 10.1507/endocrj.EJ15-0747
64. Kishida Y, Okubo H, Ohno H, Oki K, Yoneda M. Effect of miglitol on the suppression of nonalcoholic steatohepatitis development and improvement of the gut environment in a rodent model. J Gastroenterol. (2017) 52:1180–91. doi: 10.1007/s00535-017-1331-4
65. Madsen MSA, Holm JB, Pallejà A, Wismann P, Fabricius K, Rigbolt K, et al. Metabolic and gut microbiome changes following GLP-1 or dual GLP-1/GLP-2 receptor agonist treatment in diet-induced obese mice. Sci Rep. (2019) 9:15582. doi: 10.1038/s41598-019-52103-x
66. Zhao L, Chen Y, Xia F, Abudukerimu B, Zhang W, Guo Y, et al. A glucagon-like peptide-1 receptor agonist lowers weight by modulating the structure of gut microbiota. Front Endocrinol. (2018) 9:233. doi: 10.3389/fendo.2018.00233
67. Yuan X, Ni H, Chen X, Feng X, Wu Q, Chen J. Identification of therapeutic effect of glucagon-like peptide 1 in the treatment of STZ-induced diabetes mellitus in rats by restoring the balance of intestinal flora. J Cell Biochem. (2018) 119:10067–74. doi: 10.1002/jcb.27343
68. Moreira GV, Azevedo FF, Ribeiro LM, Santos A, Guadagnini D, Gama P, et al. Liraglutide modulates gut microbiota and reduces NAFLD in obese mice. J Nutr Biochem. (2018) 62:143–54. doi: 10.1016/j.jnutbio.2018.07.009
69. Zhang Q, Xiao X, Zheng J, Li M, Yu M, Ping F, et al. Featured article: structure moderation of gut microbiota in liraglutide-treated diabetic male rats. Exp Biol Med. (2018) 243:34–44. doi: 10.1177/1535370217743765
70. Wang L, Li P, Tang Z, Yan X, Feng B. Structural modulation of the gut microbiota and the relationship with body weight: compared evaluation of liraglutide and saxagliptin treatment. Sci Rep. (2016) 6:33251. doi: 10.1038/srep33251
71. Kaya D, Kaji K, Tsuji Y, Yamashita S, Kitagawa K, Ozutsumi T, et al. Tgr5 activation modulates an inhibitory effect on liver fibrosis development mediated by anagliptin in diabetic rats. Cells. (2019) 8:1153. doi: 10.3390/cells8101153
72. Dennison CA, Eslinger AJ, Reimer RA. Preconception prebiotic and sitagliptin treatment in obese rats affects pregnancy outcomes and offspring microbiota, adiposity, and glycemia. Front Endocrinol. (2017) 8:301. doi: 10.3389/fendo.2017.00301
73. Yan X, Feng B, Li P, Tang Z, Wang L. Microflora disturbance during progression of glucose intolerance and effect of sitagliptin: an animal study. J Diabetes Res. (2016) 2016:2093171. doi: 10.1155/2016/2093171
74. Olivares M, Neyrinck AM, Pötgens SA, Beaumont M, Salazar N, Cani PD, et al. The DPP-4 inhibitor vildagliptin impacts the gut microbiota and prevents disruption of intestinal homeostasis induced by a Western diet in mice. Diabetologia. (2018) 61:1838–48. doi: 10.1007/s00125-018-4647-6
75. Li L, Xu S, Guo T, Gong S, Zhang C. Effect of dapagliflozin on intestinal flora in mafa-deficient mice. Curr Pharm Des. (2018) 24:3223–31. doi: 10.2174/1381612824666180912143434
76. Lee DM, Battson ML, Jarrell DK, Hou S, Ecton KE, Weir TL, et al. SGLT2 inhibition via dapagliflozin improves generalized vascular dysfunction and alters the gut microbiota in type 2 diabetic mice. Cardiovasc Diabetol. (2018) 17:62. doi: 10.1186/s12933-018-0708-x
77. Mishima E, Fukuda S, Kanemitsu Y, Saigusa D, Mukawa C, Asaji K, et al. Canagliflozin reduces plasma uremic toxins and alters the intestinal microbiota composition in a chronic kidney disease mouse model. Am J Physiol Renal Physiol. (2018) 315:F824–33. doi: 10.1152/ajprenal.00314.2017
78. Li Y, Han L, Xu M, Guo J, Feng M, Wang X. The primary research on the gut microbes in KKAy mice. Indian J Microbiol. (2014) 54:12–9. doi: 10.1007/s12088-013-0410-3
79. Wang LY, Wang S, Wang Y, Ruan KF, Feng Y. Effect of MDG-1 on oral glucose tolerance and intestinal microecological balance in diabetic mice. World Chin J Digestol. (2011) 19:2058–62. doi: 10.11569/wcjd.v19.i19.2058
80. Bytzer P, Talley NJ, Jones MP, Horowitz M. Oral hypoglycaemic drugs and gastrointestinal symptoms in diabetes mellitus. Aliment Pharmacol Ther. (2001) 15:137–42. doi: 10.1046/j.1365-2036.2001.00896.x
