- 1School of Sport, Exercise, and Rehabilitation Sciences, College of Life and Environmental Sciences, University of Birmingham, Edgbaston, United Kingdom
- 2Institute of Systems and Metabolism Research, College of Medical and Dental Sciences, University of Birmingham, Edgbaston, United Kingdom
Background: Exercise improves glycemic control but the magnitude, and in some cases, the direction of this effect is variable. Ambient hyperglycemia has been implicated in this exercise response heterogeneity. The current study investigated whether pre-exercise hyperglycemia directly impacts the effect of exercise on glycemic control.
Methods: Twelve healthy normal glucose-tolerant males completed four trials in a randomized, crossover design. Each trial consisted of 24-h pre-intervention monitoring, a 7-h intervention, and 24-h post-intervention monitoring. Glycemic control was measured throughout the study by continuous glucose monitoring. The four interventions were no exercise (CON) or 45 min of cycling exercise (70%HRmax) preceded by 3.5 h of either normoglycemia (NG-Ex), steady-state hyperglycemia induced by constant glucose infusion (HG-Ex) or fluctuating glycemia induced by repeated glucose bolus infusions (FG-Ex).
Results: Physical activity and diet were similar between trials, and energy expenditure during exercise was matched between exercise trials (all P > 0.05). Mean glucose during the 3.5 h ± infusion period was higher in HG-Ex (mean ± SEM; 7.2 ± 0.4 mmol/L) and FG-Ex (7.3 ± 0.3 mmol/L) compared to CON (4.8 ± 0.2 mmol/L) and NG-Ex (5.0 ± 0.2 mmol/L) trials (P < 0.01). Glycemic variability was greatest in FG-Ex (P < 0.01). Following the interventions, the postprandial glucose response (iAUC) was reduced by exercise in NG-Ex compared to CON (321.1 ± 38.6 vs. 445.5 ± 49.7 mmol/L.8h, P < 0.05, d=0.81). This benefit was blunted when exercise was preceded by steady-state (HG-Ex, 425.3 ± 45.7 mmol/L.8h) and fluctuating (FG-Ex, 465.5 ± 39.3 mmol/L.8h) hyperglycemia (both P > 0.05 vs. CON).
Conclusion: Pre-exercise hyperglycemia blunted the glucoregulatory benefits of acute exercise upon postprandial glucose response, suggesting that exposure to hyperglycemia contributes to exercise response heterogeneity.
Clinical Trial Registration: ClinicalTrials.gov, identifier NCT03284216.
Introduction
The worldwide prevalence of type 2 diabetes mellitus (T2DM) continues to rise, meaning increasing proportions of the global population are at risk of or living with a range of serious microvascular and macrovascular complications (1–3). Consequently, the economic cost of managing T2DM continues to rise and places an increasing burden on healthcare systems. Optimizing interventions to improve glycemic control remains a clinical necessity.
The level of glycemia, and particularly postprandial glucose exposure (4–6), has been implicated with the aforementioned diabetic complications, as well as being predictive of a worsening glycated haemoglobin (HbA1c) level in non-diabetic individuals (7). Therefore, reducing postprandial glucose exposure is particularly important for the prevention and long-term management of glycemic control in individuals with or at risk of T2DM. Exercise training exerts potent glucoregulatory effects in those with and at risk of developing T2DM. For example, exercise training reduces HbA1c and fasting blood glucose (8, 9) as well as increases peripheral insulin sensitivity (10–12) and β-cell insulin secretory function (13, 14). A single exercise bout also potently increases glucose uptake, insulin sensitivity (15) and β-cell insulin secretory function (16) in the hours to days following each exercise bout. Similarly, and of particular relevance to the current study, reduced (i.e., improved) postprandial glucose response is also among the glucoregulatory effects of a single exercise bout (17–21). Many of these benefits are gained by individuals with and without T2DM, but the transient nature of benefits means exercise must be repeated regularly to preserve metabolic health. Accordingly, regular exercise forms the cornerstone in the prevention and management of hyperglycemia-related conditions, including T2DM (22).
While the potent glucoregulatory effects of exercise are unequivocal, the magnitude and direction of change following both acute exercise (16, 23, 24) and exercise training (25, 26) in hyperglycemic individuals vary considerably. Isolating factors contributing to this exercise response heterogeneity is vital to optimizing the glucoregulatory effects of exercise in this population and have been discussed in several recent reviews (27–30). In free-living environments, factors such as diet and exercise characteristics/adherence and exercise-medication interactions (31, 32) likely contribute to variability in this setting (30). Interestingly, evidence from recent well-controlled studies, where free-living sources of heterogeneity (e.g., exercise-drug interaction, exercise adherence, diet) are controlled, implicates the degree of hyperglycemia as one possible contributor to heterogeneity following single exercise bouts as well as exercise training (27, 28, 30). That said, equivocal conclusions have been made in studies to date, with both blunted (16, 33–35) and potentiated (23, 24) exercise-induced glucoregulatory benefits associated with higher baseline fasting plasma glucose and/or HbA1c. Furthermore, the evidence to date is also largely correlational, meaning that the direct effect of hyperglycemia on exercise-mediated improvements in glycemic control remains to be tested experimentally. Accordingly, the current study investigated the impact of pre-exercise hyperglycemia on the response to a single exercise bout in healthy normal glucose-tolerant participants. It was hypothesized that pre-exercise hyperglycemia would blunt the glucoregulatory effects of acute exercise.
Materials and Methods
Participants
Healthy, recreationally active males with normal glucose tolerance (n = 12; Table 1) were recruited from the local community to participate in the current study. Potential participants underwent an initial screening visit to determine their eligibility for the study. Individuals were excluded from participation if they smoked, had a BMI >30 kg/m2, and/or had a history of cancer, haematological, pulmonary, cardiac, hepatic, renal, metabolic, or gastrointestinal diseases. All participants provided written informed consent before participation. The CONSORT diagram in Supplemental Figure S1 shows an overview of the recruitment/screening/inclusion decisions. Formal sample size calculations were made using G*Power Version 3.1.7 (36). Ethical approval was obtained through the West Midlands - South Birmingham Research Ethics Committee (16/WM/0242) and sponsored by the University of Birmingham Research Governance. The study is registered at ClinicalTrials.gov (NCT03284216).
Screening and Habitual Monitoring
All participants completed a general health questionnaire, as well as had their body composition (height, weight, BMI, waist circumference) and HbA1c analyzed to confirm eligibility for the current study. Subsequently, participants performed a maximal incremental exercise test on a cycle ergometer (Lode Excalibur, Groningen, The Netherlands) to determine their maximum workload capacity (Wmax), maximal oxygen consumption (V̇O2max), and maximal heart rate (HRmax). After 5-min warm-up at 50 W, the workload was increased by 25 W/min until exhaustion. Oxygen consumption was assessed continuously during exercise via indirect calorimetry (Vyntus CPX, Jaeger, CareFusion, Germany), with heart rate (Polar Wearlink, Polar Electro Oy, Kempele, Finland), and ratings of perceived exertion (RPE; 6–20 Borg scale) monitored throughout. Following screening, participants completed 7 days of habitual monitoring, during which accelerometry (Actigraph wGT3X-BT, Pensacola, FL) and diet logs were used to assess habitual physical activity and diet, respectively.
Study Design
Participants completed four experimental trials in a randomized, crossover design with trials separated by ~1 week, during which time participants were instructed to maintain habitual physical activity and diet. Each trial consisted of a 24-h pre-trial monitoring period, a 7-h experimental intervention period, and a 24-h post-trial monitoring period (Figure 1). The four 7-h interventions were completed in a randomized order (determined using http://www.randomization.com/) and were: normoglycemia, no exercise (CON), normoglycemia plus exercise (NG-Ex), steady-state hyperglycemia plus exercise (HG-Ex), and fluctuating glycemia plus exercise (FG-Ex).
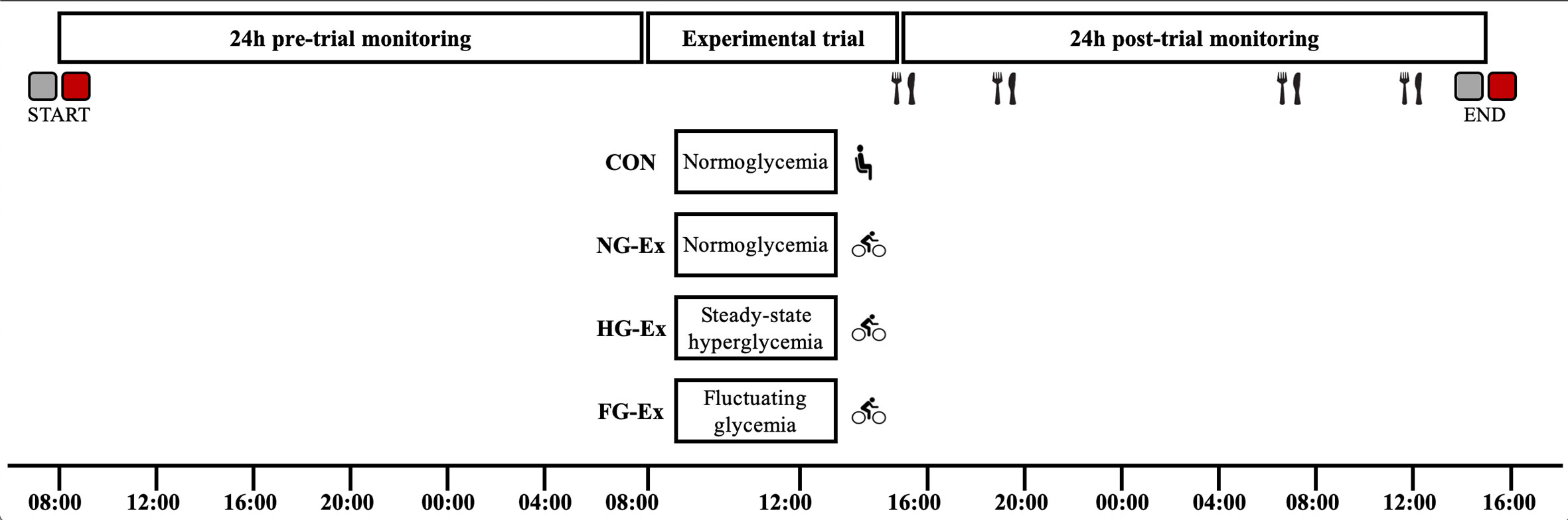
Figure 1 Schematic overview of the study design. Pre-trial monitoring involving physical activity () and CGM-derived glycemic control (
) began 24 h before each experimental trial. During experimental trials, participants were exposed to differing glycemic profiles followed by 45 min of rest (
) or exercise (
). Post-trial glycemic control was assessed under strict dietary control (
) but otherwise free-living conditions. A full description is provided in the text.
