- U.S. Department of Agriculture, Agricultural Research Service, Grand Forks Human Nutrition Research Center, Grand Forks, ND, United States
Childhood obesity in girls is associated with early puberty and menarche. Breast tissue exhibits circadian rhythms. These rhythms may be altered by environmental factors. We hypothesized that a high-fat diet (HFD) disrupts circadian rhythms in pubertal mammary glands. Weanling female C57BL/6 mice were fed the standard AIN93G diet or a HFD (providing 16% or 45% of energy from soybean oil) for 3 weeks. Mammary glands were harvested from 6-week-old mice every 4 h on Zeitgeber time over a 48-h period; rhythmic expressions of circadian genes and genes encoding estrogen receptor and progesterone receptor were analyzed by using the Cosinor model. HFD, compared to AIN93G diet, altered diurnal oscillations of circadian genes in pubertal mammary glands. These included changes in amplitude of Per2, Cry1 (reduced), Clock, Rev-erbα, and Per1 (elevated), a delay in acrophase (the hour at which the rhythm peaks) of Bmal1 by 2.2 h, and changes in mesor (the mean of the rhythm from peak to trough) of Bmal1, Per2, Cry1 (reduced), Rev-rebα, and Per1 (elevated). Furthermore, HFD altered diurnal expression of estrogen receptor and progesterone receptor at both mRNA and protein levels. These findings indicate that HFD alters circadian regulation in pubertal mammary glands, which may contribute to the disturbance of hormonal homeostasis and lead to early development and growth of mammary glands in pubertal mice.
Introduction
The prevalence of overweight and obesity in children has increased substantially worldwide (1). Childhood obesity can have long lasting health impacts. It is a risk factor for chronic diseases including breast cancer. Obese girls exhibit an earlier onset of puberty (2, 3) and breast development (4, 5) with the elevation of ovarian hormones. The earlier age of menarche is associated with an increased risk of breast cancer in adult women (6, 7). Breast cancer remains a leading cause of death for women worldwide (8).
Childhood obesity occurs with lifestyle changes in the modern world (e.g., erratic eating and sedentary behavior). These lifestyle choices, as environmental factors, disrupt diurnal patterns of circadian rhythms and contribute to metabolic dysfunction and obesity (9–11). All biological systems exhibit circadian rhythms that cycle approximately every 24 h from gene expression to physiological functions (e.g., fasting vs. eating) (12–14). The central clock is located in the hypothalamus, and peripheral clocks are in all other organs. The synchrony between the central and peripheral clocks regulates metabolism to set temporal rhythms in homeostatic regulation for health and well-being (12–14). The central clock is controlled by light input via the retina and passes these signals onto peripheral clocks.
Peripheral organs, including mammary glands, have their own intrinsic self-sustained circadian oscillations, which are sensitive to environmental changes (e.g., erratic eating) but depend on the central clock for synchronization (15, 16). At the molecular level, both central and peripheral clocks consist of interconnected transcription-translation feedback loops that regulate daily circadian oscillations. Activation of circadian locomotor output cycles kaput/aryl hydrocarbon receptor nuclear translocator-like protein 1 (Clock/Bmal1) heterodimers occurs during the rest phase (the light phase for nocturnal animals) and leads to the transcription of many clock-controlled genes, including period (Per) and cryptochrome (Cry). The Per/Cry dimers reach peak expression during the late rest phase or early active phase (the dark phase for nocturnal animals) and interact with Clock/Bmal1 to repress their transcription via a negative-feedback loop. This, in turn, leads to the start of a new cycle of Clock/Bmal1 transcription in the rest phase. Clock/Bmal1 dimers also activate the expression of nuclear receptor subfamily 1 group D member 1 (Rev-erb) that leads to the repression of Clock/Bmal1.
Consumption of a high-fat diet, as an environmental factor, disrupts the meticulously coordinated circadian system and results in metabolic dysfunction (9, 17). For example, a high-fat diet alters the eating pattern of mice with more food intake during the light phase (10), leading to phase-advance of Per2 in liver (11) and blunting the rhythmicity of Clock, Bmal1, and Per2 in liver (18, 19) and adipose tissue (18). The dampened expression of circadian genes occurs concurrently with the increases in proinflammatory cytokines and body adiposity in diet-induced obese mice (10).
Mammary glands are unique because development mainly occurs post-embryonically during puberty. The ovarian steroid hormones are involved in mammary development. Estrogen directly acts on mammary glands; estrogen receptor 1 regulates ductal morphogenesis (20, 21) and estrogen receptor 2 facilitates terminal differentiation (22). Progesterone stimulates mammary epithelial DNA synthesis and alveolar development through progesterone receptor (23, 24). Disruption of the receptor expression alters mammary development. For example, mammary glands from estrogen receptor 1 knockout mice do not undergo ductal morphogenesis (25) while those from progesterone receptor knockout mice are arrested at the simple ductal stage (24). There is growing evidence that circadian regulation plays a role in breast development and growth (26, 27).
Feeding weanling mice a high-fat diet disrupts daily oscillations of energy expenditure (28), increases body adiposity (28), and enhances mammary tumorigenesis in a mouse mammary tumor virus-polyomavirus middle T-antigen (MMTV-PyMT) model of breast cancer (29). Time-restricted feeding of weanling mice during the dark phase reduces the high-fat diet-enhanced mammary tumorigenesis (30). Time-restricted feeding is an effective tool in circadian research that restores daily rhythms in rodent models, evidenced by the restored diurnal oscillations of circadian genes (17) and energy metabolism (28).
The above findings support the concept that disruption of circadian rhythms at early stages of mammary development contributes to diet-enhanced mammary tumorigenesis. However, there is a lack of knowledge of the degree to which diet alters diurnal expression of circadian genes in mammary glands of pubertal mice. We hypothesized that a high-fat diet disrupts diurnal expression of circadian genes in pubertal mammary glands. This study tested this hypothesis by comparing differences in diurnal expressions of circadian genes and genes encoding estrogen receptor and progesterone receptor in mammary glands from pubertal mice fed the AIN93G diet or a high-fat diet.
