- 1School of Marine Science, Ningbo University, Ningbo, China
- 2College of Ocean and Earth Sciences, Xiamen University, Xiamen, China
- 3Fisheries Research Institute of Fujian, Xiamen, China
Peptide hormones commonly binding with G-protein coupled receptors (GPCRs) achieve their function in reproduction. The peppermint shrimp Lysmata vittata popular in marine ornamental trade and is known to display protandric simultaneous hermaphrodite (PSH). Knowledge on reproductive biology of this commercial species is critical for resources management and aquaculture. This study employed Illumina sequencing and bioinformatics analysis to identify peptides and their candidate GPCRs from male phase (MP) and euhermaphrodite phase (EP) of L. vittata. A total of 61 peptide and 40 peptide GPCR transcripts derive from 44 peptide families and 13 peptide GPCR families were identified, respectively. Among them, insulin-like androgenic gland hormone and crustacean female sex hormone have two unique mature peptides, respectively, and their transcripts showed higher expression levels in MP than EP, which suggest that these sex differentiation hormones might be involved in sexual characters than spermatogenesis or vitellogenesis. Overall, the first study on identification of peptides and their GPCRs in the genus Lysmata extends our knowledge of peptidergic signaling in PSH species, and provides an important basis for development of aquaculture strategies.
Introduction
Peptide hormones play an important role in crustaceans reproduction. Crustacean hyperglycemic hormone superfamily (CHHs) are the typical crustacean peptide hormones. They are classified into type-I [CHH-type, comprised of crustacean hyperglycemic hormone (CHH) and ion transport peptide (ITP)] and type-II [MIH-type, comprised of molt-inhibiting hormone (MIH), mandibular organ-inhibiting hormone (MOIH), and vitellogenesis/gonad-inhibiting hormone (VIH/GIH)] peptides, involved in inhibiting ecdysteroid, methyl farnesoate, and vitellogenin synthesis (1). Insulin-like androgenic gland hormone (IAG) secreted by a crustacean male-specific androgenic gland (AG) is regarded as a peptidergic hormone and regulates male sex differentiation. IAG silencing in the giant prawn Macrobrachium rosenbergii (2) and red claw crayfish Cherax quadricarinatus (3) resulted in masculinization arrest and functional sex reversal, leading to the production of monosex populations (4). Moreover, IAG is not the sole sex differentiation hormone in crustaceans, and crustacean female sex hormone (CFSH), a specific hormone that plays critical role in female reproductive phenotypes was recently isolated from the eyestalk of female blue crab Callinectes sapidus (5). Silencing CFSH impairs the mating and maternal care structures of females, such as absent or misplaced gonopores, sharper abdomens, as well as shorter and fewer setae on pleopods (5). Recent studies have found that several peptide hormones are also involved in crustacean reproduction. This includes the pigment-dispersing hormone (PDH) (6), neuroparsin (7), red pigment concentrating hormone (RPCH) (8), neuropeptide F (NPF) (9), short neuropeptide F (sNPF) (10), and allatostatin (AST) (11).
The colorful Lysmata shrimp collected from their natural environments is popular in marine ornamental trade, which are collected from their natural environments (12). Considering its growing demand in marine ornamental industry, it is important to study its reproductive biology for natural resources conservation and development of breeding techniques. Different from the gonochoristic reproductive system in most crustaceans, protandric simultaneous hermaphrodite (PSH) has been confirmed in all known species in genus Lysmata (13, 14). In PSH, the shrimp first develops as male (male phase, MP), and later become simultaneous hermaphrodite (euhermaphrodite phase, EP) which simultaneously produces sperms and eggs (13–15).
The peppermint shrimp Lysmata vittata is a small red-striped species, found in the coast of China, Japan, Philippines, Indonesia, and Australia (16–19). This species was also reported to have invaded the Atlantic Ocean, New Zealand and Brazil (12, 20, 21). Based on its histological features, four gonadal development stages (Stage I to IV) were defined for L. vittata. Among the four stages, stage I to III were defined as the male phase, during which testicular part of the gonads gradually develops and matures but the ovarian part remains immature. Stage IV was identified as euhermaphrodite phase, where both the testicular part and the ovarian part of the gonad mature (15). To date, study on the reproductive biology of L. vittata has mainly focused on: (1) the reproductive cycle of laboratory-reared (22), (2) ontogenetic development of gonads, and (3) external sexual characteristics (15). However, the reproduction molecular mechanisms, especially the information about peptide hormones is still unclear. Using Illumina sequencing and bioinformatics analysis, this paper tries to identify the peptide repertoire and their GPCRs in L. vittata, highlighting two sex differentiation peptide hormones, IAG and CFSH. This is useful for understanding specific PSH reproductive regulatory mechanism, and for supporting aquaculture to meet the emerging demand.
Materials and Methods
Animals
L. vittata shrimps were cultured in the aquarium at Fisheries Research Institute of Fujian, Xiamen, China. Prior to dissections, the shrimps were anesthetized on ice for 5 min. Our study does not involve endangered or protected species.
Illumina Sequencing
Total RNA from mixed tissues of MP carapace (mixture of 5 individuals, body weight 86–100 mg, stage I and stage II) and EP carapace (mixture of 3 individuals, body weight 260–300 mg, stage IV), was extracted using Trizol Reagent (Invitrogen), followed by Illumina sequencing. Briefly, mRNA with poly (A) was isolated from total RNA using Oligo (dT) beads (Invitrogen). The mRNA was broken into short fragments (about 200 bp) using fragmentation buffer. These fragments were used as templates to synthesize the first-strand cDNA with random hexamers, after which a second-strand cDNA was synthesized. Adaptors were ligated onto the second-strand cDNA following by Illumina Hiseq sequencing (HiSeq 4000 SBS Kit (300 cycles), Illumina). The raw reads were quality controlled using Trimmomatic to generate clean reads, before performing de novo assembly through Trinity (v2.5.1). All clean reads were aligned with Bowtie2 (v2.3.4), followed by joint abundance estimation and RSEM to calculate transcripts per million (TPM) values.
Bioinformatics Analysis
Peptide and GPCR sequences were collected from the shrimp de novo assembly. To identify peptide, we used the well-established workflow (23). Signal peptide of the peptide precursors were predicted using SignalP 4.1 (http://www.cbs.dtu.dk/services/SignalP/). Prohormone cleavage sites prediction based on the standards were defined by Veenstra (24) and the peptide structures were predicted based on the established propeptide processing schemes (25–27). GPCRs identification was performed as our previous study (28). Deorphanized peptide GPCRs from insects and reported peptide GPCRs from crustaceans were used as reference sequences (28–37). A phylogenetic tree was built with related sequences of these GPCRs and L. vittata GPCRs transmembrane domains. Multiple sequence alignment was performed with ClustalX and the conserved sequence motifs were highlighted by LaTEX TexShade (38). The phylogenetic analysis was calculated using PhyML (SeaView software) (39) and the resultant phylogenetic tree was visualized with Figtree v1.4.3 and Photoshop CS 6.
Results
The mRNA-sequencing and de novo assembly data are shown in Table S1. The assembled transcripts (N = 71,009) had a total size of 65,718,743 bp, an average size of 925.5 bp and N50 assembled transcripts with 1687 bp long. Using transcriptome mining, a total of 61 peptide and 40 peptide GPCR (34 belonging to A-family GPCRs (Lv-GPCR-A) and 6 belonging to B-family GPCRs [Lv-GPCR-B)] transcripts were predicted in L. vittata. These peptides included: adipokinetic hormone-corazonin-like peptide (ACP), agatoxin-like peptide, allatostatin-A (AST-A), AST-B, AST-C, AST-CC, AST-CCC, bursicon hormone, calcitonin, calcitonin-like diuretic hormone (DH31), CCHamide, CRF-like DH44, crustacean cardioactive peptide (CCAP), crustacean female sex hormone (CFSH), crustacean hyperglycemic hormone (CHH), molt-inhibiting hormone/gonad-inhibiting hormone (MIH/GIH), CHH-MIH-like peptide, ecdysis triggering hormone (ETH), eclosion hormone (EH), EFLamide, FLRFamide, glycoprotein-A2 (GPA2), glycoprotein-B5 (GPB5), Hyrg, insulin-like androgenic gland hormone (IAG), kinin, myosuppressin, natalisin, neuroparsin, neuropeptide F (NPF), orcokinin, pigment-dispersing hormone (PDH), proctolin, pyrokinin, red pigment-concentrating hormone (RPCH), RYamide, short neuropeptide F (sNPF), SIFamide, sulfakinin, tachykinin, terminal ampullae peptide (TAP), trissin and vasopressin. The peptide and GPCR transcripts source and their expression levels (TPM values) are summarized in Supplementary files (Supplementary File 1 and Table S1). Most of the peptide transcripts TPM values (51 out of 61 transcripts) in MP are higher than those in EP (Table S1). Although the expression levels of GPCRs are generally lower than peptides, their expression patterns are similar, i.e., GPCR transcripts TPM values (33 out of 40 transcripts) in MP are higher than those in EP (Table S1).
Adipokinetic Hormone-Corazonin-Like Peptide (ACP)
Two transcripts putatively encoded complete ACP precursors of 104 and 99 amino acids (aa), respectively (Supplementary File 1). These precursors have the same mature peptide, pQITFSRSWVPQamide, a highly conserved decapod ACP peptide [e.g., (40–42)].
Agatoxin-Like Peptide
One transcript was identified to encode agatoxin-like peptide precursor of 111aa (Supplementary File 1). From this precursor, a 21aa signal peptide and three distinct peptides were predicted, one of which, WRSCIPRGGSCTHRPKSCCNSSSCRCNLWGTNCRCQRMGLFQQLamide, shows 8 cysteine residues and amidated C-terminus associated with insect and decapod agatoxin-like peptides (43). Similarly, apart from toxic purposes, agatoxin-like peptide were identified in the neuroendocrine system of honey bee Apis mellifera and other insects (44).