Pre-Trial Standardization and Monitoring Period
In the afternoon of day 0, participants reported to the laboratory for insertion of the continuous glucose monitoring (CGM) glucose sensor (Dexcom G5 mobile, Camberley, UK) into the abdominal subcutaneous adipose tissue, and to receive an accelerometer and diet records. Data was not collected on this day; instead, the purpose of this visit was to allow time for CGM calibration (which required ~2–3 h) in preparation for data collection to commence on day 1. The CGM was calibrated twice daily using a glucose meter (Contour Next One, Ascensia Diabetes Care UK, Berkshire, UK) on fingertip capillary blood samples. Participants were provided with detailed written instructions, including refraining from strenuous physical activity and the consumption of alcohol and caffeine from day 0 to day 3, inclusive.
On day 1 (24-h pre-trial), diet record (food type, amount and time of ingestion), accelerometry-derived physical activity, and CGM data collection commenced and continued until 24-h post-exercise (i.e., days 1–3, inclusive). Participants consumed a self-selected diet before trial 1 and then replicated this chosen diet on day 1 of subsequent trials. Similarly, participants were instructed to replicate as closely as possible activity patterns (e.g., mode of transportation) during the 24 h before each trial. This approach to pre-trial standardization is similar to other studies assessing the acute effects of exercise on glucose metabolism (37–39). Repeated verbal and written reminders of the importance of adhering to these standardizations were provided throughout.
Experimental Trials
On day 2, participants reported to the laboratory at 08:00, after an overnight fast (≥10 h, except water). Bodyweight, height, and waist circumference were measured by standard procedures, and bilateral antecubital venous lines were placed for glucose infusion and blood sampling. The four trials were identical except for the pre-exercise glycemic intervention and the rest vs. exercise conditions. Specifically, after baseline measurements, one of four 3.5-h pre-exercise glycemic interventions commenced: CON (no infusion); NG-Ex (no infusion); HG-Ex, a constant glucose infusion of 1.2 g/kg for 3.5 h (5.71 mg/kg/min); and FG-Ex, a total of 1.2 g/kg of glucose infused over 3.5 h but divided into 8 equal boluses every 30 min (0.15 g/kg per bolus, infused over 3.5 min at 42.86 mg/kg/min). Glucose (20% w/v glucose monohydrate; Baxter FKB0213B) infusion was administered using a volumetric infusion system (Infusomat Space pump, B. Braun Medical Ltd, Sheffield, UK). Heart rate, blood pressure, oxygen saturation, and body temperature were measured throughout to monitor participant safety and well-being; no within- or between-trial differences were found (data not shown). Sixty minutes after cessation of the respective glycemic intervention (to allow for normalization of glucose levels), participants completed either a 45-min rest period (CON; remain seated) or a 45-min exercise bout (NG-Ex, HG-Ex, and FG-Ex; stationary cycling at 70% HRmax). Indirect calorimetry, heart rate and power output were measured continuously throughout the exercise/rest period. The end of the 45-min ± exercise period marked the start of the 24-h post-trial monitoring period.
Post-Trial Standardization and Monitoring Period
For the remainder of day 2 until ~15:00 on day 3 (i.e., the 24-h post-exercise period), CGM, accelerometry, and diet record monitoring continued in free-living conditions. During this time, participants were instructed to minimise any unnecessary physical activity (e.g., walking around at home was permitted but structured exercise was not) and to eat only the standardized meals provided.
The standardized 24-h diet consisted of four meals ingested at predetermined time points (lunch at 15:00, dinner at 19:00, breakfast at 07:00, lunch at 12:00). The first meal (lunch) was consumed after 30-min seated rest following the cessation of the ± exercise period, after which participants were free to leave the laboratory. The standardized 24-h diet provided 2467.6 ± 4.6 kcals and consisted of a mixed macronutrient composition (54.9 ± 0.1% of energy from carbohydrates, 31.6 ± 0.1% from fat, and 13.5 ± 0.1% from protein). Participants were instructed to consume meals within a 20-min timeframe and recorded the exact meal ingestion start and end times to allow specific data to be extracted from CGM data files. This approach ensured that the dietary intake (food type, amount and timing) was standardized within-subjects across all four trials; an important approach frequently adopted when using CGM to evaluate exercise-induced changes in measures of glycemic control in free-living settings (20, 21, 38, 40–43). In the afternoon of day 3 (≥24 h following exercise cessation), participants reported to the laboratory for removal of CGM, accelerometer and collection of diet records and activity logs, marking the end of the trial.
It should be noted that no additional calories were provided to replace those expended during exercise in the exercise trials (NG-Ex, HG-Ex, FG-Ex) compared to CON (Table 3), or to account for extra calories gained from glucose infusions in HG-Ex and FG-Ex (83.5 ± 2.8 g of glucose infused, equating to 333.8 ± 11.2 kcals). Resultant energy balance induced by experimental interventions (i.e., crudely calculated as kcal gained during infusion minus kcal expended during exercise) is as follows: CON = 61.7 ± 3.6 kcals deficit; NG-Ex = 326.1 ± 18.8 kcals deficit; HG-Ex = 10.9 ± 20.5 kcals surplus; FG-Ex = 9.8 ± 17.7 kcals surplus). Although caloric imbalances between trials may be a confounder (44), accounting for imbalances between trials with the provision of food, for example, would result in differences in macronutrient provision between trials, which in itself would also be a confounding variable. Therefore, the meals were kept constant across all conditions.
Blood Sample Analyses
HbA1c was measured in capillary blood samples during the screening visit (HemoCue HbA1c 501, Radiometer, Copenhagen, Denmark). Venous blood samples were collected in pre-chilled EDTA-coated tubes and centrifuged at 2,000 g for 15 min at 4°C, with resulting plasma samples stored at −80°C until analysis. Plasma insulin (DINS00), plasma IL-6 (HS600C), and plasma CRP (DCRP00) concentrations were determined using solid-phase sandwich ELISAs (Quantikine, Biotechne, R&D). Plasma glucose concentrations were measured using a HemoCue Glucose 201+ analyzer (Radiometer, Copenhagen, Denmark).
Calculations
Diet records were analyzed using the UK food database within MyFitnessPal (Under Armour, Baltimore, MD). The 24-h pre-trial glucose and physical activity data were derived from CGM and Actigraph data, respectively, from 08:00 on the day before the trial to 08:00 on the day of the trial. The 24-h post-trial glucose and physical activity data were derived from CGM and Actigraph data, respectively, from the end of exercise until 24-h later. CGM-derived measures of glucose exposure (mean glucose and incremental area under the curve, iAUC) and glycemic variability (standard deviation and coefficient of variation, CV%) were calculated following clinical guidelines (45). The 24-h prevalence of hyperglycemia (time spent above 8 mmol/L) and hypoglycemia (time below 4 mmol/L) were also calculated.
Postprandial glucose control was defined as the iAUC above pre-meal glucose level (average of 30-min pre-meal) over the 2-h postprandial period for four individual meals (lunch, 15:00; dinner, 19:00; breakfast, 07:00; snack, 12:00). Total postprandial glucose iAUC was also calculated as the summation of iAUCs from all four meal periods. Such approach has been used frequently when assessing the effects of exercise on postprandial glucose outside of the laboratory (18, 20, 21). Post-absorptive insulin sensitivity was estimated using the homeostasis model assessment (HOMA-IR) (46). Whole-body substrate oxidation rates were calculated from V̇O2 and V̇CO2 at rest (47) and during exercise (48).
Statistical Analysis
Either one- or two-way repeated-measures ANOVA were used where appropriate, and in the case of significant interaction effects, pairwise comparisons were made with Bonferroni corrections. Effect sizes for post hoc pairwise comparisons were calculated using Cohen’s d and are presented in the figure legends. All analyses were performed in GraphPad Prism 7.0 (La Jolla, CA) with a value of P < 0.05 considered to be statistically significant. Data are presented as mean ± SEM.
Results
Participant Characteristics and Pre-Trial Standardization
Table 1 presents characteristics and habitual physical activity levels of participants recruited to complete the study. Table 2 confirms adherence to pre-trial diet and activity standardizations, showing no between-trial differences in dietary intake, physical activity or CGM-derived glycemic control collected during 24-h pre-trial. There were also no significant changes in body composition (body mass, BMI and waist circumference) between trials (P > 0.05, data not shown).
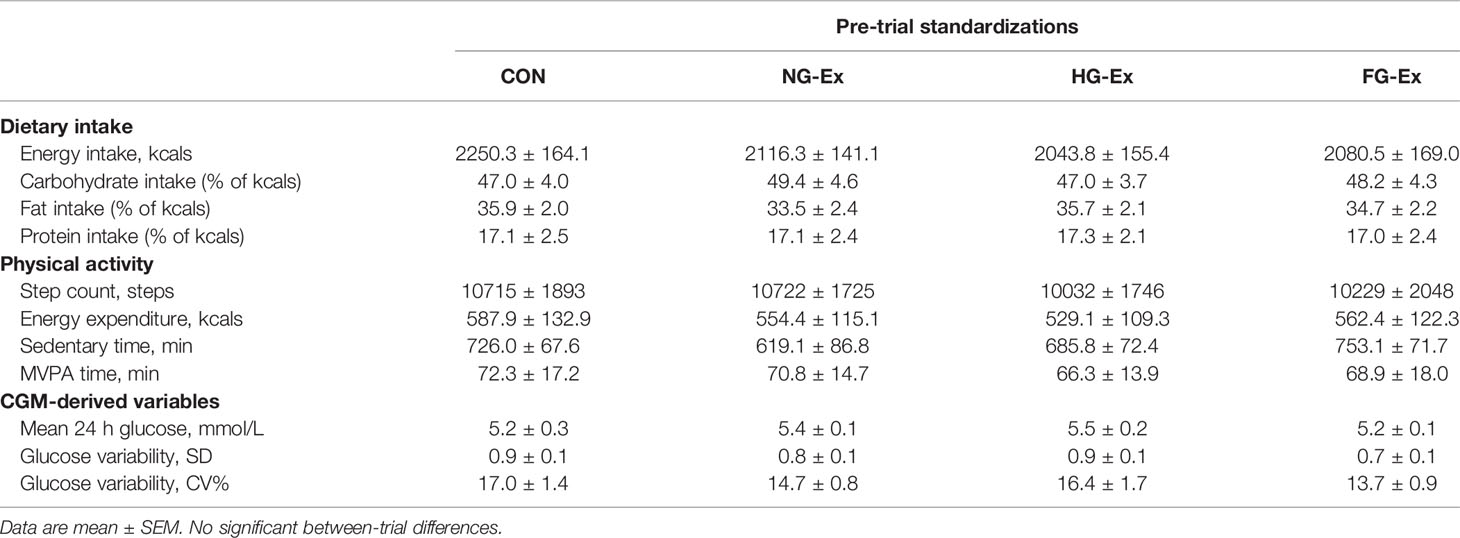
Table 2 Physical activity, dietary intake and free-living CGM variables measured for 24 h before each experimental trial.
