- 1Laboratory of Immunology and Inflammation, Department of Cell Biology, University of Brasilia, Brasilia, Brazil
- 2Département de Microbiologie-Infectiologie et d'Immunologie, Université Laval, Quebec City, QC, Canada
- 3Centre de Recherche en Infectiologie du CHU de Québec - Université Laval, Quebec City, QC, Canada
COVID-19, caused by SARS-CoV-2, is characterized by pneumonia, lymphopenia, exhausted lymphocytes and a cytokine storm. Several reports from around the world have identified obesity and severe obesity as one of the strongest risk factors for COVID-19 hospitalization and mechanical ventilation. Moreover, countries with greater obesity prevalence have a higher morbidity and mortality risk of developing serious outcomes from COVID-19. The understanding of how this increased susceptibility of the people with obesity to develop severe forms of the SARS-CoV-2 infection occurs is crucial for implementing appropriate public health and therapeutic strategies to avoid COVID-19 severe symptoms and complications in people living with obesity. We hypothesize here that increased ACE2 expression in adipose tissue displayed by people with obesity may increase SARS-CoV-2 infection and accessibility to this tissue. Individuals with obesity have increased white adipose tissue, which may act as a reservoir for a more extensive viral spread with increased shedding, immune activation and pro-inflammatory cytokine amplification. Here we discuss how obesity is related to a pro-inflammatory and metabolic dysregulation, increased SARS-CoV-2 host cell entry in adipose tissue and induction of hypercoagulopathy, leading people with obesity to develop severe forms of COVID-19 and also death. Taken together, it may be crucial to better explore the role of visceral adipose tissue in the inflammatory response to SARS-CoV-2 infection and investigate the potential therapeutic effect of using specific target anti-inflammatories (canakinumab or anakinra for IL-1β inhibition; anti-IL-6 antibodies for IL-6 inhibition), anticoagulant or anti-diabetic drugs in COVID-19 treatment of people with obesity. Defining the immunopathological changes in COVID-19 patients with obesity can provide prominent targets for drug discovery and clinical management improvement.
Introduction
On December 2019, a series of pneumonia cases without a recognized etiology was reported in Wuhan, a central China city (1). Rapidly spreading throughout the globe, Coronavirus disease (COVID-19) was recently discovered to be caused by Severe Acute Respiratory Syndrome Coronavirus 2 (SARS-CoV-2). The Word Health Organization (WHO) declared SARS-CoV-2 an international public health emergency on January 2020 and pandemic on March 2020. On June 25, 2020, ~9,527,124 COVID-19 cases were confirmed in the world and 484,972 deaths were considered to be caused by this disease. As a means of decelerating disease progression, health authorities advise their citizens to wear masks, wash hands (2) and increase in public and physical distancing (3).
COVID-19 symptoms may or may not include fever, fatigue, dry cough, dyspnoea, anosmia, dysgeusia, and diarrhea (4–6). Respiratory symptoms are believed to be caused by the occurrence of diffuse alveolar damage, tissue fibrosis, and chronic inflammatory infiltrates (7). Some of the COVID-19 patients can often also present with prominent changes in coagulation function (8). Common comorbidities observed in COVID-19 patients are hypertension, cardiovascular disease, type 2 diabetes (9), chronic obstructive pulmonary disease (10), and obesity (11).
Obesity is a major public health issue globally affecting a half a million people (12). As an inducer of cardiometabolic dysfunction, obesity is associated with increased risk for many diseases, such as Type 2 Diabetes (T2D) (13), dyslipidemia (14), hypertension (12), coronary disease (15), and coagulopathy (16). Chronic inflammation, defined as a low grade but persistent process, disrupting homeostasis and driving organ dysfunction (17). During obesity, chronic inflammation is not only associated with metabolic disturbances and decrease in heart health, but also impacts immune system function (18, 19). In this review we present evidence that indicates that people with obesity are more susceptible to develop severe forms of COVID-19 and higher mortality due to intrinsic alterations in blood coagulation parameters, inflammation, and immune response.
Incidence of COVID-19 in People With Obesity
Obesity represents a risk factor for many chronic diseases, including hypertension, dyslipidemia, diabetes mellitus type 2 (T2DM), cardiovascular disease (20), and several types of cancer (21). As a consequence of excessive or abnormal fat tissue accumulation, overweight and obesity can alter innate and adaptive immune responses, making the immune system more prone to infections and less responsive to vaccinations, antivirals, and antimicrobial drugs (22). There is growing evidence that implicates obesity as one of the main risk factors for triggering severe forms of COVID-19 and poor outcomes (23).
Several studies have reported that obesity may affect the severity of COVID-19, with a direct correlation between increasing BMI and the proportion of patients with severe COVID-19 (24). It has been reported that comorbidities related to obesity are also correlated with increased COVID-19 mortality and morbidity, such as cardiovascular disease (22.7%), hypertension (39.7%), diabetes (19.7%), respiratory disease (7.9%), and cancers (1.5%) (25).
Several reports have shown a significant incidence of people with obesity presenting higher COVID-19 mortality and morbidity in different countries. According to WHO data, the United States of America ranks first in the world in terms of prevalence of obesity (36.2%), overweight (31.7%), as well as in the number of total deaths from COVID-19. Some American studies have indicated obesity as as important comorbidity deeply related to the development of severe forms of COVID-19 (26, 27). In a study developed in a large academic hospital in New York City investigating 3,615 individuals who tested positive for COVID-19, 775 (21%) had BMI values among 30–34 kg/m2 and 595 patients (16% of the cohort) displayed BMI values higher than 35 kg/m2 (28). Diabetes and obesity also increased the risk of COVID-19 infection in Mexico (29). In a French hospital that evaluated 124 patients admitted to intensive care by COVID-19, it was found that 28.2% of the cases had a BMI > 35 kg/m2 and required invasive mechanical ventilation (30). In Spain, from 48 critically ill COVID-19 patients admitted to ICU, 48% presented obesity and 44% arterial hypertension as most prevalent comorbidities (31). In Italy, the severity of COVID-19 and the tension in the health system related to the disease have been remarkable, with an estimated fatality rate of 7.2% (32). Recent Italian studies have highlighted the role of comorbidities in their COVID-19 cases, underlying obesity in the severity of this disease (33). Despite the low prevalence of obesity in China, the severely ill COVID-19 patients were older and had comorbidities, such as obesity and diabetes mellitus more often than non-severely ill individuals (34). In Republic of Korea, clinical data of COVID-19 early cases were collected and demonstrated that of the 28 hospitalized patients, 17.9% had one or more coexisting medical conditions being obesity the most common comorbidity (35).
Therefore, considering that obesity is one of the strongest risk factors for COVID-19 severity, it is important to better understand the correlation between obesity and COVID-19 and the mechanisms that could be involved in this process. In this way, it is crucial to analyze deeper this issue, focusing on the association among SARS-CoV-2 host cell entry, adipose tissue biology, and all the inflammatory, vascular and metabolic dysfunctions that may define COVID-19 progression.
SARS-CoV-2 Host Cell Entry
Genomic analyses demonstrated that SARS-CoV-2 is 96% identical at the whole-genome level to a bat coronavirus SARS-CoV (36). It has been demonstrated that host cell entry of SARS-CoV-2 depends on the same receptor used by SARS-CoV to entry host cell, the Angiotensin-converting enzyme 2 (ACE2) (36, 37). ACE2 receptor was first described in 2000 (38, 39) and it was associated with multiple pathophysiological processes, including the pathogenesis of cardiovascular and renal diseases such as hypertension, myocardial infarction and heart failure (40), acute lung injury (ALI) (41), and acute respiratory distress syndrome (ARDS) (42, 43).
ACE2 gene contains 18 exons and 20 introns, maps to Xp22 chromosome and spans 40 kb of the genomic DNA (44). ACE2 protein is a type I transmembrane glycoprotein of 805 amino acids (~120 kDa), containing a single extracellular catalytic domain whose sequence is 41.8% identical with the domain of angiotensin-converting enzyme (ACE) (43). ACE2 is part of the renin-angiotensin-aldosterone system (RAAS), which is a peptidergic system that acts in the homeostatic regulation of the renal and cardiovascular systems, regulating extracellular fluid volume (40). Renin (an aspartyl proteinase secreted by kidney into the circulation) cleaves its starting substrate angiotensinogen to angiotensin I, which is hydrolyzed by ACE to angiotensin II. ACE2 cleaves angiotensin II to Angiotensin 1-7. Angiotensin II promotes inflammation, oxidative stress, vasoconstriction, salt and water reabsorption (45). Consequently, increased ACE2 activity can shift the balance to the Angiotensin 1-7 axis, leading to disease and inflammation protection. Moreover, ACE2 is a zinc metalloprotease multifunctional enzyme that can act on several vasoactive peptides (46), regulating important cardiovascular and renal functions. Therefore, ACE2 has an ambiguous role acting as both an important physiological receptor and a SARS-CoV-2 backdoor (47).
SARS-CoV-2 bind to its host cell receptor is a critical initial step for this virus entry into target cells. SARS-CoV-2 use the homotrimeric spike glycoprotein S on the viral envelope to bind their cellular receptors, which facilitates viral attachment to the surface of target cells, inducing endocytosis of virion particle, catalyzing the fusion between viral and host cell membranes, and allowing the entry of the virus genome into the host cell cytoplasm. Each monomer of trimeric S protein is about 180 kDa, and contains two subunits, S1 and S2. S1 mediates viral attachment to host cell and S2 intermediates membrane fusion.
SARS-CoV-2 entry in host cell requires S protein priming by cellular proteases, such as the endosomal cysteine proteases cathepsin B and L (CatB/L) and the cellular and the serine protease TMPRSS2 (37). SARS-CoV-2 entry into susceptible cells is a complex process that requires the combined action of receptor-binding and proteolytic processing of the S protein to promote an efficient virus-cell fusion (48), followed by endosomal acidification (Figure 1). Contrasting SARS-CoV, cells infected with SARS-CoV-2 form typical syncytium, suggesting that SARS-CoV-2 may mainly use the plasma membrane fusion pathway to enter and replicate inside host cells (49). This plasma membrane fusion pathway is more efficient for most viruses since it may delay host cell antiviral immunity activation compared to the viral and endosomal membrane fusion pathway (50, 51).
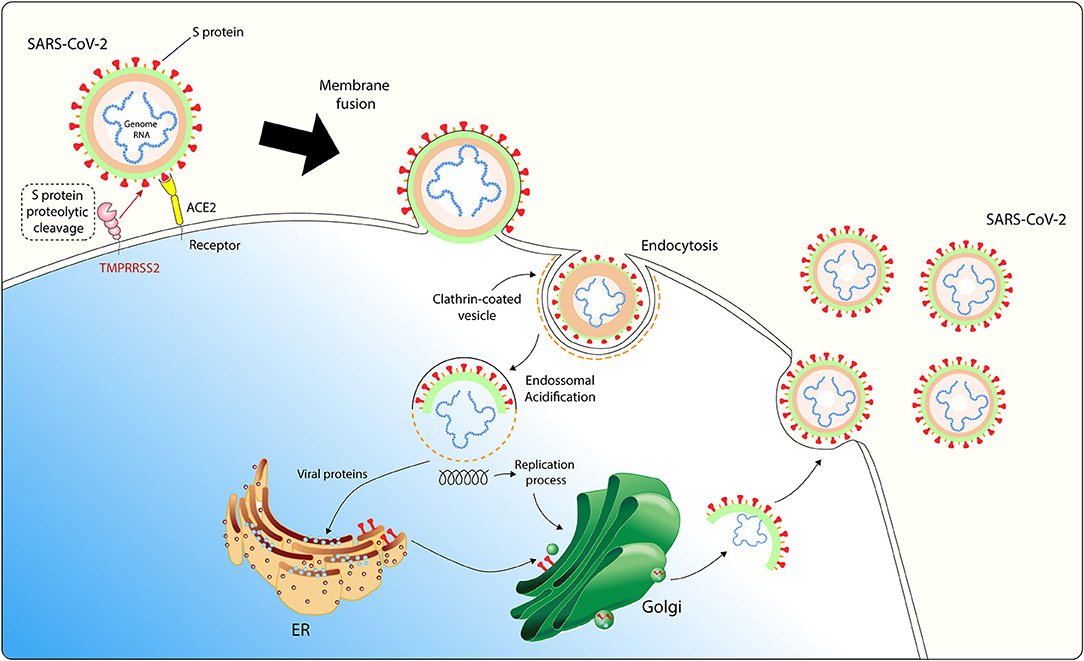
Figure 1. SARS-CoV-2 host cell entry cell depends on the angiotensin-converting enzyme 2 (ACE2) and TMPRSS2. The virus uses the homotrimeric peak glycoprotein S present on viral envelope surface to physically interact with its cell receptor, which facilitates binding to the surface of target cells, enables endocytosis of the virion particle and entry of the viral genome into the host cell cytoplasm. This cell entry process requires priming of protein S by cellular proteases, such as the serine protease TMPRSS2. After endosomal acidification, viral proteins are synthesized in host ER for viral replication and virion particles shedding occurs through Golgi apparatus. ACE2 is expressed in adipocytes and adipose tissue may act as a reservoir for SARS-CoV-2.