Materials and Methods
Animals and Diets
Weanling 3-week-old female C57BL/6 mice (Envigo, Madison, WI, USA) were housed, three per cage, in a pathogen-free room with a 12:12-h light/dark cycle and a temperature of 22 ± 1°C. The light intensity of the light phase was 32 lux. The standard AIN93G diet (31) and a modified AIN93G diet containing 45% of energy from soybean oil (hereafter referred to as the high-fat diet, HFD) were used in this study (Table 1). HFD was adjusted to provide equal amounts of vitamins and minerals on a per caloric basis to AIN93G diet (Table 1). Gross energy of each diet (Table 1) was quantified by using an oxygen bomb calorimeter (Model 6,200, Parr Instrument, Moline, IL, USA). Both diets (powder form) were kept at −20°C. Fresh diets were provided to mice every other day.
Experimental Design
After acclimation on AIN93G diet for 2 days, mice were randomly assigned to two groups of 70 each and fed AIN93G or HFD for 3 weeks. Mice had free access to their diets and deionized water and were weighed weekly. To avoid potential circadian disruption by housing mice in metabolic cages and to avoid exposure to magnetic resonance, food intake and body composition assessments were not performed. At the end of the study, five mice from each group were euthanized by an intra-peritoneal injection of a mixture of ketamine and xylazine at Zeitgeber time 0 (light on), 4, 8, 12 (light off), 16, 20, and 24 over a period of 48 h. Mammary glands of each mouse from thoracic, abdominal, and inguinal locations were collected and pooled, snap-frozen in liquid nitrogen, and stored at −80°C.
RNA Isolation and Real-Time Quantitative PCR
Total RNA was isolated from mammary glands by using the QIAzol Lysis reagent with DNAse treatment (RNeasy Lipid Tissue Mini Kit mRNA, Qiagen, Germantown, MD, USA). The quantity and quality of purified RNA were evaluated by using the NanoDrop 8000 Spectrophotometer (Thermo Scientific, Wilmington, DE, USA). cDNA was synthesized by using the high capacity cDNA reverse transcription kit (Applied Biosystems, Waltham, MA, USA). Real-time qPCR of Clock (Mm00455950_m1), Bmal1 (Mm00500223_m1), Per1 (Mm00501813_m1) and Per2 (Mm00478099_m1), Cry1 (Mm00514392_m1), Rev-erbα (Mm00520708_m1), estrogen receptors 1 (Esr1) (Mm00433149_m1) and 2 (Esr2) (Mm00599821_m1), and progesterone receptor (Pgr) (Mm00435628_m1) were analyzed and normalized to the 18s rRNA (Mm03928990_g1) by using the TaqMan predesigned gene-specific probes on the ABI QuantStudio 12K-Flex Real-time PCR system (Applied Biosystems). The relative changes in gene expression were calculated by using the 2−ΔΔCT method (32).
Quantification of Estradiol, Progesterone, and Their Receptors in Mammary Glands
Total protein was extracted from mammary glands by using the radioimmunoprecipitation assay buffer (R2078, Sigma, St Louis, MO, USA) with protease and phosphatase inhibitors (33, 34). Protein concentrations of mammary extracts were quantified by using the bicinchoninic acid protein assay (Thermo Scientific). Sandwich enzyme-linked immunosorbent assays were performed to quantify estradiol (Biovision, Milpitas, CA, USA), progesterone (MyBioSource, San Diego, CA, USA), estrogen receptor 1 and 2, and progesterone receptor (LifeSpan BioSciences, Seattle, WA, USA) in mammary glands. Samples were read within the linear range of the assay. The accuracy of the analysis was confirmed by the controls provided in each kit.
Statistical Analyses
Weekly body weight differences between the two dietary groups were analyzed by using a repeated measures analysis of variance followed by Tukey contrasts at each week. Student's t-test was performed to compare differences in concentrations of estradiol, progesterone, and their receptors in mammary glands between the two groups. The rhythmic nature of the gene and protein expression was analyzed by using the Cosinor model. Specifically, the model was y = mesor + amplitude x Cos [2π/24 × (t—acrophase)], where mesor (midline estimating statistic of rhythm) = the mean of the oscillations, amplitude = the distance between the mesor and the peak of the oscillations, acrophase = the hour at which the maximum value of the cosine wave (the peak of the rhythm) occurs, and t = time in hours. The period of the oscillations was assumed to be 24 h. Nested non-linear models were used to determine if separate model parameters (mesor, amplitude, and acrophase) were needed for each dietary group or if common parameters were sufficient. In these analyses, parameters were estimated directly for the AIN93G group, while the parameters for the HFD group were expressed as offsets from the corresponding AIN93G parameters. If the 95% confidence interval (95% CI) of the offset did not cross 0, the parameters were considered different between the AIN93G and HFD groups (p < 0.05) (35). All models were fit by using the NLIN procedure in SAS software version 9.4 (SAS Institute, Inc., Cary, NC, USA).
Results
Body Weight
Mice fed HFD were slightly heavier than mice fed AIN93G diet; the difference was significant 2 weeks after the initiation of HFD (p < 0.05) and continued throughout the study (Figure 1). At the end of the study, body weight of mice fed AIN93G diet was 18.2 ± 0.2 g and that of mice fed HFD was 19.0 ± 0.2 g (n = 70 per group).
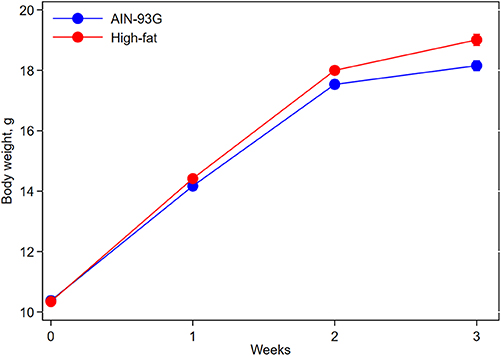
Figure 1. Body weight of pubertal mice fed AIN93G or high-fat diet. Mice fed high-fat diet were slightly heavier than mice fed AIN93G diet. The difference was significant at week 2 (p < 0.05) and week 3 (p < 0.01) after the high-fat diet (n = 70 per group).
