- 1Department of Nuclear Medicine, Tongji Medical College, Tongji Hospital, Huazhong University of Science and Technology, Wuhan, China
- 2Department of Biology, Massachusetts Institute of Technology, Cambridge, MA, United States
- 3Department of Endocrinology, Internal Medicine, Tongji Medical College, Tongji Hospital, Huazhong University of Science and Technology, Wuhan, China
Brown and beige adipose tissues play a large role in non-shivering thermogenesis (NST) in mammals, and subsequently have been studied for decades as potential therapeutic targets to treat obesity and its related metabolic diseases. However, the mechanistic regulation of brown/beige adipose tissue induction and maintenance in humans is very limited due to the ethical reasons. In fact, metabolic signaling has primarily been investigated using rodent models. A better understanding of non-shivering thermogenesis in humans is thus vital and urgent in order to treat obesity by targeting human brown adipose tissue (BAT). In this review, we summarize the anatomical and physiological differences between rodent and human BAT, current useful and mostly non-invasive methods in studying human BAT, as well as recent advancements targeting thermogenic adipocytes as a means to combat metabolic diseases in humans. Furthermore, we also discuss several novel relevant strategies of therapeutic interventions, which has been attempted in rodent experiments, and possible future investigations in humans in this field.
Introduction
In mammals, there are three kinds of adipose tissues participating in whole-body energy homeostasis. They include white adipose tissue (WAT), which stores energy in the form of triglycerides, brown adipose tissue (BAT), which dissipates energy into heat, and beige adipose tissue, which functions similarly to BAT. BAT, first characterized in 1960s, has been described as both an endocrine and a thermogenic organ (1). It consists of morphologically distinct brown adipocytes which contain multilocular lipid droplets and abundant mitochondria. BAT is the main organ which contributes to non-shivering thermogenesis (NST) in mammals (2). Classically, in response to cold, BAT activation is dependent on the sympathetic innervation (involvement of norepinephrine) and the activation of β3-adrenergic receptors (ARs) located mainly on the adipocyte membrane, followed by a lipolysis from stored triglycerides to free fatty acids, which drives mitochondria respiration and is then oxidized during this process (3–5). BAT is also involved in diet-induced thermogenesis (6), which is dependent on local sympathetic innervation and AR signaling as well (7, 8). Furthermore, NST is largely dependent on uncoupling protein 1 (UCP1), a BAT specific protein located on the mitochondrial membrane, which uncouples the respiratory chain of oxidative phosphorylation within mitochondria, leading to an increase in ATP consumption and heat generation (9). NST has been long thought to only exist in hibernating animals and human infants because of the wealth of BAT in their body for generating heat under certain circumstances to keep warm. In fact, BAT is found in almost all mammals including mice, rats, rabbits, sheep, bears, and humans except pigs (10, 11). Studies have been mostly performed using rodent models to investigate mechanisms of NST regulation. Meanwhile, brown-like adipocytes, later termed beige adipocytes, were discovered in subcutaneous WAT in rodents in response to cold stimulus (12, 13). They look morphologically similar to brown adipocytes and contain abundant UCP1-positive mitochondria, which supports their role in NST (12, 14). Besides, their activation is also triggered by a sympathetic innervation, which is similar to brown adipocytes as well (15). As long as functional BAT is detected in 2007 (16) and specifically characterized in adult humans in 2009 using 18F-fluorodeoxyglucose Positron Emission Tomography coupled with Computer Tomography (18F-FDG PET/CT) (17–19), more and more studies are performed to study BAT activation in humans mostly using PET/CT as well as other non-invasive methods due to ethical reasons. 18F-FDG positive adipose tissue in humans is primarily distributed in the cervical, supra-clavicular, supra-adrenal, and para-vertebral regions (16). Significantly, human studies have been performed to identify whether those 18F-FDG positive adipose tissues in humans are classic BAT or recruitable beige adipose tissue. There is evidence that both classical brown and beige adipocytes exist in human infant through a corpse study using magnetic resonance imaging (MRI), in addition to histological and biochemical analyses (20). In 2013, through anatomical and transcriptome profiling, it was shown that deeper cervical fat consists of classical brown adipocytes while supra-clavicular fat is composed of both classical brown and recruitable beige adipocytes in adult humans (21, 22). Moreover, global and unbiased genome-wide expression analysis of clonally derived adult human brown adipocytes from the supra-clavicular region indicates a close relationship between human brown adipocytes and mouse beige adipocytes (23). Nevertheless, targeting brown and beige adipose tissue, such as the administration of β3-AR agonists, A2A receptor agonists and other pharmaceuticals, promotes thermogenic fat-mediated NST and becomes feasible therapeutic approaches to increase energy expenditure and potentially treat obesity. Besides, certain natural molecules have also been identified to be involved in the regulation of thermogenic fat activation in humans. However, knowledges on the mechanistic regulation of brown and beige adipose tissue-mediated NST are mostly known from rodent experiments, and human BAT is more heterogeneous than rodent BAT due to its composition and possible distinct mRNA-expression profiles (23). Thus, a better understanding of the roles of brown and beige adipose tissue in energy metabolism in humans could provide additional resources to clinically treat obesity and its comorbidities.
Anatomical and Physiological Differences Between Human and Rodent Bat
An understanding of differences between rodent and human BAT could be of advantage to realize the transition of scientific research achievements from rodent to human. However, the function of BAT and its contribution to energy metabolism in humans may differ from results found in rodents. This discrepancy could be largely due to the anatomical and physiological differences between two species, shown in Figure 1. In rodents, classic BAT exists past the neonatal period into adulthood, while in humans this is still controversial. In human infants, classic BAT is found in the subcutaneous fat depot of interscapular region, and a layer of connective tissue between WAT and BAT is identified histologically (20). However, the 18F-FDG PET/CT scans reveal that adult humans do not exhibit interscapular BAT. Nevertheless, the age at which interscapular BAT atrophies and disappears in humans is still unclear. Inspiringly, it has been indicated that in certain individuals, deeper cervical adipose tissue in adult humans shares many similarities with classical rodent BAT on molecular and histological level (21). Moreover, it has been shown that tissue in the supra-clavicular region in adult humans is composed of a mixture of brown and beige adipocytes (22). Thus, adult human BAT is special and unique compared to classic BAT existing in rodents. Whether targeting adult human BAT is adequate for heat generation under certain circumstances still need to be further investigated.
