- 1Department of Physiology, University of Toronto, Toronto, ON, Canada
- 2Department of Medicine, University of Toronto, Toronto, ON, Canada
- 3Department of Obstetrics and Gynaecology, University of Toronto, Toronto, ON, Canada
Obesity is a prominent metabolic disease that predisposes individuals to multiple comorbidities, including type 2 diabetes mellitus, cardiovascular diseases, and cancer. Elevated circulating levels of fatty acids contribute to the development of obesity, in part, by targeting the hypothalamus. Palmitate, the most abundant circulating saturated fatty acid, has been demonstrated to dysregulate NAMPT and circadian clock proteins, as well as induce neuroinflammation. These effects ultimately result in hypothalamic dysregulation of feeding behavior and energy homeostasis. NAMPT is the rate-limiting enzyme of the NAD+ salvage pathway and its expression is under the control of the circadian clock. NAD+ produced from NAMPT can modulate the circadian clock, demonstrating bidirectional interactions between circadian and metabolic pathways. Using NPY/AgRP-expressing mHypoE-46 neurons as well as the novel mHypoA-BMAL1-WT/F and mHypoA-BMAL1-KO/F cell lines, we studied whether there were any interactions between NAMPT and the core circadian clock protein BMAL1 in the palmitate-mediated induction of neuroinflammation. We report that palmitate altered Nampt, Bmal1, Per2 and the inflammatory genes Nf-κb, IκBα, Il-6, and Tlr4. Contrary to studies performed with peripheral tissues, the palmitate-mediated induction in Nampt was independent of BMAL1, and basal Nampt levels did not appear to exhibit rhythmic expression. Palmitate-induced downregulation of Bmal1 and Per2 was independent of NAMPT. However, NAMPT and BMAL1 were both involved in the regulation of Nf-κb, IκBα, Il-6, and Tlr4, as NAMPT inhibition resulted in the repression of basal Nf-κb and IκBα and normalized palmitate-mediated increases in Il-6, and Tlr4. On the other hand, BMAL1 deletion repressed basal Nf-κb, but increased basal Il-6. We conclude that NAMPT and BMAL1 do not interact at the transcriptional level in hypothalamic neurons, but are independently involved in the expression of inflammatory genes.
Introduction
Obesity predisposes individuals to comorbidities, such as type 2 diabetes mellitus (T2DM), cardiovascular diseases, and cancer (1). Increased consumption of fat is a main contributing factor to obesity (2), leading to elevated levels of saturated fatty acids (SFA) both in serum (3) and the hypothalamus (4). The arcuate nucleus of the hypothalamus contains critical orexigenic neuropeptide Y/agouti-related peptide (NPY/AgRP) and anorexigenic proopiomelanocortin (POMC) neurons. These neurons sense nutrients, such as fatty acids and peripheral hormones as signals of energy levels and release their respective neuropeptides to regulate energy homeostasis. Elevated SFA in the hypothalamus results in deleterious effects marked by the induction of neuroinflammation, oxidative stress, endoplasmic reticulum (ER) stress, and circadian disruption, all of which can lead to hormonal resistance and neuropeptide dysregulation (5–12).
Cells of the body, including hypothalamic neurons, possess an intrinsic clock machinery that generates a ~24-h circadian rhythm. Several core proteins are involved in the circadian machinery: circadian locomotor output cycles kaput (CLOCK) and brain and muscle ARNT-like 1 (BMAL1) are transcription factors that heterodimerize to bind to E-box elements, inducing the expression of genes encoding period (PER) and cryptochrome (CRY). PER and CRY are transcription factors that heterodimerize to repress CLOCK and BMAL1 expression, completing a transcriptional-translational feedback loop. Animal studies have shown that shift work induces circadian dysregulation and is associated with an increased risk for weight gain and obesity (13–15). Similar effects were also observed in shift workers (16, 17). Additionally, saturated fats such as palmitate can alter the expression and rhythmicity of BMAL1, CLOCK, and PER2 to alter feeding peptide expression (6).
NAD+ is involved in intracellular redox reactions and is a cofactor for enzymes, such as the deacetylase Sirtuin 1 (SIRT1) and the DNA repair poly enzyme poly ADP-ribose polymerase (PARP). These enzymes consume NAD+ to generate nicotinamide (NAM) as a by-product. The multistep conversion of NAM back to NAD+ is regulated by the rate-limiting enzyme nicotinamide phosphoribosyltransferase (NAMPT), also known as pre-B cell enhancing factor (PBEF) or visfatin. Importantly, the 5′ regulatory region of the Nampt gene contains E-box elements, which bind BMAL1:CLOCK heterodimers to drive expression. Critically, NAMPT mRNA and protein expression is decreased in the adipose tissue of HFD-fed mice (18, 19). Moreover, NAMPT expression is modulated by palmitate in the heart and the liver (20).
The hypothalamus is susceptible to developing neuroinflammation following the onset of HFD-feeding that precedes peripheral inflammation and weight gain (10, 21, 22). Hypothalamic neuroinflammation involves the secretion of inflammatory cytokines and reactive gliosis (9–11, 23). Critically, inflammation contributes to the disruption of energy homeostasis by altering feeding neuropeptide expression and the development of resistance to key anorexigenic hormones, such as leptin and insulin (24, 25). Many processes are implicated in the development of inflammation, such as the activation of toll-like receptor 4 (TLR4) or the intracellular metabolism of fats, both of which result in the activation of inflammatory NF-κB and MAPK signaling. NAMPT and BMAL1 may play also role in palmitate-induced inflammation as SIRT1 can target and modulate NF-κB activity (26), while BMAL1 has been demonstrated regulate expression of the inflammatory cytokine interleukin 6 (Il-6) (27, 28). However, whether the interaction between NAMPT or BMAL1 is involved in SFA-induced hypothalamic neuroinflammation remains unclear.
In this study, we investigated (1) whether the saturated fatty acid palmitate alters Nampt, Bmal1, and Per2 expression in hypothalamic neurons, (2) the temporal sequence and mediators between the palmitate-induced modulations of Nampt, Bmal1, and Per2 mRNAs, and (3) whether palmitate-induced neuroinflammation is mediated by either NAMPT or BMAL1. We used primary hypothalamic cultures and immortalized hypothalamic cell lines, including the NPY/AgRP-expressing clonal mHypoE-46 cell line, as well as the novel heterogenous mHypoA-BMAL1-WT/F and mHypoA-BMAL1-KO/F cell lines. We report that palmitate upregulates Nampt in hypothalamic neurons in contrary to reports in peripheral tissues. Both basal Nampt expression and the induction of Nampt by palmitate appear to be BMAL1-independent, and palmitate-mediated changes to Bmal1 and Per2 expression are independent of NAMPT. Thus, both NAMPT and BMAL1 appear to be independently involved in alterations to inflammatory Nf-κb, IκBα, Il-6, and Tlr4 gene expression in response to palmitate.
Methods
Cell Culture and Reagents
Clonal mHypoE-46, and the heterogenous mHypoA-BMAL1-WT/F and mHypoA-BMAL1-KO/F cell lines were generated in our laboratory as previously described (29, 30). The mHypoA-BMAL1-KO/F cell line has been validated to lack BMAL1 protein, Bmal1 mRNA, and clock rhythmicity (30). Cells were grown in low glucose Dulbecco's Modified Eagle Medium (DMEM) (MilliporeSigma; Etobicoke, ON, CA) containing 5.5 mM glucose, 2% fetal bovine serum (FBS) (Gibco, Burlington, ON, CA) and 1% penicillin-streptomycin (Gibco).