Allatostatin (AST)
Three transcripts, a complete (AST-A2), a C-terminus (AST-A2) and a middle region (AST-A3) transcript with 334, 332 and 77aa, respectively that encode AST-A precursors were identified (Supplementary File 1). A total of 38 predicted peptides containing FGLamide were highly conserved motif derived from these precursors (Figure 1). Apart from SKSFSFGLamide, the rest of these peptides possess a conserved C-terminal motif YXFGLamide, e.g., SPGYAFGLamide, the signature of AST-A family (45). One transcript putatively encoded the complete AST-B precursor with 350aa. This precursor has 12 predicted mature peptides with a XWXXXXGXWamide conserved motif (Figure 1), e.g., ADWSSMRGTWGamide sequence, the signature of AST-B family (45). Three transcripts, two C-terminus partial regions (AST-C, AST-CC) and one full-length protein (AST-CCC), with 137, 192 and 108 aa, respectively that encode AST-C precursors were identified from the transcriptome assembly (Supplementary File 1). Each precursor possessed a predicted peptide with conserved motif XCXFNXXSCFX (Figure 1), i.e., pQIRYHQCYFNPISCF from AST-C, GNNNDGRLYWRCYFNAVSCF from AST-CC, and SYWKQCAFNAVSCFamide from AST-CCC (a disulfide bridge between cysteine residues in each peptide), previously reported decapod AST-C isoforms signature (46–49).
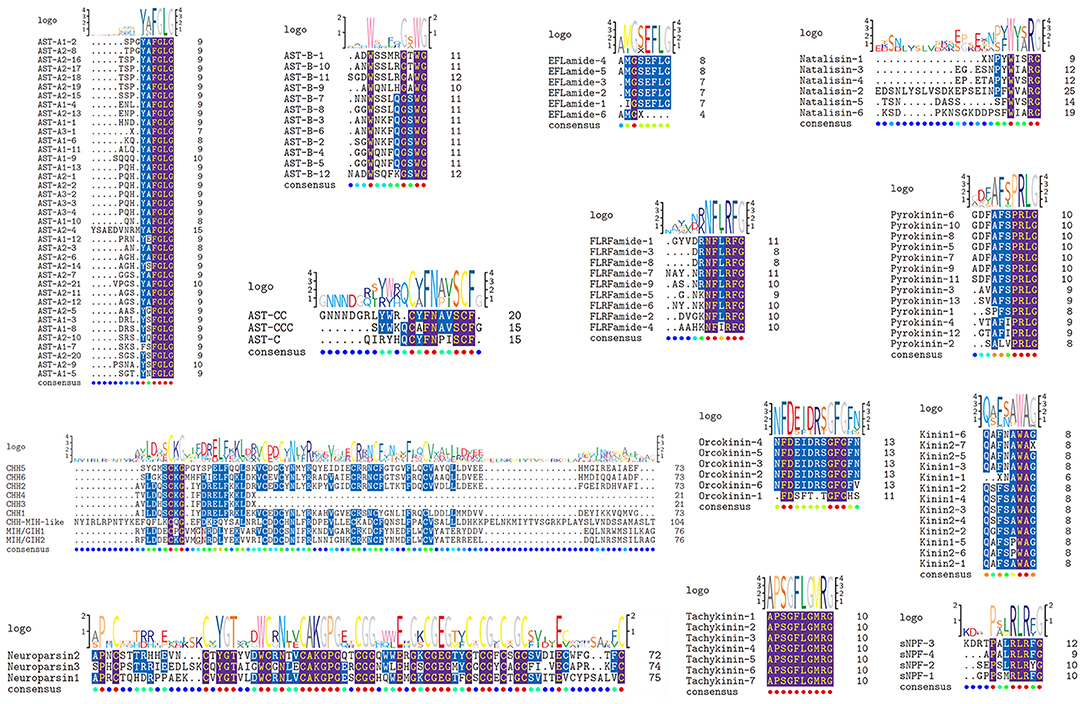
Figure 1. Identification and characterization of mature peptides in Lysmata vittata. Schematic showing the mature peptides of ASTs, CHHs, EFLamide, FLRFamide, Kinin, Natalisin, Neuroparsin, Orcokinin, Pyrokinin, sNPF, and Tachykinin identified in Lysmata vittata. Logo is shown above alignments, where the height of each letter is proportional to the observed frequency of the corresponding amino acid in the alignment column.
Bursicon Hormone
The heterodimeric peptide bursicon hormone alpha and beta subunit sequences were identified from the transcriptome assembly, encoding bursicon hormone alpha and bursicon hormone beta precursor of 148 and 136aa, respectively (Supplementary File 1). Both of these precursors start with a predicted signal peptide, followed by adjacent mature peptide with 11 cysteine residues.
Calcitonin
A single calcitonin transcript encodinng 164aa precursor was identified (Supplementary File 1). It comprised of 21aa signal peptide and three distinct peptides, one of which, TCYINAGLSHGCDYKDLVGAMAEKNYWDSLNSPamide (a disulfide bridge between two cysteine residues) is identical in structure to calcitonin from several decapod species, e.g., M. rosenbergii, the American lobster Homarus americanus, the crayfish Procambarus clarkii (43).
Calcitonin-Like Diuretic Hormone (DH31)
The predicted DH31 precursor was composed of 142aa with 23aa signal peptide and three distinct peptides (Supplementary File 1), where one of the peptides, GLDLGLGRGFSGSQAAKHLMGLAAANFAGGPamide, possesses conserved motif XXDXGLXRGXSGXXXAKXXXXXXXANXXXGPamide, the signature of DH31 family. Similar to calcitonin, L. vittata DH31 is identical in structure to DH31 from several decapod species, e.g., H. americanus, M. rosenbergii (43, 46, 50).
CCHamide
A single transcript encoding CCHamide precursor was identified (Supplementary File 1), starting with a 23aa signal peptide, followed by a C-terminal amidated peptide, i.e., VPKGGCLNYGHSCLGAHamide (a disulfide bridge between two cysteine residues), exhibiting conserved motif XCXXW/Y/FGXXCXGXHamide of CCHamide (51).
CRF-Like DH44
A single transcript was identified to encode incomplete CRF-like DH44 precursor with 230aa (Supplementary File 1). This precursor has a 45aa mature peptide, i.e., NSGLSLSIDASMKVLREALYLEMARKKQRQQMLRARHNQALLTTIamide, is similar to the previously described M. rosenbergii DH44 isoform, SSGLSLSIDASMKVLREALYLEMARKKQRQQMQRARHNQELLTSIamide (43, 50).
Crustacean Cardioactive Peptide (CCAP)
A single transcript was identified to encode CCAP precursor of 141aa (Supplementary File 1). A 30aa signal peptide and five distinct mature peptides were predicted from CCAP precursor, one of which, PFCNAFTGCamide (a disulfide bridge between two cysteine residues), is identical to previously described authentic CCAP, a conserved arthropod peptide [e.g., (25, 45)].
Crustacean Female Sex Hormone (CFSH)
Two transcripts were identified to encode CFSH precursors representing the partial N-terminus (CFSH1a) and complete protein (CFSH1b) of 208 and 229aa, respectively. A 35aa signal peptide, a CFSH precursor-related peptide and a part of the 136aa mature peptide with 6 cysteines were predicted from CFSH1a. Similarly, the CFSH1b has a 33aa signal peptide, a CFSH precursor-related peptide and the 163aa mature peptide with 8 cysteines (Supplementary File 1). Both mature peptides (except two cysteine residues lacking in CFSH1a) showed similar cysteine residues with the other decapod CFSHs (43). The phylogenetic tree revealed that two L. vittata CFSHs clustered with previously described decapod CFSH1 isoforms (43), and were analogous to M. rosenbergii CFSH1a and CFSH1b respectively (Figure S1). Therefore, we arbitrarily named them as L. vittata CFSH1a and L. vittata CFSH1b in our study.
Crustacean Hyperglycemic Hormone Superfamily (CHHs)
Nine CHHs transcripts were identified from the transcriptome assembly. Phylogenetic analysis showed that the CHHs formed two major clades: type-I CHHs and type-II CHHs. Overall, type-I CHHs clade contained three subclades: the CHHs ortholog containing CHH1-4, the CHHs ortholog containing CHH5 and CHH6, and the CHH-MIH-like peptide ortholog. In type-II CHHs clade, MIH/GIH2, the oriental river prawn Macrobrachium nipponense GIH, M. rosenbergii SGP-B and the Antarctic shrimp Chorismus antarcticus MIH/VIH formed a subgroup, separate from the M. nipponense MIH ortholog containing MIH/GIH1 (Figure 2).
Crustacean Hyperglycemic Hormone (CHH)
Six transcripts were identified to encode four complete (CHH1, CHH2, CHH5, and CHH6), one N-terminus (CHH4) and one middle region (CHH3) CHH precursors. Altogether, these sequences have a CHH precursor-related peptide (CHH-PRP) between signal peptide and mature peptide. CHH1 precursor has a 26aa signal peptide, a 37aa CHH-PRP and a 72aa mature peptide with amidated C-terminus and 6 cysteines. CHH2 precursor has a 27aa signal peptide, a 43aa CHH-PRP and a 73aa mature peptide with 6 cysteines. CHH3 precursor has a partial signal peptide, a 44aa CHH-PRP and a part of mature peptide with 1 cysteine. CHH4 precursor has a 21aa signal peptide, a 34aa CHH-PRP and a partial mature peptide (20aa) with 1 cysteine. CHH1-4 were shown to be highly conserved sequences at the N-terminus of their mature peptides (Figure 1). CHH5 precursor has a 29aa signal peptide, a 32aa CHH-PRP and a 73aa mature peptide with 6 cysteines. CHH6 precursor has a 28aa signal peptide, a 31aa CHH-PRP and a 73aa mature peptide with 6 cysteines (Supplementary File 1 and Figure 1). Different from CHH1-4, CHH5, and CHH6 exhibited high sequence similarity with 3 Caridea CHHs, with a conserved C-terminus: AIAXX (Figure 3).