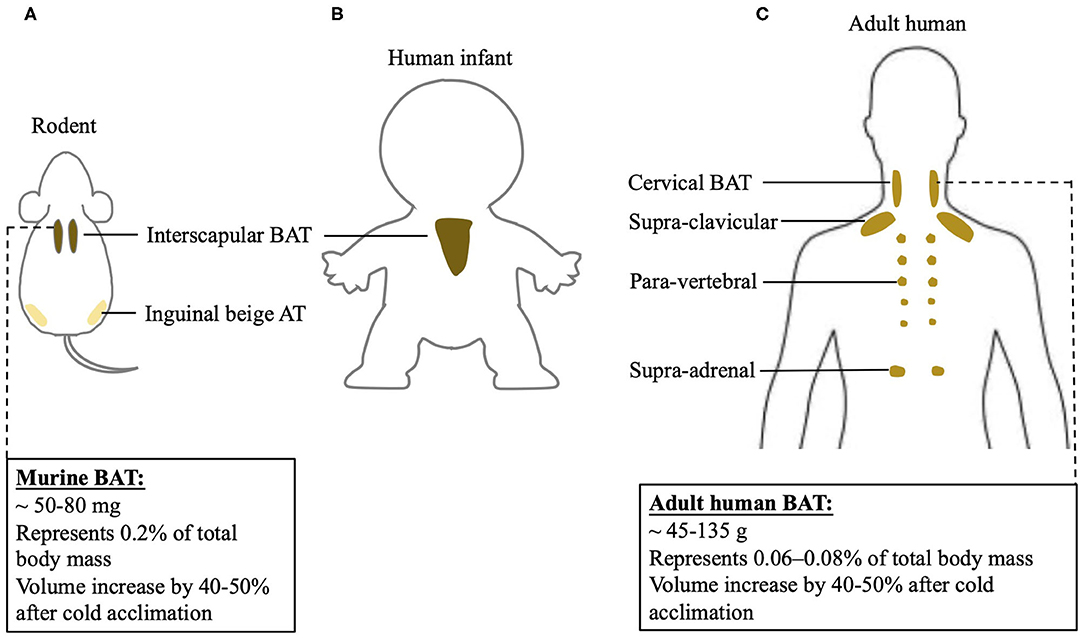
Figure 1. BAT localization in rodent and human and differences of BAT physiology between two species. BAT localization in (A) rodent; (B) human infant, and (C) adult human. Physiological characterization of BAT in mouse and adult human.
Increasing BAT mass and BAT activity could be potential mechanistic targets to induce an upregulation of BAT-mediated NST. BAT mass can be quantified using PET/CT (24). Specifically, 18F-FDG positive adipose tissue with an SUV mean threshold ≥ 1.5 is considered BAT. BAT volume, when multiplied by the density of the tissue, can be used to approximate total BAT mass in an individual (25). Human BAT reported so far is ~45–135 grams, while mice have about 50–80 milligrams of BAT. When calculated as percentage in body weight, human BAT represents 0.06–0.08% of total body mass, while mouse BAT is about 0.2% of total body mass. Cold acclimation in humans increases BAT volume by 40–50% (26–28), an increase similar to what has been observed in mice (29). Using direct PET/CT scan with [15O]O2 and [15O]H2O, it has been shown that a short-time mild cold exposure could cause a BAT-mediated oxygen consumption, which is as 0.1–0.6% of whole-body oxygen consumption in humans (30–33). Following a chronic cold exposure for 4 weeks, the contribution of BAT to whole-body oxygen consumption in humans further increases to 0.5–2.3% (24, 34). However, in mice, their oxygen consumption is increased by 38–60% after a mild cold exposure (35, 36). The drastic differences in thermogenic responses to cold stimulation between humans and rodents may be, in part, due to disparities in proportions of BAT mass relative to whole body mass and diverse analysis methods. Moreover, humans may differ from rodents in the mechanisms involved in BAT-induced energy expenditure, which actually remain largely unknown in humans. Thus, the physiological difference between rodent and human BAT has to be noticed when targeting BAT to combat obesity when using rodent models.
Non-Invasive Methods in Studying Human Bat
18F-FDG PET/CT, as a non-invasive method, is commonly used to study human BAT. Active BAT takes glucose as the source of energy metabolism, thus, 18F-labled glucose analogue FDG works as a tracer for BAT imaging. When merged with CT images, tracer aggregation in the adipose tissue region could display the location and glucose uptake of BAT. Besides, dynamic metabolic imaging can be obtained after 18F-FDG PET/CT scanning, which directly reflects the activity of BAT. However, 18F-FDG PET/CT is radioactive, which is harmful and may limit the use of its application. Other non-invasive methods to study human BAT include MRI, infrared thermography (IRT), and orthogonal assays assessing metabolic changes associated with BAT activation, such as whole-body calorimetry. Furthermore, researchers often take BAT biopsies for molecular analysis. The differences between these methods are shown in Table 1.
MRI can be used to assess the intracellular triglyceride depletion of human BAT by measuring fat content before and after BAT activation (37). Unlike PET/CT, MRI does not require radiation. However, due to its complex modeling and low sensitivity, it is not applied as frequently as PET/CT to quantify human BAT.