For primary culture experiments, whole hypothalamii from 8-week old male or female CD-1 mice (Charles River Laboratories, Senneville, QC, Canada) were extracted. Hypothalamii were gently triturated to achieve dispersion and cultured in 6-well plates for 7–9 days in neurobasal A medium (Gibco), supplemented with 10% FBS, 5% horse serum (Gibco), 1% PS, 1 × B27 serum-free supplement (Gibco) and 1 × GlutaMAX supplement (Gibco). 10 ng/μL CNTF was added to each well every day. After 7–9 days, cells were treated with 50 μM palmitate (MilliporeSigma) in low glucose DMEM (MilliporeSigma).
All animal procedures were approved by and performed in accordance with the Animal Use Protocols at the University of Toronto.
In vitro Experimental Protocols
Sodium palmitate and FK866 were purchased from MilliporeSigma. Sodium palmitate was dissolved in molecular grade water by heating to 70°C to obtain a concentration of 50 mM. FK866 was dissolved in dimethyl sulfoxide (DMSO) (Thermo Scientific) to 10 μM. Treatments were diluted 1:1,000 in low glucose DMEM to reach the final concentrations as indicated in the results section. Cells were grown to to 75–80% confluency on 60 mm plates prior to treatment.
For time course experiments, mHypoA-BMAL1-WT/F and mHypoA-BMAL1-KO/F were grown to 75–80% confluency on 60 mm plates, serum-starved for 12 h and synchronized for 30 min with 20 μM forskolin (Thermo Scientific) in DMEM containing 5% FBS. This was then replaced with fresh DMEM containing 5% FBS, indicating t = 0 h. Cells were first harvested at 6 h, then afterwards at every 6-h interval up to 36 h. The samples used to assess Nampt rhythmicity were the same as those used in the study by Clemenzi et al. (31). With these studies, circadian oscillations of the core clock genes were demonstrated in the mHypoA-BMAL1-WT/F cells, and these rhythms were completely absent in the mHypoA-BMAL1-KO/F cell line.
Quantitative Real-Time PCR (qRT-PCR)
Total mRNA was obtained using the PureLink RNA Mini Kit with on-column PureLink DNase (Ambion; Streetsville, ON, CAN) and quantified using the Nanodrop 2000c spectrophotometer. 1 μg of harvested RNA was used for cDNA synthesis with the High-Capacity cDNA Reverse Transcription Kit (Applied Biosystems). qRT-PCR was performed using gene specific primers (Table 1) and qRT-PCR was performed using gene specific primers and the Platinum SYBR Green qPCR SuperMix-UDG with ROX (Applied Biosystems) with the Applied Biosystems 7900 HT Real-Time PCR machine, then analyzed using SDS 2.4 software (Applied Biosystems). Relative mRNA quantities were determined using the ΔΔCt method or with the standard curve method and normalized to the reference gene Rpl7.
Statistical Analysis
Results were analyzed using GraphPad Prism Software 6.0 (GraphPad Software; La Jolla, CA, USA) and presented as mean ± S.E.M. Data from each figure were analyzed using t-test or two-way ANOVA, followed by the Tukey or Sidak post hoc test. Circadian rhythmicity was determined using JTK Cycle to analyze the fit to a 24 h period (32). Statistical significance is denoted as *P < 0.05, **P < 0.01, ***P < 0.001, ****P < 0.0001.
Results
Palmitate Alters Nampt, Bmal1, and Per2 mRNA Expression in Hypothalamic Neurons
Due to reports of altered Nampt mRNA in the periphery of DIO animals (18, 33), we investigated whether the same held true for hypothalamic neurons. Thus, we treated the immortalized mHypoE-46 cell line with 50 μM palmitate for 24 h and observed that palmitate upregulated Nampt (Figure 1A). We further validated our results in primary hypothalamic cultures derived from male and female CD-1 mice, observing a similar induction of Nampt with 50 μM palmitate treatment for 24 h (Figure 1A). Next, as both HFD and palmitate treatment have been shown to alter clock gene expression (6, 34), we were interested whether palmitate would also alter clock gene expression in mHypoE-46 neurons at the same time as Nampt. We observed that a 24 h treatment with 50 μM palmitate repressed both Bmal1 and Per2 mRNA (Figure 1B). As palmitate also altered inflammatory gene expression in hypothalamic neurons (10, 11), we then validated these findings in the mHypoE-46 model. Palmitate repressed Nf-κb and Iκbα while elevating Il-6 and Tlr4 mRNA (Figure 1C).
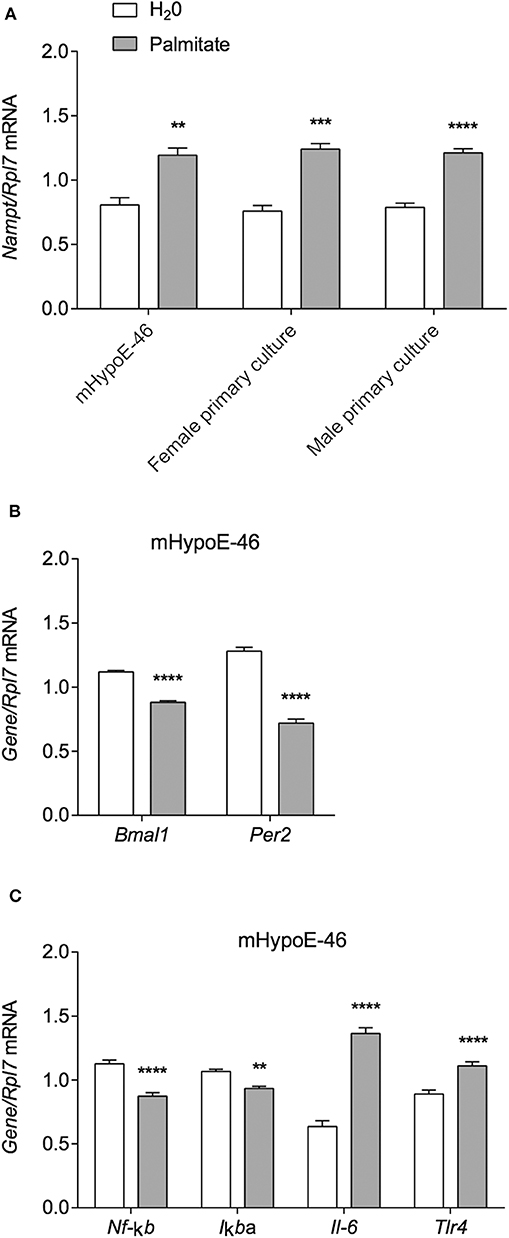
Figure 1. Palmitate alters expression of Nampt, core circadian clock genes, and inflammatory genes. mHypoE-46 neurons, as well as primary hypothalamic cultures derived from male and female CD-1 mice were treated with 50 μM palmitate for 24 h. Changes in mRNA expression of (A) Nampt, (B) Bmal1 and Per2, or (C) Nf-κb, IκBα, Il-6, and Tlr4 were assessed by qRT-PCR. n = 4 independent experiments. Data are expressed as mean ± SEM; **P < 0.01, ***P < 0.001, ****P < 0.0001 Statistical analysis was performed with an unpaired t-test.