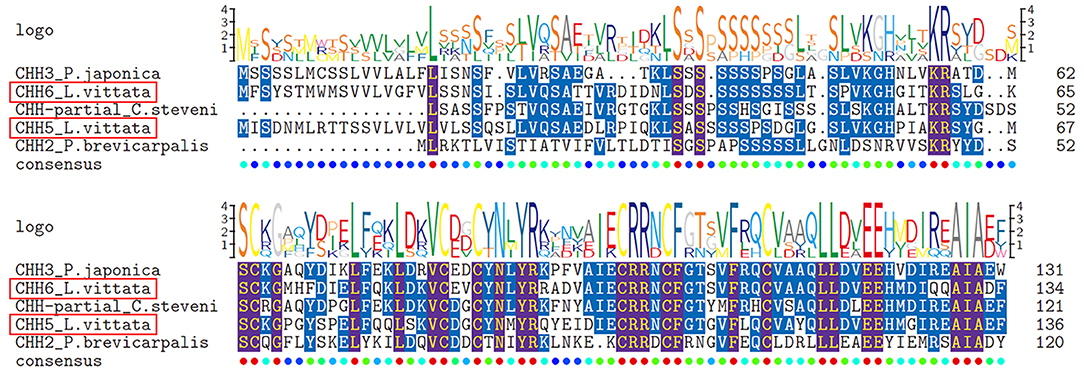
Figure 3. Comparative sequence alignment of CHHs with “AIAXX” in different Caridea. Comparative sequence alignment of CHHs with “AIAXX” in Lysmata vittata with Pandalopsis japonica, Caridion steveni and Periclimenes brevicarpalis. Sequence logo is shown above alignments.
Molt-Inhibiting Hormone/Gonad-Inhibiting Hormone (MIH/GIH)
Two transcripts were identified to encode MIH/GIH precursors with 110 and 112aa, respectively. They lack CHH-PRP and have an additional specific glycine in position 12 of the mature peptide (Gly12). The precursor MIH/GIH1 has a 32aa signal peptide and a 75aa mature peptide with amidated C-terminus. The precursor MIH/GIH2 has 34aa signal peptide and 75aa mature peptide with amidated C-terminus (Supplementary File 1 and Figure 1).
CHH-MIH-Like Peptide
One transcript was identified to encode 133aa CHH-MIH-like peptide precursor with a 29aa signal peptide and a 104aa mature peptide with 6 cysteines (Supplementary File 1 and Figure 1). This peptide defies the rules of CHH superfamily, i.e., lacks the CHH-PRP and Gly12, and it is closer to the type-I CHHs than to type-II CHHs cluster (Figure 2). It exhibits similar characteristics of CHH-MIH-like peptide from several decapod species such as, P. clarkii, E. sinensis and the white shrimp Litopenaeus vannamei (43).
Ecdysis Triggering Hormone (ETH)
One transcript was identified from the transcriptome assembly to encode ETH precursor of 135aa (Supplementary File 1). A 19aa signal peptide and two distinct mature peptides were predicted from the ETH precursor, one of which, i.e., DAGHFFAETPKHLPRIamide, is identical in structure to decapod ETH isoforms [e.g., (43)].
Eclosion Hormone (EH)
One transcript was identified to encode EH precursor of 82aa, starting with a 26aa signal peptide, followed by a 52aa mature peptides with 6 cysteines, i.e., ASITSMCIRNCGQCKEMYGDYFHGQACAESCIMTQGVSIPDCNNPATFNRFL. This is identical in structure to EH from several decapod species, e.g., M. rosenbergii, P. clarkii (37, 43, 50).
EFLamide
One transcript was identified to encode the N-terminus EFLamide precursor of 210aa, starting with a 21aa signal peptide (Supplementary File 1). Eleven peptides were predicted from this precursor, five possessing the conserved motif GSEFLamide (Figure 1), e.g., IGSEFLamide, AMGSEFLamide, the signature of EFLamide (or called GSEFLamide) family [e.g., (43, 52)]. One of these predicted peptide with incomplete sequence, AMG, was predicted as the N-terminus of EFLamide isoform.
FLRFamide (FMRFamide)
One transcript was identified to encode FLRFamide precursor with 335aa, starting with a 19aa signal peptide (Supplementary File 1). Seventeen mature peptides were predicted from FLRFamide precursor, nine of which, are 7-10aa in length with conserved motif NFL/IRFamide (Figure 1), e.g., GYVDRNFLRFamide, and AAHKNFIRFamide, the signature of FLRFamide family [e.g., (45)].
Glycoprotein-A2 (GPA2)
The predicted GPA2 precursor has 18aa signal peptide and part of mature peptide with 4 cysteines (Supplementary File 1), i.e., FQHAWQTPGCHKVGHTRKISIPECVEFDITTNACRGYCE, which shows highly conserved sequence like previously described decapod GPA2 isoforms, e.g., it is 92% identical in protein sequence to C. quadricarinatus GPA2 isoform (53).
Glycoprotein-B5 (GPB5)
One transcript was identified to encode GPB5 precursor starting with a signal peptide with no N-terminus, followed by a 125aa C-terminal amidated mature peptide with 10 cysteines (Supplementary File 1). It shows a major sequence similarity to previously described decapod GPA5 isoforms, e.g., it is 87% identical/94% positives to GPA5 of L. vannamei (43).
Hyrg
Two transcripts were identified to encode Hyrg precursors with 60aa and 63aa. Each precursor is composed of a signal peptide and a mature peptide. The peptides, i.e., YPEPAVIVDGRPNMIPDGYIQAPRFHYRGFQKPIPKYDWS from Hyrg1, LPEAAVIVEGRPNRAPDDGYVQAAPPRFHYRGFQKFVPKYDWS from Hyrg2, possess conserved motif RFHYRGF, the signature of decapod Hyrg isoforms (43).
Insulin-Like Androgenic Gland Hormone (IAG)
Two transcripts were identified to encode IAG precursors with 146aa and 153aa (Supplementary File 1). IAG1 precursor has a 28aa signal peptide, a 30aa B chain, a 42aa C peptide, and a 45aa A chain. IAG2 precursor has a 27aa signal peptide, a 32aa B chain, a 41aa C peptide, and a 38aa A chain. Both IAGs have 8 cysteine residues located at B chain and A chain, and exhibits similar characteristics as previously described IAG isoforms (4). Different from other crustaceans, they have 9 and 4aa residues between Cys4 and Cys5 in A chain of IAG1 and IAG2, respectively (Figure 4).
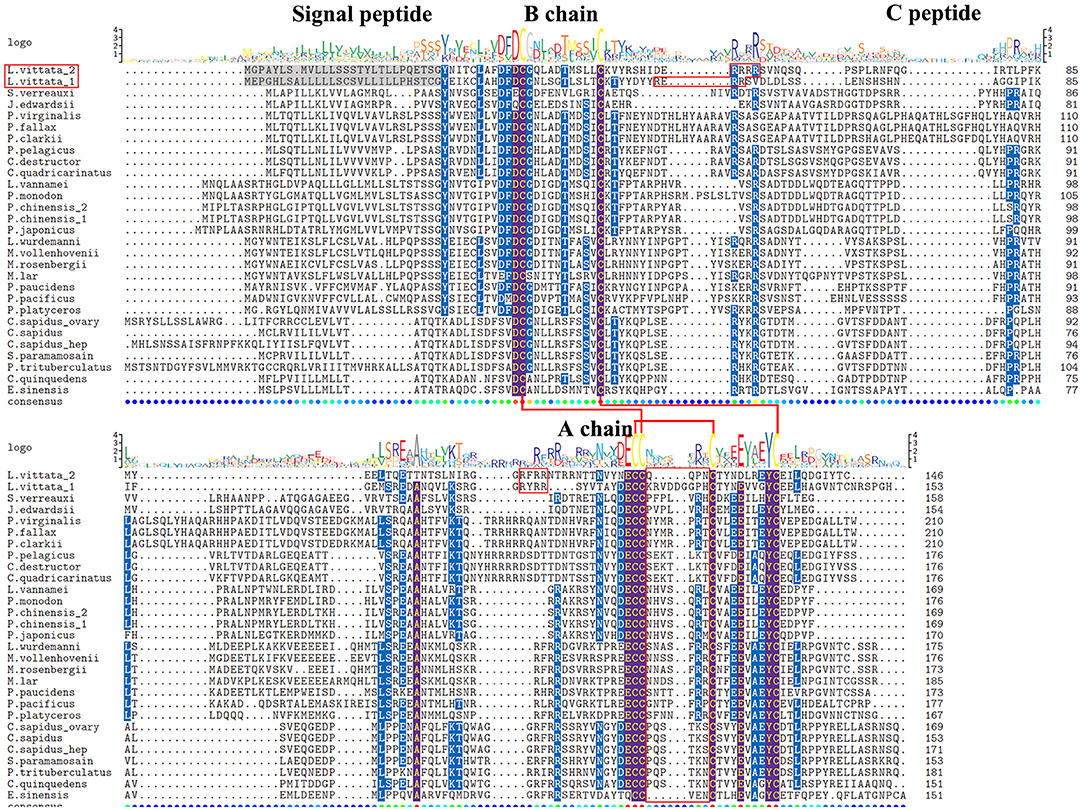
Figure 4. Comparative sequence alignment of IAG in different decapod. Comparative sequence alignment of IAG precursors in Lysmata vittata with Sagmariasus verreauxi, Jasus edwardsii, Procambarus virginalis, Procambarus fallax, Procambarus clarkii, Portunus pelagicus, Cherax destructor, Cherax quadricarinatus, Litopenaeus vannamei, Penaeus monodon, Penaeus chinensis, Penaeus japonicus, Lysmata wurdemanni, Macrobrachium vollenhovenii, Macrobrachium rosenbergii, Macrobrachium lar, Palaemon paucidens, Palaemon pacificus, Pandalus platyceros, Callinectes sapidus, Scylla paramamosain, Portunus trituberculatus, Chaceon quinquedens, and Eriocheir sinensis. Sequence logo is shown above alignments. Six conserved cysteines are highlighted in the sequence logo shown above alignments and the putative disulfide bridges are drawn with red line.
Kinin
Two nucleotide sequences were identified from the transcriptome data putatively coding for two incomplete kinin precursors with 142 and 114aa (Supplementary File 1). Both have no signal peptide. Twelve peptides were predicted from kinin1 precursor, and the first peptide lacks N-terminus. Fourteen peptides were predicted from kinin2 precursor, and the last peptide has no glycine, which is represent C-terminus amidation. Apart from these incomplete peptides, sequences of 11 mature peptides have conserved motif: XFX-A/P-WAamide (Figure 1), e.g., QSFSAWAamide, and QAFSPWAamide, the signature of kinin family (e.g., 45) (Supplementary File 1 and Figure 1).
