- School of Biological Sciences, The University of Hong Kong, Hong Kong, China
In fish models, seasonal change in feeding is under the influence of water temperature. However, the effects of temperature on appetite control can vary among fish species and the mechanisms involved have not been fully characterized. Using goldfish (Carassius auratus) as a model, seasonal changes in feeding behavior and food intake were examined in cyprinid species. In our study, foraging activity and food consumption in goldfish were found to be reduced with positive correlation to the gradual drop in water temperature occurring during the transition from summer (28.4 ± 2.2°C) to winter (15.1 ± 2.6°C). In goldfish with a 4-week acclimation at 28°C, their foraging activity and food consumption were notably higher than their counterparts with similar acclimation at 15°C. When compared to the group at 28°C during summer, the attenuation in feeding responses at 15°C during the winter also occurred with parallel rises of leptin I and II mRNA levels in the liver. Meanwhile, a drop in orexin mRNA along with concurrent elevations of CCK, MCH, POMC, CART, and leptin receptor (LepR) transcript expression could be noted in brain areas involved in feeding control. In short-term study, goldfish acclimated at 28°C were exposed to 15°C for 24 h and the treatment was effective in reducing foraging activity and food intake. The opposite was true in reciprocal experiment with a rise in water temperature to 28°C for goldfish acclimated at 15°C. In parallel time-course study with lowering of water temperature from 28 to 15°C, short-term exposure (6–12 h) of goldfish to 15°C could also increase leptin I and II mRNA levels in the liver. Similar to our seasonality study, transcript level of orexin was reduced along with up-regulation of CCK, MCH, POMC, CART, and LepR gene expression in different brain areas. Our results, as a whole, suggest that temperature-driven regulation of leptin output from the liver in conjunction with parallel modulations of orexigenic/anorexigenic signals and leptin responsiveness in the brain may contribute to the seasonal changes of feeding behavior and food intake observed in goldfish.
Introduction
Temperature change in the environment is a key factor known to affect energy metabolism (1) and body growth in animals (2), and these modulatory effects are partly mediated via regulation of food intake (3). In fish models, circannual rhythm of feeding pattern and food intake has been reported, which is under the influence of environmental cues including seasonal change in water temperature (4). However, the effects of temperature on feeding can be quite variable in different fish species. In general, a rise in water temperature tends to increase food intake, e.g., in salmon (Salmo salar) (5), cod (Gadus morhua) (6), and flounder (Pleuronectes americanus) (7), which can be attributed to the metabolic demand of enhanced body growth caused by activation of the GH/IGF-I axis observed at high temperature (especially during summer) (8–10). Nevertheless, an increase in water temperature can also induce voluntary anorexia in fish species, e.g., in Atlantic salmon (Salmo salar), and the phenomenon may be caused by a drop in the peripheral stimulator for feeding, namely ghrelin, in systemic circulation (11). Although central expression of orexigenic/anorexigenic signals modified by temperature change has been documented in fish models, e.g., up-regulation of ghrelin in the brain of Chinese perch (Siniperca chuatsi) by temperature rise (12) and elevation of CART expression in the hypothalamus of Atlantic cod (Gadus morhua) by low temperature (6), a recent study in Arctic charr (Salvelinus alpinus) has revealed that the seasonal changes of NPY, AgRP, POMC, CART, and leptin expressed in brain areas involved in feeding control did not correlate with the annual cycle of feeding reported in the species (13). To date, no consensus has been reached regarding the functional role of orexigenic/anorexigenic signals within the central nervous system (CNS) in the circannual rhythm of feeding observed in fish species.