Considering the recent findings showing that SARS-CoV-2 mainly uses TMPRSS2 for plasma membrane fusion, clinically proven inhibitors of the cellular serine protease TMPRSS2 might constitute an option for blocking SARS-CoV-2 host cell membrane entry. These results have important implications for our understanding of SARS-CoV-2 transmissibility and pathogenesis and reveal a target for therapeutic intervention.
Several studies have demonstrated how SARS-CoV-2 uses the human ACE2 as the main receptor to viral entry into host cell (36, 48, 52). Overexpression of human ACE2 led to more severe disease in a mouse model of SARS-CoV infection, indicating that viral entry into host cells is a key step for the establishment and progression of this disease (53). Moreover, Zhou and colleagues demonstrated that overexpressing human ACE2 in HeLa cells allowed increased SARS-CoV-2 infection and replication (36). ACE2-expressing cells may act as target cells and are susceptible to SARS-CoV-2 infection (54) and S protein-targeted neutralizing antibody may be prominent antiviral tools against SARS-CoV-2 infection. Several cellular types have been identified with high ACE2 expression including myocardial cells, type II alveolar cells, proximal tubule cells of the kidney, ileum and esophagus epithelial cells, and bladder urothelial cells (54), epithelial cells of oral mucosa (55), nasal epithelial cells (56), and interestingly, adipocytes (57).
SARS-CoV-2 and Adipose Tissue
In patients with obesity, in which white adipose tissue (WAT) is exacerbated and brown adipose tissue (BAT) is decreased (58), RAAS is chronically activated and predisposes the individual to a plethora of dysfunctions, including heart and kidney pathologies. These alterations are associated not only with high blood pressure (59) but also related to insulin signaling in peripheral tissues (60), inflammatory status in pancreas and death profile of β cells (61). Increased oxidative stress is believed to be the root of the cytotoxic effects induced by Ang II and aldosterone during RAAS aberrant activation (62). The resulting insulin resistance acts as a driving force for the progression of cardiometabolic syndrome, commonly associated with obesity (63).
The counterbalance for this blood pressure-increasing action of RAAS is the alternative pathway, which consists in the agonism of the G-protein-coupled Mas receptor by Ang-(1-7). This compound is generated by the enzymatic activity of ACE2 in both AngI and AngII. Ang-(1-7) induces vasorelaxation and cardioprotection (64). ACE2/Mas axis induction associates with BAT activation and WAT browning, processes that are related to anti-obesity effects (65). Due to many alterations in the physiology during obesity, including RAAS dysfunction, BAT tend to present decreased size and activation, increasing the chance of comorbidities (58).
RAAS components, including ACE2, are expressed in adipocytes and are crucial for their glucose and lipid metabolism homeostasis (63). In vivo experiments in mice showed that high-fat diet (HFD)-induced obesity is associated with increased adipose tissue ACE2 expression (40). Thus, we hypothesize here that increased ACE2 expression in adipose tissue displayed by people with obesity may increase SARS-CoV-2 infection and accessibility to this tissue. Moreover, obesity causes hyperglycemia via insulin resistance whereas growing evidence demonstrates that SARS-CoV-2 may cause hyperglycemia as well by infecting and killing B-cells (66). In addition, some drugs often used for the treatment of patients with obesity complications (such as antihypertensives, statins, thiazolidinediones) can up-regulate ACE2, thus could potentially increase the viral up-take (67–70).
Obesity is characterized by dysfunction of immune system (19). During obesity, systemic inflammatory status is influenced by intense pro-inflammatory cytokines secretion (71), increasing the chance of cytokine storm occurrence (72). In addition, obesity associates with Type I Interferon (IFN) decreased secretion, key players in antiviral immune response (73). Human studies showed that H1N1-infected patients with obesity stayed longer under ICU care (74), and investigations with Diet-induced obese (DIO) murine model informed that over nutrition impaired antiviral response against Influenza (75). Thus, individuals with obesity present immune system dysfunction that increase respiratory viral infection susceptibility (19).
It is currently known that adipocytes (76), which are the main cellular components of adipose tissue, and lung cells are targets for SARS-CoV-2 infection (77). Influenza A also presents shared tropism for lungs (78) and WAT (76). In an elegant study, Maier and colleagues showed that symptomatic adults with obesity shed influenza A virus more than 40% longer than non-obese adults. They suggested that WAT dysregulation, common in individuals with obesity, is related to prolonged viral shedding duration (76).
The alarming COVID-19 morbidity and mortality rates of individuals affected with heart pathologies may be related to epicardial adipose tissue (EAT). Classified as a visceral AT, EAT may act as a SARS-CoV-2 reservoir, prolonging viral shedding to cardiac tissue. In addition, EAT obtained from subjects with obesity tend to present higher levels of IL-6 and TNF-α (79), cytokines abundantly secreted in COVID-19 patients. Furthermore, the ACE2/Mas axis dysfunction, observed in individuals with obesity and in subjects affected by COVID-19, associates with EAT inflammation, probably due to Ang(1-7) level decrease, once this protein is associated with diminished proinflammatory macrophage polarization in EAT (80). Once metabolic syndrome, common in individuals affected by obesity, is associated with increased amounts of EAT (81), alterations in EAT amount and inflammatory status may be suggested to influence COVID-19 cardiac morbidity in individuals living with obesity. EAT measurement may play a crucial role in the management of COVID-19 progression in cardiac patients (82).
In a study investigating the influence of obesity in the prognosis of asthma patients, Elliot and others showed that individuals affected by obesity display WAT deposits in large airway walls. They found that BMI value impacts proportionally WAT deposits size, which favors both airway wall thickness increase and neutrophil infiltration within pulmonary tissue (83). Increase in lung wall thickness associates with difficulties in gas exchange (84), and immune cells infiltration is related to tissue damage and fibrosis (85). It is important to have in mind that the increased expression of ACE2 in WAT during obesity makes these intra-pulmonary deposits a susceptible point for SARS-CoV-2 infection within the lung tissue. In addition, the prolonged viral shedding that may occur in WAT would facilitate for the occurrence of pulmonary damage and consequent respiratory failure in cases of obesity (76).
Also found in lungs, adipose-like cells called lipofibroblasts (LiFs) affect pulmonary function, since the transdifferentiation of these cells to myofibroblasts leads to pulmonary fibrosis (PF) (86). LiFs present lipid droplets (LDs) within their cytoplasm containing high levels of perilipin-2. Located in the alveolar interstitium, these cells reside in the proximity of ACE2-expressing type 2 alveolar epithelial cells (AEC2), to whom they provide surfactant molecules. AEC2 are considered to be the biggest pool of ACE2-expressing cells in the lungs and LiFs proximity may indicate higher chance of PF in the lungs of infected individuals with obesity (87). In addition, the possibility of LiFs to also express ACE2 should be assessed, once PF is a common feature among deceased COVID-19 patients.
Although WAT dysfunction is associated with high rates of COVID-19 morbidity and mortality in individuals with obesity, WAT can be a promising source of mesenchymal stem cells (MSCs). As described by Leng and others, intravenous administration of clinical-grade MSCs was capable of improving pulmonary functional activity into seven COVID-19 patients (88). Due to its accessibility and amount of stem cells, subcutaneous WAT (scWAT) is the main source of MSCs, the AT-derived stem cells (ASCs). Once ASCs display high secretory activity, they possess therapeutic potential for the treatment of pulmonary damage caused by COVID-19 (89).
Therefore, we suggest that individuals with obesity tend to be more susceptible to SARS-CoV-2 infection and COVID-19 progression. These patients show aberrant RAAS activation, high ACE2 levels, low Ang(1-7) amounts, decreased antiviral immunity, higher amounts of EAT and presence of lipid deposits in large airways, which potentially act as viral reservoirs in heart and lung proximities, and higher chances of LiF-myofibroblast transdifferentiation and consequent pulmonary fibrosis. These features help to explain the disturbing statistics related to susceptibility, morbidity, and mortality of individuals affected with obesity. Research under potential applicability of ASCs is crucial for alleviating the impact of SARS-CoV-2 infection on this risk group (Figure 2).
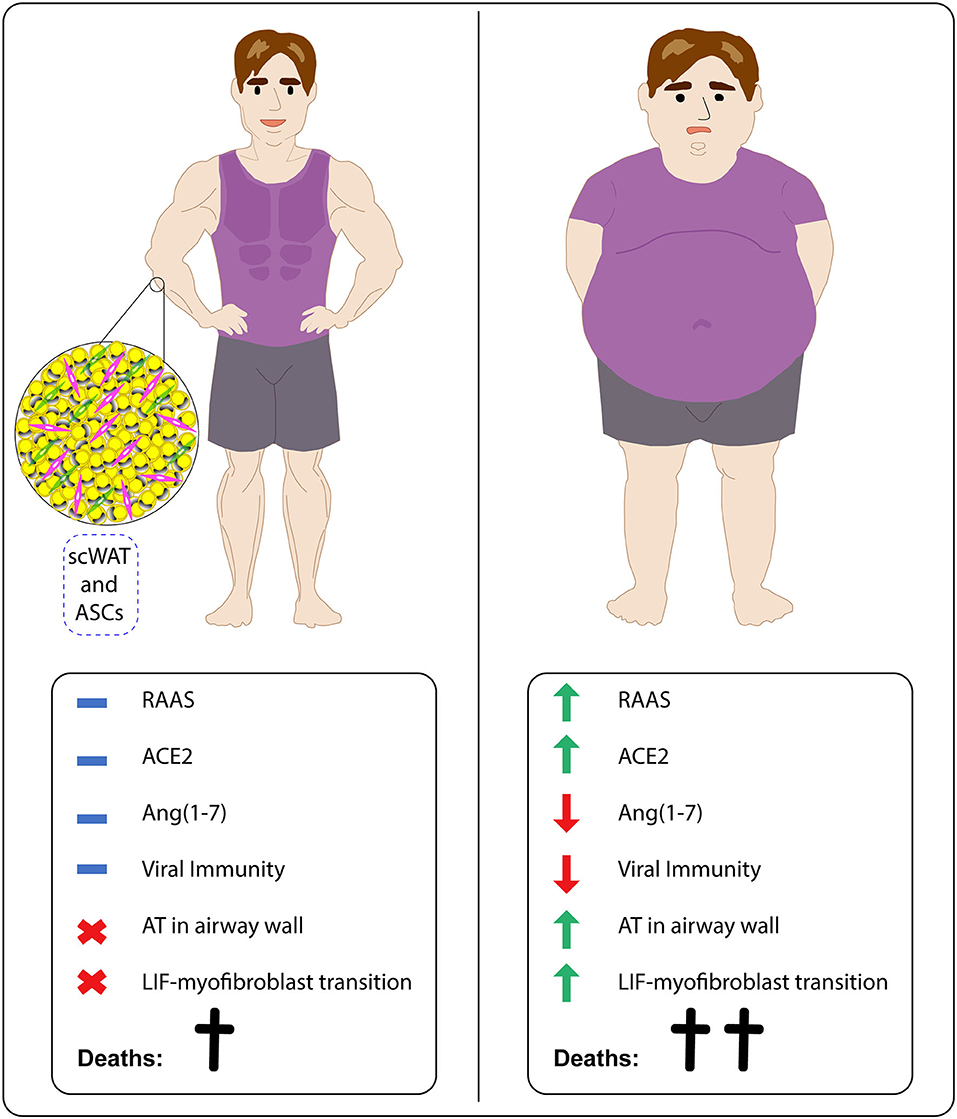
Figure 2. Obesity increases SARS-CoV-2 infection vulnerability in affected individuals by interfering in RAAS activation, antiviral immunity, fat tissue accumulation, and differentiation status of pulmonary fibrosis related cells. While lean individuals tend to show adequate RAAS activation, including ACE2 and Ang(1-7) levels, effective antiviral immune responses and absence of both adipose tissue (AT) deposits in airways and LiF-myofibroblast transdifferentiation, subjects with obesity display aberrant RAAS activation, favoring high ACE2 expression and low Ang(1-7) availability, decrease of immune responses against viruses and presence of both AT deposits in airways and LiF-myofibroblast transdifferentiation. ScWAT-derived stem cells (ASCs) could be applied in COVID-19 treatment.
Inflammatory Alterations in Obesity and COVID-19
Exacerbated inflammation is associated with increased risk of severe disease and mortality in patients with COVID-19 (90). COVID-19 patients commonly present intense pro-inflammatory markers activation such as IL-1, IL-6, IL-17, IL-18, IFN, and C-reactive protein (90, 91) with deep lymphopenia and substantial mononuclear cell infiltration in the lungs, heart, lymph nodes, spleen, and kidney (92, 93). Considering that the mortality and morbidity observed in COVID-19 patients is associated with excessive inflammation, a better understanding of the immunological parameters seen in patients infected with SARS-CoV-2 and people with obesity is necessary to better correlate COVID-19 and obesity, improving the identification of therapeutic targets.
Inflammation is an essential factor for the protection against countless threats that affects the organism during a lifetime. Deficiencies on immune system activation arises several disorders, which can be deleterious depending on the immunosuppressive potential of the disease (94). On the other hand, the chronic or excessive activation of the immune system also contributes to homeostasis breakdown and play a key role on classic inflammatory diseases progression, such as obesity and other metabolic disorders (95, 96). Obesity has been characterized by low grade chronic inflammation. This process leads to exacerbated and prolonged activation of both innate and adaptive immune responses, bringing on tissue damage and metabolic and physiologic alterations.