Blood Biochemistry During the Experimental Trials
Figure 2A shows that the glucose infusion protocol successfully induced three distinct glycemic profiles: normoglycemic (CON and NG-Ex) and two different hyperglycemic conditions (HG-Ex and FG-Ex). Mean glucose concentration (Figure 2B) and glucose iAUC (Figure 2C) during the 3.5 h ± infusion period were significantly higher during HG-Ex and FG-Ex compared to CON and NG-Ex (all comparisons P < 0.05), with no differences between CON and NG-Ex (P > 0.05). Figure 2D confirms that the FG-Ex trial had an unstable and fluctuating glucose profile, since CV%, a measure of glycemic variability, was significantly higher than in all other trials (all comparisons P < 0.05).
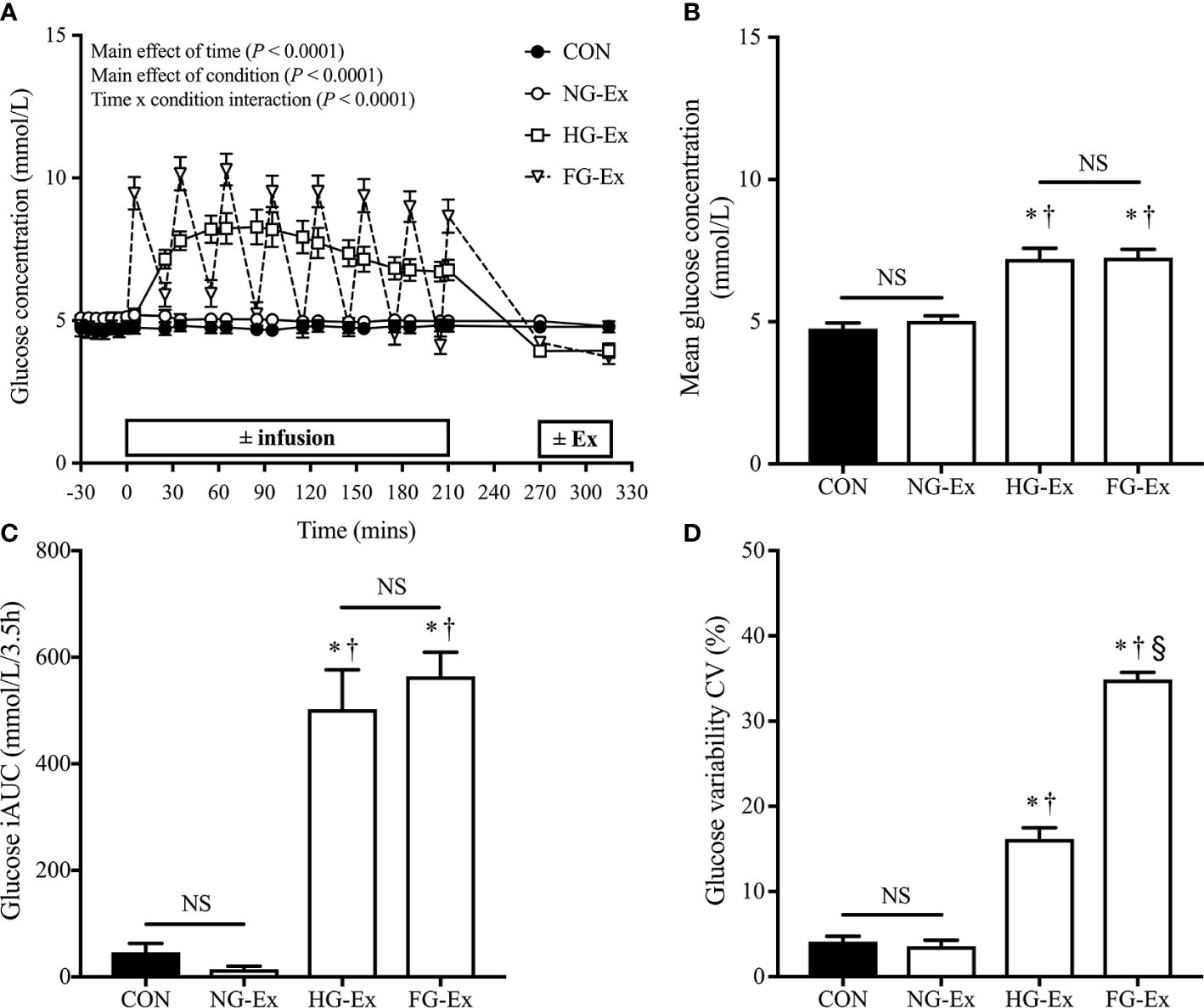
Figure 2 Glucose control during conditions of ± glucose infusion and ± exercise. (A) Time course for glucose concentration (mmol/L) during each experimental trial. (B) The mean glucose concentration (mmol/L) during each 3.5 h ± infusion period. *P < 0.05 (HG-Ex, d = 2.38; FG-Ex, d = 4.02) vs. CON. †P < 0.05 (HG-Ex, d = 2.17; FG-Ex, d = 3.83) vs. NG-Ex. (C) Glucose iAUC during each 3.5 h ± infusion period. *P < 0.05 (HG-Ex, d = 2.47; FG-Ex, d = 4.40) vs. CON. †P < 0.05 (HG-Ex, d = 2.70; FG-Ex, d = 4.94) vs. NG-Ex. (D) Glycemic variability (coefficient of variation; %CV) during each 3.5 h ± infusion period. *P < 0.05 (HG-Ex, d = 3.35; FG-Ex, d = 12.05) vs. CON. †P < 0.05 (HG-Ex, d = 3.38; FG-Ex, d = 11.45) vs. NG-Ex. §P < 0.05 (FG-Ex, d = 4.84) vs. HG-Ex.
Figure 3A shows that plasma insulin concentrations were significantly increased post-infusion vs. pre-infusion in both HG-Ex and FG-Ex (both P < 0.0001 vs. respective baseline), but not NG-Ex and CON (P > 0.05). Post-infusion values in HG-Ex and FG-Ex were also significantly higher than time-matched values in NG-Ex and CON (P < 0.0001 vs. time-matched values). There was no significant interaction effect of experimental treatments upon plasma IL-6 (Figure 3B) or plasma CRP (Figure 3C) concentrations (P > 0.05).
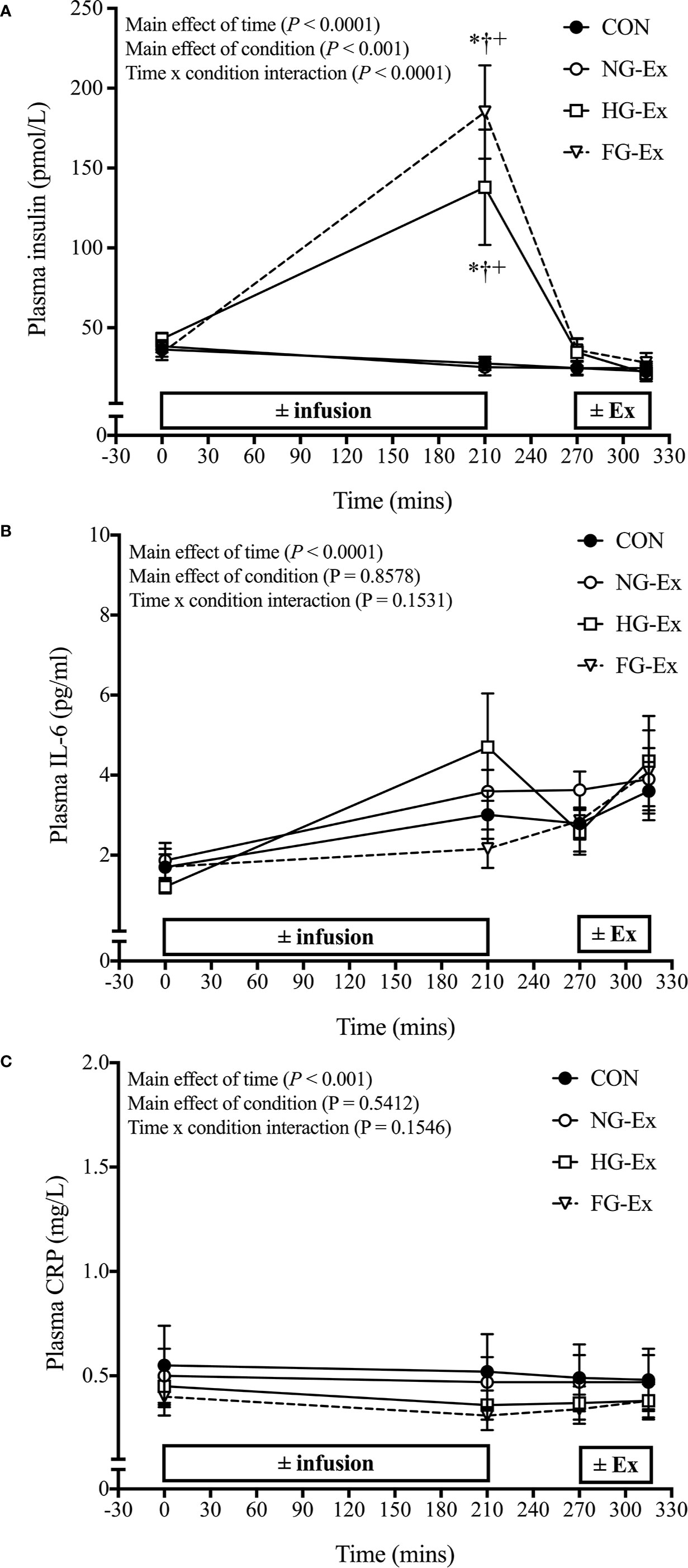
Figure 3 Time course for changes in plasma insulin, IL-6, and CRP. (A) Time course for plasma insulin (pmol/L) during each experimental trial. *P < 0.0001 (HG-Ex, d = 1.24; FG-Ex, d = 2.18) vs. time-matched CON; †P < 0.0001 (HG-Ex, d = 1.26; FG-Ex, d = 2.20) vs. time-matched NG-Ex; +P < 0.0001 (HG-Ex, d = 1.07; FG-Ex, d = 2.09) vs. pre-infusion. (B) Time course for IL-6 concentration (pg/mL) during each experimental trial. (C) Time course for CRP (mg/L) during each experimental trial.