Nampt mRNA Expression Is Not Under the Control of BMAL1
Palmitate was previously shown to alter the expression of the core molecular clock genes Bmal1, Clock, and Per2 in hypothalamic neurons over a 36 h time course (6), and interactions had been shown between core clock proteins and NAMPT expression. In mouse liver, Nampt mRNA and protein are rhythmically expressed (35). Furthermore, Nampt mRNA also demonstrated rhythmic expression in mouse embryonic fibroblasts (MEF), and the maintenance of Nampt expression required CLOCK as it was abolished in MEF-specific CLOCK knockouts (36). Accordingly, we sought to investigate the involvement of BMAL1 in palmitate-mediated induction of Nampt. We used the heterogeneous mHypoA-BMAL1-KO/F and mHypoA-BMAL1-WT/F cell lines, derived from female BMAL1-KO mice and BMAL1-WT female littermates, respectively. Using the same treatment paradigm as previously described, we observed that Nampt was not significantly induced by 24-h treatment of 50 μM palmitate in both mHypoA-BMAL1-KO/F and mHypoA-BMAL1-WT/F cell lines, although there was a trend toward increased expression in both cell lines (Figure 2A). We then questioned whether BMAL1 was required to impart rhythmic Nampt expression in hypothalamic neurons. mHypoA-BMAL1-KO/F and mHypoA-BMAL1-WT/F cell lines were serum-starved for 12 h then synchronized with 20 μM forskolin for 30 min, after which fresh DMEM was applied indicating t = 0 h. Nampt mRNA levels were not rhythmic over 24 h in mHypoA-BMAL1-WT/F (P = 0.41) nor mHypoA-BMAL1-KO/F cells (P = 1), and furthermore, basal Nampt mRNA levels were not significantly different between the two cell lines at any time point (Figure 2B). This was despite our previous finding that mHypoA-BMAL1-WT/F, but not mHypoA-BMAL1-KO/F cells, demonstrated rhythmic expression of Bmal1, Per2, Agrp, and Pomc mRNA (31).
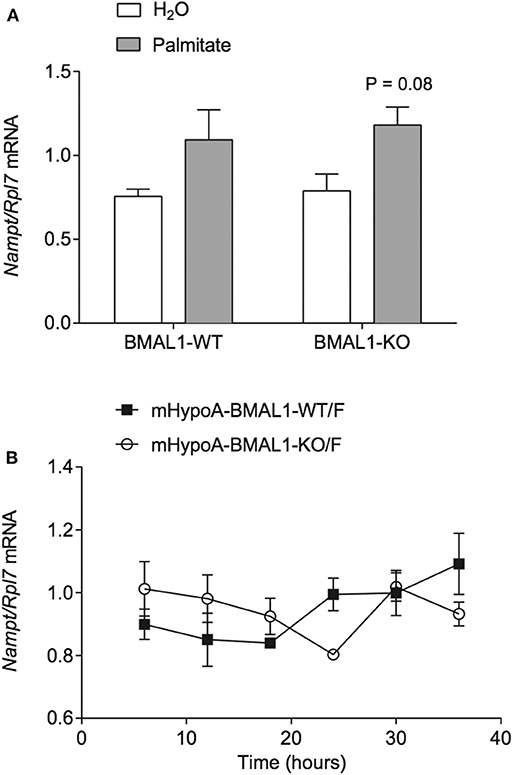
Figure 2. BMAL1 does not mediate the induction of Nampt by palmitate nor rhythmic NAMPT expression. (A) mHypoA-BMAL1-WT/F and mHypoA-BMAL1-KO/F cells were treated with 50 μM palmitate for 24 h and assessed for changes in Nampt mRNA. (B) mHypoA-BMAL1-WT/F and mHypoA-BMAL1-WT/F-BMAL1-KO/F cells were serum-starved for 12 h and synchronized for 30 min with 20 μM forskolin in DMEM containing 5% FBS. Fresh media containing 5% FBS was then applied, indicating t = 0 h. Cells were first harvested at 6 h, then every 6 h until 36 h. Nampt mRNA levels were assessed by qRT-PCR. n = 4 independent experiments. Data are expressed as mean ± SEM. Statistical analysis was performed by two-way ANOVA with the Tukey post hoc test.
Intracellular NAMPT Activity Does Not Mediate the Changes in Bmal1 and Per2 mRNA by Palmitate
Since NAMPT is able to regulate the circadian clock by the targeting BMAL1:CLOCK via NAD+ and SIRT1 (6, 35–40), we hypothesized that increased Nampt mRNA expression and thereby NAMPT and NAD+ production, would be responsible for the repression of Bmal1 and Per2 by palmitate. To block any potential increase in NAMPT enzymatic activity associated with the palmitate-mediated induction of Nampt mRNA, we treated mHypoE-46 neurons with 10 nM of specific NAMPT inhibitor inhibitor FK866. 24- and 48-h treatment with FK866 was effective at lowering NAMPT activity and NAD+ levels in hepatocarcinoma cells, hepatocytes, and neuroblastoma cells. We pre-treated with 10 nM FK866 for 1 h, followed by 24-h treatment with 50 μM palmitate. However, palmitate was unable to significantly repress Bmal1 mRNA in the presence of DMSO (as opposed to Figure 1B where DMSO was not present). Moreover, FK866 failed to attenuate the repression of Bmal1 and Per2 by palmitate (Figure 3A). As a positive control, we observed that FK866 elevated basal Nampt mRNA expression, but it failed to normalize the increase of Nampt by palmitate (Figure 3B).
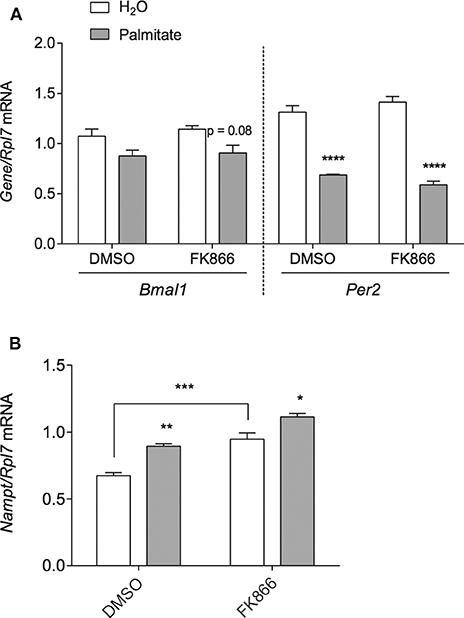
Figure 3. NAMPT activity does not mediate the repression of Bmal1 nor Per2 mRNA by palmitate. mHypoE-46 neurons were pretreated for 1 h with 10 nM FK866 or DMSO vehicle control, then treated with 50 μM palmitate for 24 h. Changes in (A) Bmal1 and Per2 mRNA or (B) Nampt mRNA were assessed by qRT-PCR. n = 4 independent experiments. Data are expressed as mean ± SEM, *P < 0.05, **P < 0.01, ***P < 0.001, ****P < 0.0001. Statistical analysis was performed by two-way ANOVA with the Tukey post hoc test.