WAT is the central organ that orchestrates obesity and is composed by different kind of adipocytes, immune and endothelium cells, among others. During obesity, pro-inflammatory cytokines are overexpressed concomitantly with adipocytes hypertrophy and hyperplasia (71). The uncontrolled increase in the number and content of adipocytes lead to hypoxic microenvironment that is associated to cellular necrosis, activating local immune response (97). In the WAT, immunological cells, such as macrophages, natural killers (NK), T and B lymphocytes are major sources of interleukin-6 (IL-6) and tumor necrosis factor-alpha (TNF-α), which are central cytokines on driving inflammation linked to comorbidities establishment (98–100). In addition, macrophages are recruited by increased expression of monocyte chemoattractant protein-1 (MCP-1) and polarized to their pro-inflammatory profile due to the abundance of IL-6 and TNF-α (101–103). The huge macrophage infiltrate in the adipose tissue that accompanies obesity increases the source of inflammatory mediators, thus maintaining a chronic and persistent inflammation that affects systemic metabolism and immune response (99).
In obesity, inflammatory markers are found altered not only in the adipose tissue, but also in the serum, liver, skeletal muscle, lung, among other organs (71, 104, 105). As a result, the impact of immunomodulatory potential of obesity compromises systemically the response against homeostasis breakdown. Some comorbidities, such as insulin resistance, T2DM, hypertension, pulmonary illness, fatty-liver, and cardiovascular diseases are direct related to the chronic inflammation provided by obesity (106–108). In addition, these inflammatory modulations alter how the organism will face different pathogens infections, leading obesity to be considered a risk factor of a great number of them.
The intensive secretion of IL-6, TNF-α, and MCP-1 sustains the unbalanced inflammation on individuals with obesity. Together, these inflammatory mediators lead to several alterations on systemic responsiveness to nosocomial infections, increasing the incidence of generalized inflammation by the cytokine storm release (109, 110). Moreover, population with obesity also maintains a chronic inflammation on respiratory tract, presenting a higher susceptibility for acute lung injury caused by viral infections, such as H1N1 (110, 111). However, despite intensive inflammation has been considered factor that plays a major role in the higher mortality of population with obesity on several viral illnesses (110), there are other alterations on immunological system that contribute to this scenario. Currently, it has been shown a downregulation of a central pathway during immune system activation against viral infection, being also a factor responsible for the greater involvement observed during obesity (73).
Studies have shown impairment of IFN secretion on individuals with obesity, besides other pro-inflammatory cytokines are being overly produced (73, 112). IFN is the most important cytokine for combating viral infections and reducing this signalization pathway makes the organism more susceptible to the severity of viral disease (113, 114). Moreover, influenza vaccination seems to have a worse performance on obese or overweighed population, demonstrating that the efficacy of adaptive immune response is also decreased (115, 116). During influenza vaccination in humans, type I IFN signalization has been shown to be modulated throughout dendritic cells activation, which is central for long-term CD8+ T cells immunity (117). In addition, the use of type I and III IFN as vaccine adjuvants have demonstrated benefits for adaptive immune response development in vivo (118). The leptin overproduction found in individuals with obesity leads to an aberrant type I interferon secretion, thus impacting how the organism will handle vaccination-induced immunity (112). Currently, it is not possible to affirm if SARS-CoV-2 vaccine response will be less effective on individuals with obesity. However, the available data regarding the immune response of obese or overweighted individuals in vivo demonstrate that hiporresponsiveness to a COVID-19 vaccine could be a concern (119–121).
Thereby, it is clear that therapeutic targets may not be focused on turning off the pan activation of the immune system, but through the induction of the appropriate mediators for better combating the infectious agent. Moreover, the maintenance of controlled levels of inflammatory mediators is essential for homeostasis establishment and tissue recovery. Therefore, therapeutic targets aiming inflammatory response may be proposed to improve treatment of COVID-19 patients with obesity, once this group is at higher risk for developing severe viral illness considering their intrinsic alterations in inflammatory profile.
Coagulation Alterations in Obesity and COVID-19
Alterations in blood coagulation parameters have been increasingly implicated in COVID-19 severity, mortality, and morbidity (122–124). Several recent studies have shown that COVID-19 is commonly complicated with coagulopathy and disseminated intravascular coagulation (DIC) or associated with hypercoagulability together with a severe inflammatory state (125), leading to higher mortality (1, 8, 126). COVID-19 patients with acute respiratory failure present a severe hypercoagulability rather than consumptive coagulopathy (127). A significant portion of the patients hospitalized with COVID-19 usually present a pattern of coagulopathy characterized by elevations in D-dimer levels (8) and fibrin/fibrinogen degradation products, while abnormalities in prothrombin time, partial thromboplastin time, and platelet counts are relatively uncommon in initial presentations (123). Indeed, the DIC seen in the COVID-19 infection is clinically evidenced with high concentrations of D-dimer, being a poor prognostic characteristic (128) and higher risk of mortality (9). COVID-19 patients show a fulminant activation of coagulation and consumption of coagulation factors, with severe thrombocytopenia (low platelet count) (129). Likewise, obesity is highly related to a hypercoagulopathy status.
Excess body weight and especially abdominal fat accumulation can increase cardiovascular diseases morbidity and mortality, directly and indirectly. Direct effects are mediated by the structural and functional adaptations of the cardiovascular system to accommodate excess body weight, as well as by adipokine effects on inflammation and vascular homeostasis, leading to a pro-inflammatory and pro-thrombotic state. Indirect mechanisms occur concomitantly to other factors, such as insulin resistance, T2DM, visceral adiposity, hypertension, and dyslipidemia (130, 131).
Apart from metabolic and hemodynamic changes, central adiposity is also characterized by a systemic oxidative stress process, leading to the loss of the antithrombotic properties of endothelium (132). This mechanism partially supports the obesity as a pro-thrombotic clinical condition, presenting increased platelet activation and decreased fibrinolysis (133). Stimulation of vascular endothelium, platelets, and other circulating vascular cells by exacerbated production of proinflammatory cytokines by people with obesity promotes the upregulation of procoagulant factors and adhesion molecules, downregulation of anticoagulant regulatory proteins, increased thrombin generation, and enhanced platelet activation (134).
Adipose tissue could play a crucial role in the induction of a procoagulant state in obesity. Obesity is associated with overproduction of procoagulant microparticles (MP) and increased Tissue factor (TF), a primary initiator of the blood coagulation cascade through its Factor VII receptor activity, leading to hypercoagulopathy (135, 136). Moreover, release of adipokines/inflammatory factors by adipose tissue, such as TNF-α, IL-8, and IL-6, can lead to the release of ville Willebrand factor (vWF) from the endothelium and elevate platelet activation and aggregation, inducing coagulation factors production and changes in the vessel wall, thus contributing to the thrombosis event (137). Platelets store cytokines and growth factors and the entire subcellular apparatus for protein synthesis involved in the coagulation cascade, IL-1β, plasminogen activator inhibitor-1 (PAI-1) and TF (138). The inflammatory effects of cytokines also result in endothelial injury (139) and the substantial increase in the production of pro-inflammatory cytokines results in a cytokine storm, leading to an elevated risk of vascular hyperpermeability, organ failure, and death (140). Moreover, the platelets of individuals with obesity exhibit a series of abnormalities contributing to the status of hypercoagulability observed in these people (141). In this way, an inherent exacerbated inflammation state and a tendency to develop hypercoagulation together are the main causes for people with obesity present higher mortality rates due to COVID-19 (Figure 3).
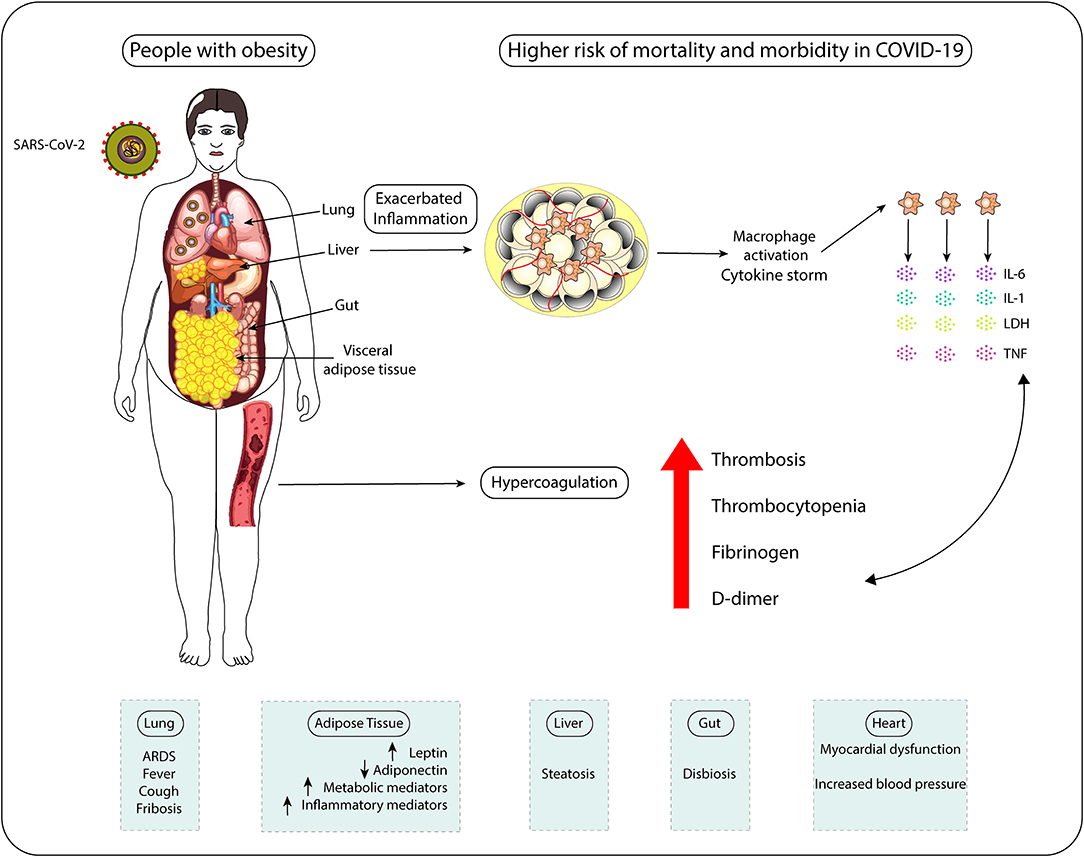
Figure 3. People with obesity display higher risk of mortality and morbidity in COVID-19 due to exacerbated inflammatory status and hypercoagulation tendency. Individuals with obesity show systemic chronic inflammation, which favors macrophage activation, cytokine storm occurrence (aberrant secretion of pro-inflammatory cytokines IL-6, IL-1, and TNF) and cytotoxicity (LDH release). This inflamed status associates with the increased clotting risk (hypercoagulation) presented by these patients. All these features make subjects with obesity more prone to develop pathological alterations in the physiology of lungs, AT, liver, heart, and intestines, which negatively influences gut microbiome composition. The impact of obesity-associated chronic inflammation on systems' physiology, including on antiviral immune responses, and the increased levels of coagulation-inducing mediators (fibrinogen and D-dimer) in COVID-19 patients help to explain the higher risks of these individuals to die of COVID-19 and to suffer with this infection compared to non-obese individuals.
It has also been shown that pro-trombotic factors are positively related to central fat. People with obesity have higher plasma concentrations of all pro-thrombotic factors (factor VII, fibrinogen, and vonWillebrand factor), as compared to non-obese individuals (142). Similarly, plasma concentrations of PAI-1, a physiological inhibitor of plasminogen activators (urokinase and tissue types) synthesized by adipose tissue, is highly elevated in plasma of people with obesity (143–145), predisposing those individuals to thrombotic complications. All of these conditions contribute to the progression of the prothrombotic state found in obesity (Figure 4).