Myosuppressin
The predicted 102aa myosuppressin precursor has a 31aa signal peptide (Supplementary File 1). Three distinct peptides were predicted from this precursor, one of which is identical in structure to conserved decapod myosuppressin family, i.e., pQDLDHVFLRFamide [e.g., (45)].
Natalisin
The predicted incomplete natalisin precursor has 102aa (Supplementary File 1). Six predicted mature peptides were released from this precursor with conserved C-terminal WXXRamide (Figure 1), e.g., EDSNLYSLVSDKEPSEINPFWVARamide, EGESNPYWIARamide, the signature of natalisin family [also called WXXRamide, e.g., (43)].
Neuroparsin
Three transcripts were predicted to encode neuroparsin precursors with 99-101aa. These precursors are composed of signal peptide and the mature peptide with 12 aligned cysteines (Supplementary File 1 and Figure 1). These mature peptides show highly conserved sequences as previously described neuroparsin isoforms, e.g., L. vittata neuroparsin1 is 63% identical/73% positive in protein sequence to L. vannamei neuroparsin [e.g., (43, 54)].
Neuropeptide F (NPF)
Two NPF transcripts were identified to encode 112 (NPF1) and 127aa (NPF2) precursors with 31aa and 29aa signal peptide (Supplementary File 1). Four distinct peptides were predicted from NPF precursors, two of which possess the C-terminal motif RPRFamide, the hallmarks of NPF family members, i.e., ARTDNTAEVLQAMHEASLAGMLSSAEVPYPSRPNVFKSPVELRQYLDALNAYYAIAGRPRFamide, KPDPTQLAAMADALKYLQELDKYYSQVSRPSPRSAPGPASQIQALEKTLKFLQLQELGKLYSLRSRPRFamide (45).
Orcokinin
The predicted orcokinin precursor is composed of 140aa, and starts with a 22aa signal peptide (Supplementary File 1). Eight mature peptides were predicted from orcokinin precursor, where five adjacent mature peptides separated by “KR” cleavage sites possess N-terminal motif NFDEIDRX (Figure 1), the signature of orcokinin family members; and one (named orcokinin-1), i.e., FDSFTTGFGHS, an identified decapod orcomyotropin isoform (45).
Pigment-Dispersing Hormone (PDH)
Two transcripts were identified to encode PDH precursors with 83 and 79aa (Supplementary File 1). The precursor PDH1 has a 22aa signal peptide and mature octadecapeptide: NSELINSLLGLPKVMNDAamide, similarly, PDH2 has a 22aa signal peptide and the mature octadecapeptide: NSGMINSLLGIPKVMTDAamide. The two mature octadecapeptides exhibit highly conserved sequences as previously described decapod PDH isoforms (e.g., 43), e.g., L. vittata PDH1 is identical to the PDH predicted from L. vannamei PDH1 precursor (55).
Proctolin
The predicted 108aa proctolin precursor has a 22aa signal peptide (Supplementary File 1). Three distinct peptides were predicted from proctolin precursor, one of which, i.e., RYLPT, is identical to the authentic proctolin [i.e., (25, 45)].
Pyrokinin
One transcript was identified to encode 272aa pyrokinin precursor with a 18aa signal peptide (Supplementary File 1). Sixteen peptides were predicted from pyrokinin precursor, thirteen of which are 7-9aa in length with conserved motif PRLamide, e.g., SPFSPRLamide, GDFAFSPRLamide, the pyrokinin family signature [i.e., (45)].
Red Pigment-Concentrating Hormone (RPCH)
One transcript was identified to encode incomplete RPCH precursor with 91aa, starting with a 21aa signal peptide (Supplementary File 1). Three distinct peptides were predicted from RPCH precursor, one of which, i.e., pQLNFSPGWamide, is identical to the authentic RPCH [i.e., (45)].
RYamide
One transcript was identified to encode RYamide precursor representing a C-terminus region (Supplementary File 1). Two distinct peptides were predicted from this precursor, one of which is an incomplete peptide with conserved “RYamide” motif, i.e., SSPSQSELPEIKIRSSRFIGGSRYamide, the RYamide family signature [i.e., (45)].
Short Neuropeptide F (sNPF)
The 173aa sNPF precursor was identified from the transcriptome data, and starts with a signal peptide (Supplementary File 1). Nine distinct peptides were predicted from this precursor, four of which are 8-11aa long with PXXRLRF/Yamide conserved motif, i.e., GPPSMRLRFamide, SEPSLRLRYamide, KDRTPALRLRFamide, APALRLRFamide, the sNPF family signature [i.e., (45)].
SIFamide
One transcript was identified to encode 76aa SIFamide precursor, starting with a 27aa signal peptide (Supplementary File 1). Two distinct peptides were predicted from SIFamide precursor, one of which, GYRKPPFNGSIFamide, identical to Gly1-SIFamide isoform [i.e., (45)].
Sulfakinin
One transcript was identified to encode 122aa sulfakinin precursor with a 21aa signal peptide (Supplementary File 1). Five distinct peptides were predicted from this precursor, where two adjacent mature peptides, pQFDEY(SO3H)GHMRFamide and AGGDYDDY(SO3H)GHLRFamide separated by carboxy-peptidase cleavage sites, possess conserved motif Y(SO3H)GHM/LRFamide, the signature of sulfakinin family [i.e., (45)].
Tachykinin
The putative tachykinin precursor is comprised of 217aa, starting with a 26aa signal peptide. Twelve predicted peptides were released by two dibasic cleavage sites (RK, KK, RR) (Supplementary File 1). Seven peptides have the same sequence: APSGFLGMRamide (Figure 1), a broadly conserved decapod tachykinin isoform [i.e., (45)].
Terminal Ampullae Peptide (TAP)
One transcript encoding TAP precursor was found in transcriptome data. This precursor composed of a 18aa signal peptide and 70aa mature peptide with 8 cysteine residues (Supplementary File 1). This peptide has 77% identical/92% positive amino acid sequence compared to the TAP predicted from M. rosenbergii TAP precursor (56).
Trissin
The predicted 200aa trissin precursor has no signal peptide (Supplementary File 1). Two distinct peptides were predicted from trissin precursor, one of which is a partial C-terminus peptide, i.e., +EVSCGSCGLECQKACGTRNFRACCFNFQ. It has 89% identical/89% positive amino acid sequence compared to the trissin predicted from C. quadricarinatus trissin precursor (53).
Vasopressin
One transcript was found from transcriptome data to encode 148aa full-length vasopressin-neurophysin precursor starting with a 19aa signal peptide (Supplementary File 1). Three distinct peptides were predicted from vasotocin-neurophysin precursor, one of which, CFITNCPPGamide (with a disulfide bridge between two cysteine residues), is structurally identical to vasopressin family (i.e., 42,43). One has a 100aa peptide with fourteen cysteine residues, and exhibits highly conserved sequence to previously described decapod neurophysin isoforms (23).
Peptide GPCRs
A total of 40 candidate peptide GPCR transcripts were predicted from L. vittata. To identify these GPCRs orthologs, hundreds of known peptide GPCRs from arthropod were collected for building phylogenetic tree. Phylogenetic analysis showed that 28 of these were clustered with known peptide receptor orthologs (Figures 5, 6). Lv-GPCR-A1 and Lv-GPCR-A2 were clustered with the AST-A receptor ortholog. Lv-GPCR-A3 was clustered with CCAP receptor ortholog. Lv-GPCR-A4 was clustered with the FMRFamide receptor ortholog. Lv-GPCR-A5 and Lv-GPCR-A6 were clustered with the natalisin receptor ortholog. Lv-GPCR-A7 was clustered with the NPF receptor ortholog. Lv-GPCR-A8 and Lv-GPCR-A31 were clustered with the RYamide receptor ortholog. Lv-GPCR-A9, Lv-GPCR-A10, Lv-GPCR-A14, Lv-GPCR-A15, Lv-GPCR-A18, Lv-GPCR-A19, Lv-GPCR-A20, Lv-GPCR-A27 were clustered with the Moody &Tre ortholog. Lv-GPCR-A13 and Lv-GPCR-A30 were clustered with the ETH receptor ortholog. Lv-GPCR-A16 and Lv-GPCR-A17 were clustered with the GPA2/GPB5 receptor ortholog. Lv-GPCR-A23, Lv-GPCR-A25, Lv-GPCR-A29, and several putative CHH receptors were clustered with Bombyx mori BNGR-A34, which has been defined as ITP receptor (57). Lv-GPCR-A33 was clustered with the CCHamide receptor ortholog. Lv-GPCR-B1 and Lv-GPCR-B4 were clustered with the calcitonin-B receptor ortholog. Lv-GPCR-B2 was clustered with the parathyroid hormone receptor (PTH)-like receptor ortholog.