NAMPT and BMAL1 Are Involved in Inflammatory Gene Expression
To investigate whether the inflammatory effects of palmitate could be attributed to altered NAMPT levels and activity, mHypoE-46 cells pre-treated with 10 nM FK866 for 1 h followed by 50 μM palmitate for 24 h and were assessed for changes in inflammatory Nf-κb, IκBα, Il-6, and Tlr4 mRNA. In the presence of 10 nM FK866, basal Nf-κb and IκBα expression were repressed, but the NAMPT inhibitor had no effect on the palmitate-mediated downregulation of these genes (Figure 4A). The induction of Il-6 and Tlr4 mRNA were blocked by 10 nM FK866 pre-treatment (Figure 4A).
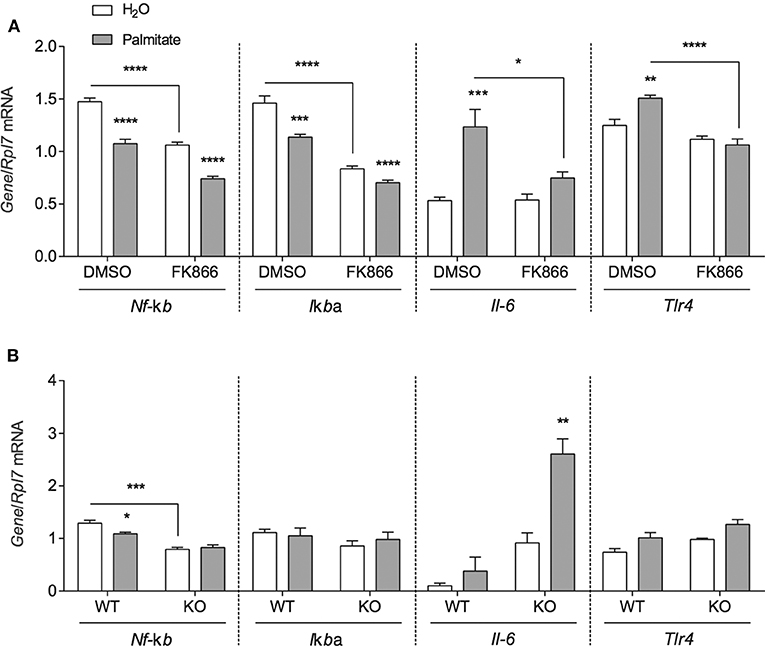
Figure 4. NAMPT and BMAL1 are involved in the induction of inflammatory gene expression by palmitate. (A) mHypoE-46 neurons were pretreated for 1 h with 10 nM FK866 or DMSO vehicle control then treated with 50 μM palmitate for 24 h. (B) mHypoA-BMAL1-WT/F and mHypoA-BMAL1-KO/F cells were treated with 50 μM palmitate for 24 h. Changes in Nf-κb, IκBα, Il-6, and Tlr4 were assessed by qRT-PCR. n = 3–4 independent experiments. Data are expressed as mean ± SEM, *P < 0.05, **P < 0.01, ***P < 0.001, ****P < 0.0001. Statistical analysis was performed by two-way ANOVA with the Tukey post hoc test.
Due to reports that IL-6 production and secretion were regulated by BMAL1 in macrophages and microglia (27, 28), we were interested whether BMAL1 also plays a role in mediating the effects of palmitate on Nf-κb, Iκbα Il-6, and Tlr4. mHypoA-BMAL1-KO/F and mHypoA-BMAL1-WT/F cells were treated with 50 μM palmitate for 24 h. We observed a significant induction in Il-6 mRNA in mHypoA-BMAL1-KO/F cells with palmitate, but not in mHypoA-BMAL1-WT/F cells, suggesting an inhibitory role of BMAL1 on Il-6 expression (Figure 4A). Similar to mHypoE-46 neurons pre-treated with 10 nM FK866, mHypoA-BMAL1-KO/F cells expressed lower basal levels of Nf-κb than in mHypoA-BMAL1-WT/F cells (Figure 4B). Additionally, palmitate repressed Nf-κb mRNA in mHypoA-BMAL1-WT/F cells, but not mHypoA-BMAL1-KO/F cells (Figure 4B). No significant changes were observed for Iκbα nor Tlr4 (Figure 4B).
Discussion
The underlying mechanisms for the pathogenesis of obesity are multifaceted and complex. Disruption of hypothalamic function by elevated saturated fatty acids is implicated in this disease as it affects peptidergic circuits that regulate feeding behavior and whole-body energy homeostasis. Importantly, the ability of saturated fats to alter NAMPT and the circadian clock as well as induce neuroinflammation has been suggested to contribute to hypothalamic dysfunction. However, it is unknown whether these processes are coupled in the hypothalamus. We demonstrate that palmitate-induced alterations of the circadian clock genes Bmal1 and Per2 as well as Nampt occur independently of each other in contrast to what had been observed in peripheral tissues. Despite this, our results suggest a role for NAMPT and BMAL1 in regulating inflammatory gene expression in the hypothalamus.
We report that treatment with the saturated fatty acid palmitate upregulated Nampt mRNA expresion in the mHypoE-46 hypothalamic cell line as well as in male-derived and female-derived primary hypothalamic cultures. A number of studies (18, 33, 41) found that Nampt mRNA and NAMPT protein levels were decreased in the liver of DIO mice. Another study similarly found a decrease in Nampt mRNA and protein expression in adipose tissue in DIO mice (18). A similar downregulation in hypothalamic NAMPT mRNA and protein was also observed following 6 and 8 weeks of HFD-feeding (42). Although the precise molecular mechanisms behind changes in NAMPT expression remain poorly understood, some studies suggest an inhibitory role of inflammatory cytokines on Nampt expression in the periphery. The cytokines IL-6 and tumor necrosis factor alpha (TNF-α) were demonstrated to repress Nampt expression in the adipose tissue of obese mice (43, 44). Similarly, in vitro studies have also demonstrated the repression of Nampt mRNA in adipocytes when stimulated by IL-6 and TNF-α (43, 45, 46). In contrast to a downregulation of Nampt in peripheral tissues, we instead report an induction of hypothalamic Nampt by palmitate. As palmitate is known to induce inflammatory signaling, the induction of Nampt may be driven by activation of the inflammatory transcription factors NF-κB and AP-1 as the respective response elements have been described in the Nampt 5' regulatory region (47, 48). The difference between the acute induction of hypothalamic Nampt in our study vs. downregulation in the hypothalamus following chronic consumption of high fat may be attributed to the temporal dynamics of the neuroinflammatory response. Previous studies have shown an induction of inflammatory markers in the hypothalamus within 24 h of exposure to high fat which temporarily subsides in the following days and weeks (10, 21). However, continued consumption of high fat ensures the return of inflammation to the hypothalamus.
The palmitate-mediated upregulation of Nampt mRNA may also result from the induction of oxidative stress. When cells are exposed to palmitate, reactive oxygen species (ROS) are generated and can damage DNA (49). DNA repair requires the NAD+ dependent enzyme PARP, which is a DNA nick sensor (49, 50). Hyper-activation of PARP following ROS-induced DNA damage can rapidly deplete intracellular NAD+, and the subsequent upregulation of Nampt expression could be a response to restore NAD+ levels similar to the Nampt induction observed with FK866.