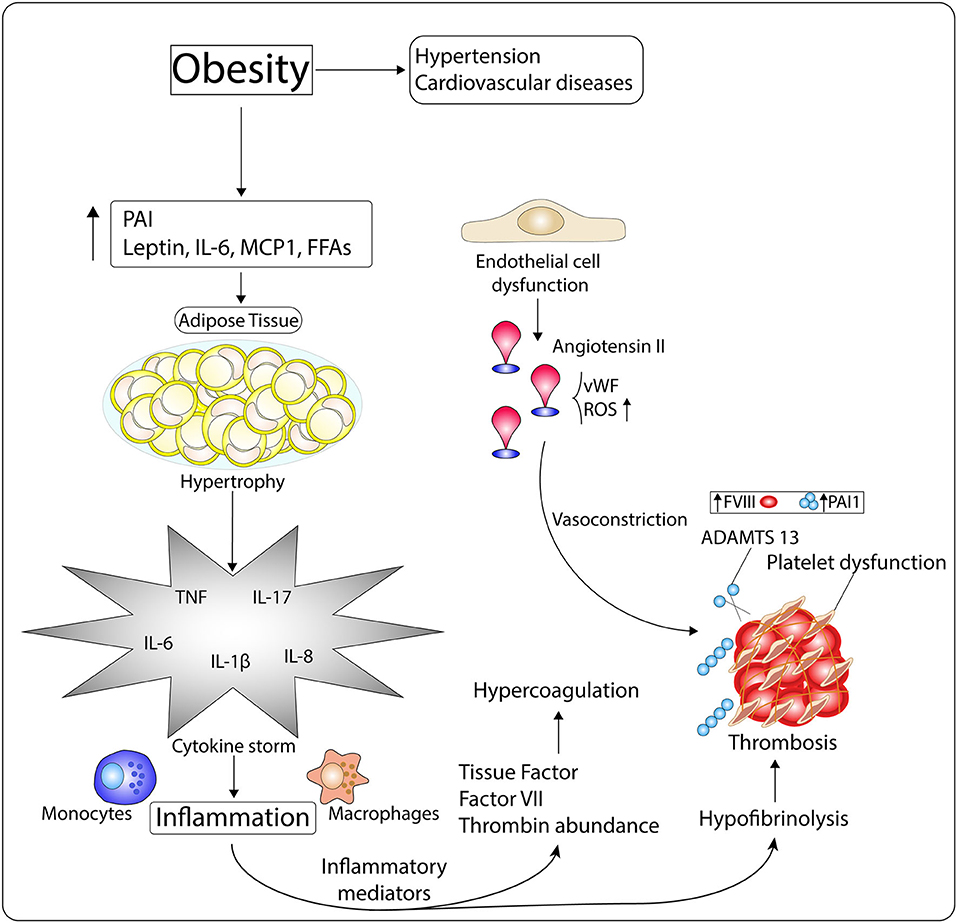
Figure 4. Mechanisms of the hypercoagulopathy and exacerbated inflammation observed in people with obesity. Obesity, which is intimately related with the pathogenesis of hypertension and cardiovascular diseases (CVDs), is characterized by high levels of Plasminogen Activator inhibitor I (PAI), leptin, IL-6, MCP-1, and free fatty acids (FFAs). These molecules induce white adipocyte hypertrophy, which then enables the occurrence of inflammation through cytokine storm. Inflammatory mediators impact on the availability of tissue factor (TF), factor VII (FVII), and thrombin-inhibiting factors, disrupting procoagulant-anticoagulant balance, and leading to hypercoagulation. Moreover, inflammation dysregulates fibrinolytic homeostasis through platelet dysfunction, increased FVIII and PAI-1 levels, and diminished ADMTS 13 activity, which enhance the risk of thrombosis. In addition, endothelial cell dysfunction, common in the obese phenotype, associates with Angiotensin II (Ang II) elevated amounts, von Willebrand factor (vWF) release, and oxidative stress, which induce vasoconstriction and thrombus formation.
Genetic factors are also correlated with higher susceptibility to coagulation impairment among individuals (146). Similarly, growing evidence has also supported the role of genetic factors as influencers in COVID-19 respiratory failure. In this context, a report has shown an association between ABO blood system and COVID-19 symptoms variation among individuals (147). This genome meta-analysis is in accordance with previous reports, in which blood-type O individuals are less affected by respiratory complications than type A (148, 149). These data are interesting, once blood-type O individuals are also less susceptible for thromboembolism development compared to non-type O (A, B, or AB) (150, 151). Until this moment, there is no evidence that obesity can be modulated by blood type. However, blood coagulation and COVID-19 severity need special attention since they are both influenced by ABO system. Understanding the inherent factors that support coagulation dysfunctions observed in COVID-19 patients is important to the establishment of therapeutics against this disease.
The impact of obesity, often related with other comorbidities, has been highlighted in severe forms of COVID-19 (152). In critically ill patients, coagulation alterations and inflammation are observed, with increased D-dimer and fibrinogen levels and, consequently, associated with a worse prognosis (126). The inflammation and hypoxemia related with a prothrombotic state are significant features of severe forms of COVID-19.
In conditions of obesity, coagulation disorders are emerging as an important issue in SARS-CoV-2 infection. Activation of leukocytes, endothelial cells, and platelets through the cytokine storm, positive regulation of TF and the subsequent generation of thrombin and fibrin formation can mediate the metabolic and cardiovascular complications associated to obesity (153). Together with coagulopathy, the thrombus formation in the microvascular environment contributes to tissue ischemia and organ dysfunction (154).
Under physiological conditions, shear stress increases the expression of ACE2, promoting the production of nitric oxide and reducing inflammation and proliferation in vascular endothelial cells. Endothelial cell activation/damage due to the coronavirus binding to the ACE2 receptor promoting acute inflammation and hypercoagulation may be of importance to explain the thrombotic burden observed (155). Primarily, angiotensin II induces PAI-1 expression by endothelial cells via the AT1 receptor, giving to a PAI-1/tPA shortcomig and a hypercoagulable state (1). Additionally, angiotensin II stimulates PAI-1 release from adipocytes and may in part account for the increased severity observed in those individuals with high BMI (156).
Clinically, the most prominent coagulation marker is elevation of D-dimer levels that has been consistently reported in many studies, representing a prognostic indicator for severity and mortality of disease (157). The high D-dimer indicates that other inflammatory markers including ferritin, IL-6, troponin I and lactate dehydrogenase (LDH) are accompanied by a secondary hypercoagulable condition (9, 158). It also has been suggested that the sustained inflammation due presence of continuous consumptive coagulopathy may contribute to the thrombocytopenia (159).
Therefore, it is highly recommended that COVID-19 patients with obesity are early and rapidly tested for coagulation screening, including the measurement of D-dimer and fibrinogen levels, following thromboembolic prophylaxis for critically ill hospitalized patients. Moreover, anti-coagulation drugs, such as heparin, may be especially important for treatment of COVID-19 patients with obesity, potentially leading to lower mortality rates in this high susceptible group of individuals.
Obesity and therapeutics against COVID-19
Obesity-related conditions increase the risk of disease severity caused by the SARS-CoV-2 (160, 161). Daily use of several medications is necessary for controlling such conditions and an important influence of them on the body's responsiveness to infections are being extensively discussed worldwide (162–165). Studies regarding this interaction create potential therapeutic targets aiming to soft or protect against the intense symptoms. In the absence of a COVID-19 vaccine, the study of available promising drugs is essential for combating the COVID-19, to reduce the high mortality rate observed on people with obesity.
As previously discussed, the chronic inflammation presented in the lung during obesity leads to a cytokine storm by overexpressing pro-inflammatory mediators, such as IL-6 and TNF-α (166). This is one of the mechanisms through which host unbalanced inflammation worsens the prognosis of individuals with obesity affected by COVID-19 (90, 167). Thereby, anti-inflammatory drugs could have an important role on protecting specially people with obesity against COVID-19 multi-organs damage, once inflammation is an inherent characteristic of obesity and COVID-19 aggravation. However, this has to be carefully analyzed, once reducing pan inflammatory response can also prolong the time required for effective virus clearance (167).
Effective class of anti-inflammatory drugs against COVID-19 is a concern. It has been suggested an increasing risk for developing severe COVID-19 by the use of non-steroidal anti-inflammatory drugs (NSAIDs) and corticosteroids. The chronic use of NSAIDs is associated to increased cardiovascular and pulmonary outcomes (168, 169), complications which are also found in COVID-19. Considering this, a synergism can in fact occur, but no evidence regarding this interaction to SARS-CoV-2 is available yet (170). Corticosteroids anti-inflammatory drugs lead to a high suppression of innate immune system and delay of viral clearance (171). Harmful responses have already been found after corticosteroids medication for different respiratory virus infection, such as influenza, SARS-CoV and MERS-CoV (165, 172). Nevertheless, the use of this class of drugs seems to have no positive interference on COVID-19 cases (173, 174). However, a preliminary in vitro study showed SARS-CoV-2 replication suppression by the use of a corticosteroid (175). Moreover, a correlation between lower gene expression of ACE2 and TMPRSS2 with inhaled corticosteroids medication in asthma patients has already been demonstrated (176). The current scenario of available data indicates that consistent evidences about benefits or harms of corticosteroids use on COVID-19 still need deep investigation for accurate conclusions. In the absence of both positive and negative conclusion about this question, clinicians are avoiding to prescribe this class of pan anti-inflammatory drug for COVID-19 cases, since controversial reports make the conclusion about their real impact still unknown.
Considering the above mentioned points, reports have suggested that specific cytokine blocking could be more efficient for protection against COVID-19 than systemic anti-inflammatory drugs (165, 177). In this context, the use of monoclonal antibodies makes it possible to selectively inhibit key agents that drive to hyperinflammation during COVID-19. Preliminary evidence suggests that IL-6 blockade could be helpful on curbing the cytokine storm, being a highly promising treatment for severe COVID-19 (178). Nevertheless, the clinical trials that could provide trustable answer about this question are still in progress (179, 180). In addition, studies regarding TNF-α therapy in COVID-19 are scarce and need urgent attention of the scientific community, given the importance of this cytokine on inflammatory diseases (181). As already been reported, SARS-CoV increases this TNF-α production, leading to tissue damage (182). Moreover, the treatment with anti-TNF antibodies reduces the severity of lung disease for both influenza and respiratory syncytial virus (183), indicating that it could be efficient on COVID-19 cases.
Besides blocking such pro-inflammatory cytokines, the improvement of antiviral responses is also an interesting point to be considered. As earlier discussed, increasing evidence suggests obesity-associated impairment of IFN secretion, enhancing the susceptibility of this group for viral severe illnesses (73, 112). Reports have shown that IFN-β treatment reduces SARS-CoV RNA replication in vitro (184, 185). Indeed, type 1 IFNs are being pointed as potential effective therapeutic for COVID-19 and seems to be even more effective for SARS-CoV-2 compared to other coronaviruses (186). Moreover, given the impact of this cytokine for adaptive immune system activation, it might be suggested IFN also as a vaccine adjuvant for enhancing effective anti-viral protective response for especially individuals with obesity.
Obesity-induced chronic inflammation leads to alterations of hemodynamic properties and increasing risk for coagulopathies establishment. Moreover, the activation of coagulation pathway also triggers inflammation (167). Some recent case reports brought thromboembolism as a COVID-19 complication (187–189). Considering the interplay between coagulopathies and inflammation, the combination of anti-inflammatories and anticoagulants drugs could be key for avoiding systemic complications in COVID-19 patients with obesity. Heparin and its low-molecular derivate are examples of frequently used anti-coagulant drugs for tromboprophylaxis due to their inherent potential of preventing blood clot occurrence (190). Currently, heparin is also known for its anti-inflammatory properties, expanding its potential for the treatment of coagulopathies (191). Heparin is already recommended as prophylactic agent against thromboembolism for COVID-19 cases and preliminary data suggests a better prognosis after the treatment (192, 193); it is important to emphasize that heparin anti-inflammatory properties in addition to its anticoagulant function may explain its great potential compared to single target anticoagulant drugs (191). In addition, a report has shown that heparin also binds to the spike protein and partially inhibits SARS-CoV-2 invasion in vitro, thus presenting anti-viral potential (194). Besides, other anticoagulant drugs can also act on immune response, such as antithrombins and anti-factor Xa. Study the effectiveness of these drugs can be an important step for ameliorating complications that specially overweighed individuals are suffering by COVID-19 (167).
Chronic inflammation provided by obesity also intermediates the establishment of metabolic disorders. Diabetes and hypertension are considered a risk factor for developing SARS-CoV-2 severe illness, both in the presence and absence of obesity (160). The high mortality rate that affects these groups brought questions regarding the impact of daily medication on the viral infectiveness capacity. The use of angiotensin-converting enzyme inhibitors (ACEi) and angiotensin-receptor blockers (ARBs) seems to improve ACE2 expression on pulmonary cells (195). Increasing the number of the viral entry receptor could lead to higher severity of the disease. However, the available data about these modulations of humans' RAAS is too limited for conclusions (196). Until now, the impact of discontinuing this medication on individuals with diabetes or hypertension conditions can be much worse, due to protection of vital organs provided by them (197, 198). In addition, there is recent evidence that, in fact, those drugs could protect against COVID-19, once the virus leads to a reduction of ACE2; increasing the amount of this receptor could interfere on the viral pathway somehow (198). Moreover, ACE2 plays an important role on reducing inflammation, what could ameliorate complications of COVID-19 (199). A report has shown a reduction of IL-6 secretion and an improvement of antiviral immune response, decreasing the viral load (163). Regarding other medicines used for diabetes, such as metformin, no interaction was found with ACE2 expression, indicating that its intake should not be a concern (200). Analyzing the available data about anti-hypertensive and anti-diabetic drugs, it is clear that the harms related to their interaction with COVID-19 are not evidenced enough compared to the known risks of stopping the treatment. Thereby, the use of those medications may not be discouraged, once these untreated comorbidities highly increase the mortality risks for COVID-19, so as the risks of developing secondary health problems.
Conclusion
A better understanding of the link between obesity and severe complications following COVID-19 infection is vital for implementing appropriate public health and therapeutic strategies to avoid COVID-19 severe symptoms and complications in people living with obesity. Adipose tissue from people with obesity show high expression of ACE2 receptor and can function as SARS-CoV-2 reservoir. Moreover, obesity can cause hyperglycemia via insulin resistance. SARS-CoV-2 may also cause hyperglycemia as well by infecting and killing pancreatic B-cells, leading to a worsen metabolic dysfunction of people with obesity and a poor prognostic of COVID-19. People with obesity present a pro-inflammatory and metabolic dysregulation that may favor the occurrence of the cytokine storm, implicated in COVID-19 pathophysiology of severe cases. Pulmonary lipofibroblasts can transdifferentiate into myofibroblasts aggravating the development of pulmonary fibrosis and consequently, contributing to the clinical severity of COVID-19, with development of a severe acute respiratory syndrome. Taken together, it may be crucial to better explore the role of visceral adipose tissue in the inflammatory response to SARS-CoV-2 infection and investigate the potential therapeutic effect of using specific anti-inflammatories (canakinumab or anakinra for IL-1β inhibition), anticoagulant (heparin), or anti-diabetic drugs in COVID-19 treatment since patients with obesity may benefit to a greater extent from a treatment that modulates these parameters.