Expression of Circadian Genes in Mammary Glands
Consumption of HFD altered diurnal oscillations of circadian genes in mammary glands (Figure 2). Compared to AIN93G diet, HFD induced >30% reduction in amplitude of Per2 and Cry1 and >50% elevation in amplitude of Clock, Rev-erbα, and Per1 (Figure 2, Table 2). HFD also delayed the acrophase of Bmal1 by 2.2 h (Figure 2B, Table 2). There was no difference in acrophase between the two groups for the other five circadian genes analyzed (Table 2). Compared to AIN93G diet, HFD resulted in >20% reduction in mesor of Bmal1, Per2, and Cry1 and >30% elevation in mesor of Rev-erbα and Per1 (Figure 2, Table 2).
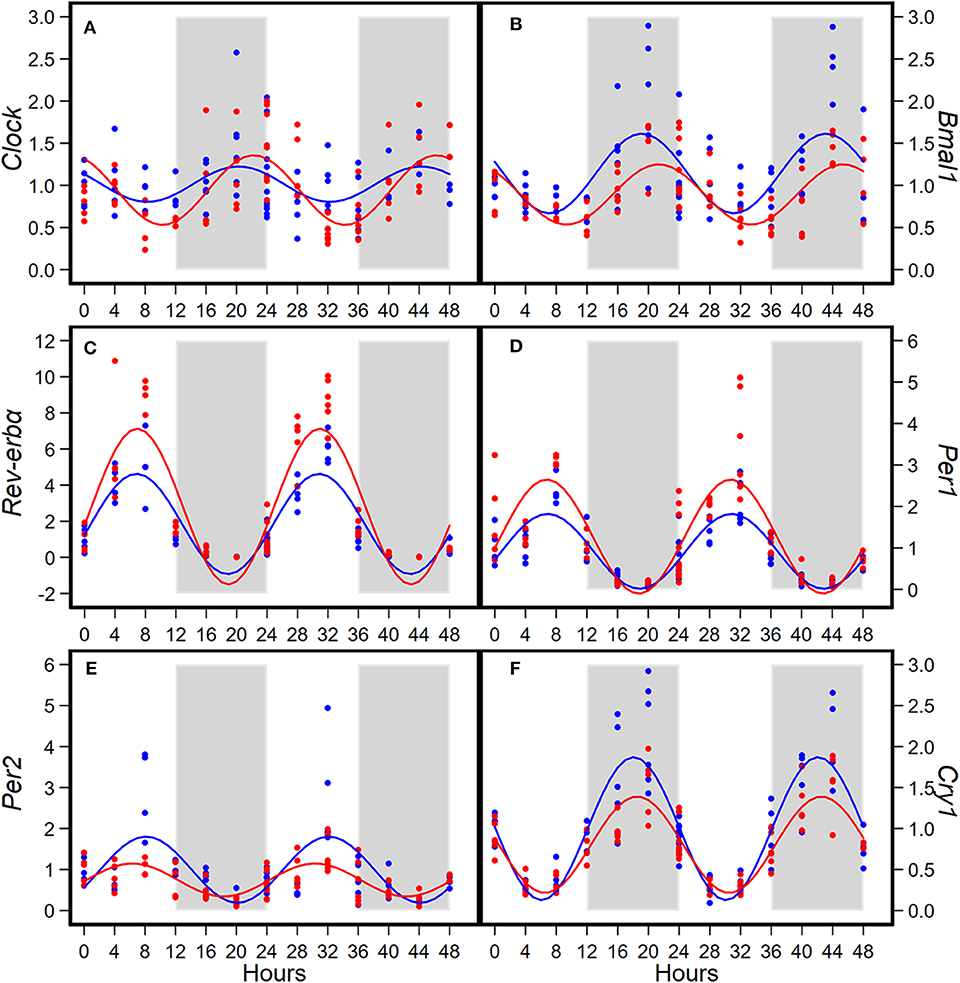
Figure 2. Diurnal expressions of Clock (A), Bmal1 (B), Rev-erbα (C), Per1 (D), Per2 (E), and Cry1 (F) in mammary glands from pubertal mice fed AIN93G or high-fat diet (n = 5 per time point per group). Open background: light phase; gray background: dark phase; blue circles and line: AIN93G diet; red circles and line: high-fat diet. The rhythm curves were generated by using the Cosinor model y = mesor + amplitude x Cos [2π/24 × (t—acrophase)].
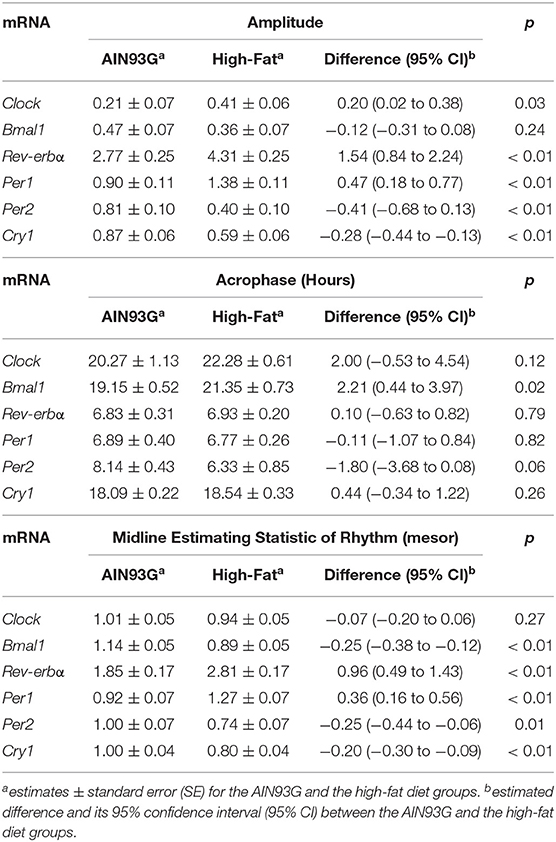
Table 2. Estimated values obtained from the Cosinor model of fold changes in expression of circadian genes in mammary glands from pubertal mice fed AIN93G or high-fat diet.