Unlike reports in peripheral tissues, our study demonstrates that Nampt mRNA expression does not appear to be rhythmic in hypothalamic neurons. Furthermore, the expression of Nampt mRNA did not appear to require BMAL1 as expression levels were not significantly different between the mHypoA-BMAL1-WT/F and mHypoA-BMAL1-KO/F cell lines. This is in contrast to a study that reported abolished Nampt oscillation in the livers of CLOCK mutant mice (36). Similarly, in a rodent shift-work model that forces activity during the normal resting period, the expression of Nampt was decoupled from circadian clock in the liver, resulting in a loss of rhythmicity (51). Although we did not observe rhythmic Nampt expression, Agrp, Pomc, Bmal1, and Per2 possessed circadian rhythmicity in the same mHypoA-BMAL1-WT/F cells, demonstrating that this cell line possesses a functional circadian clock (31). While this would indicate that Nampt does not appear to have rhythmic expression in hypothalamic neurons, we would suggest some caution with this interpretation as these results are from a set of heterogeneous cell lines. Further experiments will be undertaken using clonal cell lines that have previously been used in the analysis of circadian regulation of neuropeptide expression.
Furthermore, palmitate failed to increase Nampt in mHypoA-BMAL1-WT/F and mHypoA-BMAL1-KO/F cells. However, trends toward increased Nampt were detected in both cell lines with palmitate, suggesting that BMAL1 might not regulate Nampt expression in this model. As we also observed a repression in Bmal1 and Per2 mRNA with palmitate in mHypoE-46 neurons, this suggests overall repression of core circadian clock components, making it unlikely to be involved in the induction of Nampt. A limitation to this interpretation is that it is unknown whether the observed repression is due to changes in the phase or amplitudes of rhythmic Bmal1 and Per2 expression. We have previously reported significant reductions in the amplitudes, but not the phases, of Bmal1 and Per2 mRNA in a similar NPY/AgRP-expressing mHypoE-44 model (6). However, it is still possible that palmitate alters the rhythmic properties of clock genes in mHypoE-46 neurons differently than in mHypoE-44 neurons.
Neuroinflammation is characteristic of obesity and a consequence of HFD-feeding. Specifically, NF-κB signaling is a key contributor to the deleterious effects of palmitate. For example, NF-κB inhibition was able to diminish the palmitate-mediated increases in Npy and inflammatory gene expression (10, 12, 52). Our results demonstrate that NAMPT inhibition with FK866 had an anti-inflammatory effect, ablating the induction of Il-6 and Tlr4 mRNA by palmitate. TLR4 plays an important role in the induction of neuroinflammation by palmitate and signaling downstream of the receptor leads to NF-κB activation via degradation of the inhibitory IκBα subunit. This results in the translocation of NF-κB into the nucleus where it drives the expression of inflammatory genes including Il-6 (53). Furthermore, FK866 also downregulated basal Iκbα and Nf-κb mRNA, but did not normalize the palmitate-mediated downregulation of these genes suggesting that FK866 and palmitate repress Nf-κb and Iκbα expression via independent mechanisms. The FK866-mediated repression of basal Nf-κb and Iκbα may lead to lower protein levels, thus decreasing the ability of palmitate to drive Il-6 and Tlr4 expression through NF-κB signaling (53–56). Together, these findings suggest that FK866 may prevent the induction of inflammatory genes by targeting NF-κB at the mRNA or protein level.
Besides NAMPT, the circadian clock is an established regulator of immune responses in microglia and macrophages (27, 57). Studies have shown that BMAL1 regulates Il-6 expression and secretion (27, 28). Specifically, BMAL1 in monocytes was involved in negatively regulating circulating levels of inflammatory cytokines including CCL2, IL-1β, IL-6 and IFN-γ, and furthermore, BMAL1 deletion resulted in higher expression of Ccl2 and Ccl8 mRNA in monocytes and macrophages (58). Moreover, circadian dysregulation or low BMAL1 expression resulted in higher circulating levels of pro-inflammatory cytokines (59). In agreement with these studies, we saw that Il-6 expression was higher in mHypoA-BMAL1-KO/F cells than in mHypoA-BMAL1-WT/F cells, suggesting a negative role of BMAL1 on Il-6 expression in hypothalamic neurons. Additionally, palmitate did not alter Iκbα, Il-6, nor Tlr4 in mHypoA-BMAL1-WT/F neurons unlike what was observed in mHypoE-46 cells. As the mHypoA-BMAL1-WT/F cell line is heterogeneous compared to the clonal mHypoE-46 cell line, it is possible that not all neuronal subpopulations within the mHypoA-BMAL1-WT/F cell line have the same response to palmitate. For example, the mHypoA-BMAL1-WT/F cell line contains POMC-expressing neurons, which did not have robust inflammatory responses to palmitate at 24 h as Il-6 was not induced (unpublished observation, DDB).
Besides acting as an intracellular enzyme in the NAD+ salvage pathway, NAMPT can also be secreted as a cytokine to extracellular space. Higher levels of extracellular NAMPT, also known as visfatin, are associated with metabolic disorders. A meta-analysis uncovered a positive correlation between circulating NAMPT and parameters of obesity (60). Furthermore, several studies reported considerably higher plasma levels of circulating NAMPT in T2DM patients than in healthy controls, independent of BMI (61–63). Whether NAMPT is secreted from hypothalamic neurons is still unclear. In the CNS, glia but not neurons, may secrete NAMPT as it was observed in primary glial cultures, but not primary mouse cerebral cortex neuronal cultures (64). Regardless, NAMPT has been demonstrated to play an important role in inflammation. TLR4 was recently demonstrated to be a receptor of secreted NAMPT, leading to the induction of NF-κB-mediated inflammation (65). NAMPT-mediated inflammation has been demonstrated in the periphery (65–67). Moreover, NAMPT was also demonstrated to induce inflammatory responses in microglia and the hypothalamus (68, 69). Thus, the inflammatory role of intracellular NAMPT shown herein, or of the secreted form, may dysregulate hypothalamic function.
In this study, we sought to elucidate the complex interplay between circadian dysregulation and neuroinflammation in the context of hypothalamic exposure to palmitate. Our study revealed that palmitate altered mRNA expression levels of Nampt, the circadian clock genes Bmal1 and Per2, as well as the inflammatory genes Iκbα, Nf-κb, Il-6 and Tlr4, in hypothalamic neurons. The changes in Nampt expression did not require BMAL1 and conversely, changes in Bmal1 and Per2 did not require NAMPT; these findings suggest that these two systems do not interact at the mRNA level. We used the NAMPT inhibitor FK866 and a BMAL-1 KO cell line to demonstrate that both NAMPT and BMAL1 were involved in regulating inflammatory gene expression. Furthermore, NAMPT inhibition was able to attenuate palmitate-induced expression of inflammatory genes Il-6 and Tlr4. Taken together, our findings demonstrate that hypothalamic neuroinflammation in response to palmitate involves the circadian clock and metabolism, and that NAMPT inhibition may have therapeutic effects for palmitate-induced hypothalamic neuroinflammation.