Similar to the MRI, IRT does not require radioactivity for its measurements. The anterior supraclavicular temperature measured by IRT has been shown to be positively correlated with energy expenditure and changes in parallel with standard uptake value (SUV) obtained from PET imaging (38, 39). Nevertheless, IRT is mostly useful for measuring the temperature of superficial adipose tissue, which might be inapplicable and imprecise for a temperature measurement of deeper parts. Besides, the anatomical localization of an IRT scan appears to be difficult.
Whole-body calorimetry can be used to determine energy expenditure of humans (38). When paired with blood serum analysis of metabolites such as high-density lipoprotein, triglycerides, fasting glucose, non-esterified fatty acids, etc., this method provides insight into whole-body energy metabolism. It is often paired with other methods of studying BAT function in humans.
Remarkably, certain biomarkers in serum have been characterized in several studies that correlates with BAT mass and BAT activity in humans. A previous study from our lab revealed that serum concentration of miRNA-92a, derived from BAT exosomes, is negatively correlated with human BAT activity (40). Similarly, BAT-derived exosomal miR-122-5p, has also been shown to be negatively correlated with human BAT activity (41). Meanwhile, a recent study reported a positive correlation of lysophosphatidylcholine-acyl C16:0 and Fibroblast growth factor 21 (FGF21) with human BAT activity (42, 43). Undoubtedly, novel diagnostic tools are needed for assessing BAT function in large and repeated cohort studies in humans.
Current Advancements of Combating Obesity With Bat in Humans
Targeting brown and beige adipose tissue has been a viable therapeutic approach to combat obesity. The role of these two types of thermogenic adipose tissue is better established in rodents, but their function and regulation in adult humans remain largely unknown. Although cold exposure is an effective way to stimulate BAT activity in humans, pharmacological stimulations are much more achievable and efficient. Based on the findings in rodent experiments that the β3-AR signaling and adenosine–A2A receptor signaling play prominent roles in the regulation of BAT function (7, 44, 45), the effects of β3-AR agonists and adenosine on BAT activation and energy metabolism have been studied in humans (Figure 2).
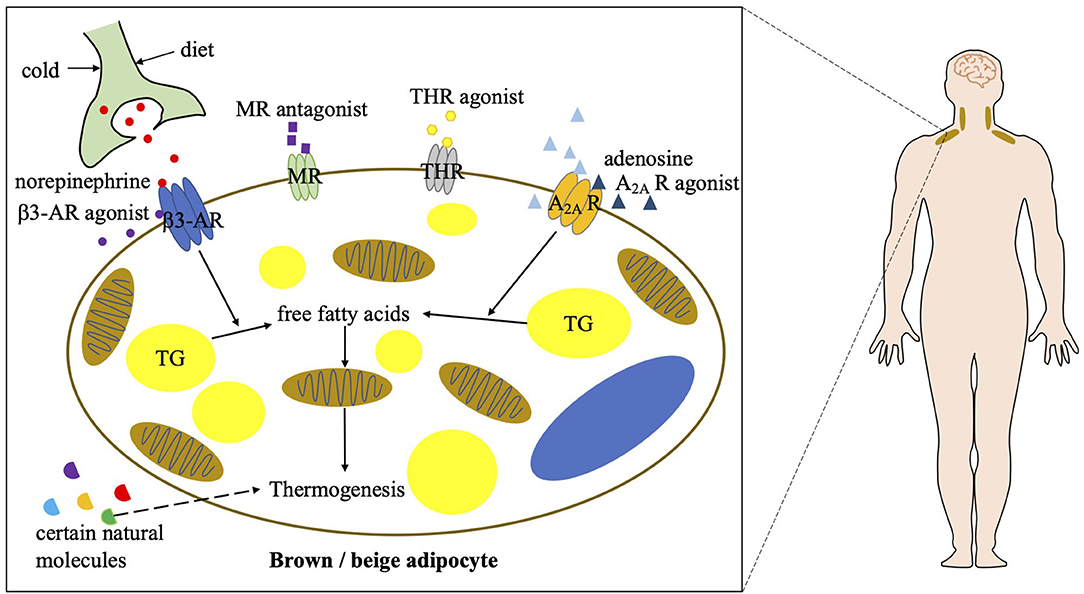
Figure 2. Current strategies of combating obesity via targeting human BAT. A2A R, A2A receptor; β3-AR, beta 3 adrenergic receptor; MR, mineralocorticoid receptor; THR, thyroid hormone receptor; TG, triglycerol.
β3-AR Agonists
In the last few decades, different β3-AR agonists were developed by companies and their contribution to BAT activation has been studied. The effects of β3-AR agonists on thermogenic fat-mediated energy metabolism have long been observed in rodents. However, due to a lower expression of β3-AR in human adipocytes compared with murine adipocytes, most β3-AR agonists have poor bioavailability in patients (46). On the other hand, due to a low selectivity of those β3-AR agonists and localization of β3-AR elsewhere, they can have fatal effects on the cardiovascular system (46–48). None of the previous β3-AR agonists have been approved for clinical use to treat metabolic diseases. In recent years, several β3-AR agonists including mirabegron, vibegron, ritobegron, and solabegron have been repurposed for other diseases (49–51). Some have been approved for clinical use to treat overactive bladders and urinary incontinence. Their effects on BAT activation and metabolism in humans have been the focus of several clinical trials as well. The results show that both acute (2 days) and chronic (28 days) administration of mirabegron dramatically induces BAT activity, measured by PET/CT, and boosts resting energy expenditure in healthy humans (52, 53). Moreover, biomarkers indicative of healthy metabolism such as high-density lipoprotein, ApoA1, non-esterified fatty acids, total bile acids and adiponectin are increased, and insulin sensitivity is improved after mirabegron treatment. Furthermore, in obese and insulin-resistant humans, chronic mirabegron administration promotes glucose tolerance and induces “beiging” in subcutaneous WAT, in parallel with an improvement of β-cell function (54). However, mirabegron induced metabolic upregulation does not result in weight loss. Despite of an accelerated heartbeat and increased systolic blood pressure after mirabegron treatment (53), mirabegron administration may promote BAT activity and thereby benefits obesity and obesity-related metabolic disorders. Further studies are needed to develop novel applications of β3-AR agonists and, in particular, reduce the above by-effects to treat metabolic diseases.