To unveil the mechanisms underlying temperature modulation of feeding in fish models and their functional implications in seasonal variations in feeding behavior and food intake, goldfish was used as the animal model for our study as (i) it is a representative of cyprinid species, the members of which are commercial fish with high market values in Asian countries, and (ii) the background information for feeding behaviors and appetite control are well-documented in the species (7). In the present study, we sought to address the questions on: (i) Whether the goldfish displays a seasonal change in feeding dependent on water temperature which can be reflected by alterations in feeding behavior and food intake? (ii) Can these feeding responses be induced by short-term and/or long-term manipulation of water temperature? (iii) Can the feeding responses caused by temperature change be explained by parallel modifications of orexigenic/anorexigenic signals expressed in the CNS or in periphery tissues (e.g., in the liver)? Using goldfish adapted to water temperature at different times of the year but maintained under a constant photoperiod, different types of feeding behaviors and food consumption were monitored over an 8-month period covering the transition from summer to winter and correlated to the corresponding change in water temperature. To confirm that the differences in feeding responses observed between the summer and winter months indeed were caused by temperature change, long-term and short-term exposure of goldfish to the “summer temperature” (28°C) and “winter temperature” (15°C) were performed to test if the treatment could mimic the seasonal changes in feeding. To elucidate the mechanisms for feeding control by temperature, parallel measurements of leptin I and II mRNA expression in the liver and transcript levels of NPY, AgRP, orexin, CART, POMC, CCK, MCH, and leptin receptor (LepR) in selected brain areas were also conducted. The results of our study have provided new information on the mechanisms for feeding control by temperature change in the environment which may contribute to the seasonal cycle of food intake observed in goldfish.
Materials and Methods
Animal Maintenance and Preparation Prior to Experiments
Goldfish (Carassius auratus) with body weight of 28–34 g were acquired from local pet stores and maintained at 20 ± 2°C in well-aerated 700 L tanks (a total of ~300 fish with 50–60 fish/tank in ×5 circular tanks with a diameter of 120 cm and water depth of 60 cm) under a 12-h dark:12-h light photoperiod with regular replacement of water at a rate of ~10% total volume every 48 h using a Gardena® C1060 automatic irrigation system (Gardena, Ulm, Germany). To minimize the influence of reproductive status on feeding, mixed sexes of goldfish during sexual regression were used in our studies. For seasonal change in feeding responses (including food consumption and different types of feeding behaviors), the fish were housed in 200 L tanks (with 20–25 fish/tank in ×4 replica tanks with the dimension of 73 × 60 × 60 cm) in a separate room open to the outside via ventilation vents (to allow for seasonal change in water temperature) but maintained under the same photoperiod setting. For monitoring of feeding responses in our seasonality study and long-term/short-term acclimation experiments to summer (28°C)/winter temperature (15°C), the fish were housed in 20 L “observation tanks” (2 fish/tank with the dimension of 35 × 25 × 20 cm and up to ×8 replica tanks per group for individual experiments) with temperature maintained (± 1°C) by a submerged heater and cooling coil linked with a PolyScience® thermal controller (Preston Industries Inc., Niles, IL). To study the feeding responses after temperature acclimation, goldfish were entrained for 14 days with a “one-meal-per-day” feeding schedule (14) (with food provision of 2% BW/fish at 10:00 a.m. using an automatic feeder) prior to the scoring of feeding behaviors/measurement of food intake. For the studies on target gene expression, goldfish were sacrificed by anesthesia with 50 mg/L MS222 (Sigma-Aldrich, St. Louis, MO) followed by spinosectomy according to the procedures approved by the Committee for Animal Use for Research and Teaching at the University of the Hong Kong.
Seasonal Change in Feeding and Its Correlation With Water Temperature
Seasonal changes in feeding behavior and food consumption were monitored in goldfish over a period of 8 months (Jul, 2016–Feb, 2017) covering the transition from summer to winter under a 12-h dark:12-h light photoperiod. The period from July to Sept of 2016 covered the summer months with an average water temperature at 28.4 ± 2.2°C, from Sept to Nov of 2016 covered the autumn months with water temperature at 24.0 ± 1.7°C, from Nov to Dec of 2016 covered the early-mid phase of winter with water temperature at 20.4 ± 3.5°C, and Jan–Feb of 2017 covered the peak phase of winter with water temperature at 15.1 ± 2.6°C. After a 14-day entrainment of “one-meal-per-day” feeding schedule, feeding behaviors and food consumption were measured as described previously (14). In this case, different patterns of feeding behaviors were recorded for 2 h using a KPsec VD 714 Surveillance System (Avtech) after introduction of food pellets (TetraMin GmBH, Germany; with ~47% crude protein, ~10% crude oils & fat, ~6% crude fiber and supplements of vitamins and minerals). By the end of the 2-h period, the remains of unconsumed pellets were collected and routinely dried in a 45°C oven for 3–4 days until a constant mass had been acquired. After correction for the loss of soluble components in food pellets (~0.15% by weight), the mass difference of the remains vs. the amount added at the beginning (~2% BW/fish) was used as an index for food intake. Based on the video recorded, the cumulative counts of three types of feeding behaviors observed in goldfish, namely complete feeding/surface foraging, incomplete feeding/food spitting and bottom feeding/bottom foraging, were scored manually in a single-blind manner by the parameters defined by Volkoff and Peter (15). Briefly, complete feeding was defined as the feeding act of engulfing food pellets on water surface in a single foraging movement. Incomplete feeding, in contrast, referred to the “food rejection act” of regurgitation/spitting of food pellet without swallowing. Unlike complete feeding occurred on the water surface, bottom feeding was defined as the feeding act to pick up food pellets/debris sunk to the bottom. In the present study, the data for food intake were also correlated with water temperature in individual experiments conducted at different times of the year using Pearson product-moment correlation analysis.