Author Contributions
GP-N, HB-M, SF, GK, and KM wrote different sections of the manuscript. KM revised, wrote, and prepared the manuscript. IS prepared the figures.
Conflict of Interest
The authors declare that the research was conducted in the absence of any commercial or financial relationships that could be construed as a potential conflict of interest.
References
1. Huang C, Wang Y, Li X, Ren L, Zhao J, Hu Y, et al. Clinical features of patients infected with 2019 novel coronavirus in Wuhan, China. Lancet. (2020) 395:497–506. doi: 10.1016/S0140-6736(20)30183-5
2. WHO. Rational Use of Personal Protective Equipment for Coronavirus Disease 2019 (COVID-19). (2020). p. 1–7.
3. Sencan I, Kuzi S. Global threat of COVID 19 and evacuation of the citizens of different countries. TURKISH J Med Sci. (2020) 50:534–43. doi: 10.3906/sag-2004-21
4. Wan Y, Li J, Shen L, Zou Y, Hou L, Zhu L, et al. Enteric involvement in hospitalised patients with COVID-19 outside Wuhan. Lancet Gastroenterol Hepatol. (2020) 5:534–5. doi: 10.1016/S2468-12532030118-7
5. Wang D, Hu B, Hu C, Zhu F, Liu X, Zhang J, et al. Clinical characteristics of 138 hospitalized patients with 2019 novel coronavirus–infected Pneumonia in Wuhan, China. JAMA. (2020) 323:1061. doi: 10.1001/jama.2020.1585
6. Xydakis MS, Dehgani-Mobaraki P, Holbrook EH, Geisthoff UW, Bauer C, Hautefort C, et al. Smell and taste dysfunction in patients with COVID-19. Lancet Infect Dis. (2020). doi: 10.1016/S1473-3099(20)30293-0. [Epub ahead of print].
7. Rubin JE, Crowe SE. Histopathologic changes and SARS–CoV-2 immunostaining in the lung of a patient with COVID-19. Ann Intern Med. (2020) 172:ITC1–ITC14. doi: 10.7326/AWED202001070
8. Xiong M, Liang X, Wei Y. Changes in blood coagulation in patients with severe coronavirus disease 2019 (COVID-19): a meta-analysis. Br J Haematol. (2020) 189:1050–2. doi: 10.1111/bjh.16725
9. Zhou F, Yu T, Du R, Fan G, Liu Y, Liu Z, et al. Clinical course and risk factors for mortality of adult inpatients with COVID-19 in Wuhan, China: a retrospective cohort study. Lancet. (2020) 395:1054–62. doi: 10.1016/S0140-6736(20)30566-3
10. Lippi G, Henry BM. Chronic obstructive pulmonary disease is associated with severe coronavirus disease 2019 (COVID-19). Respir Med. (2020) 167:105941. doi: 10.1016/j.rmed.2020.105941
11. Flint SW, Tahrani AA. COVID-19 and obesity—lack of clarity, guidance, and implications for care. Lancet Diabetes Endocrinol. (2020) 8:474–5. doi: 10.1016/S2213-8587(20)30156-X
12. Boini KM, Koka S, Xia M, Ritter JK, Gehr TW, Li P. Sphingolipids in obesity and related complications. Front Biosci. (2018) 22:96–116. doi: 10.2741/4474
13. Jaganjac M, Almuraikhy S, Al-Khelaifi F, Al-Jaber M, Bashah M, Mazloum NA, et al. Combined metformin and insulin treatment reverses metabolically impaired omental adipogenesis and accumulation of 4-hydroxynonenal in obese diabetic patients. Redox Biol. (2017) 12:483–90. doi: 10.1016/j.redox.2017.03.012
14. Valencak TG, Osterrieder A, Schulz TJ. Sex matters: the effects of biological sex on adipose tissue biology and energy metabolism. Redox Biol. (2017) 12:806–13. doi: 10.1016/j.redox.2017.04.012
15. Gordon-Larsen P, Heymsfield SB. Obesity as a disease, not a behavior. Circulation. (2018) 137:1543–5. doi: 10.1161/CIRCULATIONAHA.118.032780
16. Nieuwdorp M, Stroes ES, Meijers JC, Büller H. Hypercoagulability in the metabolic syndrome. Curr Opin Pharmacol. (2005) 5:155–9. doi: 10.1016/j.coph.2004.10.003
17. Zhang K, Kaufman RJ. From endoplasmic-reticulum stress to the inflammatory response. Nature. (2008) 454:455–62. doi: 10.1038/nature07203
18. Sheridan PA, Paich HA, Handy J, Karlsson EA, Hudgens MG, Sammon AB, et al. Obesity is associated with impaired immune response to influenza vaccination in humans. Int J Obes. (2012) 36:1072–7. doi: 10.1038/ijo.2011.208
19. Hemalatha Rajkumar PB. The impact of obesity on immune response to infection and vaccine: an insight into plausible mechanisms. Endocrinol Metab Syndr. (2013) 2:113. doi: 10.4172/2161-1017.1000113
20. Upadhyay J, Farr O, Perakakis N, Ghaly W, Mantzoros C. Obesity as a disease. Med Clin North Am. (2018) 102:13–33. doi: 10.1016/j.mcna.2017.08.004
21. Lauby-Secretan B, Scoccianti C, Loomis D, Grosse Y, Bianchini F, Straif K. Body fatness and cancer — Viewpoint of the IARC Working Group. N Engl J Med. (2016) 375:794–8. doi: 10.1056/NEJMsr1606602
22. Dhurandhar NV, Bailey D, Thomas D. Interaction of obesity and infections. Obes Rev. (2015) 16:1017–29. doi: 10.1111/obr.12320
23. Klonoff DC, Umpierrez GE. COVID-19 in patients with diabetes: risk factors that increase morbidity. Metabolism. (2020) 108:154224. doi: 10.1016/j.metabol.2020.154224
24. Zheng KI, Gao F, Wang X-B, Sun Q-F, Pan K-H, Wang T-Y, et al. Obesity as a risk factor for greater severity of COVID-19 in patients with metabolic associated fatty liver disease. Metabolism. (2020) 108:154244. doi: 10.1016/j.metabol.2020.154244
25. Deng G, Yin M, Chen X, Zeng F. Clinical determinants for fatality of 44, 672 patients with COVID-19. Crit Care. (2020) 24:179. doi: 10.1186/s13054-020-02902-w
26. Kass DA, Duggal P, Cingolani O. Correspondence severe COVID-19 disease. Lancet. (2020) 6736:19–20. doi: 10.1016/S0140-6736(20)31024-2
27. Richardson S, Hirsch JS, Narasimhan M, Crawford JM, McGinn T, Davidson KW, et al. Presenting characteristics, comorbidities, and outcomes among 5700 patients hospitalized with COVID-19 in the New York City Area. JAMA. (2020) 10022:1–8. doi: 10.1001/jama.2020.6775
28. Lighter J, Phillips M, Hochman S, Sterling S, Johnson D, Francois F, et al. Obesity in patients younger than 60 years is a risk factor for Covid-19 hospital admission. Infect Dis Soc Am. (2020). doi: 10.1093/cid/ciaa415. [Epub ahead of print].
29. Bello-Chavolla OY, Bahena-López JP, Antonio-Villa NE, Vargas-Vázquez A, González-Díaz A, Márquez-Salinas A, et al. Predicting mortality due to SARS-CoV-2: a mechanistic score relating obesity and diabetes to COVID-19 outcomes in Mexico. J Clin Endocrinol Metab. (2020) 105:dgaa346. doi: 10.1210/clinem/dgaa346
30. Simonnet A, Chetboun M, Poissy J, Raverdy V, Noulette J, Duhamel A, et al. High prevalence of obesity in severe acute respiratory syndrome coronavirus-2 (SARS-CoV-2) requiring invasive mechanical ventilation. Obesity. (2020) 28:1195–99. doi: 10.1002/oby.22831
31. Barrasa H, Rello J, Tejada S, Martín A, Balziskueta G, Vinuesa C, et al. SARS-Cov-2 in Spanish intensive care: early experience with 15-day survival in Vitoria. Anaesth Crit Care Pain Med. (2020). doi: 10.1016/j.accpm.2020.04.001. [Epub ahead of print].
32. Onder G, Rezza G, Brusaferro S. Case-fatality rate and characteristics of patients dying in relation to COVID-19 in Italy. J Am Med Assoc. (2020) 2019:2019–20. doi: 10.1001/jama.2020.4683
33. Finer N, Garnett SP, Bruun JM. COVID-19 and obesity. Clin Obes. (2020) 10:1–2. doi: 10.1111/cob.12365
34. Chen Q, Zheng Z, Zhang C, Zhang X, Wu H, Wang J, et al. Clinical characteristics of 145 patients with corona virus disease 2019 (COVID-19) in Taizhou, Zhejiang, China. Infection. (2020) 2019:1–9. doi: 10.1007/s15010-020-01432-5
35. Kim ES, Chin BS, Kang CK, Kim NJ, Kang YM, Choi JP, et al. Clinical course and outcomes of patients with severe acute respiratory syndrome coronavirus 2 infection: a preliminary report of the first 28 patients from the korean cohort study on COVID-19. J Korean Med Sci. (2020) 35:1–12. doi: 10.3346/JKMS.2020.35.E142
36. Zhou P, Yang X-L, Wang X-G, Hu B, Zhang L, Zhang W, et al. A Pneumonia outbreak associated with a new coronavirus of probable bat origin. Nature. (2020) 579:270–3. doi: 10.1038/s41586-020-2012-7
37. Hoffmann M, Kleine-Weber H, Schroeder S, Krüger N, Herrler T, Erichsen S, et al. SARS-CoV-2 cell entry depends on ACE2 and TMPRSS2 and is blocked by a clinically proven protease inhibitor. Cell. (2020) 181:271–80.e8. doi: 10.1016/j.cell.2020.02.052
38. Donoghue M, Hsieh F, Baronas E, Godbout K, Gosselin M, Stagliano N, et al. A novel angiotensin-converting enzyme–related carboxypeptidase (ACE2) converts angiotensin I to angiotensin 1-9. Circ Res. (2000) 87:E1–9. doi: 10.1161/01.RES.87.5.e1
39. Tipnis SR, Hooper NM, Hyde R, Karran E, Christie G, Turner AJ. A human homolog of angiotensin-converting enzyme. J Biol Chem. (2000) 275:33238–43. doi: 10.1074/jbc.M002615200
40. Patel VB, Zhong J-C, Grant MB, Oudit GY. Role of the ACE2/Angiotensin 1–7 axis of the renin–angiotensin system in heart failure. Circ Res. (2016) 118:1313–26. doi: 10.1161/CIRCRESAHA.116.307708
41. Imai Y, Kuba K, Rao S, Huan Y, Guo F, Guan B, et al. Angiotensin-converting enzyme 2 protects from severe acute lung failure. Nature. (2005) 436:112–6. doi: 10.1038/nature03712
42. Kuba K, Imai Y, Rao S, Jiang C, Penninger JM. Lessons from SARS: control of acute lung failure by the SARS receptor ACE2. J Mol Med. (2006) 84:814–20. doi: 10.1007/s00109-006-0094-9
43. Hamming I, Cooper M, Haagmans B, Hooper N, Korstanje R, Osterhaus A, et al. The emerging role of ACE2 in physiology and disease. J Pathol. (2007) 212:1–11. doi: 10.1002/path.2162
44. Luo Y, Liu C, Guan T, Li Y, Lai Y, Li F, et al. Association of ACE2 genetic polymorphisms with hypertension-related target organ damages in south Xinjiang. Hypertens Res. (2019) 42:681–9. doi: 10.1038/s41440-018-0166-6
45. Bader M, Ganten D. Update on tissue renin–angiotensin systems. J Mol Med. (2008) 86:615–21. doi: 10.1007/s00109-008-0336-0
46. Vickers C, Hales P, Kaushik V, Dick L, Gavin J, Tang J, et al. Hydrolysis of biological peptides by human angiotensin-converting enzyme-related carboxypeptidase. J Biol Chem. (2002) 277:14838–43. doi: 10.1074/jbc.M200581200
47. Yan T, Xiao R, Lin G. Angiotensin-converting enzyme 2 in severe acute respiratory syndrome coronavirus and SARS-CoV-2: a double-edged sword? FASEB J. (2020) 34:6017–26. doi: 10.1096/fj.202000782
48. Walls AC, Park YJ, Tortorici MA, Wall A, McGuire AT, Veesler D. Structure, function, and antigenicity of the SARS-CoV-2 spike glycoprotein. Cell. (2020) 181:281–92.e6. doi: 10.1016/j.cell.2020.02.058
49. Xia S, Liu M, Wang C, Xu W, Lan Q, Feng S, et al. Inhibition of SARS-CoV-2 (previously 2019-nCoV) infection by a highly potent pan-coronavirus fusion inhibitor targeting its spike protein that harbors a high capacity to mediate membrane fusion. Cell Res. (2020) 30:343–55. doi: 10.1038/s41422-020-0305-x
50. Shirato K, Kanou K, Kawase M, Matsuyama S. Clinical isolates of human coronavirus 229E bypass the endosome for cell entry. J Virol. (2017) 91:e01387-16. doi: 10.1128/JVI.01387-16
51. Shirato K, Kawase M, Matsuyama S. Wild-type human coronaviruses prefer cell-surface TMPRSS2 to endosomal cathepsins for cell entry. Virology. (2018) 517:9–15. doi: 10.1016/j.virol.2017.11.012
52. Lan J, Ge J, Yu J, Shan S, Zhou H, Fan S, et al. Structure of the SARS-CoV-2 spike receptor-binding domain bound to the ACE2 receptor. Nature. (2020) 581:215–20. doi: 10.1038/s41586-020-2180-5
53. Yang XH, Deng W, Tong Z, Liu YX, Zhang LF, Zhu H, et al. Mice transgenic for human angiotensin-converting enzyme 2 provide a model for SARS coronavirus infection. Comp Med. (2007) 57:450–9.