Expression of Estrogen Receptor and Progesterone Receptor Genes in Mammary Glands
HFD altered diurnal expression of genes encoding estrogen receptor and progesterone receptor in mammary glands (Figure 3, Table 3). There were no significant differences in diurnal oscillations of Esr1 between the two groups, regarding amplitude, acrophase, and mesor (Figure 3A, Table 3). HFD, compared to AIN93G diet, resulted in a >30% reduction in amplitude and a 20% reduction in mesor of Esr2 (Figure 3B, Table 3). HFD also delayed acrophase of Pgr by 2.8 h and elevated the mesor of Pgr by 60% (Figure 3C, Table 3).
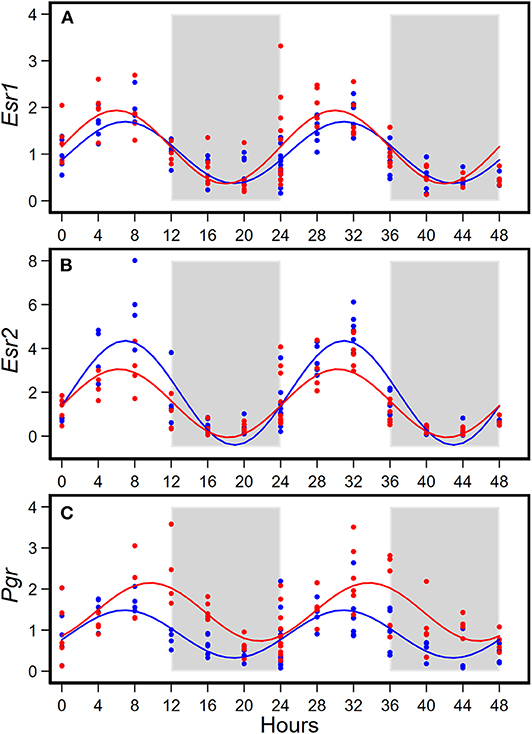
Figure 3. Diurnal expressions of genes encoding estrogen receptor 1 (Esr1, A), estrogen receptor 2 (Esr2, B), and progesterone receptor (Pgr, C) in mammary glands from pubertal mice fed AIN93G or high-fat diet (n = 5 per time point per group). Open background: light phase; gray background: dark phase; blue circles and line: AIN93G diet; red circles and line: high-fat diet. The rhythm curves were generated by using the Cosinor model y = mesor + amplitude x Cos [2π/24 × (t—acrophase)].
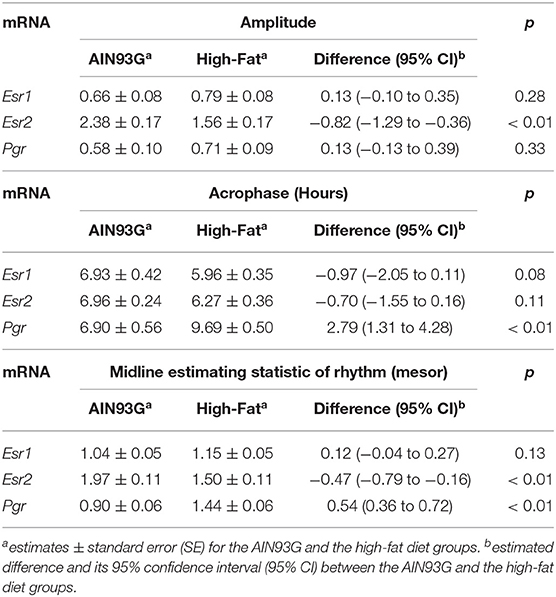
Table 3. Estimated values obtained from the Cosinor model of fold changes in the expression of genes encoding estrogen receptors and progesterone receptor in mammary glands from pubertal mice fed AIN93G or high-fat diet.
Concentrations of Estrogen, Progesterone, and Their Receptors in Mammary Glands
HFD altered diurnal oscillations of concentrations of estrogen, progesterone, and their receptors in mammary glands (Figures 4, 5, Table 4).
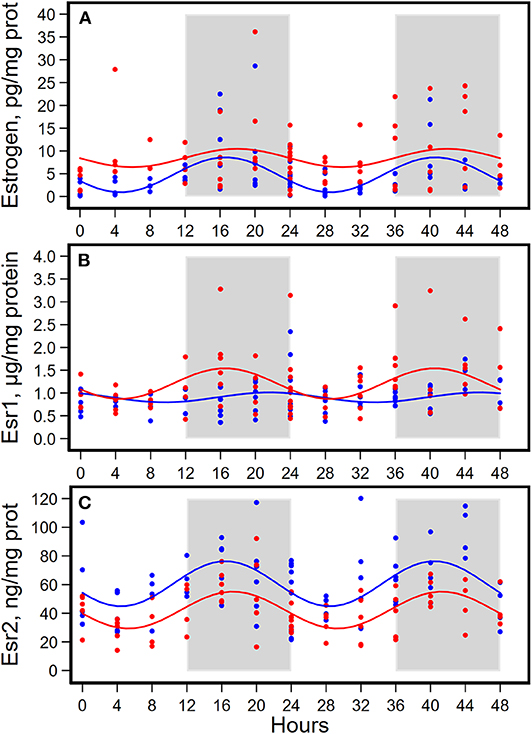
Figure 4. Diurnal expressions of concentrations of estrogen (A), estrogen receptor 1 (Esr1, B), and estrogen receptor 2 (Esr2, C) in mammary glands from pubertal mice fed AIN93G or high-fat diet (n = 5 per time point per group). Open background: light phase; gray background: dark phase; blue circles and line: AIN93G diet; red circles and line: high-fat diet. The rhythm curves were generated by using the Cosinor model y = mesor + amplitude x Cos [2π/24 × (t—acrophase)].