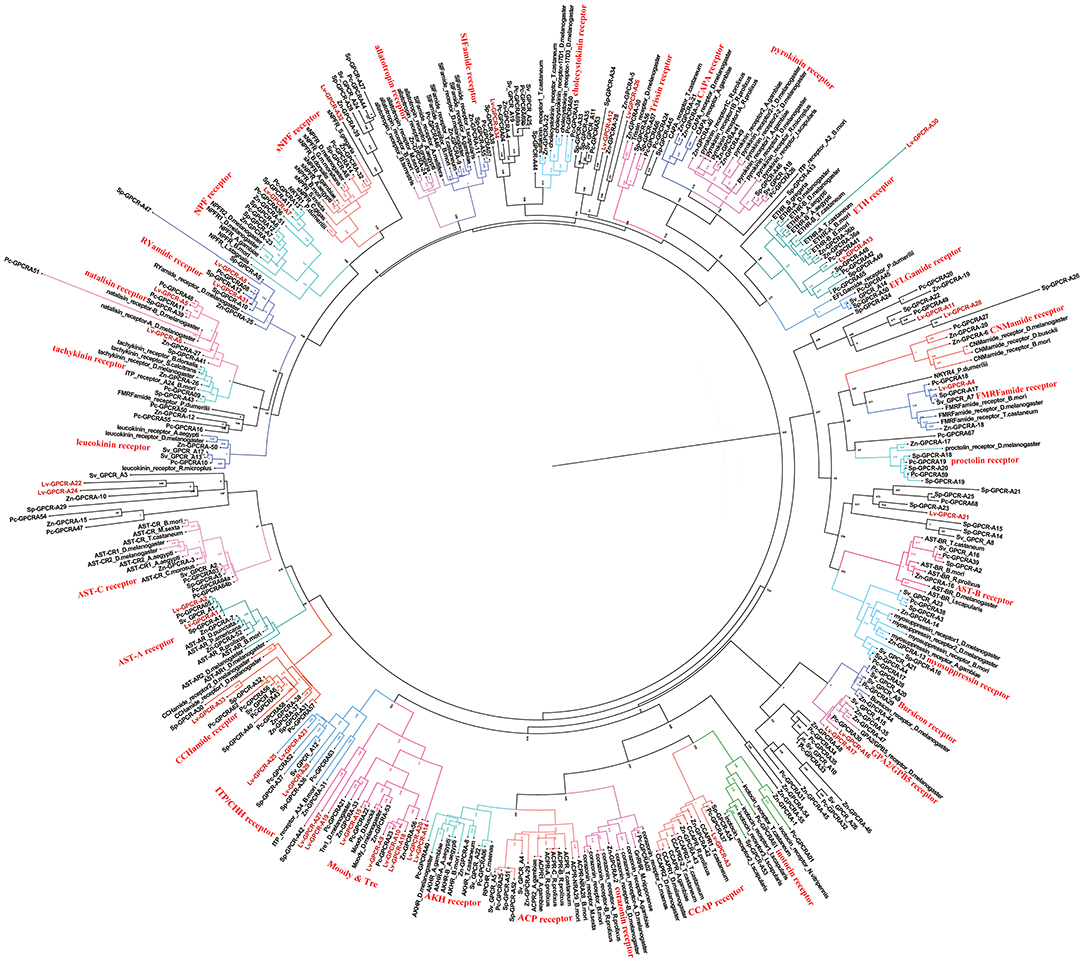
Figure 5. Phylogeny of the A-family peptide GPCRs. Cladogram of peptide GPCRs showing connections in the clustermap of A-family peptide receptors. Lv-GPCR, Lysmata vittata GPCR; Pc-GPCR, Procambarus clarkii GPCR; Sv-GPCR, Sagmariasus verreauxi GPCR; Sp-GPCR, Scylla paramamosain GPCR; Zn-GPCR, Zootermopsis nevadensis GPCR.
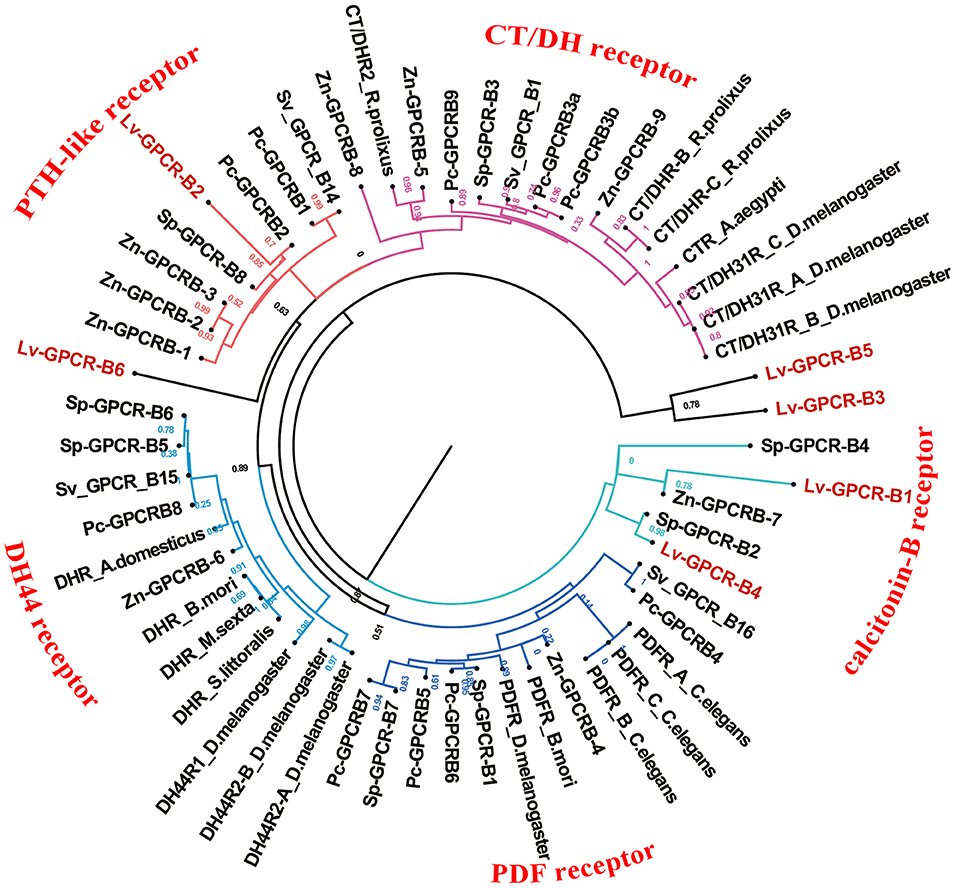
Figure 6. Phylogeny of the B-family peptide GPCRs. Cladogram of peptide GPCRs depicting connections in the clustermap of B-family peptide receptors. Lv-GPCR, Lysmata vittata GPCR; Pc-GPCR, Procambarus clarkii GPCR; Sv-GPCR, Sagmariasus verreauxi GPCR; Sp-GPCR, Scylla paramamosain GPCR; Zn-GPCR, Zootermopsis nevadensis GPCR.
Discussion
The RNA-seq and bioinformatics analysis is highly effective for identifying peptides and their GPCRs (23, 26, 28, 37, 43, 58, 59). In this study, 61 transcripts derived from 44 peptide families and 40 transcripts derive from 13 peptide GPCRs were identified. This is the first study on genus Lysmata to characterize its peptide repertoire and their GPCRs.
Comparison of Peptide Sequences
Considering that the L. vittata peptide transcripts were computationally-generated from short reads and the mature peptide structures were predicted based on a bioinformatics workflow, the peptide repertoire from one Caridean shrimp, M. rosenbergii reported before was chosen for sequence comparison to provide increased confidence of the L. vittata peptide sequences/structures reported in present study (43, 50). Overall, sequence alignments of the predicted peptide amino acid sequences in L. vittata and full-length M. rosenbergii peptide precursors show that all peptides especially the mature peptides are conserved in the two species (Supplementary File 2). For example, the predicted mature peptides of calcitonin (TCYINAGLSHGCDYKDLVGAMAEKNYWDSLNSPamide), DH31 (GLDLGLGRGFSGSQAAKHLMGLAAANFAGGPamide), EH (ASITSMCIRNCGQCKEMYGDYFHGQACAESCIMTQGVSIPDCNNPATFNRFL), and a large number of relative short mature peptides (e.g., PQHYAFGLamide from AST-A, ADWSSMRGTWamide from AST-B, QIRYHQCYNPISCF from AST-C, DAGHFFAETPKHLPRIamide from ETH) from L. vittata are identical in amino acid sequences/structures to the corresponding mature peptides reported in M. rosenbergii. This suggests the putative peptide sequences in our study are mostly accurate.
In addition to the reported peptides data from M. rosenbergii, the peptide repertoire from another Lysmata species, a marine shrimp Lysmata wurdemanni, was chosen for sequence comparison. The L. wurdemanni peptide sequences from the only public Lysmata transcriptome data, i.e., the L. wurdemanni embryo and adult (brain and muscle) Sequence Read Archive (SRA) (SRR8715485, SRR8715486), was downloaded for peptide mining based on our bioinformatics workflow. A total of 78 transcripts derived from 45 peptide families were predicted from L. wurdemanni, mainly significant amino acid similar to those from L. vittata (Supplementary File 2). A large number of peptide families were identified from the two Lysmata shrimps, but a number of established peptide groups were not identified in each shrimp. No sequences encoding CNMamide, corazonin, elevenin, HanSolin, HIGSLYRamide, or RFLamide proteins were found in the L. vittata assembly. Similarly, no sequences encoding Bursicon, ETH, HanSolin, HIGSLYRamide, Hyrg, IAG, or RFLamide proteins were found in the L. wurdemanni assembly. Given the significant peptide sequence resemblance between the two Lysmata shrimps, we hypothesize that CNMamide, corazonin, and elevenin are likely to be found in L. vittata. On the other hand, Bursicon, ETH and IAG are likely to be found in L. wurdemanni. The hypothesis was verified when L. wurdemanni IAG was cloned from the testicular part of L. wurdemanni (60). In contrast, HanSolin, HIGSLYRamide and RFLamide families are likely to be absent in L. vittata, as these peptide families have not been identified from the current Caridean shrimps peptide data (43, 50, 61). Of which, HanSolin and RFLamide are recently-identified peptides from the stick insect, Carausius morosus (62), and subsequently were found in most Coleoptera species (63), and HIGSLYRamide have been identified only from brachyuran [e.g., (23, 40, 41)]. Therefore, additional transcriptome, peptidome, and/or a genome data can be created to clarify the presence/absence of these peptide families in L. vittata.
Peptides/GPCRs Expression
Given that the experimental design of each development stage single libraries comprised of pooled RNA samples, we did not investigate expression changes statistically. However, it is possible that the expression values presented provides valuable preliminary data to identify candidates for prospective study on PSH species peptides. Similar to expression patterns of previously reported peptides/GPCRs (23, 64–66), stage-specific expression of peptides/peptide GPCRs existed in L. vittata, i.e., TPM values of peptides/peptide GPCR transcripts in MP are higher than in EP. In L. vittata MP, higher expression levels of the peptidergic signaling promotes the growth of body size and development of testicular part of the gonad. For instance, NPFs, sNPF and IAGs have higher expression in MP, where NPF and sNPF were proved as the feeding behavior controllers in arthropod (67), and NPF has been shown to increase food intake in penaeid shrimp (68). Similar functions of NPF and sNPF are proposed in L. vittata. IAG is regarded as the regulator of male sex differentiation in crustaceans (2–4), and high IAG expression in MP suggest that IAG might be involved in promoting masculinization and developing testicular part of the gonad in L. vittata. In contrast, a number of peptides show different expression patterns, e.g., TPM values of two PDH transcripts in MP are lower than those in EP. PDH regulates pigment distribution controlling circadian rhythm (69), and also shows different expression levels during the mud crab Scylla paramamosain vitellogenesis (6), but the reproductive function of PDH is yet to be proved in crustaceans. TAP shows high expression in both MP and EP, i.e., TPM value of MP and EP is 24.3 and 29.9, respectively. In M. rosenbergii, TAP was distributed on terminal ampullae and sperms, as it participates in sperm proteolytic activity and plays a key role in sperm maturation (56). We hypothesized that TAP play similar functional roles in L. vittata sperm, in gonad of both MP and EP (15). Moreover, TPM values of neuroparsins and RPCH are higher than 15 in MP. They play a role in regulation in crustacean ovarian development (7, 8). These results imply that transcriptomes of different L. vittata gonadal stages should be analyzed to assess reproductive differences in peptidergic signaling in PSH species.