Data Availability Statement
The datasets generated for this study are available on request to the corresponding author.
Ethics Statement
All animal procedures were approved by and performed in accordance with the Animal Use Protocols at the University of Toronto.
Author Contributions
AT, WH, NJ, and JC performed the experiments and performed the statistical analysis. AT, WH, NJ, JC, and DB contributed conception and design of the study. AT and WH organized the database. AT, WH, and JC wrote the first draft of the manuscript. DB edited and wrote sections of the manuscript. All authors contributed to manuscript revision, read and approved the submitted version.
Funding
This work was supported by grants from the Canadian Institutes for Health Research (CIHR-MOP-133676), Natural Sciences and Engineering Research Council (NSERC-RGPIN-2018-06144), Canada Foundation for Innovation and Canada Research Chairs Program (DB). JC was supported by NSERC. AT was supported by an Ontario Graduate Scholarship (OGS), and the Banting & Best Diabetes Centre (BBDC).
Conflict of Interest
The authors declare that the research was conducted in the absence of any commercial or financial relationships that could be construed as a potential conflict of interest.
Acknowledgments
We thank the members of the Belsham Lab for inspiration and helpful discussions.
References
1. Pi-Sunyer X. The medical risks of obesity. Postgrad Med. (2009) 121:21–33. doi: 10.3810/pgm.2009.11.2074
3. Gambino R, Bugianesi E, Rosso C, Mezzabotta L, Pinach S, Alemanno N, et al. Different serum free fatty acid profiles in NAFLD subjects and healthy controls after oral fat load. Int J Mol Sci. (2016) 17:479. doi: 10.3390/ijms17040479
4. Posey KA, Clegg DJ, Printz RL, Byun J, Morton GJ, Vivekanandan-Giri A, et al. Hypothalamic proinflammatory lipid accumulation, inflammation, and insulin resistance in rats fed a high-fat diet. Am J Physiol Endocrinol Metabol. (2009) 296:E1003–12. doi: 10.1152/ajpendo.90377.2008
5. Mayer CM, Belsham DD. Palmitate attenuates insulin signaling and induces endoplasmic reticulum stress and apoptosis in hypothalamic neurons: rescue of resistance and apoptosis through adenosine 5' monophosphate-activated protein kinase activation. Endocrinology. (2010) 151:576–85. doi: 10.1210/en.2009-1122
6. Fick LJ, Fick GH, Belsham DD. Palmitate alters the rhythmic expression of molecular clock genes and orexigenic neuropeptide Y mRNA levels within immortalized, hypothalamic neurons. Biochem Biophys Res Commun. (2011) 413:414–9. doi: 10.1016/j.bbrc.2011.08.103
7. Estadella D, Da Penha Oller Do Nascimento CM, Oyama LM, Ribeiro EB, Damaso AR, De Piano A. Lipotoxicity: effects of dietary saturated and transfatty acids. Mediators Inflamm. (2013) 2013:137579. doi: 10.1155/2013/137579
8. Han J, Kaufman RJ. The role of ER stress in lipid metabolism and lipotoxicity. J Lipid Res. (2016) 57:1329–38. doi: 10.1194/jlr.R067595
9. Ye W, Ramos EH, Wong BC, Belsham DD. Beneficial effects of metformin and/or salicylate on palmitate- or TNFalpha-induced neuroinflammatory marker and neuropeptide gene regulation in immortalized NPY/AgRP neurons. PLoS ONE. (2016) 11:e0166973. doi: 10.1371/journal.pone.0166973
10. Dalvi PS, Chalmers JA, Luo V, Han DY, Wellhauser L, Liu Y, et al. High fat induces acute and chronic inflammation in the hypothalamus: effect of high-fat diet, palmitate and TNF-alpha on appetite-regulating NPY neurons. Int J Obes. (2017) 41:149–58. doi: 10.1038/ijo.2016.183
11. Tse EK, Belsham DD. Palmitate induces neuroinflammation, ER stress, and Pomc mRNA expression in hypothalamic mHypoA-POMC/GFP neurons through novel mechanisms that are prevented by oleate. Mol Cell Endocrinol. (2018) 472:40–9. doi: 10.1016/j.mce.2017.11.017
12. Tse EK, Salehi A, Clemenzi MN, Belsham DD. Role of the saturated fatty acid palmitate in the interconnected hypothalamic control of energy homeostasis and biological rhythms. Am J Physiol Endocrinol Metab. (2018) 315:E133–40. doi: 10.1152/ajpendo.00433.2017
13. Di Lorenzo L, De Pergola G, Zocchetti C, L'abbate N, Basso A, Pannacciulli N, et al. Effect of shift work on body mass index: results of a study performed in 319 glucose-tolerant men working in a Southern Italian industry. Int J Obes Relat Metab Disord. (2003) 27:1353–8. doi: 10.1038/sj.ijo.0802419
14. Ha M, Park J. Shiftwork and metabolic risk factors of cardiovascular disease. J Occup Health. (2005) 47:89–95. doi: 10.1539/joh.47.89
15. Sun M, Feng W, Wang F, Li P, Li Z, Li M, et al. Meta-analysis on shift work and risks of specific obesity types. Obes Rev. (2018) 19:28–40. doi: 10.1111/obr.12621
16. Kurose T, Yabe D, Inagaki N. Circadian rhythms and diabetes. J Diabetes Investig. (2011) 2:176–7. doi: 10.1111/j.2040-1124.2011.00105.x
17. James SM, Honn KA, Gaddameedhi S, Van Dongen HPA. Shift work: disrupted circadian rhythms and sleep-implications for health and well-being. Curr Sleep Med Rep. (2017) 3:104–12. doi: 10.1007/s40675-017-0071-6
18. Yoshino J, Mills KF, Yoon MJ, Imai SI. Nicotinamide mononucleotide, a key NAD(+) intermediate, treats the pathophysiology of diet- and age-induced diabetes in mice. Cell Metabolism. (2011) 14:528–36. doi: 10.1016/j.cmet.2011.08.014
19. Chalkiadaki A, Guarente L. High-fat diet triggers inflammation-induced cleavage of SIRT1 in adipose tissue to promote metabolic dysfunction. Cell Metab. (2012) 16:180–8. doi: 10.1016/j.cmet.2012.07.003
20. Penke M, Schuster S, Gorski T, Gebhardt R, Kiess W, Garten A. Oleate ameliorates palmitate-induced reduction of NAMPT activity and NAD levels in primary human hepatocytes and hepatocarcinoma cells. Lipids Health Dis. (2017) 16:191. doi: 10.1186/s12944-017-0583-6
21. Thaler JP, Yi CX, Schur EA, Guyenet SJ, Hwang BH, Dietrich MO, et al. Obesity is associated with hypothalamic injury in rodents and humans. J Clin Invest. (2012) 122:153–62. doi: 10.1172/JCI59660