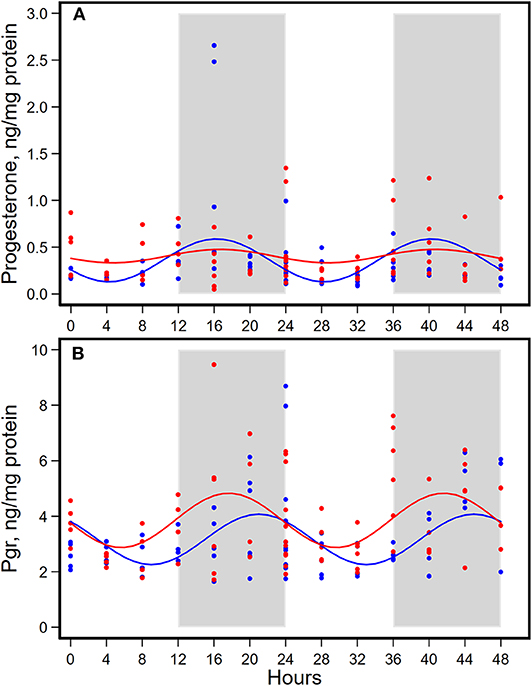
Figure 5. Diurnal expressions of concentrations of progesterone (A) and progesterone receptor (Pgr, B) in mammary glands from pubertal mice fed AIN93G or high-fat diet (n = 5 per time point per group). Open background: light phase; gray background: dark phase; blue circles and line: AIN93G diet; red circles and line: high-fat diet. The rhythm curves were generated by using the Cosinor model y = mesor + amplitude x Cos [2π/24 × (t—acrophase)].
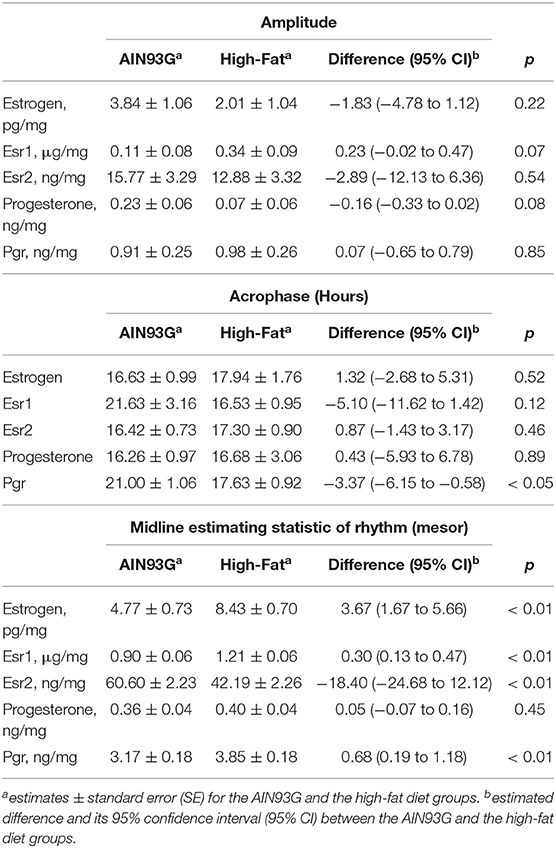
Table 4. Estimated values from the Cosinor model of concentrations of estrogen, progesterone, and their receptors in mammary glands from pubertal mice fed AIN93G or high-fat diet.
HFD elevated mesor of estrogen by 70%, but it did not result in significant changes in amplitude and acrophase of estrogen (Figure 4A, Table 4). HFD resulted in a 30% elevation in Esr1 mesor (Figure 4B, Table 4) and a 30% reduction in Esr2 mesor (Figure 4C, Table 4). Differences in mesor of estrogen and its receptors between the two groups were reflected by changes in their respective concentrations in mammary glands. HFD elevated estrogen by 90%, Esr1 by 30%, and reduced Esr2 by 30% (Table 5).
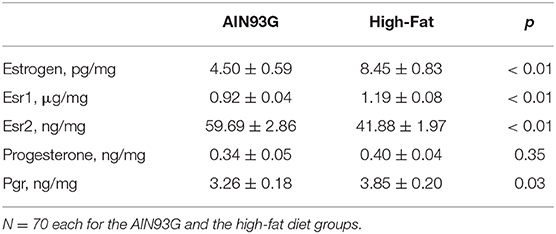
Table 5. Concentrations of estrogen, progesterone, and their receptors in mammary glands from pubertal mice fed AIN93G or high-fat diet.
HFD did not alter the expression of progesterone in amplitude, acrophase, or mesor (Figure 5A, Table 4). HFD advanced acrophase of Pgr by 3.4 h and elevated mesor of Pgr by 20% (Figure 5B, Table 4). HFD elevated Pgr by 20%, but there was no significant difference in the concentration of progesterone between the two dietary groups (Table 5).
Discussion
The present study showed diurnal expression of circadian genes in mammary glands of pubertal mice. HFD altered the rhythmic expression of circadian genes in mammary glands, evidenced by changes in amplitude, acrophase, or mesor of the rhythmic oscillations. These findings support our hypothesis that HFD disrupts diurnal expression of circadian genes in pubertal mammary glands.
Alterations in diurnal expression of circadian genes by HFD indicate that a high-fat consumption can disrupt the circadian clock in pubertal mammary glands. It has been suggested that the circadian clock is involved in cellular pathways critical for cell division and breast homeostasis. For example, elevated Per2 expression is correlated positively with cMyc and Cyclin D1 levels, whereas expression of Per1 and Bmal1 is correlated with β-casein in HC-11 mouse mammary epithelial cells and in developing mammary glands of growing C57BL/6J mice (26). Other studies have found that Per2 mutant mice exhibit deregulated expression of cMyc and Cyclin D1 (36) and that overexpression of Per2 reduces cell proliferation and induces apoptosis in EMT6 mammary epithelial cells (37). It also has been shown that the rhythmic expression of circadian genes in mammary glands varies at different stages of breast development (26, 38). While the aim of the present study was not to elucidate the role of circadian regulation in mammary gland development of pubertal mice, it suggests that alterations in diurnal circadian gene expression by a high-fat diet may disturb mammary development and growth at the pubertal stage.