Key Peptides
Multiple members of the CHH superfamily contain 6 aligned cysteine residues, and nine transcripts of these peptides were found in this study. Given that CHH1-4 some regions in this gene have the same sequences, we speculated they are from different alternative splicing of one gDNA, and this phenomenon is common in type-I CHHs (1, 70). The C-terminal motif “AIAXX” of CHH5 and CHH6, members of type-I CHHs, seems to be more common in Caridean shrimps than other crustaceans. In type-II CHHs, MIH, and GIH show high similarity in sequences, with MIH participating in molting and GIH regulating reproduction process. These genes have been subdivided based on primary structures and motifs (71). However, this rule does not apply for type-II CHHs in Caridea, making it difficult to subdivide MIH and GIH based on primary structures in this species. Therefore, we named two L. vittata type-II CHHs as “MIH/GIH”. These L. vittata MIH/GIHs were clustered into two subgroups, in which MIH/GIH2 was grouped with M. nipponense GIH. Type-II CHH was reported to inhibit ovary development (72). This suggests that MIH/GIH2 could be GIH. MIH/GIH (MIH/GIH1) was grouped with the predicted M. nipponense MIH, implying that may be MIH. CHH-MIH-like peptides were clustered with some predicted shrimp ITPs, but it is not appropriate to name these peptides as “ITP,” because they neither have the typical CHH-PRP of ITP, nor are they similar to L. vannamei ITP, which modulate osmoregulation in shrimp (73).
Two unique mature peptides of IAGs were found in a species for the first time in this study. In the Chinese shrimp Fenneropenaeus chinensis, two IAG isoforms were identified from one gene (74). Three C. sapidus IAG genes were identified from AG, hepatopancreas and ovary. However, mature peptides of these genes are identical (75–77). Only one IAG was cloned from L. wurdemanni, and this IAG showed higher sequence similarity with those of the genus Macrobrachium than with the two L. vittata IAGs (60). In this study, expression levels of two IAGs in MP shrimp were higher than in EP shrimp, of which, the TPM value of IAG1 was 76 in MP shrimp, but not expressed in EP shrimp. In L. vittata, it was reported that the male external sexual character had disappeared whereas testicular part had some degree of degeneration in EP shrimp (15), of which were ascribed to the down-regulation of IAGs. In M. rosenbergii, silencing of IAG not only arrested the degeneration of male secondary sexual characteristics and testis, but also prevented testicular spermatogenesis (2). M. rosenbergii IAG dsRNA injections canceled spermatozoa in the sperm duct and testis (2). In L. vittata EP, both ovarian and testicular parts were mature, testicular part being filled with many spermatozoa (15). It seems that spermatogenesis was unaffected following down-regulation of IAGs in L. vittata.
In many species, including in L. vittata, CFSH has one to three paralog genes (43). In this study, two CFSH transcripts were almost not expressed in EP, which is the ovarian mature stage. In female crabs with CFSH knock-down, the brooding and mating systems were abnormal at puberty (5). Analysis of expression pattern of L. vittata CFSH revealed that it might be involved in the development of female phenotypes at puberty, rather than the vitellogenesis and ovarian maturation.
Two sexual systems, two sex differentiation hormones, and two unigenes of these hormones exist in an individual, implying that a complicated sexual regulatory network exists in L. vittata. RNAi should be performed to reveal the discover bisexual mechanism regulated by sex differentiation hormones in L. vittata. This species is expected to be an ideal model for RNAi experiments because it is small, has a transparent body and short reproductive cycle (22).
Summary
Total RNA was extracted from L. vittata mixed tissues and used to mine peptides. More than 60 peptide transcripts were identified. However, this method has the following limitation, i.e., the expression levels of some tissue specific (e.g., eyestalk) peptides may be too low to assemble long enough transcript encoding complete precursor. Here, 15 peptide transcripts encoded incomplete precursors. Some of them showed low expression levels, such as CFSH1a, CHH3, GPA2, and RYamide. Furthermore, the peptides contained several similar peptide paracopies making it difficult to assemble the complete CDSs encoding such precursors (43), as in the cases of AST-A, kinin, and natalisin in our study. Notably, 28 predicted Lv-GPCRs were grouped with known peptide GPCRs, including: AST-A receptor, CCAP receptor, FMRFamide receptor, natalisin receptor, NPF receptor, RYamide receptor, Moody & Tre, ETH receptor, GPA2/GPB5 receptor, CHH receptors, CCHamide receptor, calcitonin-B receptor, and PTH-like receptor. Together with the identified peptides in L. vittata, we speculate that AST-A, FLRFamide, natalisin, NPF, RYamide, ETH, GPA2/GPB5, CHHs, CCHamide, and calcitonin ligand-receptor pairs are expressed in L. vittata. In conclusion, complete peptide/GPCR genes remain to be cloned and confirmed through PCR experiments. Moreover, in vitro ligand-receptor binding tests are required to determine Lv-GPCRs. Nevertheless, identification of peptides and the associated GPCRs in L. vittata extends our knowledge on peptidergic signaling in PSH species, and provides experimental basis for further studies on the of function peptides in reproduction. This will promote aquaculture development of this shrimp.
Data Availability Statement
The datasets generated for this study can be found in the SRA accession: PRJNA561673.
Ethics Statement
The study was approved by Xiamen University animal care committee.
Author Contributions
CB: conceptualization, data curation, software, writing-original draft. FL: Sample collection, data curation. YY: project administration, editing. QL: sample collection. HY: conceptualization, editing, supervision.
Conflict of Interest
The authors declare that the research was conducted in the absence of any commercial or financial relationships that could be construed as a potential conflict of interest.
Acknowledgments
This study was supported by the special fund of marine and fisheries structure adjustment in Fujian (No. 2017HYJG03), the National Natural Science Foundation of China (No. 31902350) and the K. C. Wong Magna Fund in Ningbo University. We wish to thank the staff of Majorbio (Shanghai, China) for performing Illumina sequencing.
Supplementary Material
The Supplementary Material for this article can be found online at: https://www.frontiersin.org/articles/10.3389/fendo.2020.00226/full#supplementary-material
Supplementary File 1. Peptide precursors and their expression levels in male phase (MP) and euhermaphrodite phase (EP) of L. vittata.
Supplementary File 2. Comparative sequence alignment of peptide precursor in L. vittata with M. rosenbergii and L. wurdemanni.
Table S1. Supplementary information.
References
1. Webster SG, Keller R, Dircksen H. The CHH-superfamily of multifunctional peptide hormones controlling crustacean metabolism, osmoregulation, moulting, and reproduction. Gen Comp Endocrinol. (2012) 175:217–33. doi: 10.1016/j.ygcen.2011.11.035
2. Ventura T, Manor R, Aflalo ED, Weil S, Raviv S, Glazer L, et al. Temporal silencing of an androgenic gland-specific insulin-like gene affecting phenotypical gender differences and spermatogenesis. Endocrinology. (2008) 150:1278–86. doi: 10.1210/en.2008-0906
3. Rosen O, Manor R, Weil S, Gafni O, Linial A, Aflalo ED, et al. A sexual shift induced by silencing of a single insulin-like gene in crayfish: ovarian upregulation and testicular degeneration. PLoS ONE. (2010) 5:e15281. doi: 10.1371/journal.pone.0015281
4. Ventura T, Sagi A. The insulin-like androgenic gland hormone in crustaceans: From a single gene silencing to a wide array of sexual manipulation-based biotechnologies. Biotechnol Adv. (2012) 30:1543–50. doi: 10.1016/j.biotechadv.2012.04.008
5. Zmora N, Chung JS. A novel hormone is required for the development of reproductive phenotypes in adult female crabs. Endocrinology. (2014) 155:230–9. doi: 10.1210/en.2013-1603
6. Huang X, Ye H, Huang H, Yu K, Huang Y. Two beta-pigment-dispersing hormone (β-PDH) isoforms in the mud crab, Scylla paramamosain: implication for regulation of ovarian maturation and a photoperiod-related daily rhythmicity. Anim Reprod Sci. (2014) 150:139–47. doi: 10.1016/j.anireprosci.2014.09.004
7. Yang SP, He JG, Sun CB, Chan SF. Characterization of the shrimp neuroparsin (MeNPLP): RNAi silencing resulted in inhibition of vitellogenesis. FEBS open bio. (2014) 4:976–86. doi: 10.1016/j.fob.2014.09.005
8. Zeng H, Bao C, Huang H, Ye H, Li S. The mechanism of regulation of ovarian maturation by red pigment concentrating hormone in the mud crab Scylla paramamosain. Anim Reprod Sci. (2016) 164:152–61. doi: 10.1016/j.anireprosci.2015.11.025
9. Tinikul Y, Engsusophon A, Kruangkum T, Thongrod S, Tinikul R, Sobhon P. Neuropeptide F stimulates ovarian development and spawning in the female giant freshwater prawn, Macrobrachium rosenbergii, and its expression in the ovary during ovarian maturation cycle. Aquaculture. (2017) 469:128–36. doi: 10.1016/j.aquaculture.2016.11.026
10. Bao C, Yang Y, Huang H, Ye H. Inhibitory role of the mud crab short neuropeptide F in vitellogenesis and oocyte maturation via autocrine/paracrine signaling. Front Endocrinol. (2018) 9:390. doi: 10.3389/fendo.2018.00390
11. Liu A, Liu F, Shi W, Huang H, Wang G, Ye H. C-type allatostatin and its putative receptor from the mud crab serve an inhibitory role in ovarian development. J Exp Biol. (2019) 2019:222. doi: 10.1242/jeb.207985
12. Almeida AS, Barros-Alves SP, Hirose GL, Alves DFR. Reproductive output of the ornamental shrimp Lysmata vittata (Stimpson, 1860) (Decapoda: Caridea) in wild populations and under different maturation diets. Invertebr Reprod Dev. (2018) 62:257–267. doi: 10.1080/07924259.2018.1509903
13. Bauer RT. Simultaneous hermaphroditism in caridean shrimps: a unique and puzzling sexual system in the Decapoda. J Crustac Biol. (2000) 20:116–28. doi: 10.1163/1937240X-90000014
14. Bauer RT, Newman WA. Protandric simultaneous hermaphroditism in the marine shrimp Lysmata californica (Caridea: Hippolytidae). J Crustac Biol. (2004) 24:131–9. doi: 10.1651/C-2426
15. Chen D, Liu F, Zhu Z, Lin Q, Zeng C, Ye H. Ontogenetic development of gonads and external sexual characters of the protandric simultaneous hermaphrodite peppermint shrimp, Lysmata vittata (Caridea: Hippolytidae). PLoS ONE. (2019) 14:e0215406. doi: 10.1371/journal.pone.0215406
16. Chace FA Jr. The Caridean shrimps (Crustacea: Decapoda) of the Albatross Philippine Expedition, 1907-1910, Part 7: Families Atyidae, Eugonatonotidae, Rhynchocinetidae, Bathypalaemonidae, Processidae, and Hippolytidae. Smithsonian Contributions to Zoology. (1997) 587:1–106.