Adenosine and A2A Receptor Agonists
Adenosine is an extracellular molecule involved in whole-body energy metabolism. In response to sympathetic stimulation by noradrenaline, an endogenous adenosine is released locally in BAT (44). Adenosine in binding with A2A receptors has been shown to not only increase BAT activation but also induce “beiging” in rodents, resulting in a reduction in diet-induced obesity and an improvement in glucose tolerance. Furthermore, an A2A receptor agonist, CGS21680, also induces BAT activation and results in an increase in energy expenditure in mice. The effect of exogenous adenosine on human BAT as well as A2A receptor density has been investigated using PET/CT imaging (55). It has been shown that adenosine administration dramatically increases BAT activity in humans. Its induced BAT activation is even greater than that induced by cold exposure. Besides, radioligand detectable A2A receptors decrease after cold exposure due to a release of endogenous adenosine, which binds on the A2A receptors. Collectively, these results indicate that targeting A2A receptors on thermogenic adipocytes is potentially another approach to treat obesity. Specifically, adenosine and A2A receptor agonists could be potential therapeutic drugs to enhance BAT function. However, further investigations are required to assess their safety, considering their potentially deleterious effects on the cardiovascular system.
Other Potential Approaches to Combat Obesity With Human BAT
Other well-known pharmacological approaches to stimulate human BAT activity also include PPARγ agonists, mineralocorticoid receptor antagonists, and thyroid hormone receptor agonists. Among them, certain PPARγ agonists have been shown to potentially induce beige fat development (56, 57), which may be beneficial in the treatment of obesity and its related metabolic disorders. Mineralocorticoid receptor antagonists have been shown to positively correlates to BAT thermogenesis in humans (58), which may also potentially benefit obesity. Thyroid hormones have been long discovered to induce thermogenesis and subsequent high metabolic rate in humans, which is thought to be caused through a mechanism involving the activation of human BAT. Certain thyroid hormone receptor agonists have been identified to promote beige fat development and induce heat generation in rodents even at ambient temperature (59). However, the mechanisms of the above pharmaceuticals in thermogenic fat activation remain unclear, so that their roles in human BAT activation and obesity treatment need to be further investigated. In addition to pharmacological approaches, some natural molecules are also involved in human BAT activation, which includes secretin, cardiac natriuretic peptides, bile acids, myokines, capsaicin, and so on (60–64). They may also contribute to BAT-mediated energy consumption and benefit obese patients. However, their individual mechanisms are still disputed or frankly unclear. Further investigations of these molecules in energy metabolism in humans are required.
Prospects From Rodent Experiments to a Better Metabolic Health in Humans
There is increasing evidence that BAT acts physiologically as a “metabolic sink” in the human body (65). 18F-FDG and 18F-fluoro-thiaheptadecanoic acid (18F-FTHA) PET/CT imaging clearly display a dynamic uptake of glucose and free fatty acids into the BAT after cold stimulation (66). BAT plays an important role in glucose homeostasis and promoting insulin sensitivity in humans (67). Its oxidative capacity is largely associated with whole body energy expenditure. At thermoneutrality, food intake activates glucose uptake in human BAT via diet-induced thermogenesis (6). Furthermore, BAT has been shown to contribute to excessive energy expenditure under certain pathological conditions such as hyperthyroidism and cachexia, which are both characterized by emaciation (68, 69). Of note, human BAT is different to murine BAT due to its composition, localization, and oxidative capacity after certain stimulation. An anatomical and physiological comparison of BAT between human and rodent mentioned in previous paragraph may be inadequate to conclude the difference of human and rodent BAT contribution to whole body energy metabolism. However, these shortcomings should be taken into consideration when using rodent models to study BAT.
Gene profiling of human 18F-FDG positive adipose tissues indicates a cellular heterogeneity of adult human BAT (21–23), which is still being investigated. It is known that thermogenic adipocytes respond to cold and pharmacological stimulation (52, 53, 55), which is similar to the findings in rodents. Hence, by increasing the volume or function of thermogenic fat, one can enhance the metabolic benefits of these unique adipocytes. A maximal oxidative capacity of human BAT could be increased by 150% after a cold acclimation (2), while the β3-AR agonist mirabegron could boost human resting metabolic rate by 13% or resting energy expenditure by 10.7% (52, 53). An increased metabolic activity may benefit metabolic diseases, although the contribution of these approaches to weight loss remains either unclear or disappointing. Such outcome may result from the relative lower proportion of BAT in the whole body. However, WAT accounts for 20–35% of the body weight (70). In the case that beige fat exists in WAT contributing to energy consumption (12), it is promising to induce beige fat development in WAT. Notably, in recent years, studies using rodent models have shown a high plasticity of beige adipocytes regarding to its origin and regulation, the results of which have been summarized in our latest review article (71). Unlike BAT, the origin of murine beige adipocytes reported so far could be white adipocyte via transdifferentiation or distinct progenitors including PDGFRα+, mural, or MyoD+ progenitors via differentiation (72–77). Moreover, the regulatory mechanisms of beige fat development in rodents vary under different circumstances, which also include non-UCP1 dependent and non-β3-AR dependent mechanisms (44, 58, 77–81). Limited knowledge is known about the origin and regulatory mechanisms of adult human BAT. It is believed that the unique adult human BAT could also be heterogeneous, which requires further investigations. The current findings in rodents could provide more evidences and increase possibilities for targeting thermogenic fat to treat obesity and its related metabolic diseases in humans in the future.