Circadian genes have alternative biological functions, independent from their circadian functions, in mammary development. For example, Per2 expression is high in virgin mammary glands and Per1 expression is the highest in lactating mammary glands (26). The findings that Per2 deficient mice exhibit fewer bifurcations and a lack of distal migration of the ducts is evidence that Per2 participates in mammary branching morphogenesis (39). Providing further evidence for this, Clock mutant mice showed a defect in lactation (40, 41). We found that HFD reduced mesor of Per2 and elevated that of Per1 in pubertal mammary glands. These findings suggest the possibility that HFD may disrupt the alternative biological functions of circadian genes, through which it may disturb the normal mammary development and growth in pubertal mice.
In the present study, pubertal mammary glands exhibited diurnal expression of estrogen, progesterone, and their receptors at both mRNA and protein levels. It has been proposed that steroid hormone signaling is interconnected with circadian signaling and that the circadian clock regulates steroid hormones and their receptors. Per2 deficient mice exhibit increased numbers of Esr1-positive and Pgr-positive cells in mammary glands (39). In vitro studies showed that Per2 suppresses Esr1 transcription and induces Esr1 degradation and that Per2 is estrogen-inducible in Esr1 positive breast cancer cells (42). Bmal1 knockdown down-regulates estrogen synthesis and aromatase expression in ovarian granulosa cells (43). Disrupted expression of circadian genes, including Clock, Bmal1, Per2, Rev-erbα, and Cry1, also occurs in Esr1 negative MCF10A mammary epithelial cells (44). We found that HFD altered diurnal oscillations of components of the female sex steroid hormone system. This includes elevated mesor of estrogen, Esr1 and Pgr and a reduced Esr2 mesor. Similarly, HFD increased concentrations of estrogen, Esr1 and Pgr and lowered that of Esr2 in mammary glands. Our findings support reports showing the existence of an interconnection between circadian signaling and steroid hormone signaling (39, 42) and indicate that a high fat diet may disrupt this interconnection.
Ovarian sex steroid hormones and their receptors play important roles in breast physiology (20, 21, 24). Mammary glands grow at the same rate as other organs until puberty, when elevations in ovarian hormones in blood circulation initiate morphological changes in mammary glands. Estrogen is essential for mammary development (20), but it is also a potent mitogen (45, 46). Thus, the elevated expression of estrogen, along with disrupted expression of Esr and Pgr, in pubertal mammary glands by a high fat diet may lead to aberrant mammary development. This is supported by a previous report that pubertal intake of a high fat diet alters mammary gland development in C57BL/6 mice (47).
In this study, we observed a lack of cyclical expression of Esr1 protein, compared to the cyclical expression of Esr1, in mammary glands from mice fed AIN93G diet. Moreover, we found that HFD delayed acrophase of Pgr but advanced acrophase of Pgr protein. These findings were unexpected. They suggest the possibility of post-translational modification or involvement of other mechanisms which may have affected the rhythmic expression during translation. This certainly warrants further investigation. Nevertheless, the elevated concentrations of Esr1 and Pgr proteins in mammary glands by HFD indicate the powerfulness of a high-fat consumption in modulating biological rhythms of pubertal mammary glands.
A limitation of this study is that we did not examine the pubertal stage of mice at the end of the study. This was to avoid circadian disruption by animal handling and vaginal lavage procedures. Furthermore, due to the limited quantity of mammary tissues available from 6-week old mice, additional experiments to evaluate proliferative and developmental status of mammary glands were not feasible. These measurements are certainly considerations for future studies, which can help better understand the roles of a high-fat diet on rhythmic alterations in pubertal mammary glands.
In summary, the present study showed the existence of diurnal oscillations of circadian genes, estrogen, progesterone, and their receptors in mammary glands of pubertal mice. Consumption of a HFD disrupted these oscillations and altered concentrations of estrogen, estrogen receptors, and progesterone receptor in mammary glands. These findings indicate that HFD-mediated circadian disruption may disturb the hormonal equilibrium of mammary glands, which may lead to aberrant development of mammary glands in pubertal mice.
Data Availability Statement
The datasets generated for this study are available on request to the corresponding author.
Ethics Statement
The animal study was reviewed and approved by IACUC Grand Forks Human Nutrition Research Center.
Author Contributions
LY and SS designed the study, conducted experiments, collected data, interpreted results, and wrote the manuscript. LJ performed statistical analyses and contributed to manuscript writing. All authors contributed to review and revision of the manuscript and agreed to be accountable for the content of the work.
Funding
This work was supported by USDA Agricultural Research Service Projects #3062-51000-050-00D and #3062-51000-056-00D (LY).
Conflict of Interest
The authors declare that the research was conducted in the absence of any commercial or financial relationships that could be construed as a potential conflict of interest.
Acknowledgments
Authors gratefully acknowledge Dr. Forrest Nielsen for providng valuable input in manuscript preparation and revision, Lana DeMars for technical support, and vivarium staff for preparing diets and providing high-quality animal care. All authors read and approved the final manuscript.
Abbreviations
Bmal1, aryl hydrocarbon receptor nuclear translocator-like protein 1; Clock, circadian locomotor output cycles kaput; Cry. Cryptochrome; Esr, estrogen receptor; HFD, high-fat diet; Per, period; Prg, progesterone receptor; Rev-erbα, nuclear receptor subfamily 1, group D, member 1.
References
1. Ng M, Fleming T, Robinson M, Thomson B, Graetz N, Margono C, et al. Global, regional, and national prevalence of overweight and obesity in children and adults during 1980-2013: a systematic analysis for the global burden of disease study 2013. Lancet. (2014) 384:766–81. doi: 10.1016/S0140-6736(14)60460-8
2. Li W, Liu Q, Deng X, Chen Y, Liu S, Story M. Association between obesity and puberty timing: a systematic review and meta-Analysis. Int J Environ Res Public Health. (2017) 14:1266. doi: 10.3390/ijerph14101266
3. He F, Guan P, Liu Q, Crabtree D, Peng L, Wang H. The relationship between obesity and body compositions with respect to the timing of puberty in chongqing adolescents: a cross-sectional study. BMC Public Health. (2017) 17:664. doi: 10.1186/s12889-017-4681-1
4. Kaplowitz PB. Link between body fat and the timing of puberty. Pediatrics. (2008) 312(Suppl. 1):S208–17. doi: 10.1542/peds.2007-1813F
5. Rosenfield RL, Lipton RB, Drum ML. Thelarche, pubarche, and menarche attainment in children with normal and elevated body mass index. Pediatrics. (2009) 123:84–8. doi: 10.1542/peds.2008-0146
6. Peeters PH, Verbeek AL, Krol A, Matthyssen MM, De Waard F. Age at menarche and breast cancer risk in nulliparous women. Breast Cancer Res Treat. (1995) 33:55–61.