Physiological Responses to Exercise During the Trials
The exercise/rest period lasted 44.6 ± 0.4 min and characteristics of these periods are shown in Table 3. Heart rate, power output, V̇O2, work done, and energy expenditure were similar between the three exercise trials (P > 0.05) and significantly higher than during the resting trial (P < 0.05). RER was significantly higher during the three exercise trials vs. CON (P < 0.05; Table 3), and also significantly higher in HG-Ex and FG-Ex compared to NG-Ex (P < 0.05; Table 3). Furthermore, carbohydrate oxidation rates during exercise/rest were significantly higher in all three exercise trials (NG-Ex, HG-Ex, and FG-Ex) compared to CON (P < 0.05), with rates during HG-Ex and FG-Ex also significantly higher than NG-Ex (P < 0.05; Figure 4A). Fat oxidation rates during exercise in NG-Ex were significantly higher than all other trials (P < 0.05), with no further between-condition differences (P > 0.05; Figure 4B).
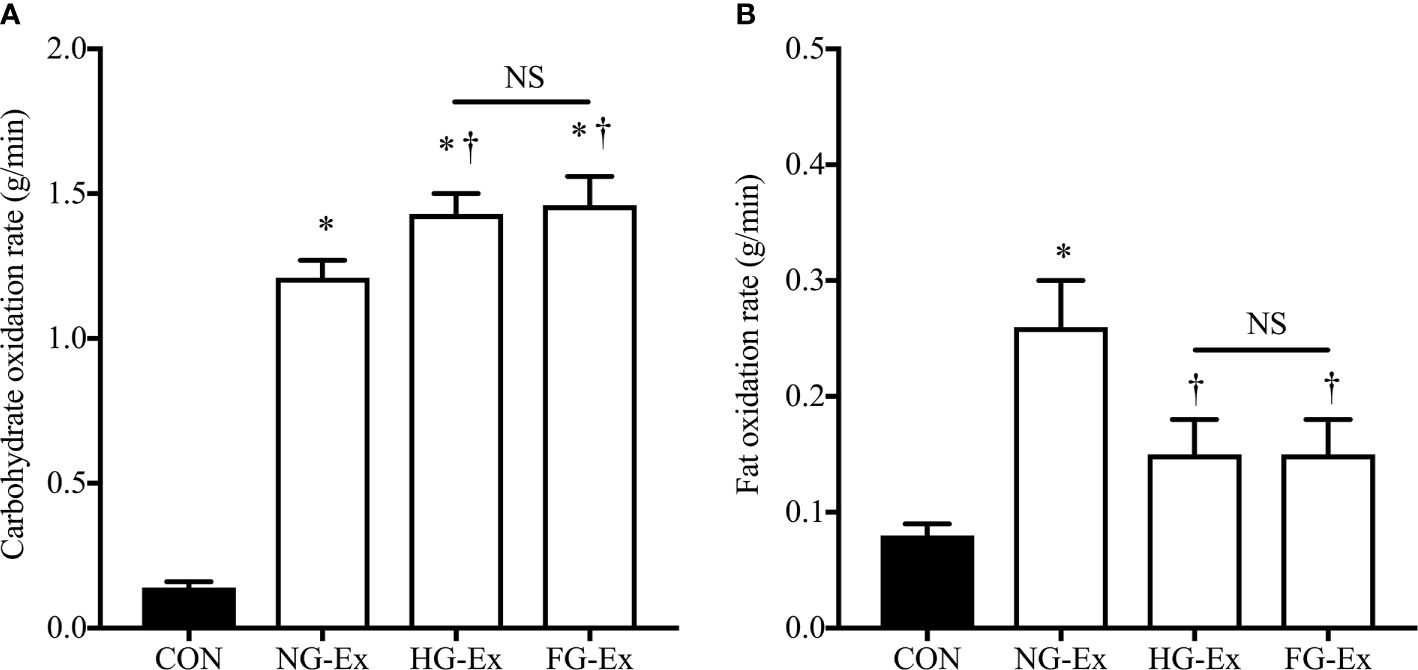
Figure 4 Rates of substrate oxidation during exercise. (A) Carbohydrate oxidation rates during the 45-min ± exercise period within the four experimental trials. *P < 0.05 (NG-Ex, d = 6.85; HG-Ex, d = 7.06; FG-Ex, d = 5.18) vs. CON. †P < 0.05 (HG-Ex, d = 0.96; FG-Ex, d = 0.84) vs. NG-Ex. (B) Fat oxidation rates during the 45-min ± exercise period within the four experimental trials. *P < 0.05 (NG-Ex, d = 1.81) vs. CON. †P < 0.05 (HG-Ex, d = 0.90; FG-Ex, d = 0.89) vs. NG-Ex.
Post-Trial Standardization
Table 4 confirms that both dietary intake and physical activity levels during the 24-h post-trial period were similar between trials (P > 0.05).
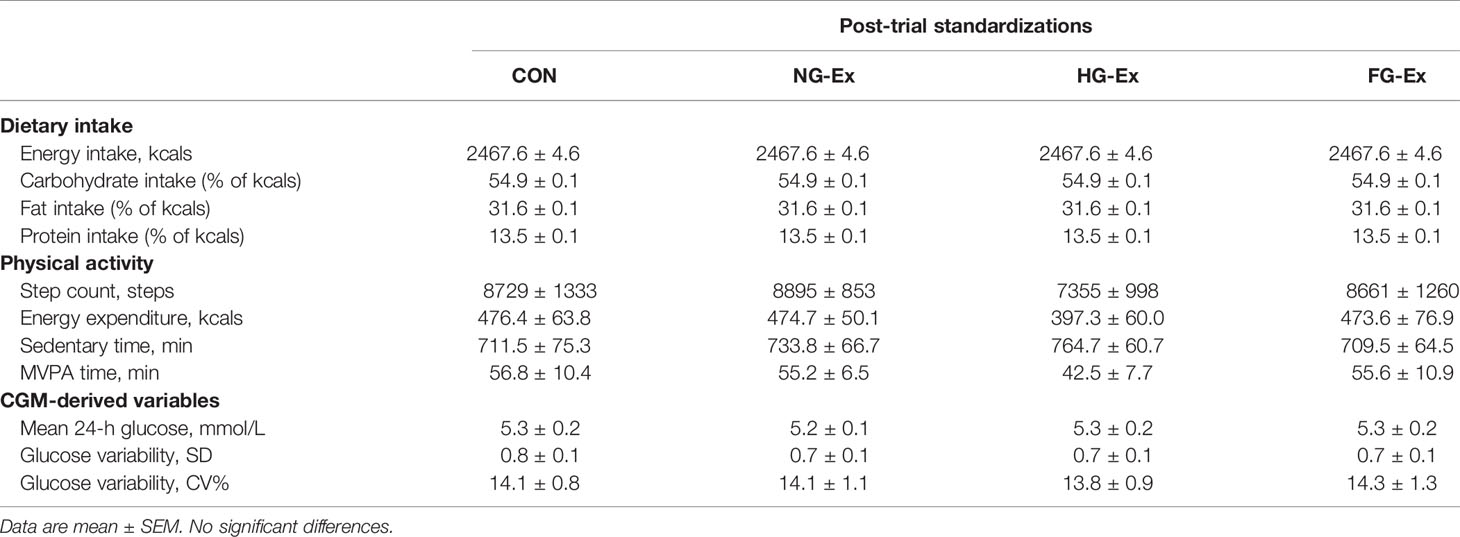
Table 4 Physical activity, dietary intake, and free-living CGM variables measured for 24 h following each experimental trial.
Post-Trial 24-h Glycemic Control
There were no significant between-condition differences in 24-h glucose exposure (i.e., mean 24-h glucose concentrations) during the post-exercise period (P > 0.05; Table 4). Since the regulatory mechanisms (e.g., changes in glucose uptake and insulin sensitivity) of exercise-induced glucoregulatory benefits follow time-dependent profiles (15), the 24-h post-exercise period was broken down to discreet periods, namely: 0–6, 0–12, 0–18, 6–12, 6–18, 6–24, 12–18, 12–24, and 18–24 h. However, there were no significant between-condition differences in mean glucose during these periods (P > 0.05). Measures of 24-h glycemic variability, namely, SD and %CV, were not significantly affected by the experimental conditions (Table 4, both P > 0.05). Similarly, there were no significant between-condition differences in the 24-h prevalence of hyperglycemia or hypoglycemia (both P > 0.05; data not shown).
Post-Trial Postprandial Glycemic Control
There were no significant between-condition differences in mean glucose or glucose iAUC in the 2-h postprandial period following the four meals provided when analyzed per meal (P > 0.05). However, as presented in Figure 5, total postprandial glucose response, measured as the sum of post-meal glucose iAUC, was significantly reduced by exercise in NG-Ex compared to CON (321.1 ± 38.6 mmol/L.8h vs. 445.5 ± 49.7 mmol/L.8h, P < 0.05). However, such benefits were blunted when exercise was preceded by steady-state hyperglycemia (425.3 ± 45.7 mmol/L.8h) and fluctuating glycemia (465.5 ± 39.3 mmol/L.8h) in HG-Ex and FG-Ex, respectively (both P > 0.05 vs. CON).