17. Marin IN, Korn OM, Kornienko ES. The caridean shrimp Lysmata vittata (Stimpson, 1860) (Decapoda: Hippolytidae): A new species for the fauna of Russia. Russ J Mar Biol. (2012) 38:359–363. doi: 10.1134/S1063074012040062
18. Marin I, Korn OM, Kornienko ES. On the presence of the caridean shrimp Lysmata vittata (Decapoda, Hippolytidae) in the Russian waters of the Sea of Japan. Crustaceana. (2012) 85:123. doi: 10.1163/156854012X623548
19. Wang YR, Sha ZL. Description of two new species of Lysmata Risso, 1816 (Decapoda, Lysmatidae) from the China seas, with remarks on Lysmata vittata (Stimpson 1860). Zootaxa. (2018) 4392:28–40. doi: 10.11646/zootaxa.4392.1.2
20. Alves DFR, Lima DJM, Hirose GL, Martinez PA, Dolabella SS, Barros-Alves SP. Morphological and molecular analyses confirm the occurrence of two sympatric Lysmata shrimp (Crustacea, Decapoda) in the southwestern Atlantic. Zootaxa. (2018) 4526:41–55. doi: 10.11646/zootaxa.4526.1.3
21. Soledade GO, Baeza JA, Boehs G, Simões SM, Santos PS, Costa RC, et al. A precautionary tale when describing species in a world of invaders: Morphology, coloration and genetics demonstrate that Lysmata rauli is not a new species endemic to Brazil but a junior synonym of the Indo-Pacific L. vittata. J Crustac Biol. (2013) 33:66–77. doi: 10.1163/1937240X-00002122
22. Alves DFR, Greco LSL, de Paiva Barros-Alves S, Hirose GL. Sexual system, reproductive cycle and embryonic development of the red-striped shrimp Lysmata vittata, an invader in the western Atlantic Ocean. PLoS ONE. (2019) 14:e0210723. doi: 10.1371/journal.pone.0210723
23. Bao C, Yang Y, Huang H, Ye H. Neuropeptides in the cerebral ganglia of the mud crab, Scylla paramamosain: transcriptomic analysis and expression profiles during vitellogenesis. Sci Rep. (2015) 5:17055. doi: 10.1038/srep17055
24. Veenstra JA. Mono-and dibasic proteolytic cleavage sites in insect neuroendocrine peptide precursors. Arch Insect Biochem. (2000) 43:49–63. doi: 10.1002/(SICI)1520-6327(200002)43:2<49::AID-ARCH1>3.0.CO;2-M
25. Li L, Kelley WP, Billimoria CP, Christie AE, Pulver SR, Sweedler JV, et al. Mass spectrometric investigation of the neuropeptide complement and release in the pericardial organs of the crab, Cancer borealis. J Neurochem. (2003) 87:642–56. doi: 10.1046/j.1471-4159.2003.02031.x
26. Dircksen H, Neupert S, Predel R, Verleyen P, Huybrechts J, Strauss J, et al. Genomics, transcriptomics, and peptidomics of Daphnia pulex neuropeptides and protein hormones. J Proteome Res. (2011) 10:4478–504. doi: 10.1021/pr200284e
27. Derst C, Dircksen H, Meusemann K, Zhou X, Liu S, Predel R. Evolution of neuropeptides in non-pterygote hexapods. BMC Evol Biol. (2016) 16:51. doi: 10.1186/s12862-016-0621-4
28. Bao C, Yang Y, Zeng C, Huang H, Ye H. Identifying neuropeptide GPCRs in the mud crab, Scylla paramamosain, by combinatorial bioinformatics analysis. Gen Comp Endocrinol. (2018) 269:122–30. doi: 10.1016/j.ygcen.2018.09.002
29. Alexander JL, Oliphant A, Wilcockson DC, Audsley N, Down RE, Lafont R, et al. Functional characterization and signalling systems of corazonin and red pigment concentrating hormone in the green shore crab, Carcinus maenas. Front Neurosci. (2017) 11:752. doi: 10.3389/fnins.2017.00752
30. Buckley SJ, Fitzgibbon QP, Smith GG, Ventura T. In silico prediction of the G-protein coupled receptors expressed during the metamorphic molt of Sagmariasus verreauxi (Crustacea: Decapoda) by mining transcriptomic data: RNA-seq to repertoire. Gen Comp Endocrinol. (2016) 228:111–27. doi: 10.1016/j.ygcen.2016.02.001
31. Bauknecht P, Jékely G. Large-scale combinatorial deorphanization of Platynereis neuropeptide GPCRs. Cell Rep. (2015) 12:684–93. doi: 10.1016/j.celrep.2015.06.052
32. Caers J, Verlinden H, Zels S, Vandersmissen HP, Vuerinckx K, Schoofs L. More than two decades of research on insect neuropeptide GPCRs: an overview. Front Endocrinol. (2012) 3:151. doi: 10.3389/fendo.2012.00151
33. Du Y, Ma K, Qiu G. Discovery of the genes in putative GnRH signaling pathway with focus on characterization of GnRH-like receptor transcripts in the brain and ovary of the oriental river prawn Macrobrachium nipponense. Aquaculture. (2015) 442:1–11. doi: 10.1016/j.aquaculture.2015.02.016
34. Frooninckx L, Van Rompay L, Temmerman L, Van Sinay E, Beets I, Janssen T, et al. Neuropeptide GPCRs in C. elegans. Front Endocrinol. (2012) 3:167. doi: 10.3389/fendo.2012.00167
35. Thiel D, Bauknecht P, Jékely G, Hejnol A. An ancient FMRFamide-related peptide–receptor pair induces defence behaviour in a brachiopod larva. Open Biol. (2017) 7:170136. doi: 10.1098/rsob.170136
36. Veenstra JA. The contribution of the genomes of a termite and a locust to our understanding of insect neuropeptides and neurohormones. Front Physiol. (2014) 5:454. doi: 10.3389/fphys.2014.00454
37. Veenstra JA. The power of next-generation sequencing as illustrated by the neuropeptidome of the crayfish Procambarus clarkii. Gen Comp Endocrinol. (2015) 224:84–95. doi: 10.1016/j.ygcen.2015.06.013
38. Beitz E. TeXshade: shading and labeling of multiple sequence alignments using LaTeX2e. Bioinformatics. (2000) 16:135–9. doi: 10.1093/bioinformatics/16.2.135
39. Gouy M, Guindon S, Gascuel O. SeaView version 4: a multiplatform graphical user interface for sequence alignment and phylogenetic tree building. Mol Biol Evol. (2009) 27:221–4. doi: 10.1093/molbev/msp259
40. Christie AE. Prediction of Scylla olivacea (Crustacea; Brachyura) peptide hormones using publicly accessible transcriptome shotgun assembly (TSA) sequences. Gen Comp Endocrinol. (2016) 230–231:1–16. doi: 10.1016/j.ygcen.2016.03.008
41. Christie AE. Expansion of the neuropeptidome of the globally invasive marine crab Carcinus maenas. Gen Comp Endocrinol. (2016) 235:150–69. doi: 10.1016/j.ygcen.2016.05.013
42. Christie AE, Chi M. Prediction of the neuropeptidomes of members of the Astacidea (Crustacea, Decapoda) using publicly accessible transcriptome shotgun assembly (TSA) sequence data. Gen Comp Endocrinol. (2015) 224:38–60. doi: 10.1016/j.ygcen.2015.06.001
43. Veenstra JA. Similarities between decapod and insect neuropeptidomes. PeerJ. (2016) 4:e2043. doi: 10.7717/peerj.2043
44. Sturm S, Ramesh D, Brockmann A, Neupert S, Predel R. Agatoxin-like peptides in the neuroendocrine system of the honey bee and other insects. J Proteomics. (2016) 132:77–84. doi: 10.1016/j.jprot.2015.11.021
45. Chistie AE, Stemmler EA, Dickinson PS. Crustacean neuropeptides. Cell Mol Life Sci. (2010) 67:4135–69. doi: 10.1007/s00018-010-0482-8
46. Christie AE, Chi M, Lameyer TJ, Pascual MG, Shea DN, Stanhope ME, et al. Neuropeptidergic signaling in the American lobster Homarus americanus: new insights from high-throughput nucleotide sequencing. PLoS ONE. (2015) 10:e0145964. doi: 10.1371/journal.pone.0145964
47. Dickinson PS, Wiwatpanit T, Gabranski ER, Ackerman RJ, Stevens JS, Cashman CR, et al. Identification of SYWKQCAFNAVSCFamide: a broadly conserved crustacean C-type allatostatin-like peptide with both neuromodulatory and cardioactive properties. J Exp Biol. (2009) 212:1140–52. doi: 10.1242/jeb.028621
48. Ma M, Szabo TM, Jia C, Marder E, Li L. Mass spectrometric characterization and physiological actions of novel crustacean C-type allatostatins. Peptides. (2009) 30:1660–8. doi: 10.1016/j.peptides.2009.05.023
49. Stemmler EA, Bruns EA, Cashman CR, Dickinson PS, Christie AE. Molecular and mass spectral identification of the broadly conserved decapod crustacean neuropeptide pQIRYHQCYFNPISCF: the first PISCF-allatostatin (Manduca sexta- or C-type allatostatin) from a non-insect. Gen Comp Endocrinol. (2010) 165:1–10. doi: 10.1016/j.ygcen.2009.05.010
50. Suwansa-Ard S, Thongbuakaew T, Wang T, Zhao M, Elizur A, Hanna PJ, et al. In silico neuropeptidome of female Macrobrachium rosenbergii based on transcriptome and peptide mining of eyestalk, central nervous system and ovary. PLoS ONE. (2015) 10:e0123848. doi: 10.1371/journal.pone.0123848