7. Lee SY, Kim MT, Kim SW, Song MS, Yoon SJ. Effect of lifetime lactation on breast cancer risk: a Korean women's cohort study. Int J Cancer. (2003) 105:390–3. doi: 10.1002/ijc.11078
8. Global Burden of Disease Cancer Collaboration, Fitzmaurice C, Abate D, Abbasi N, Abbastabar H, Abd-Allah F, et al. Global, regional, and national cancer incidence, mortality, years of life lost, years lived with disability, and disability-adjusted life-years for 29 cancer groups, 1990 to 2017: a systematic analysis for the global burden of disease study. JAMA Oncol. (2019) 5:1749–68. doi: 10.1001/jamaoncol.2019.2996
9. Zarrinpar A, Chaix A, Panda S. Daily eating patterns and their impact on health and disease. Trends Endocrinol Metab. (2016) 27:69–83. doi: 10.1016/j.tem.2015.11.007
10. Cunningham PS, Ahern SA, Smith LC, Da Silva Santos CS, Wager TT, Bechtold DA. Targeting of the circadian clock via CK1delta/epsilon to improve glucose homeostasis in obesity. Sci Rep. (2016) 6:29983. doi: 10.1038/srep29983
11. Branecky KL, Niswender KD, Pendergast JS. Disruption of daily rhythms by high-fat diet is reversible. PLoS ONE. (2015) 10:e0137970. doi: 10.1371/journal.pone.0137970
12. Panda S. Circadian physiology of metabolism. Science. (2016) 354:1008–15. doi: 10.1126/science.aah4967
13. Poggiogalle E, Jamshed H, Peterson CM. Circadian regulation of glucose, lipid, and energy metabolism in humans. Metabolism. (2018) 84:11–27. doi: 10.1016/j.metabol.2017.11.017
14. Johnston JD, Ordovas JM, Scheer FA, Turek FW. Circadian rhythms, metabolism, and chrononutrition in rodents and humans. Adv Nutr. (2016) 7:399–406. doi: 10.3945/an.115.010777
15. Stokkan KA, Yamazaki S, Tei H, Sakaki Y, Menaker M. Entrainment of the circadian clock in the liver by feeding. Science. (2001) 291:490–3. doi: 10.1126/science.291.5503.490
16. Marcheva B, Ramsey KM, Buhr ED, Kobayashi Y, Su H, Ko CH, et al. Disruption of the clock components CLOCK and BMAL1 leads to hypoinsulinaemia and diabetes. Nature. (2010) 466:627–31. doi: 10.1038/nature09253
17. Hatori M, Vollmers C, Zarrinpar A, Ditacchio L, Bushong EA, Gill S, et al. Time-restricted feeding without reducing caloric intake prevents metabolic diseases in mice fed a high-fat diet. Cell Metab. (2012) 15:848–60. doi: 10.1016/j.cmet.2012.04.019
18. Kohsaka A, Laposky AD, Ramsey KM, Estrada C, Joshu C, Kobayashi Y, et al. High-fat diet disrupts behavioral and molecular circadian rhythms in mice. Cell Metabolism. (2007) 6:414–21. doi: 10.1016/j.cmet.2007.09.006
19. Sun L, Wang Y, Song Y, Cheng XR, Xia S, Rahman MR, et al. Resveratrol restores the circadian rhythmic disorder of lipid metabolism induced by high-fat diet in mice. Biochem Biophys Res Commun. (2015) 458:86–91. doi: 10.1016/j.bbrc.2015.01.072
20. Bordini B, Rosenfield RL. Normal pubertal development: part I: the endocrine basis of puberty. Pediatr Rev. (2011) 32:223–9. doi: 10.1542/pir.32-6-223
21. Korach KS, Couse JF, Curtis SW, Washburn TF, Lindzey J, Kimbro KS, et al. Estrogen receptor gene disruption: molecular characterization and experimental and clinical phenotypes. Recent Prog Horm Res. (1996) 51:159–86; discussion 186–158.