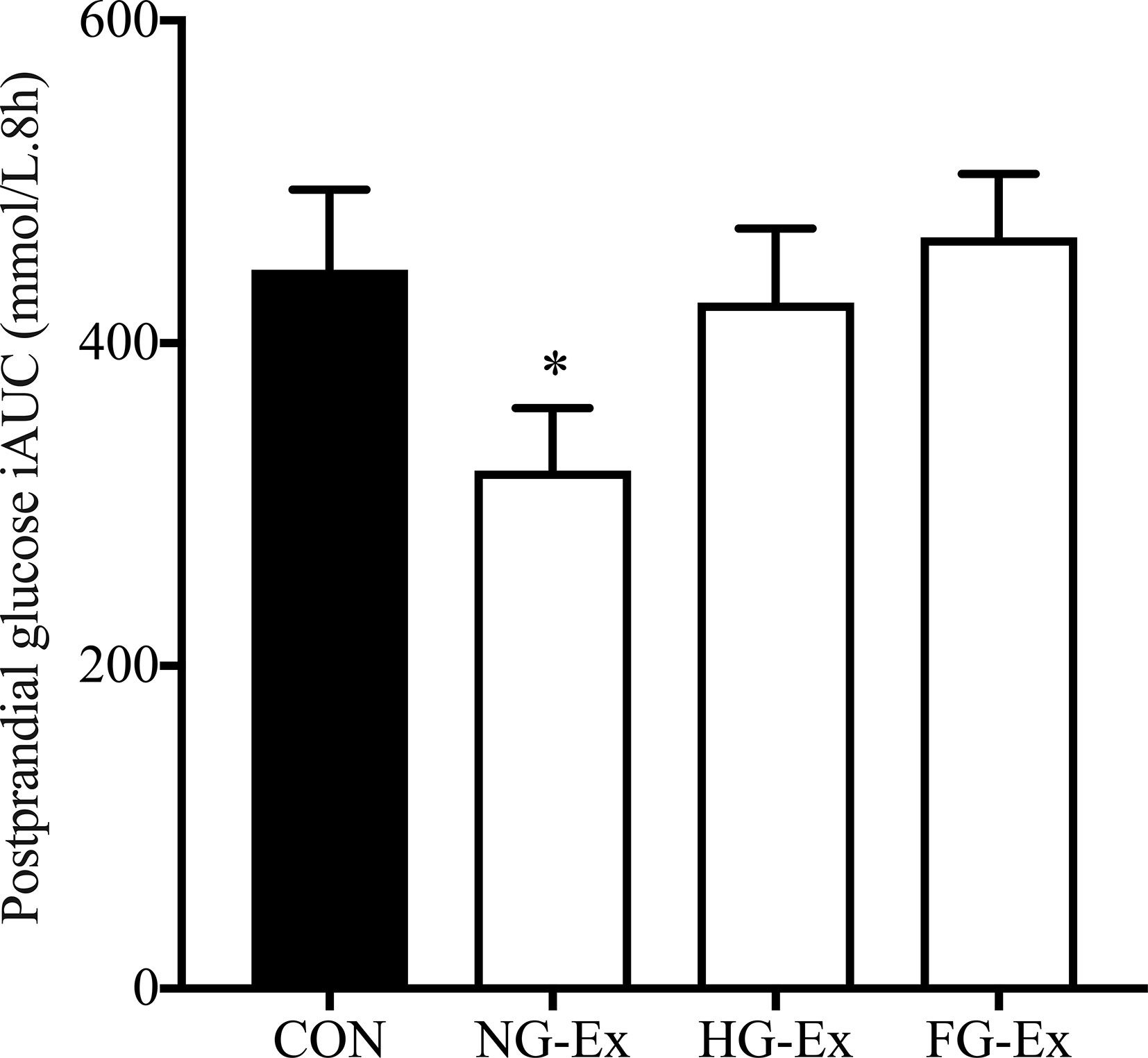
Figure 5 The effects of the four interventions on total postprandial glucose iAUC. Total postprandial glucose response (the sum of post-meal glucose iAUC across four standardized meals) was measured using CGM following four experimental trials. *P < 0.05 (NG-Ex, d = 0.81) vs. CON.
Discussion
The current study is the first to experimentally investigate the direct effect of hyperglycemia on exercise-induced benefits in glucose control. The findings demonstrate that pre-exercise hyperglycemia blunts the exercise-induced improvement in postprandial glucose response following a single exercise bout in healthy normal glucose-tolerant individuals.
Excessive postprandial glucose exposure contributes to the worsening of HbA1c in non-diabetic individuals (7), as well as exacerbating the risk of developing diabetic complications and premature mortality (4, 6). Accordingly, reducing postprandial glucose is a prime therapeutic target in the prevention and management of hyperglycemia-related conditions, such as T2DM. In this study, when exercise was preceded by normoglycemia, total postprandial glucose iAUC was significantly reduced compared to the non-exercise CON condition (d = 0.81; Figure 5). While reference ranges for CGM-derived treatment targets are constantly being refined (45, 49), a reference range for what constitutes a clinically important exercise-induced change in a CGM-derived variable does not currently exist. Nevertheless, the improved postprandial glucose response in the current study is in agreement with previous research also demonstrating the potency of exercise in improving this specific CGM-derived measure of glycemic control (18, 20, 21). However, this beneficial effect was blunted when the same individuals were exposed to steady-state hyperglycemia or fluctuating glycemia before exercise (Figure 5). This is the first experimental evidence demonstrating a direct effect of pre-exercise hyperglycemia upon the potency of exercise to induce glucoregulatory benefits. The findings, therefore, support that hyperglycemia contributes to the inter-individual heterogeneity of the metabolic response to exercise.
The magnitude and profile of an individual’s postprandial glucose response are determined by multiple factors. These include meal characteristics such as caloric content and macronutrient composition, but also the nutrient-induced gastrointestinal responses, tissue glucose uptake, insulin sensitivity, pancreatic β-cell insulin secretory function, glucose effectiveness, and hepatic and renal glucose handling (50–53), all of which are regulated by exercise. Therefore, the exercise-induced enhancement in postprandial glucose control seen in the NG-Ex trial is likely attributable to coordinated exercise-mediated enhancements in any of the above factors. As a corollary, if pre-exercise hyperglycemia impairs any of these adaptive processes, this would feasibly blunt exercise-induced improvements in postprandial glucose response. This notion is supported by evidence demonstrating that chronic hyperglycemia is associated with smaller exercise-induced improvements in peripheral insulin sensitivity, which in turn was linked with blunted exercise-induced improvements in postprandial glucose response (54).
Furthermore, hyperglycemia impairs skeletal muscle cell insulin sensitivity (55–58), pancreatic β-cell insulin secretory capacity (59, 60), and endothelial cell function (61, 62) within in vitro experimental models. Likewise, skeletal muscle (63, 64) and hepatic (65) insulin sensitivity, insulin secretory capacity (63), and vascular function (66) are impaired by exposure to experimental hyperglycemia in vivo, even in healthy individuals with normal glucose tolerance. Such hyperglycemia-induced impairments could also explain the elevated postprandial glucose response following the HG-Ex and FG-Ex trials.
Impaired function of key glucoregulatory tissues, including muscle, liver, and the endocrine pancreas, may also contribute indirectly to poorer postprandial glucose response following HG-Ex and FG-Ex since the impaired function of these tissues at baseline has also been associated with poorer outcomes following exercise. For example, blunted exercise-induced improvements in insulin sensitivity have been documented in individuals with higher baseline insulin resistance (54), and poorer contraction-induced improvements in glucose metabolism and insulin sensitivity has been found in primary myotubes from insulin resistant (e.g., obese and/or T2DM) vs. healthy donors (67, 68). Similarly, poorer baseline pancreatic β-cell function is predictive of poorer exercise responsiveness (33). Collectively, in this study, pre-exercise hyperglycemia may have impaired the function of glucoregulatory tissues (muscle, pancreas, etc.) while also blunting their ability to respond to exercise.
Markers of inflammation and oxidative stress are elevated in T2DM and are increased by experimental hyperglycemia (66). Since excessive levels of inflammation and oxidative stress can impair exercise adaptations (69), pre-exercise hyperglycemia-induced inflammation and oxidative stress may influence exercise-induced benefits. However, we found no significant interaction effect in two markers of systemic inflammation (IL-6 and CRP; Figure 3) suggesting that inflammation may not explain our findings. That said, tissue inflammation was not examined and these outcomes may be different in a patient population. Alternatively, pre-exercise hyperglycemia appeared to promote carbohydrate utilization (Figure 4A) and suppress fat utilization (Figure 4B) during exercise compared to exercise under normoglycemic conditions despite matched exercise stimuli (Table 3). Such alterations in substrate use during exercise may contribute to altered glucoregulation during the post-exercise period.
In contrast to the exercise-induced improvements in postprandial glucose, there were no significant effects of exercise upon mean 24-h glucose concentration, glucose SD or glucose CV%. This contradicts the improvements in 24-h glycemic control seen in hyperglycemic participants (20, 38, 40, 70). The smaller margin for improvement in the normal glucose-tolerant participants in the current study (HbA1c = 5.4 ± 0.1%) compared to the aforementioned studies in hyperglycemic individuals may contribute to this discrepancy. Interestingly, there were also no significant effects of HG-Ex or FG-Ex on these markers of 24-h glycemic control compared to either normoglycemic condition, highlighting that the ability of healthy humans to regulate glucose homeostasis under a challenge from distinctly different stimuli (i.e., exercise and hyperglycemia) is remarkably well-preserved.
Given the aim of the current study to investigate possible contributing factors to response heterogeneity in glycemic improvements following exercise, it would be remiss to not discuss variability in outcomes. Inter-individual heterogeneity showed that 9, 7, and 7 out of 12 individuals showed a decrease in 24-h mean glucose, glucose SD, and glucose CV%, respectively, following exercise. This variability in responses likely contributes to the lack of significance seen for these outcomes. A further anecdotal observation is that pre-exercise hyperglycemia in HG-Ex and FG-Ex reduced the participants’ enjoyment during exercise when compared to NG-Ex. This is important because reduced enjoyment during exercise could feasibly impact exercise adherence in a free-living setting.