22. Thaler JP, Guyenet SJ, Dorfman MD, Wisse BE, Schwartz MW. Hypothalamic inflammation: marker or mechanism of obesity pathogenesis? Diabetes. (2013) 62:2629–34. doi: 10.2337/db12-1605
23. Haversen L, Danielsson KN, Fogelstrand L, Wiklund O. Induction of proinflammatory cytokines by long-chain saturated fatty acids in human macrophages. Atherosclerosis. (2009) 202:382–93. doi: 10.1016/j.atherosclerosis.2008.05.033
24. Bence KK, Delibegovic M, Xue B, Gorgun CZ, Hotamisligil GS, Neel BG, et al. Neuronal PTP1B regulates body weight, adiposity and leptin action. Nat Med. (2006) 12:917–24. doi: 10.1038/nm1435
25. Jais A, Bruning JC. Hypothalamic inflammation in obesity and metabolic disease. J Clin Invest. (2017) 127:24–32. doi: 10.1172/JCI88878
26. Kauppinen A, Suuronen T, Ojala J, Kaarniranta K, Salminen A. Antagonistic crosstalk between NF-kappaB and SIRT1 in the regulation of inflammation and metabolic disorders. Cell Signal. (2013) 25:1939–48. doi: 10.1016/j.cellsig.2013.06.007
27. Nakazato R, Hotta S, Yamada D, Kou M, Nakamura S, Takahata Y, et al. The intrinsic microglial clock system regulates interleukin-6 expression. Glia. (2017) 65:198–208. doi: 10.1002/glia.23087
28. Early JO, Menon D, Wyse CA, Cervantes-Silva MP, Zaslona Z, Carroll RG, et al. Circadian clock protein BMAL1 regulates IL-1beta in macrophages via NRF2. Proc Natl Acad Sci USA. (2018) 115:E8460–8. doi: 10.1073/pnas.1800431115
29. Dalvi PS, Nazarians-Armavil A, Tung S, Belsham DD. Immortalized neurons for the study of hypothalamic function. Am J Physiol Regul Integr Comp Physiol. (2011) 300:R1030–52. doi: 10.1152/ajpregu.00649.2010
30. Loganathan N, Salehi A, Chalmers JA, Belsham DD. Bisphenol A Alters Bmal1, Per2, and Rev-Erba mRNA and requires bmal1 to increase neuropeptide Y expression in hypothalamic neurons. Endocrinology. (2019) 160:181–92. doi: 10.1210/en.2018-00881
31. Clemenzi MN, Martchenko A, Loganathan N, Tse EK, Brubaker PL, Belsham DD. Analysis of Western diet, palmitate and BMAL1 regulation of neuropeptide Y expression in the murine hypothalamus and BMAL1 knockout cell models. Mol Cell Endocrinol. (2020) 507:110773. doi: 10.1016/j.mce.2020.110773
32. Hughes ME, Hogenesch JB, Kornacker K. JTK_CYCLE: an efficient nonparametric algorithm for detecting rhythmic components in genome-scale data sets. J Biol Rhythms. (2010) 25:372–80. doi: 10.1177/0748730410379711
33. Choi SE, Fu T, Seok S, Kim DH, Yu E, Lee KW, et al. Elevated microRNA-34a in obesity reduces NAD+ levels and SIRT1 activity by directly targeting NAMPT. Aging Cell. (2013) 12:1062–72. doi: 10.1111/acel.12135
34. Kohsaka A, Laposky AD, Ramsey KM, Estrada C, Joshu C, Kobayashi Y, et al. High-fat diet disrupts behavioral and molecular circadian rhythms in mice. Cell Metab. (2007) 6:414–21. doi: 10.1016/j.cmet.2007.09.006
35. Ramsey KM, Yoshino J, Brace CS, Abrassart D, Kobayashi Y, Marcheva B, et al. Circadian clock feedback cycle through NAMPT-mediated NAD+ biosynthesis. Science. (2009) 324:651–4. doi: 10.1126/science.1171641
36. Nakahata Y, Sahar S, Astarita G, Kaluzova M, Sassone-Corsi P. Circadian control of the NAD+ salvage pathway by CLOCK-SIRT1. Science. (2009) 324:654–7. doi: 10.1126/science.1170803
37. Yeung F, Hoberg JE, Ramsey CS, Keller MD, Jones DR, Frye RA, et al. Modulation of NF-kappaB-dependent transcription and cell survival by the SIRT1 deacetylase. EMBO J. (2004) 23:2369–80. doi: 10.1038/sj.emboj.7600244
38. Asher G, Gatfield D, Stratmann M, Reinke H, Dibner C, Kreppel F, et al. SIRT1 regulates circadian clock gene expression through PER2 deacetylation. Cell. (2008) 134:317–28. doi: 10.1016/j.cell.2008.06.050
39. Belden WJ, Dunlap JC. SIRT1 is a circadian deacetylase for core clock components. Cell. (2008) 134:212–4. doi: 10.1016/j.cell.2008.07.010
40. Nakahata Y, Kaluzova M, Grimaldi B, Sahar S, Hirayama J, Chen D, et al. The NAD+-dependent deacetylase SIRT1 modulates CLOCK-mediated chromatin remodeling and circadian control. Cell. (2008) 134:329–40. doi: 10.1016/j.cell.2008.07.002
41. Schuster S, Penke M, Gorski T, Gebhardt R, Weiss TS, Kiess W, et al. FK866-induced NAMPT inhibition activates AMPK and downregulates mTOR signaling in hepatocarcinoma cells. Biochem Biophys Res Commun. (2015) 458:334–40. doi: 10.1016/j.bbrc.2015.01.111
42. De Guia RM, Hassing AS, Skov LJ, Ratner C, Plucinska K, Madsen S, et al. Fasting- and ghrelin-induced food intake is regulated by NAMPT in the hypothalamus. Acta Physiol. (2020) 228:e13437. doi: 10.1111/apha.13437
43. Kralisch S, Klein J, Lossner U, Bluher M, Paschke R, Stumvoll M, et al. Interleukin-6 is a negative regulator of visfatin gene expression in 3T3-L1 adipocytes. Am J Physiol Endocrinol Metab. (2005) 289:E586–590. doi: 10.1152/ajpendo.00090.2005
44. Gouranton E, Romier B, Marcotorchino J, Tourniaire F, Astier J, Peiretti F, et al. Visfatin is involved in TNF alpha-mediated insulin resistance via an NAD(+)/Sirt1/PTP1B pathway in 3T3-L1 adipocytes. Adipocyte. (2014) 3:180–9. doi: 10.4161/adip.28729
45. Friebe D, Loffler D, Schonberg M, Bernhard F, Buttner P, Landgraf K, et al. Impact of metabolic regulators on the expression of the obesity associated genes FTO and NAMPT in human preadipocytes and adipocytes. PLoS ONE. (2011) 6:e19526. doi: 10.1371/journal.pone.0019526
46. Kim HS, Han SY, Sung HY, Park SH, Kang MK, Han SJ, et al. Blockade of visfatin induction by oleanolic acid via disturbing IL-6-TRAF6-NF-kappa B signaling of adipocytes. Exp Biol Med. (2014) 239:284–92. doi: 10.1177/1535370213514511
47. Zhang LQ, Heruth DP, Ye SQ. Nicotinamide phosphoribosyltransferase in human diseases. J Bioanal Biomed. (2011) 3:13–25. doi: 10.4172/1948-593X.1000038
48. Ahmad R, Al-Roub A, Kochumon S, Akther N, Thomas R, Kumari M, et al. The synergy between palmitate and TNF-alpha for CCL2 production is dependent on the TRIF/IRF3 pathway: implications for metabolic inflammation. J Immunol. (2018) 200:3599–611. doi: 10.4049/jimmunol.1701552