54. Zou X, Chen K, Zou J, Han P, Hao J, Han Z. Single-cell RNA-seq data analysis on the receptor ACE2 expression reveals the potential risk of different human organs vulnerable to 2019-nCoV infection. Front. Med. (2020)14:185–92. doi: 10.1007/s11684-020-0754-0
55. Xu H, Zhong L, Deng J, Peng J, Dan H, Zeng X, et al. High expression of ACE2 receptor of 2019-nCoV on the epithelial cells of oral mucosa. Int J Oral Sci. (2020) 12:8. doi: 10.1038/s41368-020-0074-x
56. Sungnak W, Huang N, Bécavin C, Berg M, Queen R, Litvinukova M, et al. (2020). SARS-CoV-2 entry factors are highly expressed in nasal epithelial cells together with innate immune genes. Nat. Med. 26:681–7. doi: 10.1038/s41591-020-0868-6
57. Kruglikov IL, Scherer PE. The role of adipocytes and adipocyte-like cells in the severity of COVID-19 infections. Obesity. (2020) 28:1187–90. doi: 10.1002/oby.22856
58. Shimizu I, Walsh K. The whitening of brown fat and its implications for weight management in obesity. Curr Obes Rep. (2015) 4:224–9. doi: 10.1007/s13679-015-0157-8
59. Pacurari M, Kafoury R, Tchounwou PB, Ndebele K. The renin-angiotensin-aldosterone system in vascular inflammation and remodeling. Int J Inflam. (2014) 2014:689360. doi: 10.1155/2014/689360
60. Velloso LA, Folli F, Sun XJ, White MF, Saad MJA, Kahn CR. Cross-talk between the insulin and angiotensin signaling systems. Proc Natl Acad Sci USA. (1996) 93:12490–5. doi: 10.1073/pnas.93.22.12490
61. Yuan L, Li X, Xui GL, Qi CJ. Effects of renin-angiotensin system blockade on islet function in diabetic rats. J Endocrinol Invest. (2010) 33:13–9. doi: 10.1007/BF03346544
62. Luther JM, Luo P, Kreger MT, Brissova M, Dai C, Whitfield TT, et al. Aldosterone decreases glucose-stimulated insulin secretion in vivo in mice and in murine islets. Diabetologia. (2011) 54:2152–63. doi: 10.1007/s00125-011-2158-9
63. Jing F, Mogi M, Horiuchi M. Role of renin-angiotensin-aldosterone system in adipose tissue dysfunction. Mol Cell Endocrinol. (2013) 378:23–8. doi: 10.1016/j.mce.2012.03.005
64. Borghi F, Sevá-Pessôa B, Grassi-Kassisse DM. The adipose tissue and the involvement of the renin–angiotensin–aldosterone system in cardiometabolic syndrome. Cell Tissue Res. (2016) 366:543–8. doi: 10.1007/s00441-016-2515-6
65. Kawabe Y, Mori J, Morimoto H, Yamaguchi M, Miyagaki S, Ota T, et al. ACE2 exerts anti-obesity effect via stimulating brown adipose tissue and induction of browning in white adipose tissue. Am J Physiol Endocrinol Metab. (2019) 317:E1140–9. doi: 10.1152/ajpendo.00311.2019
66. Yang L, Han Y, Nilsson-Payant BE, Gupta V, Wang P, Duan X, et al. A human pluripotent stem cell-based platform to study SARS-CoV-2 tropism and model virus infection in human cells and organoids. Cell Stem Cell. (2020) 27:125–36.e7. doi: 10.1016/j.stem.2020.06.015
67. Zhang W, Xu Y-Z, Liu B, Wu R, Yang Y-Y, Xiao X-Q, et al. Pioglitazone upregulates angiotensin converting enzyme 2 expression in insulin-sensitive tissues in rats with high-fat diet-induced nonalcoholic steatohepatitis. Sci World J. (2014) 2014:1–7. doi: 10.1155/2014/603409
68. Romaní-Pérez M, Outeiriño-Iglesias V, Moya CM, Santisteban P, González-Matías LC, Vigo E, et al. Activation of the GLP-1 receptor by liraglutide increases ACE2 expression, reversing right ventricle hypertrophy, and improving the production of SP-A and SP-B in the lungs of type 1 diabetes rats. Endocrinology. (2015) 156:3559–69. doi: 10.1210/en.2014-1685
69. Tikoo K, Patel G, Kumar S, Karpe PA, Sanghavi M, Malek V, et al. Tissue specific up regulation of ACE2 in rabbit model of atherosclerosis by atorvastatin: role of epigenetic histone modifications. Biochem Pharmacol. (2015) 93:343–51. doi: 10.1016/j.bcp.2014.11.013
70. Petrakis D, Margină D, Tsarouhas K, Tekos F, Stan M, Nikitovic D, et al. Obesity - a risk factor for increased COVID-19 prevalence, severity and lethality (Review). Mol. Med. Rep. (2020) 22:9–19. doi: 10.3892/mmr.2020.11127
71. Faloia E, Michetti G, De Robertis M, Luconi MP, Furlani G, Boscaro M. Inflammation as a link between obesity and metabolic syndrome. J Nutr Metab. (2012) 2012:7. doi: 10.1155/2012/476380
72. Mirsoian A, Bouchlaka MN, Sckisel GD, Chen M, Pai CCS, Maverakis E, et al. Adiposity induces lethal cytokine storm after systemic administration of stimulatory immunotherapy regimens in aged mice. J Exp Med. (2014) 211:2373–83. doi: 10.1084/jem.20140116
73. Teran-Cabanillas E, Montalvo-Corral M, Caire-Juvera G, Moya-Camarena SY, Hernández J. Decreased interferon-α and interferon-β production in obesity and expression of suppressor of cytokine signaling. Nutrition. (2013) 29:207–12. doi: 10.1016/j.nut.2012.04.019
74. Louie JK, Acosta M, Samuel MC, Schechter R, Vugia DJ, Harriman K, et al. A novel risk factor for a novel virus: obesity and 2009 pandemic influenza a (H1N1). Clin Infect Dis. (2011) 52:301–12. doi: 10.1093/cid/ciq152
75. Smith AG, Sheridan PA, Harp JB, Beck MA. Diet-induced obese mice have increased mortality and altered immune responses when infected with influenza virus. J Nutr. (2007) 137:1236–43. doi: 10.1093/jn/137.5.1236
76. Maier HE, Lopez R, Sanchez N, Ng S, Gresh L, Ojeda S, et al. Obesity increases the duration of influenza a virus shedding in adults. J Infect Dis. (2018) 218:1378–82. doi: 10.1093/infdis/jiy370
77. Yao X-H, He Z-C, Li T-Y, Zhang H-R, Wang Y, Mou H, et al. Pathological evidence for residual SARS-CoV-2 in pulmonary tissues of a ready-for-discharge patient. Cell Res. (2020) 30:541–3. doi: 10.1038/s41422-020-0318-5
78. Ibricevic A, Pekosz A, Walter MJ, Newby C, Battaile JT, Brown EG, et al. Influenza virus receptor specificity and cell tropism in mouse and human airway epithelial cells. J Virol. (2006) 80:7469–80. doi: 10.1128/jvi.02677-05
79. Zhao L. Obesity accompanying COVID-19: the role of epicardial fat. Obesity. (2020). doi: 10.1002/oby.22867. [Epub ahead of print].
80. Patel VB, Mori J, McLean BA, Basu R, Das SK, Ramprasath T, et al. ACE2 deficiency worsens epicardial adipose tissue inflammation and cardiac dysfunction in response to diet-induced obesity. Diabetes. (2016) 65:85–95. doi: 10.2337/db15-0399
81. Iacobellis G. Local and systemic effects of the multifaceted epicardial adipose tissue depot. Nat Rev Endocrinol. (2015) 11:363–71. doi: 10.1038/nrendo.2015.58
82. Malavazos AE, Goldberger JJ, Iacobellis G. Does epicardial fat contribute to COVID-19 myocardial inflammation? Eur Heart J. (2020) 41:2333. doi: 10.1093/eurheartj/ehaa471
83. Elliot JG, Donovan GM, Wang KCW, Green FHY, James AL, Noble PB. Fatty airways: implications for obstructive disease. Eur Respir J. (2019) 54:1900857. doi: 10.1183/13993003.00857-2019
84. Wagner PD. The physiological basis of pulmonary gas exchange: implications for clinical interpretation of arterial blood gases. Eur Respir J. (2015) 45:227–43. doi: 10.1183/09031936.00039214
85. Kruger P, Saffarzadeh M, Weber ANR, Rieber N, Radsak M, von Bernuth H, et al. Neutrophils: between host defence, immune modulation, and tissue injury. PLoS Pathog. (2015) 11:e1004651. doi: 10.1371/journal.ppat.1004651
86. Boros LG, Torday JS, Paul Lee WN, Rehan VK. Oxygen-induced metabolic changes and transdifferentiation in immature fetal rat lung lipofibroblasts. Mol Genet Metab. (2002) 77:230–6. doi: 10.1016/S1096-71920200140-3
87. Zhu Y, Jiang M, Gao L, Huang X. Single cell analysis of ace2 expression reveals the potential targets for 2019-nCoV. Preprints. (2020) 1–14. doi: 10.20944/preprints202002.0221.v1
88. Leng Z, Zhu R, Hou W, Feng Y, Yang Y, Han Q, et al. Transplantation of ACE2- Mesenchymal stem cells improves the outcome of patients with covid-19 pneumonia. Aging Dis. (2020) 11:216–28. doi: 10.14336/AD.2020.0228
89. Gentile P, Garcovich S. Concise review: adipose-derived stem cells (ASCs) and adipocyte-secreted exosomal microRNA (A-SE-miR) modulate cancer growth and promote wound repair. J Clin Med. (2019) 8:855. doi: 10.3390/jcm8060855
90. Mehta P, McAuley DF, Brown M, Sanchez E, Tattersall RS, Manson JJ. COVID-19: consider cytokine storm syndromes and immunosuppression. Lancet. (2020) 395:1033–4. doi: 10.1016/S0140-6736(20)30628-0
91. Ulhaq ZS, Soraya GV. Interleukin-6 as a potential biomarker of COVID-19 progression. Méd Mal Infect. (2020) 50:382–3. doi: 10.1016/j.medmal.2020.04.002
92. Merad M, Martin JC. Pathological inflammation in patients with COVID-19: a key role for monocytes and macrophages. Nat Rev Immunol. (2020) 20:355–62. doi: 10.1038/s41577-020-0331-4
93. Xu Z, Shi L, Wang Y, Zhang J, Huang L, Zhang C, et al. Pathological findings of COVID-19 associated with acute respiratory distress syndrome. Lancet Respir Med. (2020) 8:420–2. doi: 10.1016/S2213-26002030076-X
94. Hayes BF, Fauci AS. Disorders of the immune system. Natl Inst Alergy Infect Dis. (2012) 302–21. Retrieved from: https://www.niaid.nih.gov/research/immune-systemdisorders
95. Hotamisligil GS. Inflammation and metabolic disorders 1. Nature. (2006) 444:860–7. doi: 10.1038/nature05485
96. Rodríguez-Hernández H, Simental-Mendía LE, Rodríguez-Ramírez G, Reyes-Romero MA. Obesity and inflammation: epidemiology, risk factors, and markers of inflammation. Int J Endocrinol. (2013) 2013:678159. doi: 10.1155/2013/678159
97. Cinti S, Mitchell G, Barbatelli G, Murano I, Ceresi E, Faloia E, et al. Adipocyte death defines macrophage localization and function in adipose tissue of obese mice and humans. J Lipid Res. (2005) 46:2347–55. doi: 10.1194/jlr.M500294-JLR200
98. Matthews VB, Allen TL, Risis S, Chan MHS, Henstridge DC, Watson N, et al. Interleukin-6-deficient mice develop hepatic inflammation and systemic insulin resistance. Diabetologia. (2010) 53:2431–41. doi: 10.1007/s00125-010-1865-y
99. Saltiel AR, Olefsky JM. Inflammatory linking obesity and metabolic disease and metabolic disease. J Clin Invest. (2017) 127:1–4. doi: 10.1172/JCI92035