Author Contributions
RP and YC wrote the manuscript. RP, XZ, PM, and YC edited the manuscript and approved the submitted version.
Funding
This work was supported by a grant from Tongji Hospital in Huazhong University of Science and Technology (Grant No. 2201103295 to YC).
Conflict of Interest
The authors declare that the research was conducted in the absence of any commercial or financial relationships that could be construed as a potential conflict of interest.
References
1. Trayhurn P, Arch JR. New physiological aspects of brown adipose tissue. Curr Obes Rep. (2014) 3:414–21. doi: 10.1007/s13679-014-0125-8
2. Blondin DP, Daoud A, Taylor T, Tingelstad HC, Bezaire V, Richard D, et al. Four-week cold acclimation in adult humans shifts uncoupling thermogenesis from skeletal muscles to brown adipose tissue. J Physiol. (2017) 595:2099–113. doi: 10.1113/JP273395
3. Steiner G, Loveland M, Schonbaum E. Effect of denervation on brown adipose tissue metabolism. Am J Physiol. (1970) 218:566–70. doi: 10.1152/ajplegacy.1970.218.2.566
4. Arner P. Human fat cell lipolysis: biochemistry, regulation and clinical role. Best Pract Res Clin Endocrinol Metab. (2005) 19:471–82. doi: 10.1016/j.beem.2005.07.004
5. Braun K, Oeckl J, Westermeier J, Li Y, Klingenspor M. Non-adrenergic control of lipolysis and thermogenesis in adipose tissues. J Exp Biol. (2018) 221(Suppl. 1):jeb165381. doi: 10.1242/jeb.165381
6. Vosselman MJ, Brans B, van der Lans AA, Wierts R, van Baak MA, Mottaghy FM, et al. Brown adipose tissue activity after a high-calorie meal in humans. Am J Clin Nutr. (2013) 98:57–64. doi: 10.3945/ajcn.113.059022
7. Bachman ES, Dhillon H, Zhang CY, Cinti S, Bianco AC, Kobilka BK, et al. βAR signaling required for diet-induced thermogenesis and obesity resistance. Science. (2002) 297:843–5. doi: 10.1126/science.1073160
8. Kozak LP. Brown fat and the myth of diet-induced thermogenesis. Cell Metab. (2010) 11:263–7. doi: 10.1016/j.cmet.2010.03.009
9. Cannon B, Nedergaard J. Brown adipose tissue: function and physiological significance. Physiol Rev. (2004) 84:277–359. doi: 10.1152/physrev.00015.2003
10. Smith RE, Horwitz BA. Brown fat and thermogenesis. Physiol Rev. (1969) 49:330–425. doi: 10.1152/physrev.1969.49.2.330
11. Trayhurn P, Temple NJ, Van Aerde J. Evidence from immunoblotting studies on uncoupling protein that brown adipose tissue is not present in the domestic pig. Can J Physiol Pharmacol. (1989) 67:1480–5. doi: 10.1139/y89-239
12. Wu J, Bostrom P, Sparks LM, Ye L, Choi JH, Giang AH, et al. Beige adipocytes are a distinct type of thermogenic fat cell in mouse and human. Cell. (2012) 150:366–76. doi: 10.1016/j.cell.2012.05.016
13. Young P, Arch JR, Ashwell M. Brown adipose tissue in the parametrial fat pad of the mouse. FEBS Lett. (1984) 167:10–4. doi: 10.1016/0014-5793(84)80822-4
14. Harms M, Seale P. Brown and beige fat: development, function and therapeutic potential. Nat Med. (2013) 19:1252–63. doi: 10.1038/nm.3361
15. Seale P, Conroe HM, Estall J, Kajimura S, Frontini A, Ishibashi J, et al. Prdm16 determines the thermogenic program of subcutaneous white adipose tissue in mice. J Clin Invest. (2011) 121:96–105. doi: 10.1172/JCI44271
16. Nedergaard J, Bengtsson T, Cannon B. Unexpected evidence for active brown adipose tissue in adult humans. Am J Physiol Endocrinol Metab. (2007) 293:E444–52. doi: 10.1152/ajpendo.00691.2006
17. Cypess AM, Lehman S, Williams G, Tal I, Rodman D, Goldfine AB, et al. Identification and importance of brown adipose tissue in adult humans. N Engl J Med. (2009) 360:1509–17. doi: 10.1056/NEJMoa0810780
18. van Marken Lichtenbelt WD, Vanhommerig JW, Smulders NM, Drossaerts JM, Kemerink GJ, Bouvy ND, et al. Cold-activated brown adipose tissue in healthy men. N Engl J Med. (2009) 360:1500–8. doi: 10.1056/NEJMoa0808718
19. Virtanen KA, Lidell ME, Orava J, Heglind M, Westergren R, Niemi T, et al. Functional brown adipose tissue in healthy adults. N Engl J Med. (2009) 360:1518–25. doi: 10.1056/NEJMoa0808949
20. Lidell ME, Betz MJ, Dahlqvist Leinhard O, Heglind M, Elander L, Slawik M, et al. Evidence for two types of brown adipose tissue in humans. Nat Med. (2013) 19:631–4. doi: 10.1038/nm.3017
21. Cypess AM, White AP, Vernochet C, Schulz TJ, Xue R, Sass CA, et al. Anatomical localization, gene expression profiling and functional characterization of adult human neck brown fat. Nat Med. (2013) 19:635–9. doi: 10.1038/nm.3112
22. Jespersen NZ, Larsen TJ, Peijs L, Daugaard S, Homoe P, Loft A, et al. A classical brown adipose tissue mRNA signature partly overlaps with brite in the supraclavicular region of adult humans. Cell Metab. (2013) 17:798–805. doi: 10.1016/j.cmet.2013.04.011
23. Shinoda K, Luijten IH, Hasegawa Y, Hong H, Sonne SB, Kim M, et al. Genetic and functional characterization of clonally derived adult human brown adipocytes. Nat Med. (2015) 21:389–94. doi: 10.1038/nm.3819
24. Richard MA, Pallubinsky H, Blondin DP. Functional characterization of human brown adipose tissue metabolism. Biochem J. (2020) 477:1261–86. doi: 10.1042/BCJ20190464
25. Martin AD, Daniel MZ, Drinkwater DT, Clarys JP. Adipose tissue density, estimated adipose lipid fraction and whole body adiposity in male cadavers. Int J Obes Relat Metab Disord. (1994) 18:79–83.