49. Massudi H, Grant R, Guillemin GJ, Braidy N. NAD+ metabolism and oxidative stress: the golden nucleotide on a crown of thorns. Redox Rep. (2012) 17:28–46. doi: 10.1179/1351000212Y.0000000001
50. Ying WH, Alano CC, Garnier P, Swanson RA. NAD(+) as a metabolic link between DNA damage and cell death. J Neurosci Res. (2005) 79:216–23. doi: 10.1002/jnr.20289
51. Salgado-Delgado RC, Saderi N, Basualdo Mdel C, Guerrero-Vargas NN, Escobar C, Buijs RM. Shift work or food intake during the rest phase promotes metabolic disruption and desynchrony of liver genes in male rats. PLoS ONE. (2013) 8:e60052. doi: 10.1371/journal.pone.0060052
52. Tran DQ, Tse EK, Kim MH, Belsham DD. Diet-induced cellular neuroinflammation in the hypothalamus: Mechanistic insights from investigation of neurons and microglia. Mol Cell Endocrinol. (2016) 438:18–26. doi: 10.1016/j.mce.2016.05.015
53. Libermann TA, Baltimore D. Activation of interleukin-6 gene expression through the NF-kappa B transcription factor. Mol Cell Biol. (1990) 10:2327–34. doi: 10.1128/MCB.10.5.2327
54. Brasier AR. The nuclear factor-kappaB-interleukin-6 signalling pathway mediating vascular inflammation. Cardiovasc Res. (2010) 86:211–8. doi: 10.1093/cvr/cvq076
55. Shyu KG, Wang BW, Lin CM, Chang H. Cyclic stretch enhances the expression of toll-like receptor 4 gene in cultured cardiomyocytes via p38 MAP kinase and NF-kappaB pathway. J Biomed Sci. (2010) 17:15. doi: 10.1186/1423-0127-17-15
56. Guzzo C, Ayer A, Basta S, Banfield BW, Gee K. IL-27 enhances LPS-induced proinflammatory cytokine production via upregulation of TLR4 expression and signaling in human monocytes. J Immunol. (2012) 188:864–73. doi: 10.4049/jimmunol.1101912
57. Oishi Y, Hayashi S, Isagawa T, Oshima M, Iwama A, Shimba S, et al. Bmal1 regulates inflammatory responses in macrophages by modulating enhancer RNA transcription. Sci Rep. (2017) 7:7086. doi: 10.1038/s41598-017-07100-3
58. Nguyen KD, Fentress SJ, Qiu Y, Yun K, Cox JS, Chawla A. Circadian gene Bmal1 regulates diurnal oscillations of Ly6C(hi) inflammatory monocytes. Science. (2013) 341:1483–8. doi: 10.1126/science.1240636
59. Castanon-Cervantes O, Wu M, Ehlen JC, Paul K, Gamble KL, Johnson RL, et al. Dysregulation of inflammatory responses by chronic circadian disruption. J Immunol. (2010) 185:5796–805. doi: 10.4049/jimmunol.1001026
60. Chang YH, Chang DM, Lin KC, Shin SJ, Lee YJ. Visfatin in overweight/obesity, type 2 diabetes mellitus, insulin resistance, metabolic syndrome and cardiovascular diseases: a meta-analysis and systemic review. Diabetes Metab Res Rev. (2011) 27:515–27. doi: 10.1002/dmrr.1201
61. Chen MP, Chung FM, Chang DM, Tsai JC, Huang HF, Shin SJ, et al. Elevated plasma level of visfatin/pre-B cell colony-enhancing factor in patients with type 2 diabetes mellitus. J Clin Endocrinol Metab. (2006) 91:295–9. doi: 10.1210/jc.2005-1475
62. Lopez-Bermejo A, Chico-Julia B, Fernandez-Balsells M, Recasens M, Esteve E, Casamitjana R, et al. Serum visfatin increases with progressive beta-cell deterioration. Diabetes. (2006) 55:2871–5. doi: 10.2337/db06-0259
63. El-Mesallamy HO, Kassem DH, El-Demerdash E, Amin AI. Vaspin and visfatin/Nampt are interesting interrelated adipokines playing a role in the pathogenesis of type 2 diabetes mellitus. Metabolism. (2011) 60:63–70. doi: 10.1016/j.metabol.2010.04.008
64. Zhao Y, Liu XZ, Tian WW, Guan YF, Wang P, Miao CY. Extracellular visfatin has nicotinamide phosphoribosyltransferase enzymatic activity and is neuroprotective against ischemic injury. Cns Neuroscience and Therapeutics. (2014) 20:539–47. doi: 10.1111/cns.12273
65. Camp SM, Ceco E, Evenoski CL, Danilov SM, Zhou T, Chiang ET, et al. Unique toll-like receptor 4 activation by NAMPT/PBEF induces NF kappa B signaling and inflammatory lung injury. Sci Rep. (2015) 5:13135. doi: 10.1038/srep13135
66. Jacques C, Holzenberger M, Mladenovic Z, Salvat C, Pecchi E, Berenbaum F, et al. Proinflammatory actions of visfatin/nicotinamide phosphoribosyltransferase. (Nampt) involve regulation of insulin signaling pathway and Nampt enzymatic activity. J Biol Chem. (2012) 287:15100–8. doi: 10.1074/jbc.M112.350215
67. Wu MH, Tsai CH, Huang YL, Fong YC, Tang CH. Visfatin promotes IL-6 and TNF-alpha production in human synovial fibroblasts by repressing miR-199a-5p through ERK, p38 and JNK signaling pathways. Int J Mol Sci. (2018) 19:190. doi: 10.3390/ijms19010190
68. Park BS, Jin SH, Park JJ, Park JW, Namgoong IS, Kim YI, et al. Visfatin induces sickness responses in the brain. PLoS ONE. (2011) 6:e15981. doi: 10.1371/journal.pone.0015981
Keywords: obesity, NAMPT, visfatin, circadian, palmitate, hypothalamus, inflammation
Citation: Tran A, He W, Jiang N, Chen JTC and Belsham DD (2020) NAMPT and BMAL1 Are Independently Involved in the Palmitate-Mediated Induction of Neuroinflammation in Hypothalamic Neurons. Front. Endocrinol. 11:351. doi: 10.3389/fendo.2020.00351
Received: 05 March 2020; Accepted: 05 May 2020;
Published: 12 June 2020.
Edited by:
Alfonso Abizaid, Carleton University, CanadaReviewed by:
Xavier Bonnefont, INSERM U1191 Institut de Génomique Fonctionnelle (IGF), FranceShimon Amir, Concordia University, Canada
Copyright © 2020 Tran, He, Jiang, Chen and Belsham. This is an open-access article distributed under the terms of the Creative Commons Attribution License (CC BY). The use, distribution or reproduction in other forums is permitted, provided the original author(s) and the copyright owner(s) are credited and that the original publication in this journal is cited, in accordance with accepted academic practice. No use, distribution or reproduction is permitted which does not comply with these terms.
*Correspondence: Denise D. Belsham, ZC5iZWxzaGFtJiN4MDAwNDA7dXRvcm9udG8uY2E=
†These authors share first authorship