100. Stolarczyk E. Adipose tissue inflammation in obesity: a metabolic or immune response? Curr Opin Pharmacol. (2017) 37:35–40. doi: 10.1016/j.coph.2017.08.006
101. Kanda H, Tateya S, Tamori Y, Kotani K, Hiasa K, Kitazawa, R, et al. MCP-1 contributes to macrophage infiltration into adipose tissue, insulin resistance, and hepatic steatosis in obesity1. J Clin Invest. (2006) 116:1494–505. doi: 10.1172/JCI26498DS1
102. Lumeng CN, Bodzin JL, Saltiel AR, Lumeng CN, Bodzin JL, Saltiel AR. Obesity induces a phenotypic switch in adipose tissue macrophage polarization Find the latest version : obesity induces a phenotypic switch in adipose tissue macrophage polarization. J Clin Invest. (2007) 117:175–84. doi: 10.1172/JCI29881.both
103. Kawano Y, Nakae J, Watanabe N, Kikuchi T, Tateya S, Tamori Y, et al. Colonic pro-inflammatory macrophages cause insulin resistance in an intestinal Ccl2/Ccr2-dependent manner. Cell Metab. (2016) 24:295–310. doi: 10.1016/j.cmet.2016.07.009
104. McClean KM, Kee F, Young IS, Elborn JS. Obesity and the lung: 1 Epidemiology. Thorax. (2008) 63:649–54. doi: 10.1136/thx.2007.086801
105. Schmidt FM, Weschenfelder J, Sander C, Minkwitz J, Thormann J, Chittka T, et al. Inflammatory cytokines in general and central obesity and modulating effects of physical activity. PLoS ONE. (2015) 10:e0121971. doi: 10.1371/journal.pone.0121971
106. Al-Goblan AS, Al-Alfi MA, Khan MZ. Mechanism linking diabetes mellitus and obesity. Diabetes Metab Syndr Obes Targets Ther. (2014) 7:587–91. doi: 10.2147/DMSO.S67400
107. Jiang SZ, Lu W, Zong XF, Ruan HY, Liu Y. Obesity and hypertension. Exp Ther Med. (2016) 12:2395–9. doi: 10.3892/etm.2016.3667
108. Sarwar R, Pierce N, Koppe S. Obesity and nonalcoholic fatty liver disease: current perspectives. Diabetes Metab Syndr Obes Targets Ther. (2018) 11:533–42. doi: 10.2147/DMSO.S146339
109. Dominguez-Cherit G, Lapinsky SE, Macias AE, Torre A, De Poblano-morales M, Baltazar-torres JA, et al. Critically Ill patients with 2009 influenza A(H1N1) in Mexico. JAMA. (2009) 302:1880–87. doi: 10.1001/jama.2009.1536
110. Tsatsanis C, Margioris AN, Kontoyiannis DP. Association between H1N1 infection severity and obesity—adiponectin as a potential etiologic factor. J Infect Dis. (2010) 202:459–60. doi: 10.1086/653842
111. Milner JJ, Rebeles J, Dhungana S, Stewart DA, Sumner SCJ, Meyers HM, et al. Obesity increases mortality and modulates the lung metabolome during pandemic H1N1 influenza virus infection in mice. Physiol Behav. (2016) 176:139–48. doi: 10.1016/j.physbeh.2017.03.040
112. Terán-Cabanillas E, Hernández J. Role of leptin and SOCS3 in inhibiting the type I interferon response during obesity. Inflammation. (2017) 40:58–67. doi: 10.1007/s10753-016-0452-x
113. Fensterl V, Sen GC. Interferons and viral infections. Biofactors. (2009) 35:14–20. doi: 10.1002/biof.6
114. Murira A, Lamarre A. Type-I interferon responses: from friend to foe in the battle against chronic viral infection. Front Immunol. (2016) 7:609. doi: 10.3389/fimmu.2016.00609
115. Park H, Shim S, Lee E, Cho W, Park S, Jeon H, et al. (2014). Obesity-induced chronic inflammation is associated with the reduced efficacy of influenza vaccine. Hum Vaccin Immunother. 10:1181–6. doi: 10.4161/hv.28332
116. Luzi L, Radaelli MG. Influenza and obesity: its odd relationship and the lessons for COVID-19 pandemic. Acta Diabetol. (2020) 57:759–64. doi: 10.1007/s00592-020-01522-8
117. Athale S, Banchereau R, Thompson-snipes L, Wang Y, Palucka K, Pascual V, et al. Influenza vaccines differentially regulate the interferon response in human dendritic cell subsets. Sci Transl Med. (2017) 9:eaaf9194. doi: 10.1126/scitranslmed.aaf9194.Influenza
118. Ye L, Ohnemus A, Ong LC, Gad HH, Hartmann R, Lycke N, et al. Type I and Type III interferons differ in their adjuvant activities for influenza vaccines. J Virol. (2019) 93:1–8. doi: 10.1128/jvi.01262-19
119. Gardner EM, Beli E, Clinthorne JF, Duriancik DM. Energy intake and response to infection with influenza. Annu Rev Nutr. (2019) 31:353–67. doi: 10.1146/annurev-nutr-081810-160812
120. Kim Y, Kim J, Kim D, Nam J, Shim S, Choi Y, et al. Diet-induced obesity dramatically reduces the efficacy of a 2009 pandemic H1N1 vaccine in a mouse model. J Infect Dis. (2012) 205:244–51. doi: 10.1093/infdis/jir731
121. Painter SD, Ovsyannikova IG, Poland GA, Vaccine C, Clinic M. The weight of obesity on the human immune response to vaccination. Vaccine. (2015) 33:4422–9. doi: 10.1016/j.vaccine.2015.06.101.The
122. Arentz M, Yim E, Klaff L, Lokhandwala S, Riedo FX, Chong M, et al. Characteristics and outcomes of 21 critically Ill patients with COVID-19 in Washington state. JAMA. (2020) 323:1612. doi: 10.1001/jama.2020.4326
123. Connors JM, Levy JH. COVID-19 and its implications for thrombosis and anticoagulation. Blood. (2020) 135:2033–40. doi: 10.1182/blood.2020006000
124. Terpos E, Ntanasis-Stathopoulos I, Elalamy I, Kastritis E, Sergentanis TN, Politou M, et al. Hematological findings and complications of COVID-19. Am J Hematol. (2020) 95:834–47. doi: 10.1002/ajh.25829
125. Panigada M, Bottino N, Tagliabue P, Grasselli G, Novembrino C, Chantarangkul V, et al. Hypercoagulability of COVID-19 patients in intensive care unit: a report of thromboelastography findings and other parameters of hemostasis. J Thromb Haemost. (2020) 18:1738–42. doi: 10.1111/jth.14850
126. Tang N, Li D, Wang X, Sun Z. Abnormal coagulation parameters are associated with poor prognosis in patients with novel coronavirus pneumonia. J Thromb Haemost. (2020) 18:844–7. doi: 10.1111/jth.14768
127. Spiezia L, Boscolo A, Poletto F, Cerruti L, Tiberio I, Campello E, et al. COVID-19-related severe hypercoagulability in patients admitted to intensive care unit for acute respiratory failure. Thromb Haemost. (2020) 120:998–1000. doi: 10.1055/s-0040-1710018
128. Liu PP, Blet A, Smyth D, Li H. The science underlying COVID- 19: implications for the cardiovascular system. Circulation. (2020) 142:68–78. doi: 10.1161/circulationaha.120.047549
129. Lippi G, Plebani M, Henry BM. Thrombocytopenia is associated with severe coronavirus disease 2019 (COVID-19) infections: a meta-analysis. Clin Chim Acta. (2020) 506:145–8. doi: 10.1016/j.cca.2020.03.022
130. Tchernof A, Després JP. Pathophysiology of human visceral obesity: an update. Physiol Rev. (2013) 93:359–404. doi: 10.1152/physrev.00033.2011
131. Csige I, Ujvárosy D, Szabó Z, Lorincz I, Paragh G, Harangi M, et al. The impact of obesity on the cardiovascular system. J Diabetes Res. (2018) 2018:3407306. doi: 10.1155/2018/3407306
132. Anfossi G, Russo I, Trovati M. Platelet dysfunction in central obesity. Nutr Metab Cardiovasc Dis. (2009) 19:440–9. doi: 10.1016/j.numecd.2009.01.006
133. Barale C, Russo I. Influence of cardiometabolic risk factors on platelet function. Int J Mol Sci. (2020) 21:1–27. doi: 10.3390/ijms21020623
134. Levi M, Van Der Poll T, Schultz M. Infection and inflammation as risk factors for thrombosis and atherosclerosis. Semin Thromb Hemost. (2012) 38:506–14. doi: 10.1055/s-0032-1305782
135. Campello E, Zabeo E, Radu CM, Spiezia L, Gavasso S, Fadin M, et al. Hypercoagulability in overweight and obese subjects who are asymptomatic for thrombotic events. Thromb Haemost. (2015) 113:85–96. doi: 10.1160/TH14-02-0156
136. Witkowski M, Landmesser U, Rauch U. Tissue factor as a link between inflammation and coagulation. Trends Cardiovasc Med. (2016) 26:297–303. doi: 10.1016/j.tcm.2015.12.001
137. Vilahur G, Ben-Aicha S, Badimon L. New insights into the role of adipose tissue in thrombosis. Cardiovasc Res. (2017) 113:1046–54. doi: 10.1093/cvr/cvx086
138. Beavers CJ, Heron P, Smyth SS, Bain JA, Macaulay TE. Obesity and antiplatelets-does one size fit all? Thromb Res. (2015) 136:712–6. doi: 10.1016/j.thromres.2015.07.015
139. Van der Meijden PEJ, Heemskerk JWM. Platelet biology and functions: new concepts and clinical perspectives. Nat. Rev. Cardiol. (2018) 16:166–79. doi: 10.1038/s41569-018-0110-0
140. Vardhana SA, Wolchok JD. The many faces of the anti-COVID immune response. J Exp Med. (2020) 217:e20200678. doi: 10.1084/jem.20200678
141. Barrachina MN, Sueiro AM, Izquierdo I, Hermida-Nogueira L, Guitián E, Casanueva FF, et al. GPVI surface expression and signalling pathway activation are increased in platelets from obese patients: elucidating potential anti-atherothrombotic targets in obesity. Atherosclerosis. (2019) 281:62–70. doi: 10.1016/j.atherosclerosis.2018.12.023
142. De Pergola G, De Mitrio V, Giorgino F, Sciaraffia M, Minenna A, Bari LDi, et al. Increase in both pro-thrombotic and anti-thrombotic factors in obese premenopausal women: relationship with body fat distribution. Int J Obes. (1997) 21:527–35. doi: 10.1038/sj.ijo.0800435
143. Skurk T, Hauner H. Obesity and impaired fibrinolysis: role of adipose production of plasminogen activator inhibitor-1. Int J Obes. (2004) 28:1357–64. doi: 10.1038/sj.ijo.0802778
144. Alessi M-C, Poggi M, Juhan-Vague I. Plasminogen activator inhibitor-1, adipose tissue and insulin resistance. Curr Opin Lipidol. (2007) 18:240–5. doi: 10.1097/MOL.0b013e32814e6d29
145. Raiko JRH, Oikonen M, Wendelin-Saarenhovi M, Siitonen N, Kähönen M, Lehtimäki T, et al. Plasminogen activator inhitor-1 associates with cardiovascular risk factors in healthy young adults in the Cardiovascular Risk in Young Finns Study. Atherosclerosis. (2012) 224:208–12. doi: 10.1016/j.atherosclerosis.2012.06.062
146. Liumbruno GM, Franchini M. Beyond immunohaematology: the role of the ABO blood group in human diseases. Blood Transfus. (2013) 11:491–9. doi: 10.2450/2013.0152-13
147. Ellinghaus D, Degenhardt F, Bujanda L, Buti M, Albillos A, Invernizzi P, et al. Genomewide association study of severe Covid-19 with respiratory failure. N Engl J Med. (2020). doi: 10.1056/NEJMoa2020283. [Epub ahead of print].