81. Sedighi M, Razavi S, Navab-Moghadam F, Khamseh ME, Alaei-Shahmiri F, Mehrtash A, et al. Comparison of gut microbiota in adult patients with type 2 diabetes and healthy individuals. Microb Pathog. (2017) 111:362–9. doi: 10.1016/j.micpath.2017.08.038
82. Candela M, Biagi E, Soverini M, Consolandi C, Quercia S, Severgnini M, et al. Modulation of gut microbiota dysbioses in type 2 diabetic patients by macrobiotic Ma-Pi 2 diet. Br J Nutr. (2016) 116:80–93. doi: 10.1017/S0007114516001045
83. Derrien M, Belzer C, de Vos WM. Akkermansia muciniphila and its role in regulating host functions. Microb Pathog. (2017) 106:171–81. doi: 10.1016/j.micpath.2016.02.005
84. Wang J, Li W, Wang C, Wang L, He T, Hu H, et al. Enterotype bacteroides is associated with a high risk in patients with diabetes: a pilot study. J Diabetes Res. (2020) 2020:6047145. doi: 10.1155/2020/6047145
85. Cani PD, Possemiers S, Van de Wiele T, Guiot Y, Everard A, Rottier O, et al. Changes in gut microbiota control inflammation in obese mice through a mechanism involving GLP-2-driven improvement of gut permeability. Gut. (2009) 58:1091–103. doi: 10.1136/gut.2008.165886
86. Ley RE, Backhed F, Turnbaugh P, Lozupone CA, Knight RD, Gordon JI. Obesity alters gut microbial ecology. Proc Natl Acad Sci USA. (2005) 102:11070–5. doi: 10.1073/pnas.0504978102
87. Pedersen C, Ijaz UZ, Gallagher E, Horton F, Ellis RJ, Jaiyeola E, et al. Fecal enterobacteriales enrichment is associated with increased in vivo intestinal permeability in humans. Physiol Rep. (2018) 6:e13649. doi: 10.14814/phy2.13649
88. Rizzatti G, Lopetuso LR, Gibiino G, Binda C, Gasbarrini A. Proteobacteria: a common factor in human diseases. Biomed Res Int. (2017) 2017:9351507. doi: 10.1155/2017/9351507
89. King CH, Desai H, Sylvetsky AC, LoTempio J, Ayanyan S, Carrie J, et al. Baseline human gut microbiota profile in healthy people and standard reporting template. PLoS ONE. (2019) 14:e0206484. doi: 10.1371/journal.pone.0206484
90. Dalile B, Van Oudenhove L, Vervliet B, Verbeke K. The role of short-chain fatty acids in microbiota-gut-brain communication. Nat Rev Gastroenterol Hepatol. (2019) 16:461–78. doi: 10.1038/s41575-019-0157-3
Keywords: anti-hyperglycemic drugs, microbiome, microbiota, association, systematic review
Citation: Cao TTB, Wu K-C, Hsu J-L, Chang C-S, Chou C, Lin C-Y, Liao Y-M, Lin P-C, Yang L-Y and Lin H-W (2020) Effects of Non-insulin Anti-hyperglycemic Agents on Gut Microbiota: A Systematic Review on Human and Animal Studies. Front. Endocrinol. 11:573891. doi: 10.3389/fendo.2020.573891
Received: 18 June 2020; Accepted: 20 August 2020;
Published: 23 September 2020.
Edited by:
Mora Murri, University of Málaga, SpainReviewed by:
Maria Insenser, Centre for Biomedical Network Research (CIBER), SpainCopyright © 2020 Cao, Wu, Hsu, Chang, Chou, Lin, Liao, Lin, Yang and Lin. This is an open-access article distributed under the terms of the Creative Commons Attribution License (CC BY). The use, distribution or reproduction in other forums is permitted, provided the original author(s) and the copyright owner(s) are credited and that the original publication in this journal is cited, in accordance with accepted academic practice. No use, distribution or reproduction is permitted which does not comply with these terms.
*Correspondence: Hsiang-Wen Lin, aHNpYW5nd2xAZ21haWwuY29t
Disclaimer: All claims expressed in this article are solely those of the authors and do not necessarily represent those of their affiliated organizations, or those of the publisher, the editors and the reviewers. Any product that may be evaluated in this article or claim that may be made by its manufacturer is not guaranteed or endorsed by the publisher.
Research integrity at Frontiers
Learn more about the work of our research integrity team to safeguard the quality of each article we publish.