In the present study, healthy, recreationally active, non-diabetic individuals were exposed to blood glucose profiles similar to those seen in T2DM (i.e., elevated and unstable) for 3.5 h. It is important to note that the short-term, acute nature of this likely represents a different metabolic and physiological challenge compared to the chronic state of hyperglycemia and/or glycemic instability in T2DM. Nevertheless, the approach used enabled us to isolate the effect of acute hyperglycemia from other symptoms or comorbidities of T2DM (e.g., chronic low-grade inflammation, dyslipidaemia, impaired insulin sensitivity and/or secretion, etc.), while also avoiding changes in circulating incretin hormones (e.g., GLP-1) otherwise induced by oral glucose ingestion (71), thus justifying the study design used. That said, since pancreatic clamp conditions were not employed during glucose infusion in the current study, the possibility that increased plasma insulin in hyperglycemic (HG-Ex and FG-Ex) vs. normoglycemic (NG-Ex and CON) conditions (Figure 3A) contributed to the responses seen cannot be excluded. Additionally, short-term hyperglycemia, such as during a 2-h hyperglycemic clamp, reduces plasma glucagon concentration, and suppresses endogenous glucose production in healthy individuals (63), and such effects may contribute to responses observed in the current study. Furthermore, since exercise in the current study is acute and of moderate-intensity, whether exercise within training regimes and/or of greater intensity elicits the same outcomes remains to be determined. Future studies should also aim to quantify the long-term impact of changes in CGM-derived outcomes on the risk of developing hyperglycemia-related conditions/complications.
Using glucose infusion to induce hyperglycemia introduced caloric and carbohydrate imbalances between trials. Specifically, there was an energy deficit in CON (−61.7 ± 3.6 kcals) and NG-Ex (−326.1 ± 18.8 kcals) and a slight energy surplus in HG-Ex (+10.9 ± 20.5 kcals) and FG-Ex (+9.8 ± 17.7 kcals). Similarly, while more carbohydrate was oxidized during exercise within the infusion trials compared to NG-Ex (Figure 4A), this difference does not account for the amount of glucose infused during HG-Ex and FG-Ex. While the thermic effect of glucose infusion itself, which increases resting energy expenditure (by ~5%–11%) (72–74) and carbohydrate oxidation (75), may attenuate these between-trial imbalances to some extent, the possibility that they contributed to findings cannot be excluded. Excess glucose may have increased liver (76) and skeletal muscle (64) glycogen storage which may impact the capacity for postprandial glucose disposal during the post-exercise period in the infusion trials only. That said, while glucose infusion to maintain glucose concentrations at +2.5 mmol/L above fasting for 3 days doubled muscle glycogen (64), the short-term, acute nature of infusion in the current study (3.5 h) is unlikely to induce such a stark increase. Additionally, moderate-intensity exercise still reduces muscle glycogen under conditions of experimental hyperglycemia (via glucose infusion) (77) and when pre-exercise muscle glycogen is elevated (78). Furthermore, post-exercise glycogen storage is not solely reliant on changes at the muscle itself, but also splanchnic bed responses that increase the rate of oral glucose appearance in the circulation (79).
Compensating for the aforementioned imbalances with altered food provision without several additional control trials would introduce other confounding variables (e.g., differences in macronutrient provision), meaning that imbalance of some form is somewhat inevitable. Therefore, in line with many studies evaluating the effects of exercise vs. rest (20, 21, 38) or normo- vs. hyperglycemia (63, 64) upon glucose metabolism, compensation of these caloric and carbohydrate imbalances were not included, with the meals kept constant across all conditions. Moreover, this should not detract from the significance of the findings since the exercise-induced improvements in the function of key glucoregulatory tissues (e.g., muscle, pancreas, liver, gastrointestinal tract, etc.) that contribute to improved postprandial glucose result from more than simply an energy and glycogen deficit.
A key methodological strength of the current study is the balance between having strict control of pre-trial and post-trial standardization, glycemic interventions, exercise interventions, and dietary control, while monitoring clinically relevant outcomes (i.e., CGM-derived glycemic control) in an otherwise free-living, ecologically-valid setting. Additionally, unlike some previous exercise vs. control studies using CGM-derived outcomes (18, 70, 80), trial order was randomized to minimise the risk of bias. The consumption of the same controlled diet and the same physical activity levels across all 24-h post-trial periods increases the confidence that observed effects upon postprandial glucose are likely attributable to differences in the experimental treatments (i.e., ± glucose infusion, ± exercise) during the respective trials.
In the context of ecological validity, this study implicates a direct inhibitory effect of pre-exercise hyperglycemia upon exercise-induced improvements in postprandial glucose control. Therefore, it could be speculated that reducing hyperglycemia before exercise and/or coinciding exercise sessions with periods of improved glucose control (i.e., lower, more stable levels) may be a necessary strategy for optimizing the therapeutic effects of exercise in individuals with hyperglycemia-related conditions, such as T2DM. Hyperglycemia-lowering medication, dietary interventions, or optimizing exercise-meal timing may provide viable options in this regard—that said, both diet and medication have themselves been implicated in variable exercise responsiveness (27, 30). Therefore, an individualized approach to glucose management will likely be best and more work in that area is required. Future work must also resolve how the findings translate to longer-term exercise training interventions in patients with T2DM before extrapolation to disease management. That said, large portions of the worldwide population are non-diabetic meaning these results hold clinical significance in the context of disease prevention.
In conclusion, this study provides the first experimental evidence that pre-exercise hyperglycemia can blunt the glucoregulatory benefits of acute exercise, suggesting that hyperglycemia contributes to exercise response heterogeneity. Resolving hyperglycemia before exercise and/or coinciding exercise sessions with periods of improved glucose control may, therefore, be vital for maximizing the therapeutic effects of exercise in individuals with and at risk of hyperglycemia-related conditions, such as T2DM.
Data Availability Statement
The raw data supporting the conclusions of this article will be made available by the authors, without undue reservation.
Ethics Statement
Ethical approval was obtained through the West Midlands - South Birmingham Research Ethics Committee (16/WM/0242) and sponsored by the University of Birmingham Research Governance. All participants provided written informed consent before participation.
Author Contributions
SC and TPJS conceived the idea, designed the study, and implemented the trials. SC completed the data analysis and wrote the manuscript. SC and TPJS interpreted the findings and discussed the data. TPJS edited the manuscript. All authors agree to be accountable for the content of the work. All authors contributed to the article and approved the submitted version.
Funding
The study was funded in part by the Physiological Society and the European Commission (Marie Skłodowska-Curie Individual Fellowship).
Conflict of Interest
The authors declare that the research was conducted in the absence of any commercial or financial relationships that could be construed as a potential conflict of interest.
Acknowledgments
The authors thank the research participants for their time, Ascensia Diabetes Care UK for the provision of Contour Next One blood glucose monitors, and Andy Nicholson at Dexcom UK for his analytical and technical support throughout the study.
Supplementary Material
The Supplementary Material for this article can be found online at: https://www.frontiersin.org/articles/10.3389/fendo.2020.566548/full#supplementary-material
References
1. Rubin J, Matsushita K, Ballantyne CM, Hoogeveen R, Coresh J, Selvin E. Chronic hyperglycemia and subclinical myocardial injury. J Am Coll Cardiol (2012) 59:484–9. doi: 10.1016/j.jacc.2011.10.875
2. Sarwar N, Gao P, Kondapally Seshasai SR, Gobin R, Kaptoge S, Di Angelantonio E, et al. Diabetes mellitus, fasting blood glucose concentration, and risk of vascular disease: A collaborative meta-analysis of 102 prospective studies. Lancet (2010) 375:2215–22. doi: 10.1016/S0140-6736(10)60484-9
3. Ceriello A. Hyperglycemia and the vessel wall: the pathophysiological aspects on the atherosclerotic burden in patients with diabetes. Eur J Cardiovasc Prev Rehabil (2010) 17:S15–9. doi: 10.1097/01.hjr.0000368193.24732.66
4. Marini MA, Succurro E, Castaldo E, Cufone S, Arturi F, Sciacqua A, et al. Cardiometabolic risk profiles and carotid atherosclerosis in individuals with prediabetes identified by fasting glucose, postchallenge glucose, and hemoglobin A 1c criteria. Diabetes Care (2012) 35:1144–9. doi: 10.2337/dc11-2032