148. Zhao J, Yang Y, Huang H, Li D, Gu D, Lu X, et al. Relationship between the ABO blood group and the COVID-19 susceptibility. medRxiv [Preprint]. (2020). doi: 10.1101/2020.03.11.20031096
149. Zietz M, Tatonetti NP. (2020). Testing the association between blood type and COVID-19 infection, intubation, and death. medRxiv [Preprint]. doi: 10.1101/2020.04.08.20058073
150. Franchini M, Mannucci PM. ABO blood group and thrombotic vascular disease. Thromb. Haemost. (2014) 112:1103–9. doi: 10.1160/TH14-05-0457
151. Song J, Chen F, Campos M, Bolgiano D, Houck K. Quantitative Influence of ABO Blood Groups on Factor VIII and Its Ratio to von Willebrand Factor, Novel Observations from an ARIC Study of 11, 673 Subjects. PLoS ONE. (2016) 10:e0132626. doi: 10.1371/journal.pone.0132626
152. Klok FA, Kruip MJHA, van der Meer NJM, Arbous MS, Gommers DAMPJ, Kant KM, et al. Incidence of thrombotic complications in critically ill ICU patients with COVID-19. Thromb. Res. (2020) 191:145–7. doi: 10.1016/j.thromres.2020.04.013
153. Katz JN, Kolappa KP, Becker RC. Beyond thrombosis: the versatile platelet in critical illness. Chest. (2011) 139:658–68. doi: 10.1378/chest.10-1971
154. Frantzeskaki F, Armaganidis A, Orfanos SE. Immunothrombosis in acute respiratory distress syndrome: cross talks between inflammation and coagulation. Respiration. (2017) 93:212–25. doi: 10.1159/000453002
155. Chen G, Zhao J, Ning Q, Chen G, Wu D, Guo W, et al. Clinical and immunological features of severe and moderate coronavirus disease 2019. J Clin Invest. (2020) 130:2620–9. doi: 10.1172/JCI137244
156. Rao S, Lau A, So H-C. Exploring diseases / traits and blood proteins causally related to expression of ACE2, the putative receptor of SARS-CoV-2 : a mendelian randomization analysis highlights tentative relevance of diabetes-related traits. Diabetes Care. (2020) 43:1–11. doi: 10.2337/dc20-0643
157. Iba T, Levy JH, Levi M, Thachil J. Coagulopathy in COVID-19. J Thromb Haemost. (2020). doi: 10.1111/jth.14975. [Epub ahead of print].
158. Ranucci M, Ballotta A, Di Dedda U, Bayshnikova E, Dei Poli M, Resta M, et al. The procoagulant pattern of patients with COVID-19 acute respiratory distress syndrome. J. Thromb. Haemost. (2020). 18:1747–51. doi: 10.1111/jth.14854
159. Qu R, Ling Y, Zhang Y-H-Z, Wei L-Y, Chen X, Li X-M, et al. Platelet-to-lymphocyte ratio is associated with prognosis in patients with CoronaVirus disease-19. J Med Virol. (2020). doi: 10.1002/jmv.25767. [Epub ahead of print].
160. CDC. People Who Are at Higher Risk for Severe Illness. Centers Disease Control Prevention (2020) p. 4–7.
161. World Obesity Federation. Coronavirus (COVID-19) & Obesity. World Obesity Federation (2020). p. 5–7.
162. Brown JD. Antihypertensive drugs and risk of COVID-19? Lancet Respir Med. (2020) 8:e28. doi: 10.1016/S2213-2600(20)30158-2
163. Meng J, Xiao G, Zhang J, He X, Ou M, Bi J, et al. Renin-angiotensin system inhibitors improve the clinical outcomes of COVID-19 patients with hypertension. Emerg Microbes Infect. (2020) 9:757–60. doi: 10.1080/22221751.2020.1746200
164. Russell B, Moss C, Rigg A, Van Hemelrijck M. COVID-19 and treatment with NSAIDs and corticosteroids: should we be limiting their use in the clinical setting? Ecancermedicalscience. (2020) 14:1–3. doi: 10.3332/ecancer.2020.1023
165. Sanders JM, Monogue ML, Jodlowski TZ, Cutrell JB. Pharmacologic treatments for coronavirus disease 2019 (COVID-19): a review. JAMA. (2020). 323:1824–36. doi: 10.1001/jama.2020.6019
166. Mancuso P. Obesity and lung inflammation. J Appl Physiol. (2010) 108:722–8. doi: 10.1152/japplphysiol.00781.2009
167. Jose RJ, Manuel A. COVID-19 cytokine storm: the interplay between inflammation and coagulation. Lancet Respir. (2019) 2019:2019–20. doi: 10.1016/S2213-26002030216-2
168. Baigent C, Bhala N, Emberson J, Merhi A, Abramson S, Arber N, et al. Vascular and upper gastrointestinal effects of non-steroidal anti-inflammatory drugs: meta-analyses of individual participant data from randomised trials. Lancet. (2013) 382:769–79. doi: 10.1016/S0140-67361360900-9
169. Little P, Moore M, Kelly J, Williamson I, Leydon G, McDermott L, et al. Ibuprofen, paracetamol, and steam for patients with respiratory tract infections in primary care: pragmatic randomised factorial trial. BMJ. (2013) 347:f6041. doi: 10.1136/bmj.f6041
170. Little P. Non-steroidal anti-inflammatory drugs and covid-19. BMJ. (2020) 368:1–2. doi: 10.1136/bmj.m1185
171. Hui DS. Systemic corticosteroid therapy may delay viral clearance in patients with middle east respiratory syndrome coronavirus infection. Am J Respir Crit Care Med. (2018) 197:700–1. doi: 10.1164/rccm.201712-2371ED
172. Ni YN, Chen G, Sun J, Liang BM, Liang ZA. The effect of corticosteroids on mortality of patients with influenza pneumonia: a systematic review and meta-analysis. Crit Care. (2019) 23:1–9. doi: 10.1186/s13054-019-2395-8
173. Halpin DMG, Singh D, Hadfield RM. Inhaled corticosteroids and COVID-19: a systematic review and clinical perspective. Eur Respir J. (2020) 55:2001009. doi: 10.1183/13993003.01009-2020
174. Zha L, Li S, Pan L, Tefsen B, Li Y, French N, et al. Corticosteroid treatment of patients with coronavirus disease 2019 (COVID-19). Med J Aust. (2020) 2019:1–5. doi: 10.5694/mja2.50577
175. Matsuyama S, Kawase M, Nao N, Shirato K, Ujike M, Kamitani W, et al. The inhaled corticosteroid ciclesonide blocks coronavirus RNA replication by targeting viral NSP15. bioRxiv [Preprint]. (2020). doi: 10.1101/2020.03.11.987016
176. Peters MC, Sajuthi S, Deford P, Christenson S, Rios CL, Montgomery MT, et al. COVID-19 related genes in sputum cells in asthma: relationship to demographic features and corticosteroids. Am J Respir Crit Care Med. (2020) 202:83–90. doi: 10.1164/rccm.202003-0821OC
177. Liu B, Li M, Zhou Z, Guan X, Xiang Y. Can we use interleukin-6 (IL-6) blockade for coronavirus disease 2019 (COVID-19)-induced cytokine release syndrome (CRS)? J. Autoimmun. (2020) 111:102452. doi: 10.1016/j.jaut.2020.102452
178. Roumier M, Paule R, Groh M, Vallee A, Ackermann F. Interleukin-6 blockade for severe COVID-19. Br Med J. (2020). doi: 10.1101/2020.04.20.20061861. [Epub ahead of print].
179. NCT04275414. Bevacizumab in Severe or Critical Patients With COVID-19 Pneumonia (BEST-CP). ClinicalTrials.gov (2020). p. 1–9. doi: 10.31525/ct1-nct04275414
180. NCT04315298. Evaluation of the Efficacy and Safety of Sarilumab in Hospitalized Patients With COVID-19. (2020) p. 1–9. Available online at: https://clinicaltrials.gov/show/NCT04315298
181. Feldmann M, Maini RN, Woody JN, Holgate ST, Winter G, Rowland M, et al. Trials of anti-tumour necrosis factor therapy for COVID-19 are urgently needed. Lancet. (2020) 395:1407–9. doi: 10.1016/S0140-67362030858-8
182. Haga S, Yamamoto N, Nakai-Murakami C, Osawa Y, Tokunaga K, Sata T, et al. Modulation of TNF-α-converting enzyme by the spike protein of SARS-CoV and ACE2 induces TNF-α production and facilitates viral entry. Proc Natl Acad Sci USA. (2008) 105:7809–14. doi: 10.1073/pnas.0711241105
183. Hussell T, Pennycook A, Openshaw PJ. Inhibition of tumor necrosis factor reduces the severity of virus-specific lung immunopathology. Eur J Immunol. (2001) 31:2566–73. doi: 10.1002/1521-4141(200109)31:9<2566::aid-immu2566>3.0.co;2-l
184. Cinatl J, Morgenstern B, Bauer G, Chandra P, Rabenau H, Doerr HW. Treatment of SARS with human interferons. Lancet. (2003) 362:293–4. doi: 10.1016/S0140-67360314482-0
185. Hensley LE, Fritz EA, Jahrling PB, Karp CL, Huggins JW, Geisbert TW. Interferon- β 1a and SARS coronavirus replication. Emerg Infect Dis. (2004) 10:317–9. doi: 10.3201/eid1002.030482
186. Sallard E, Lescure F, Yazdanpanah Y, Mentre F, Pei N. (2020). Type 1 interferons as a potential treatment against COVID-19. Antiviral Res. 178:104791. doi: 10.1016/j.antiviral.2020.104791
187. Grillet F, Behr J, Calame P, Aubry S, Delabrousse E. Acute pulmonary embolism associated with COVID-19 pneumonia detected by pulmonary CT angiography. Radiology. (2020). doi: 10.1148/radiol.2020201544
188. Lee A, Connors JM, Kreuziger LB, Murphy M, Gernsheimer T, Lin Y. COVID-19 and coagualopathy: frequently asked questions. Am Soc Hematol. (2020) 1–2. Retrieved from: https://www.hematology.org/covid-19/covid-19-and-coagulopathy
189. Xie Y, Wang X, Yang P, Zhang S. COVID-19 complicated by acute pulmonary embolism. Radiol Cardiothorac Imaging. (2020) 2:e200067. doi: 10.1148/ryct.2020200067
190. Alikhan R, Forster R, At C. Heparin for the prevention of venous thromboembolism in acutely ill medical patients (excluding stroke and myocardial infarction). Cochrane Database Syst Rev. (2014) 2014:CD003747. doi: 10.1002/14651858.CD003747.pub4
191. Mousavi S, Moradi M, Khorshidahmad T, Motamedi M. Anti-inflammatory effects of heparin and its derivatives: a systematic review. Adv Pharmacol Sci. (2015) 2015:507151. doi: 10.1155/2015/507151
192. Savioli F. Is there a rationale for heparin use among severe COVID-19 patients? Einstein. (2020) 18:eED5758. doi: 10.31744/einstein_journal/2020ed5758
193. Tang N, Bai H, Chen X, Gong J, Li D, Sun Z. Anticoagulant treatment is associated with decreased mortality in severe coronavirus disease 2019 patients with coagulopathy. J Thromb Haemost. (2020) 18:1094–9. doi: 10.1111/jth.14817
194. Mycroft-west CJ, Su D, Pagani I, Rudd TR, Elli S. Heparin inhibits cellular invasion by SARS-CoV-2: structural dependence of the interaction of the surface protein (spike) S1 receptor binding domain with heparin. Immunol Netw. (2020). 1–22. doi: 10.1101/2020.04.28.066761
195. Fang L, Karakiulakis G, Roth M. Are patients with hypertension and diabetes mellitus at increased risk for COVID-19 infection? Lancet Respir. (2020) 8:e21. doi: 10.1016/S2213-2600(20)30116-8
196. Mcmurray JJV, Pfeffer MA, Solomon SD. Renin – angiotensin – aldosterone system inhibitors in patients with Covid-19. N Engl J Med. (2020) 382:1653–9. doi: 10.1056/NEJMsr2005760
197. Rossi GP, Sanga V, Barton M. Potential harmful effects of discontinuing ACE-inhibitors and ARBs in COVID-19 patients. Elife. (2020) 9:1–8. doi: 10.7554/eLife.57278
198. Zhang P, Zhu L, Cai J, Lei F, Qin J-J, Xie J, et al. Association of inpatient use of angiotensin-converting enzyme inhibitors and angiotensin II receptor blockers with mortality among patients with hypertension hospitalized with COVID-19. Circ Res. (2020) 126:1671–81. doi: 10.1161/CIRCRESAHA.120.317134
199. Rodrigues Prestes TR, Rocha NP, Miranda AS, Teixeira AL, Simoes-E-Silva AC. The anti-inflammatory potential of receptor axis : evidence from basic and clinical research. Curr Drug Targets. (2017) 18:1301–13. doi: 10.2174/1389450117666160727142401
Keywords: adipose tissue, COVID-19, Obesity, SARS-CoV-2, hypercoagulopathy, ACE-2
Citation: Pasquarelli-do-Nascimento G, Braz-de-Melo HA, Faria SS, Santos IO, Kobinger GP and Magalhães KG (2020) Hypercoagulopathy and Adipose Tissue Exacerbated Inflammation May Explain Higher Mortality in COVID-19 Patients With Obesity. Front. Endocrinol. 11:530. doi: 10.3389/fendo.2020.00530
Received: 03 June 2020; Accepted: 30 June 2020;
Published: 28 July 2020.
Edited by:
Jeff M. P. Holly, University of Bristol, United KingdomReviewed by:
Luca Spiezia, University of Padova, ItalyErik Albert Karlsson, Institut Pasteur du Cambodge, Cambodia
Copyright © 2020 Pasquarelli-do-Nascimento, Braz-de-Melo, Faria, Santos, Kobinger and Magalhães. This is an open-access article distributed under the terms of the Creative Commons Attribution License (CC BY). The use, distribution or reproduction in other forums is permitted, provided the original author(s) and the copyright owner(s) are credited and that the original publication in this journal is cited, in accordance with accepted academic practice. No use, distribution or reproduction is permitted which does not comply with these terms.
*Correspondence: Kelly Grace Magalhães, a2VsbHltYWdhbGhhZXMmI3gwMDA0MDt1bmIuYnI=