26. Blondin DP, Labbe SM, Tingelstad HC, Noll C, Kunach M, Phoenix S, et al. Increased brown adipose tissue oxidative capacity in cold-acclimated humans. J Clin Endocrinol Metab. (2014) 99:E438–46. doi: 10.1210/jc.2013-3901
27. Lee P, Smith S, Linderman J, Courville AB, Brychta RJ, Dieckmann W, et al. Temperature-acclimated brown adipose tissue modulates insulin sensitivity in humans. Diabetes. (2014) 63:3686–98. doi: 10.2337/db14-0513
28. van der Lans AA, Hoeks J, Brans B, Vijgen GH, Visser MG, Vosselman MJ, et al. Cold acclimation recruits human brown fat and increases nonshivering thermogenesis. J Clin Invest. (2013) 123:3395–403. doi: 10.1172/JCI68993
29. Kalinovich AV, de Jong JM, Cannon B, Nedergaard J. UCP1 in adipose tissues: two steps to full browning. Biochimie. (2017) 134:127–37. doi: 10.1016/j.biochi.2017.01.007
30. Muzik O, Mangner TJ, Granneman JG. Assessment of oxidative metabolism in brown fat using PET imaging. Front Endocrinol. (2012) 3:15. doi: 10.3389/fendo.2012.00015
31. Muzik O, Mangner TJ, Leonard WR, Kumar A, Janisse J, Granneman JG. 15O PET measurement of blood flow and oxygen consumption in cold-activated human brown fat. J Nucl Med. (2013) 54:523–31. doi: 10.2967/jnumed.112.111336
32. Din MU, Raiko J, Saari T, Kudomi N, Tolvanen T, Oikonen V, et al. Human brown adipose tissue [(15)O]O2 PET imaging in the presence and absence of cold stimulus. Eur J Nucl Med Mol Imaging. (2016) 43:1878–86. doi: 10.1007/s00259-016-3364-y
33. Din MU, Saari T, Raiko J, Kudomi N, Maurer SF, Lahesmaa M, et al. Postprandial oxidative metabolism of human brown fat indicates thermogenesis. Cell Metab. (2018) 28:207–16.e3. doi: 10.1016/j.cmet.2018.05.020
34. Blondin DP, Tingelstad HC, Noll C, Frisch F, Phoenix S, Guerin B, et al. Dietary fatty acid metabolism of brown adipose tissue in cold-acclimated men. Nat Commun. (2017) 8:14146. doi: 10.1038/ncomms14146
35. Golozoubova V, Cannon B, Nedergaard J. UCP1 is essential for adaptive adrenergic nonshivering thermogenesis. Am J Physiol Endocrinol Metab. (2006) 291:E350–7. doi: 10.1152/ajpendo.00387.2005
36. Goldgof M, Xiao C, Chanturiya T, Jou W, Gavrilova O, Reitman ML. The chemical uncoupler 2,4-dinitrophenol (DNP) protects against diet-induced obesity and improves energy homeostasis in mice at thermoneutrality. J Biol Chem. (2014) 289:19341–50. doi: 10.1074/jbc.M114.568204
37. Oreskovich SM, Ong FJ, Ahmed BA, Konyer NB, Blondin DP, Gunn E, et al. MRI reveals human brown adipose tissue is rapidly activated in response to cold. J Endocr Soc. (2019) 3:2374–84. doi: 10.1210/js.2019-00309
38. Sun L, Verma S, Michael N, Chan SP, Yan J, Sadananthan SA, et al. Brown adipose tissue: multimodality evaluation by PET, MRI, infrared thermography, and whole-body calorimetry (TACTICAL-II) Obesity. (2019) 27:1434–42. doi: 10.1002/oby.22560
39. Nirengi S, Wakabayashi H, Matsushita M, Domichi M, Suzuki S, Sukino S, et al. An optimal condition for the evaluation of human brown adipose tissue by infrared thermography. PLoS ONE. (2019) 14:e0220574. doi: 10.1371/journal.pone.0220574
40. Chen Y, Buyel JJ, Hanssen MJ, Siegel F, Pan R, Naumann J, et al. Exosomal microRNA miR-92a concentration in serum reflects human brown fat activity. Nat Commun. (2016) 7:11420. doi: 10.1038/ncomms11420
41. Okamatsu-Ogura Y, Matsushita M, Bariuan JV, Nagaya K, Tsubota A, Saito M. Association of circulating exosomal miR-122 levels with BAT activity in healthy humans. Sci Rep. (2019) 9:13243. doi: 10.1038/s41598-019-49754-1
42. Boon MR, Bakker LEH, Prehn C, Adamski J, Vosselman MJ, Jazet IM, et al. LysoPC-acyl C16:is associated with brown adipose tissue activity in men. Metabolomics. (2017) 13:48. doi: 10.1007/s11306-017-1185-z
43. Soundarrajan M, Deng J, Kwasny M, Rubert NC, Nelson PC, El-Seoud DA, et al. Activated brown adipose tissue and its relationship to adiposity and metabolic markers: an exploratory study. Adipocyte. (2020) 9:87–95. doi: 10.1080/21623945.2020.1724740
44. Gnad T, Scheibler S, von Kugelgen I, Scheele C, Kilic A, Glode A, et al. Adenosine activates brown adipose tissue and recruits beige adipocytes via A2A receptors. Nature. (2014) 516:395–9. doi: 10.1038/nature13816
45. Jung RT, Shetty PS, James WP, Barrand MA, Callingham BA. Reduced thermogenesis in obesity. Nature. (1979) 279:322–3. doi: 10.1038/279322a0
46. Arch JR. Challenges in β(3)-adrenoceptor agonist drug development. Ther Adv Endocrinol Metab. (2011) 2:59–64. doi: 10.1177/2042018811398517