22. Forster C, Makela S, Warri A, Kietz S, Becker D, Hultenby K, et al. Involvement of estrogen receptor beta in terminal differentiation of mammary gland epithelium. Proc Natl Acad Sci USA. (2002) 99:15578–83. doi: 10.1073/pnas.192561299
23. Fendrick JL, Raafat AM, Haslam SZ. Mammary gland growth and development from the postnatal period to postmenopause: ovarian steroid receptor ontogeny and regulation in the mouse. J Mammary Gland Biol Neoplasia. (1998) 3:7–22. doi: 10.1023/a:1018766000275
24. Brisken C, Park S, Vass T, Lydon JP, O'malley BW, Weinberg RA. A paracrine role for the epithelial progesterone receptor in mammary gland development. Proc Natl Acad Sci USA. (1998) 95:5076–81. doi: 10.1073/pnas.95.9.5076
25. Bocchinfuso WP, Lindzey JK, Hewitt SC, Clark JA, Myers PH, Cooper R, et al. Induction of mammary gland development in estrogen receptor-alpha knockout mice. Endocrinology. (2000) 141:2982–94. doi: 10.1210/endo.141.8.7609
26. Metz RP, Qu X, Laffin B, Earnest D, Porter WW. Circadian clock and cell cycle gene expression in mouse mammary epithelial cells and in the developing mouse mammary gland. Dev Dyn. (2006) 235:263–71. doi: 10.1002/dvdy.20605
27. Rossetti S, Corlazzoli F, Gregorski A, Azmi NH, Sacchi N. Identification of an estrogen-regulated circadian mechanism necessary for breast acinar morphogenesis. Cell Cycle. (2012) 11:3691–700. doi: 10.4161/cc.21946
28. Sundaram S, Yan L. Time-restricted feeding reduces adiposity in mice fed a high-fat diet. Nutr Res. (2016) 36:603–11. doi: 10.1016/j.nutres.2016.02.005
29. Sundaram S, Yan L. High-fat diet enhances mammary tumorigenesis and pulmonary metastasis and alters inflammatory and angiogenic profiles in MMTV-PyMT mice. Anticancer Res. (2016) 36:6279–87. doi: 10.21873/anticanres.11223
30. Sundaram S, Yan L. Time-restricted feeding mitigates high-fat diet-enhanced mammary tumorigenesis in MMTV-PyMT mice. Nutr Res. (2018) 59:72–9. doi: 10.1016/j.nutres.2018.07.014
31. Reeves PG, Nielsen FH, Fahey GCJ. AIN-93 purified diets for laboratory rodents: final report of the American institute of nutrition ad hoc writing committee on the reformulation of the AIN-76A rodent diet. J Nutr. (1993) 123:1939–51. doi: 10.1093/jn/123.11.1939
32. Livak KJ, Schmittgen TD. Analysis of relative gene expression data using real-time quantitative PCR and the 2(-Delta Delta C(T)) method. Methods. (2001) 25:402–8. doi: 10.1006/meth.2001.1262
33. Ortega FJ, Moreno-Navarrete JM, Mayas D, Garcia-Santos E, Gomez-Serrano M, Rodriguez-Hermosa JI, et al. Breast cancer 1 (BrCa1) may be behind decreased lipogenesis in adipose tissue from obese subjects. PLoS ONE. (2012) 7:e33233. doi: 10.1371/journal.pone.0033233
34. Yan L, Sundaram S, Nielsen FH. Voluntary running of defined distances reduces body adiposity and its associated inflammation in C57BL/6 mice fed a high-fat diet. Appl Physiol Nutr Metab. (2017) 42:11179–84. doi: 10.1139/apnm-2017-0285
36. Fu L, Pelicano H, Liu J, Huang P, Lee C. The circadian gene period2 plays an important role in tumor suppression and DNA damage response in vivo. Cell. (2002) 111:41–50. doi: 10.1016/S0092-8674(02)00961-3
37. Hua H, Wang Y, Wan C, Liu Y, Zhu B, Yang C, et al. Circadian gene mPer2 overexpression induces cancer cell apoptosis. Cancer Sci. (2006) 97:589–96. doi: 10.1111/j.1349-7006.2006.00225.x
38. Casey T, Patel O, Dykema K, Dover H, Furge K, Plaut K. Molecular signatures reveal circadian clocks may orchestrate the homeorhetic response to lactation. PLoS ONE. (2009) 4:e7395. doi: 10.1371/journal.pone.0007395
39. Mcqueen CM, Schmitt EE, Sarkar TR, Elswood J, Metz RP, Earnest D, et al. PER2 regulation of mammary gland development. Development. (2018) 145:dev157966. doi: 10.1242/dev.157966
40. Hoshino K, Wakatsuki Y, Iigo M, Shibata S. Circadian clock mutation in dams disrupts nursing behavior and growth of pups. Endocrinology. (2006) 147:1916–23. doi: 10.1210/en.2005-1343
41. Dolatshad H, Campbell EA, O'hara L, Maywood ES, Hastings MH, Johnson MH. Developmental and reproductive performance in circadian mutant mice. Hum Reprod. (2006) 21:68–79. doi: 10.1093/humrep/dei313
42. Gery S, Virk RK, Chumakov K, Yu A, Koeffler HP. The clock gene Per2 links the circadian system to the estrogen receptor. Oncogene. (2007) 26:7916–20. doi: 10.1038/sj.onc.1210585
43. Zhang J, Liu J, Zhu K, Hong Y, Sun Y, Zhao X, et al. Effects of BMAL1-SIRT1-positive cycle on estrogen synthesis in human ovarian granulosa cells: an implicative role of BMAL1 in PCOS. Endocrine. (2016) 53:574–84. doi: 10.1007/s12020-016-0961-2
44. Rossetti S, Esposito J, Corlazzoli F, Gregorski A, Sacchi N. Entrainment of breast (cancer) epithelial cells detects distinct circadian oscillation patterns for clock and hormone receptor genes. Cell Cycle. (2012) 11:350–60. doi: 10.4161/cc.11.2.18792
45. Sternlicht MD. Key stages in mammary gland development: the cues that regulate ductal branching morphogenesis. Breast Cancer Res. (2006) 8:201. doi: 10.1186/bcr1368
46. Yager JD, Davidson NE. Estrogen carcinogenesis in breast cancer. N Engl J Med. (2006) 354:270–82. doi: 10.1056/NEJMra050776
Keywords: circadian rhythm, mammary glands, puberty, diet, mice
Citation: Sundaram S, Johnson LK and Yan L (2020) High-Fat Diet Alters Circadian Rhythms in Mammary Glands of Pubertal Mice. Front. Endocrinol. 11:349. doi: 10.3389/fendo.2020.00349
Received: 25 February 2020; Accepted: 04 May 2020;
Published: 18 June 2020.
Edited by:
Claudia Torres-Farfan, Austral University of Chile, ChileReviewed by:
Damasia Becu-Villalobos, CONICET Instituto de Biología y Medicina Experimental (IBYME), ArgentinaMark Andrew Lawson, University of California, San Diego, United States
Copyright © 2020 Sundaram, Johnson and Yan. This is an open-access article distributed under the terms of the Creative Commons Attribution License (CC BY). The use, distribution or reproduction in other forums is permitted, provided the original author(s) and the copyright owner(s) are credited and that the original publication in this journal is cited, in accordance with accepted academic practice. No use, distribution or reproduction is permitted which does not comply with these terms.
*Correspondence: Lin Yan, bGluLnlhbkB1c2RhLmdvdg==
†Present address: Sneha Sundaram, Covance Inc., Greenfield, IN, United States