5. Cavalot F. Do data in the literature indicate that glycemic variability is a clinical problem? Glycemic variability and vascular complications of diabetes. Diabetes Obes Metab (2013) 15:3–8. doi: 10.1111/dom.12140
6. Cavalot F, Petrelli A, Traversa M, Bonomo K, Fiora E, Conti M, et al. Postprandial blood glucose is a stronger predictor of cardiovascular events than fasting blood glucose in type 2 diabetes mellitus, particularly in women: Lessons from the San Luigi Gonzaga diabetes study. J Clin Endocrinol Metab (2006) 91:813–9. doi: 10.1210/jc.2005-1005
7. Færch K, Alssema M, Mela DJ, Borg R, Vistisen D. Relative contributions of preprandial and postprandial glucose exposures, glycemic variability, and non-glycemic factors to HbA1c in individuals with and without diabetes. Nutr Diabetes (2018) 8:0–8. doi: 10.1038/s41387-018-0047-8
8. Boniol M, Dragomir M, Autier P, Boyle P. Physical activity and change in fasting glucose and HbA1c: a quantitative meta-analysis of randomized trials. Acta Diabetol (2017) 54:983–91. doi: 10.1007/s00592-017-1037-3
9. Colberg SR, Sigal RJ, Yardley JE, Riddell MC, Dunstan DW, Dempsey PC, et al. Physical activity/exercise and diabetes: A position statement of the American Diabetes Association. Diabetes Care (2016) 39:2065–79. doi: 10.2337/dc16-1728
10. Kasumov T, Solomon TPJ, Hwang C, Huang H, Haus JM, Zhang R, et al. Improved insulin sensitivity after exercise training is linked to reduced plasma C14:0 ceramide in obesity and type 2 diabetes. Obesity (2015) 23:1414–21. doi: 10.1002/oby.21117
11. Meex RCR, Schrauwen-Hinderling VB, Moonen-Kornips E, Schaart G, Mensink M, Phielix E, et al. Restoration of muscle mitochondrial function and metabolic flexibility in type 2 diabetes by exercise training is paralleled by increased myocellular fat storage and improved insulin sensitivity. Diabetes (2010) 59:572–9. doi: 10.2337/db09-1322
12. Kirwan JP, Solomon TPJ, Wojta DM, Staten MA, Holloszy JO. Effects of 7 days of exercise training on insulin sensitivity and responsiveness in type 2 diabetes mellitus. Am J Physiol Metab (2009) 297:E151–6. doi: 10.1152/ajpendo.00210.2009
13. Dela F, von Linstow ME, Mikines KJ, Galbo H. Physical training may enhance β-cell function in type 2 diabetes. Am J Physiol Metab (2004) 287:E1024–31. doi: 10.1152/ajpendo.00056.2004
14. Solomon TPJ, Haus JM, Kelly KR, Rocco M, Kashyap SR, Kirwan JP. Improved pancreatic β-cell function in type 2 diabetic patients after lifestyle-induced weight loss is related to glucose-dependent insulinotropic polypeptide. Diabetes Care (2010) 33:1561–6. doi: 10.2337/dc09-2021
15. Sylow L, Richter EA. Current advances in our understanding of exercise as medicine in metabolic disease. Curr Opin Physiol (2019) 12:12–9. doi: 10.1016/j.cophys.2019.04.008
16. Knudsen SH, Karstoft K, Winding K, Holst JJ, Pedersen BK, Solomon TPJ. Effects of acute exercise on pancreatic endocrine function in subjects with type 2 diabetes. Diabetes Obes Metab (2015) 17:207–10. doi: 10.1111/dom.12413
17. Kearney ML, Thyfault JP. Exercise and postprandial glycemic control in type 2 diabetes. Curr Diabetes Rev (2016) 12:199–210. doi: 10.2174/1573399811666150615112441
18. Gillen JB, Little JP, Punthakee Z, Tarnopolsky MA, Riddell MC, Gibala MJ. Acute high-intensity interval exercise reduces the postprandial glucose response and prevalence of hyperglycemia in patients with type 2 diabetes. Diabetes Obes Metab (2012) 14:575–7. doi: 10.1111/j.1463-1326.2012.01564.x
19. MacLeod SF, Terada T, Chahal BS, Boulé NG. Exercise lowers postprandial glucose but not fasting glucose in type 2 diabetes: A meta-analysis of studies using continuous glucose monitoring. Diabetes Metab Res Rev (2013) 29:593–603. doi: 10.1002/dmrr.2461
20. Oberlin DJ, Mikus CR, Kearney ML, Hinton PS, Manrique C, Leidy HJ, et al. One bout of exercise alters free-living postprandial glycemia in type 2 diabetes. Med Sci Sports Exerc (2014) 46:232–8. doi: 10.1249/MSS.0b013e3182a54d85
21. van Dijk JW, Venema M, van Mechelen W, Stehouwer CDA, Hartgens F, van Loon LJC. Effect of moderate-intensity exercise versus activities of daily living on 24-hour blood glucose homeostasis in male patients with type 2 diabetes. Diabetes Care (2013) 36:3448–53. doi: 10.2337/dc12-2620
22. ADA. 5. Lifestyle management: Standards of medical care in diabetes — 2019. Diabetes Care (2019) 42:S46–60. doi: 10.2337/dc19-S005
23. van Dijk JW, Manders RJF, Canfora EE, Van Mechelen W, Hartgens F, Stehouwer CDA, et al. Exercise and 24-h glycemic control: Equal effects for all type 2 diabetes patients? Med Sci Sports Exerc (2013) 45:628–35. doi: 10.1249/MSS.0b013e31827ad8b4
24. Terada T, Friesen A, Chahal BS, Bell GJ, McCargar LJ, Boulé NG. Exploring the variability in acute glycemic responses to exercise in type 2 diabetes. J Diabetes Res (2013) 2013:591574. doi: 10.1155/2013/591574
25. Boulé NG, Weisnagel SJ, Lakka TA, Tremblay A, Bergman RN, Rankinen T, et al. Effects of exercise training on glucose homeostasis: the HERITAGE Family Study. Diabetes Care (2005) 28:108–14. doi: 10.2337/diacare.28.1.108
26. Bouchard C, Blair SN, Church TS, Earnest CP, Hagberg JM, Häkkinen K, et al. Adverse metabolic response to regular exercise: is it a rare or common occurrence? PLoS One (2012) 7:e37887. doi: 10.1371/journal.pone.0037887
27. Solomon TP. Sources of inter-individual variability in the therapeutic response of blood glucose control to exercise in type 2 diabetes: Going beyond exercise dose. Front Physiol (2018) 9:896. doi: 10.3389/fphys.2018.00896
28. Sparks LM. Exercise training response heterogeneity: Physiological and molecular insights. Diabetologia (2017) 60:2329–36. doi: 10.1007/s00125-017-4461-6
29. Böhm A, Weigert C, Staiger H, Häring HU. Exercise and diabetes: Relevance and causes for response variability. Endocrine (2016) 51:390–401. doi: 10.1007/s12020-015-0792-6
30. Færch K, Hulman A PJ. Solomon T. Heterogeneity of pre-diabetes and type 2 diabetes: Implications for prediction, prevention and treatment responsiveness. Curr Diabetes Rev (2015) 12:30–41. doi: 10.2174/1573399811666150416122903
31. Malin SK, Gerber R, Chipkin SR, Braun B. Independent and combined effects of exercise training and metformin on insulin sensitivity in individuals with prediabetes. Diabetes Care (2012) 35:131–6. doi: 10.2337/dc11-0925
32. Sharoff CG, Hagobian TA, Malin SK, Chipkin SR, Yu H, Hirshman MF, et al. Combining short-term metformin treatment and one bout of exercise does not increase insulin action in insulin-resistant individuals. Am J Physiol Endocrinol Metab (2010) 298:E815–23. doi: 10.1152/ajpendo.00517.2009
33. Solomon TPJ, Malin SK, Karstoft K, Kashyap SR, Haus JM, Kirwan JP. Pancreatic β-cell function is a stronger predictor of changes in glycemic control after an aerobic exercise intervention than insulin sensitivity. J Clin Endocrinol Metab (2013) 98:4176–86. doi: 10.1210/jc.2013-2232
34. AbouAssi H, Slentz CA, Mikus CR, Tanner CJ, Bateman LA, Willis LH, et al. The effects of aerobic, resistance, and combination training on insulin sensitivity and secretion in overweight adults from STRRIDE AT/RT: a randomized trial. J Appl Physiol (2015) 118:1474–82. doi: 10.1152/japplphysiol.00509.2014
35. Malin SK, Kirwan JP. Fasting hyperglycemia blunts the reversal of impaired glucose tolerance after exercise training in obese older adults. Diabetes Obes Metab (2012) 14:835–41. doi: 10.1111/j.1463-1326.2012.01608.x
36. Faul F, Erdfelder E, Lang AG, Buchner A. G*Power 3: A flexible statistical power analysis program for the social, behavioral, and biomedical sciences. Behav Res Methods (2007) 39:175–91. doi: 10.3758/BF03193146
37. Jakobsen I, Solomon TPJ, Karstoft K. The acute effects of interval-type exercise on glycemic control in type 2 diabetes subjects: Importance of interval length. A controlled, counterbalanced, crossover study. PLoS One (2016) 11:1–15. doi: 10.1371/journal.pone.0163562
38. Manders RJF, Van Dijk JWM, Van Loon LJC. Low-intensity exercise reduces the prevalence of hyperglycemia in type 2 diabetes. Med Sci Sports Exerc (2010) 42:219–25. doi: 10.1249/MSS.0b013e3181b3b16d
39. Karstoft K, Christensen CS, Pedersen BK, Solomon TPJ. The acute effects of interval- vs continuous-walking exercise on glycemic control in subjects with type 2 diabetes: A crossover, controlled study. J Clin Endocrinol Metab (2014) 99:3334–42. doi: 10.1210/jc.2014-1837
40. van Dijk JW, Tummers K, Stehouwer CDA, Hartgens F, van Loon LJC. Exercise therapy in type 2 diabetes: Is daily exercise required to optimize glycemic control? Diabetes Care (2012) 35:948–54. doi: 10.2337/dc11-2112
41. Karstoft K, Clark MA, Jakobsen I, Müller IA, Pedersen BK, Solomon TPJ, et al. The effects of 2 weeks of interval vs continuous walking training on glycemic control and whole-body oxidative stress in individuals with type 2 diabetes: a controlled, randomised, crossover trial. Diabetologia (2017) 60:508–17. doi: 10.1007/s00125-016-4170-6
42. van Dijk JW, Manders RJF, Tummers K, Bonomi AG, Stehouwer CDA, Hartgens F, et al. Both resistance- and endurance-type exercise reduce the prevalence of hyperglycemia in individuals with impaired glucose tolerance and in insulin-treated and non-insulin-treated type 2 diabetic patients. Diabetologia (2012) 55:1273–82. doi: 10.1007/s00125-011-2380-5
43. Nygaard H, Rønnestad BR, Hammarström D, Holmboe-Ottesen G, Høstmark AT. Effects of exercise in the fasted and postprandial state on interstitial glucose in hyperglycemic individuals. J Sports Sci Med (2017) 16:254–63.