47. Bhadada SV, Patel BM, Mehta AA, Goyal RK. β(3) receptors: role in cardiometabolic disorders. Ther Adv Endocrinol Metab. (2011) 2:65–79. doi: 10.1177/2042018810390259
48. Zhang ZS, Cheng HJ, Onishi K, Ohte N, Wannenburg T, Cheng CP. Enhanced inhibition of L-type Ca2+ current by beta3-adrenergic stimulation in failing rat heart. J Pharmacol Exp Ther. (2005) 315:1203–11. doi: 10.1124/jpet.105.089672
49. Vonesh E, Gooch KL, Khangulov V, Schermer CR, Johnston KM, Szabo SM, et al. Cardiovascular risk profile in individuals initiating treatment for overactive bladder - Challenges and learnings for comparative analysis using linked claims and electronic medical record databases. PLoS ONE. (2018) 13:e0205640. doi: 10.1371/journal.pone.0205640
50. Mitcheson HD, Samanta S, Muldowney K, Pinto CA, Rocha BA, Green S, et al. Vibegron (RVT-901/MK-4618/KRP-114V) administered once daily as monotherapy or concomitantly with tolterodine in patients with an overactive bladder: a multicenter, phase IIb, randomized, double-blind, controlled trial. Eur Urol. (2019) 75:274–82. doi: 10.1016/j.eururo.2018.10.006
51. Keam SJ. Vibegron: first global approval. Drugs. (2018) 78:1835–9. doi: 10.1007/s40265-018-1006-3
52. O'Mara AE, Johnson JW, Linderman JD, Brychta RJ, McGehee S, Fletcher LA, et al. Chronic mirabegron treatment increases human brown fat, HDL cholesterol, and insulin sensitivity. J Clin Invest. (2020) 130:2209–19. doi: 10.1172/JCI131126
53. Cypess AM, Weiner LS, Roberts-Toler C, Franquet Elia E, Kessler SH, Kahn PA, et al. Activation of human brown adipose tissue by a β3-adrenergic receptor agonist. Cell Metab. (2015) 21:33–8. doi: 10.1016/j.cmet.2014.12.009
54. Finlin BS, Memetimin H, Zhu B, Confides AL, Vekaria HJ, El Khouli RH, et al. The β3-adrenergic receptor agonist mirabegron improves glucose homeostasis in obese humans. J Clin Invest. (2020) 130:2319–31. doi: 10.1172/JCI134892
55. Lahesmaa M, Oikonen V, Helin S, Luoto P, Din MU, Pfeifer A, et al. Regulation of human brown adipose tissue by adenosine and A2A receptors - studies with [(15)O]H2O and [(11)C]TMSX PET/CT. Eur J Nucl Med Mol Imaging. (2019) 46:743–50. doi: 10.1007/s00259-018-4120-2
56. Ohno H, Shinoda K, Spiegelman BM, Kajimura S. PPARγ agonists induce a white-to-brown fat conversion through stabilization of PRDM16 protein. Cell Metab. (2012) 15:395–404. doi: 10.1016/j.cmet.2012.01.019
57. Qiang L, Wang L, Kon N, Zhao W, Lee S, Zhang Y, et al. Brown remodeling of white adipose tissue by SirT1-dependent deacetylation of Pparγ. Cell. (2012) 150:620–32. doi: 10.1016/j.cell.2012.06.027
58. Thuzar M, Law WP, Dimeski G, Stowasser M, Ho KKY. Mineralocorticoid antagonism enhances brown adipose tissue function in humans: a randomized placebo-controlled cross-over study. Diabetes Obes Metab. (2019) 21:509–16. doi: 10.1111/dom.13539
59. Lin JZ, Martagon AJ, Cimini SL, Gonzalez DD, Tinkey DW, Biter A, et al. Pharmacological activation of thyroid hormone receptors elicits a functional conversion of white to brown fat. Cell Rep. (2015) 13:1528–37. doi: 10.1016/j.celrep.2015.10.022
60. Li Y, Schnabl K, Gabler SM, Willershauser M, Reber J, Karlas A, et al. Secretin-activated brown fat mediates prandial thermogenesis to induce satiation. Cell. (2018) 175:1561–74.e12. doi: 10.1016/j.cell.2018.10.016
61. Bordicchia M, Liu D, Amri EZ, Ailhaud G, Dessi-Fulgheri P, Zhang C, et al. Cardiac natriuretic peptides act via p38 MAPK to induce the brown fat thermogenic program in mouse and human adipocytes. J Clin Invest. (2012) 122:1022–36. doi: 10.1172/JCI59701
62. Broeders EP, Nascimento EB, Havekes B, Brans B, Roumans KH, Tailleux A, et al. The bile acid chenodeoxycholic acid increases human brown adipose tissue activity. Cell Metab. (2015) 22:418–26. doi: 10.1016/j.cmet.2015.07.002
63. Bostrom P, Wu J, Jedrychowski MP, Korde A, Ye L, Lo JC, et al. A PGC1-α-dependent myokine that drives brown-fat-like development of white fat and thermogenesis. Nature. (2012) 481:463–8. doi: 10.1038/nature10777
64. Panchal SK, Bliss E, Brown L. Capsaicin in metabolic syndrome. Nutrients. (2018) 10:630. doi: 10.3390/nu10050630
65. Chouchani ET, Kajimura S. Metabolic adaptation and maladaptation in adipose tissue. Nat Metab. (2019) 1:189–200. doi: 10.1038/s42255-018-0021-8