51. Hansen KK. Hauser F, Williamson M, Weber SB, Grimmelikhuijzen CJ. The Drosophila genes CG14593 and CG30106 code for G-protein-coupled receptors specifically activated by the neuropeptides CCHamide-1 and CCHamide-2. Biochem Biophys Res Commun. (2011) 404:184–189. doi: 10.1016/j.bbrc.2010.11.089
52. Christie AE. Prediction of the peptidomes of Tigriopus californicus and Lepeophtheirus salmonis (Copepoda, Crustacea). Gen Comp Endocrinol. (2014) 201:87–106. doi: 10.1016/j.ygcen.2014.02.015
53. Nguyen TV, Cummins SF, Elizur A, Ventura T. Transcriptomic characterization and curation of candidate neuropeptides regulating reproduction in the eyestalk ganglia of the Australian crayfish, Cherax quadricarinatus. Sci Rep. (2016) 6:38658. doi: 10.1038/srep38658
54. Ma M, Gard AL, Xiang F, Wang J, Davoodian N, Lenz PH, et al. Combining in silico transcriptome mining and biological mass spectrometry for neuropeptide discovery in the Pacific white shrimp Litopenaeus vannamei. Peptides. (2010) 31:27–43. doi: 10.1016/j.peptides.2009.10.007
55. Ohira T, Tsutsui N, Kawazoe I, Wilder MN. Isolation and characterization of two pigment-dispersing hormones from the whiteleg shrimp, Litopenaeus vannamei. Zool Sci. (2006) 23:601–6. doi: 10.2108/zsj.23.601
56. Ma WM, Qian YQ, Wang MR, Yang F, Yang WJ. A novel terminal ampullae peptide is involved in the proteolytic activity of sperm in the prawn, Macrobrachium rosenbergii. Reproduction. (2010) 140:235–45. doi: 10.1530/REP-10-0062
57. Nagai C, Mabashi-Asazuma H, Nagasawa H, Nagata S. Identification and characterization of receptors for ion transport peptide (ITP) and ITP-like (ITPL) in the silkworm Bombyx mori. J Biol Chem. (2014) 289:32166–77. doi: 10.1074/jbc.M114.590646
58. Christie AE, Yu A. Identification of peptide hormones and their cognate receptors in Jasus edwardsii–A potential resource for the development of new aquaculture management strategies for rock/spiny lobsters. Aquaculture. (2019) 503:636–62. doi: 10.1016/j.aquaculture.2018.11.059
59. Nguyen TV, Rotllant GE, Cummins SF, Elizur A, Ventura T. Insights into sexual maturation and reproduction in the norway lobster (ephrops norvegicus) via in silico prediction and characterization of neuropeptides and G protein-coupled receptors. Front Endocrinol. (2018) 9:430. doi: 10.3389/fendo.2018.00430
60. Zhang D, Sun M, Liu X. Phase-specific expression of an insulin-like androgenic gland factor in a marine shrimp Lysmata wurdemanni: implication for maintaining protandric simultaneous hermaphroditism. PLoS ONE. (2017) 12:e0172782. doi: 10.1371/journal.pone.0172782
61. Toullec JY, Corre E, Mandon P, Gonzalez-Aravena M, Ollivaux C, Lee CY. Characterization of the neuropeptidome of a Southern Ocean decapod, the Antarctic shrimp Chorismus antarcticus: Focusing on a new decapod ITP-like peptide belonging to the CHH peptide family. Gen Comp Endocrinol. (2017) 252:60–78. doi: 10.1016/j.ygcen.2017.07.015
62. Liessem S, Ragionieri L, Neupert S, Büschges A, Predel R. Transcriptomic and neuropeptidomic analysis of the stick insect, Carausius morosus. J Proteome Res. (2018) 17:2192–204. doi: 10.1021/acs.jproteome.8b00155
63. Veenstra JA. Coleoptera genome and transcriptome sequences reveal numerous differences in neuropeptide signaling between species. PeerJ. (2019) 7:e7144. doi: 10.7717/peerj.7144
64. Christie AE, Roncalli V, Wu LS, Ganote CL, Doak T, Lenz PH. Peptidergic signaling in Calanus finmarchicus (Crustacea, Copepoda): in silico identification of putative peptide hormones and their receptors using a de novo assembled transcriptome. Gen Comp Endocrinol. (2013) 187:117–35. doi: 10.1016/j.ygcen.2013.03.018
65. Lee SS, Ding Y, Karapetians N, Rivera-Perez C, Noriega FG, Adams ME. Hormonal signaling cascade during an early-adult critical period required for courtship memory retention in Drosophila. Curr Biol. (2017) 27:2798–809. doi: 10.1016/j.cub.2017.08.017
66. Oliphant A, Hawkes MK, Cridge AG, Dearden PK. Transcriptomic characterisation of neuropeptides and their putative cognate G protein-coupled receptors during late embryo and stage-1 juvenile development of the Aotearoa-New Zealand crayfish, Paranephrops zealandicus. Gen Comp Endocrinol. (2020) 2020:113443. doi: 10.1016/j.ygcen.2020.113443
67. Nässel DR, Wegener C. A comparative review of short and long neuropeptide F signaling in invertebrates: any similarities to vertebrate neuropeptide Y signaling? Peptides. (2011) 32:1335–55. doi: 10.1016/j.peptides.2011.03.013
68. Christie AE, Chapline MC, Jackson JM, Dowda JK, Hartline N, Malecha SR, et al. Identification, tissue distribution and orexigenic activity of neuropeptide F (NPF) in penaeid shrimp. J Exp Biol. (2011) 214:1386–96. doi: 10.1242/jeb.053173
69. Fernlund P. Structure of a light-adapting hormone from the shrimp, Pandalus borealis. BBA-Protein Struct. (1976) 439:17–25. doi: 10.1016/0005-2795(76)90155-0
70. Jeon JM, Kim BK, Lee JH, Kim HJ, Kang CK, Mykles DL, et al. Two type I crustacean hyperglycemic hormone (CHH) genes in Morotoge shrimp (Pandalopsis japonica): cloning and expression of eyestalk and pericardial organ isoforms produced by alternative splicing and a novel type I CHH with predicted structure shared with type II CHH peptides. Comp Biochem Phys B. (2012) 162:88–99. doi: 10.1016/j.cbpb.2012.04.003
71. Lacombe C, Greve P, Martin G. Overview on the sub-grouping of the crustacean hyperglycemic hormone family. Neuropeptides. (1999) 33:71–80. doi: 10.1054/npep.1999.0016
72. Qiao H, Xiong Y, Zhang W, Fu H, Jiang S, Sun S, et al. Characterization, expression, and function analysis of gonad-inhibiting hormone in Oriental River prawn, Macrobrachium nipponense and its induced expression by temperature. Comp Biochem Phys A. (2015) 185:1–8. doi: 10.1016/j.cbpa.2015.03.005
73. Tiu SH, He JG, Chan SM. The LvCHH-ITP gene of the shrimp (Litopenaeus vannamei) produces a widely expressed putative ion transport peptide (LvITP) for osmo-regulation. Gene. (2007) 396:226–35. doi: 10.1016/j.gene.2007.02.027
74. Li S, Li F, Sun Z, Xiang J. Two spliced variants of insulin-like androgenic gland hormone gene in the Chinese shrimp, Fenneropenaeus chinensis. Gen Comp Endocrinol. (2012) 177:246–55. doi: 10.1016/j.ygcen.2012.04.010
75. Chung JS. An insulin-like growth factor found in hepatopancreas implicates carbohydrate metabolism of the blue crab Callinectes sapidus. Gen Comp Endocrinol. (2014) 199:56–64. doi: 10.1016/j.ygcen.2014.01.012
76. Chung JS, Manor R, Sagi A. Cloning of an insulin-like androgenic gland factor (IAG) from the blue crab, Callinectes sapidus: implications for eyestalk regulation of IAG expression. Gen Comp Endocrinol. (2011) 173:4–10. doi: 10.1016/j.ygcen.2011.04.017
77. Huang X, Ye H, Chung JS. The presence of an insulin-like androgenic gland factor (IAG) and insulin-like peptide binding protein (ILPBP) in the ovary of the blue crab, Callinectes sapidus and their roles in ovarian development. Gen Comp Endocrinol. (2017) 249:64–70. doi: 10.1016/j.ygcen.2017.05.001
Keywords: peptides, GPCRs, bioinformatics, protandric simultaneous hermaphrodite, Lysmata vittata
Citation: Bao C, Liu F, Yang Y, Lin Q and Ye H (2020) Identification of Peptides and Their GPCRs in the Peppermint Shrimp Lysmata vittata, a Protandric Simultaneous Hermaphrodite Species. Front. Endocrinol. 11:226. doi: 10.3389/fendo.2020.00226
Received: 12 December 2019; Accepted: 30 March 2020;
Published: 30 April 2020.
Edited by:
Honoo Satake, Suntory Foundation for Life Sciences, JapanCopyright © 2020 Bao, Liu, Yang, Lin and Ye. This is an open-access article distributed under the terms of the Creative Commons Attribution License (CC BY). The use, distribution or reproduction in other forums is permitted, provided the original author(s) and the copyright owner(s) are credited and that the original publication in this journal is cited, in accordance with accepted academic practice. No use, distribution or reproduction is permitted which does not comply with these terms.
*Correspondence: Haihui Ye, aGFpaHVpeWUmI3gwMDA0MDt4bXUuZWR1LmNu