44. Braun B, Brooks GA. Critical importance of controlling energy status to understand the effects of “exercise” on metabolism. Exerc Sport Sci Rev (2008) 36:2–4. doi: 10.1097/jes.0b013e31815e42c2
45. Battelino T, Danne T, Bergenstal RM, Amiel SA, Beck R, Biester T, et al. Clinical targets for continuous glucose monitoring data interpretation: Recommendations from the international consensus on time in range. Diabetes Care (2019) 42:1593–603. doi: 10.2337/dci19-0028
46. Matthews DR, Hosker JP, Rudenski AS, Naylor BA, Treacher DF, Turner RC. Homeostasis model assessment: insulin resistance and β-cell function from fasting plasma glucose and insulin concentrations in man. Diabetologia (1985) 28:412–9. doi: 10.1007/BF00280883
47. Frayn KN. Calculation of substrate oxidation rates in vivo from gaseous exchange. J Appl Physiol (1983) 55:628–34. doi: 10.1152/jappl.1983.55.2.628
48. Jeukendrup AE, Wallis GA. Measurement of substrate oxidation during exercise by means of gas exchange measurements. Int J Sports Med (2005) 26:S28–37. doi: 10.1055/s-2004-830512
49. Danne T, Nimri R, Battelino T, Bergenstal RM, Close KL, DeVries JH, et al. International consensus on use of continuous glucose monitoring. Diabetes Care (2017) 40:1631–40. doi: 10.2337/dc17-1600
50. Solomon TPJ, Eves FF, Laye MJ. Targeting postprandial hyperglycemia with physical activity may reduce cardiovascular disease risk. But what should we do, and when is the right time to move? Front Cardiovasc Med (2018) 5:99. doi: 10.3389/fcvm.2018.00099
51. Nathan DM, Davidson MB, DeFronzo RA, Heine RJ, Henry RR, Pratley R, et al. Impaired fasting glucose and impaired glucose tolerance: Implications for care. Diabetes Care (2007) 30:753–9. doi: 10.2337/dc07-9920
52. Abdul-Ghani MA, Jenkinson CP, Richardson DK, Tripathy D, DeFronzo RA. Insulin secretion and action in subjects with impaired fasting glucose and impaired glucose tolerance: Results from the veterans administration genetic epidemiology study. Diabetes (2006) 55:1430–5. doi: 10.2337/db05-1200
53. van Wijck K, Lenaerts K, Grootjans J, Wijnands KAP, Poeze M, van Loon LJC, et al. Physiology and pathophysiology of splanchnic hypoperfusion and intestinal injury during exercise: Strategies for evaluation and prevention. Am J Physiol Gastrointest Liver Physiol (2012) 303:155–68. doi: 10.1152/ajpgi.00066.2012
54. Malin SK, Haus JM, Solomon TPJ, Blaszczak A, Kashyap SR, Kirwan JP. Insulin sensitivity and metabolic flexibility following exercise training among different obese insulin-resistant phenotypes. Am J Physiol Endocrinol Metab (2013) 305:E1292–8. doi: 10.1152/ajpendo.00441.2013
55. Aas V, Kase ET, Solberg R, Jensen J, Rustan AC. Chronic hyperglycemia promotes lipogenesis and triacylglycerol accumulation in human skeletal muscle cells. Diabetologia (2004) 47:1452–61. doi: 10.1007/s00125-004-1465-9
56. Aas V, Hessvik NP, Wettergreen M, Hvammen AW, Hallén S, Thoresen GH, et al. Chronic hyperglycemia reduces substrate oxidation and impairs metabolic switching of human myotubes. Biochim Biophys Acta Mol Basis Dis (2011) 1812:94–105. doi: 10.1016/j.bbadis.2010.09.014
57. Green CJ, Henriksen TI, Pedersen BK, Solomon TPJ. Glucagon like peptide-1-induced glucose metabolism in differentiated human muscle satellite cells is attenuated by hyperglycemia. PLoS One (2012) 7:e44284. doi: 10.1371/journal.pone.0044284
58. Zhang W, Liu J, Tian L, Liu Q, Fu Y, Garvey WT. TRIB3 mediates glucose-induced insulin resistance via a mechanism that requires the hexosamine biosynthetic pathway. Diabetes (2013) 62:4192–200. doi: 10.2337/db13-0312
59. Vasu S, McClenaghan NH, McCluskey JT, Flatt PR. Cellular responses of novel human pancreatic β-cell line, 1.1B4 to hyperglycemia. Islets (2013) 5:170–7. doi: 10.4161/isl.26184
60. Bensellam M, Van Lommel L, Overbergh L, Schuit FC, Jonas JC. Cluster analysis of rat pancreatic islet gene mRNA levels after culture in low-, intermediate- and high-glucose concentrations. Diabetologia (2009) 52:463–76. doi: 10.1007/s00125-008-1245-z
61. Quagliaro L, Piconi L, Assaloni R, Martinelli L, Motz E, Ceriello A. Intermittent high glucose enhances apoptosis related to oxidative stress in human umbilical vein endothelial cells: The role of protein kinase C and NAD(P)H-oxidase activation. Diabetes (2003) 52:2795–804. doi: 10.2337/diabetes.52.11.2795
62. Quagliaro L, Piconi L, Assaloni R, Da Ros R, Maier A, Zuodar G, et al. Intermittent high glucose enhances ICAM-1, VCAM-1 and E-selectin expression in human umbilical vein endothelial cells in culture: The distinct role of protein kinase C and mitochondrial superoxide production. Atherosclerosis (2005) 183:259–67. doi: 10.1016/j.atherosclerosis.2005.03.015
63. Solomon TPJ, Knudsen SH, Karstoft K, Winding K, Holst JJ, Pedersen BK. Examining the effects of hyperglycemia on pancreatic endocrine function in humans: Evidence for in vivo glucotoxicity. J Clin Endocrinol Metab (2012) 97:4682–91. doi: 10.1210/jc.2012-2097
64. Shannon C, Merovci A, Xiong J, Tripathy D, Lorenzo F, McClain D, et al. Effect of chronic hyperglycemia on glucose metabolism in subjects with normal glucose tolerance. Diabetes (2018) 67:2507–17. doi: 10.2337/db18-0439
65. Tripathy D, Merovci A, Basu R, Abdul-Ghani M, Defronzo RA. Mild physiologic hyperglycemia induces hepatic insulin resistance in healthy normal glucose-tolerant participants. J Clin Endocrinol Metab (2019) 104:2842–50. doi: 10.1210/jc.2018-02304
66. Ceriello A, Esposito K, Piconi L, Ihnat MA, Thorpe JE, Testa R, et al. Oscillating glucose is more deleterious to endothelial function and oxidative stress than mean glucose in normal and type 2 diabetic patients. Diabetes (2008) 57:1349–54. doi: 10.2337/db08-0063
67. Park S, Turner KD, Zheng D, Brault JJ, Zou K, Chaves AB, et al. Electrical pulse stimulation induces differential responses in insulin action in myotubes from severely obese individuals. J Physiol (2019) 597:449–66. doi: 10.1113/JP276990
68. Feng YZ, Nikolić N, Bakke SS, Kase ET, Guderud K, Hjelmesæth J, et al. Myotubes from lean and severely obese subjects with and without type 2 diabetes respond differently to an in vitro model of exercise. Am J Physiol Cell Physiol (2015) 308:C548–56. doi: 10.1152/ajpcell.00314.2014
69. Radak Z, Chung HY, Koltai E, Taylor AW, Goto S. Exercise, oxidative stress and hormesis. Ageing Res Rev (2008) 7:34–42. doi: 10.1016/j.arr.2007.04.004
70. Macdonald AL, Philp A, Harrison M, Bone AJ, Watt PW. Monitoring exercise-induced changes in glycemic control in type 2 diabetes. Med Sci Sports Exerc (2006) 38:201–7. doi: 10.1249/01.mss.0000183852.31164.5a
71. Mingrone G, Panunzi S, De Gaetano A, Ahlin S, Spuntarelli V, Bondia-Pons I, et al. Insulin sensitivity depends on the route of glucose administration. Diabetologia (2020) 63:1382–95. doi: 10.1007/s00125-020-05157-w
72. Acheson KJ, Ravussin E, Wahren J, Jequier E. Thermic effect of glucose in man. Obligatory and facultative thermogenesis. J Clin Invest (1984) 74:1572–80. doi: 10.1172/JCI111573
73. Ravussin E, Acheson KJ, Vernet O, Danforth E, Jéquier E. Evidence that insulin resistance is responsible for the decreased thermic effect of glucose in human obesity. J Clin Invest (1985) 76:1268–73. doi: 10.1172/JCI112083
74. Ravussin E, Bogardus C, Schwartz RS, Robbins DC, Wolfe RR, Horton ES, et al. Thermic effect of infused glucose and insulin in man. Decreased response with increased insulin resistance in obesity and noninsulin-dependent diabetes mellitus. J Clin Invest (1983) 72:893–902. doi: 10.1172/JCI111060
75. Yki-Jarvinen H, Bogardus C, Howard BV. Hyperglycemia stimulates carbohydrate oxidation in humans. Am J Physiol Metab (1987) 253:E376–82. doi: 10.1152/ajpendo.1987.253.4.E376
76. Shulman GI, DeFronzo RA, Rossetti L. Differential effect of hyperglycemia and hyperinsulinemia on pathways of hepatic glycogen repletion. Am J Physiol Metab (1991) 260:E731–5. doi: 10.1152/ajpendo.1991.260.5.E731
77. Mohebbi H, Campbell IT, Keegan MA, Malone JJ, Hulton AT, MacLaren DPM. Hyperinsulinaemia and hyperglycemia promote glucose utilization and storage during low- and high-intensity exercise. Eur J Appl Physiol (2020) 120:127–35. doi: 10.1007/s00421-019-04257-9
78. Hargreaves M, McConell G, Proietto J. Influence of muscle glycogen on glycogenolysis and glucose uptake during exercise in humans. J Appl Physiol (1995) 78:288–92. doi: 10.1152/jappl.1995.78.1.288
79. Hamilton KS, Gibbons FK, Bracy DP, Lacy DB, Cherrington AD, Wasserman DH. Effect of prior exercise on the partitioning of an intestinal glucose load between splanchnic bed and skeletal muscle. J Clin Invest (1996) 98:125–35. doi: 10.1172/JCI118756
Keywords: exercise, type 2 diabetes, postprandial, glycemic control, hyperglycemia, heterogeneity, variability
Citation: Carter S and Solomon TPJ (2020) Exercise-Induced Improvements in Postprandial Glucose Response Are Blunted by Pre-Exercise Hyperglycemia: A Randomized Crossover Trial in Healthy Individuals. Front. Endocrinol. 11:566548. doi: 10.3389/fendo.2020.566548
Received: 23 June 2020; Accepted: 15 September 2020;
Published: 15 October 2020.
Edited by:
Hans Ulrich Häring, Tübingen University Hospital, GermanyReviewed by:
Asimina Mitrakou-Fanariotou, National and Kapodistrian University of Athens, GreeceDominik H. Pesta, German Center for Diabetes Research (DZD), Germany
Copyright © 2020 Carter and Solomon. This is an open-access article distributed under the terms of the Creative Commons Attribution License (CC BY). The use, distribution or reproduction in other forums is permitted, provided the original author(s) and the copyright owner(s) are credited and that the original publication in this journal is cited, in accordance with accepted academic practice. No use, distribution or reproduction is permitted which does not comply with these terms.
*Correspondence: Steven Carter, c2MyOTg4QGJhdGguYWMudWs=
†Present address: Thomas Solomon, Blazon Scientific, London, United KingdomSteven Carter,University of Bath,Bath, United Kingdom