66. Ouellet V, Labbe SM, Blondin DP, Phoenix S, Guerin B, Haman F, et al. Brown adipose tissue oxidative metabolism contributes to energy expenditure during acute cold exposure in humans. J Clin Invest. (2012) 122:545–52. doi: 10.1172/JCI60433
67. Matsushita M, Yoneshiro T, Aita S, Kameya T, Sugie H, Saito M. Impact of brown adipose tissue on body fatness and glucose metabolism in healthy humans. Int J Obes. (2014) 38:812–7. doi: 10.1038/ijo.2013.206
68. Lahesmaa M, Orava J, Schalin-Jantti C, Soinio M, Hannukainen JC, Noponen T, et al. Hyperthyroidism increases brown fat metabolism in humans. J Clin Endocrinol Metab. (2014) 99:E28–35. doi: 10.1210/jc.2013-2312
69. Kir S, Spiegelman BM. Cachexia and brown fat: a burning issue in cancer. Trends Cancer. (2016) 2:461–3. doi: 10.1016/j.trecan.2016.07.005
70. Gallagher D, Visser M, Sepulveda D, Pierson RN, Harris T, Heymsfield SB. How useful is body mass index for comparison of body fatness across age, sex, and ethnic groups? Am J Epidemiol. (1996) 143:228–39. doi: 10.1093/oxfordjournals.aje.a008733
71. Pan R, Zhu X, Maretich P, Chen Y. Combating obesity with thermogenic fat: current challenges and advancements. Front Endocrinol. (2020) 11:185. doi: 10.3389/fendo.2020.00185
72. Barbatelli G, Murano I, Madsen L, Hao Q, Jimenez M, Kristiansen K, et al. The emergence of cold-induced brown adipocytes in mouse white fat depots is determined predominantly by white to brown adipocyte transdifferentiation. Am J Physiol Endocrinol Metab. (2010) 298:E1244–53. doi: 10.1152/ajpendo.00600.2009
73. Frontini A, Vitali A, Perugini J, Murano I, Romiti C, Ricquier D, et al. White-to-brown transdifferentiation of omental adipocytes in patients affected by pheochromocytoma. Biochim Biophys Acta. (2013) 1831:950–9. doi: 10.1016/j.bbalip.2013.02.005
74. Lee YH, Petkova AP, Granneman JG. Identification of an adipogenic niche for adipose tissue remodeling and restoration. Cell Metab. (2013) 18:355–67. doi: 10.1016/j.cmet.2013.08.003
75. Long JZ, Svensson KJ, Tsai L, Zeng X, Roh HC, Kong X, et al. A smooth muscle-like origin for beige adipocytes. Cell Metab. (2014) 19:810–20. doi: 10.1016/j.cmet.2014.03.025
76. Vishvanath L, MacPherson KA, Hepler C, Wang QA, Shao M, Spurgin SB, et al. Pdgfrβ + mural preadipocytes contribute to adipocyte hyperplasia induced by high-fat-diet feeding and prolonged cold exposure in adult mice. Cell Metab. (2016) 23:350–9. doi: 10.1016/j.cmet.2015.10.018
77. Chen Y, Ikeda K, Yoneshiro T, Scaramozza A, Tajima K, Wang Q, et al. Thermal stress induces glycolytic beige fat formation via a myogenic state. Nature. (2019) 565:180–5. doi: 10.1038/s41586-018-0801-z
78. Armani A, Cinti F, Marzolla V, Morgan J, Cranston GA, Antelmi A, et al. Mineralocorticoid receptor antagonism induces browning of white adipose tissue through impairment of autophagy and prevents adipocyte dysfunction in high-fat-diet-fed mice. FASEB J. (2014) 28:3745–57. doi: 10.1096/fj.13-245415
79. Zeng X, Ye M, Resch JM, Jedrychowski MP, Hu B, Lowell BB, et al. Innervation of thermogenic adipose tissue via a calsyntenin 3β-S100b axis. Nature. (2019) 569:229–35. doi: 10.1038/s41586-019-1156-9
80. Kazak L, Chouchani ET, Jedrychowski MP, Erickson BK, Shinoda K, Cohen P, et al. A creatine-driven substrate cycle enhances energy expenditure and thermogenesis in beige fat. Cell. (2015) 163:643–55. doi: 10.1016/j.cell.2015.09.035
Keywords: obesity, brown adipose tissue, beige adipose tissue, non-shivering thermogenesis, human, rodent
Citation: Pan R, Zhu X, Maretich P and Chen Y (2020) Metabolic Improvement via Enhancing Thermogenic Fat-Mediated Non-shivering Thermogenesis: From Rodents to Humans. Front. Endocrinol. 11:633. doi: 10.3389/fendo.2020.00633
Received: 02 June 2020; Accepted: 05 August 2020;
Published: 10 September 2020.
Edited by:
Kirsi Virtanen, University of Eastern Finland, FinlandReviewed by:
Tobias Fromme, Technical University of Munich, GermanyTakashi Yazawa, Asahikawa Medical University, Japan
Copyright © 2020 Pan, Zhu, Maretich and Chen. This is an open-access article distributed under the terms of the Creative Commons Attribution License (CC BY). The use, distribution or reproduction in other forums is permitted, provided the original author(s) and the copyright owner(s) are credited and that the original publication in this journal is cited, in accordance with accepted academic practice. No use, distribution or reproduction is permitted which does not comply with these terms.
*Correspondence: Yong Chen, dGoueS5jaGVuQHZpcC4xNjMuY29t