Feeding Changes With Long-Term Acclimation to Summer and Winter Temperature
To confirm that seasonal variations in feeding observed were caused by temperature change in the environment, goldfish maintained at 20°C during the autumn period (Sep–Oct, 2017) were divided into two groups and subjected to long-term acclimation for 4 weeks in water tanks maintained at summer temperature (28°C) and winter temperature (15°C), respectively. During the period, the fish were trained with “one-meal-per-day” feeding at 28/15°C and used for the scoring of feeding behaviors and food consumption as described in the preceding section. To examine the mechanisms involved in temperature regulation of feeding behavior and food intake, parallel experiments were also performed to study the effects of a 4-week acclimation at 28°C during the summer (July–Aug, 2016) and 15°C during the winter (Jan–Feb, 2017) on transcript expression of feeding regulators identified in the liver and brain areas involved in feeding control in fish models, including the telencephalon, hypothalamus and optic tectum (7). The long-term acclimation at respective temperatures for the two seasons was conducted to minimize the effect of daily fluctuations of water temperature on target gene expression. After acclimation to the respective temperature, the liver and target brain areas were excised and total RNA and genomic DNA were extracted with Trizol (Invitrogen) according to the instructions of the manufacturer. DNA contents in individual samples were quantified by OD260/280 reading and the data obtained were then used for subsequent data normalization for target gene expression. The RNA samples prepared were digested with DNase I, reversely transcribed by Superscript II (Invitrogen), and subjected to real-time PCR for transcript measurement of target regulators for feeding in goldfish using a RotorGene-Q qPCR System (Qiagen) with a Lightcycler® 480 SYBR Green I Master Kit (Roche) (16). PCR reactions were conducted with primers and PCR conditions for different gene targets as shown in Table 1. In our study, parallel measurements of β actin and elongation factor Iα (EF-Iα) gene expression were also conducted to serve as internal controls.
Feeding Responses and Gene Expression Induced by Short-Term Temperature Change
To study the short-term responses induced by temperature change, goldfish trained with “one-meal-per-day” feeding and acclimated at 28°C were transferred to water tanks at 15°C for 24 h. Parallel transfer of goldfish to water tanks at 28°C was used as a control treatment. After 24-h exposure to temperature drop, feeding experiment was initiated (at 28°C for control and 15°C for treatment) to monitor the effects of acute temperature change on feeding behaviors and food consumption as described previously. To test for the reversibility of temperature effect, reciprocal experiment was also performed by transferring goldfish acclimated at 15°C to water tanks at 28°C for 24 h. In this case, parallel transfer to water tanks at 15°C was used as the control group. To establish the time-course of target gene expression for feeding regulators associated with the short-term temperature change, goldfish acclimated at 28°C was housed in water tanks linked with the thermal controlling unit to allow for a gradual drop of water temperature to 15°C in 6 h without disturbing the fish (Figure 1). After that, the fish was maintained at 15°C until the end of the 24-h period. For the control treatment, fish were housed in water tanks at 28°C with no temperature change over the same period. In our study, the liver as well as selected brain areas including the telencephalon, hypothalamus and optic tectum were harvested from individual fish at 24 and 12 h before and at 0, 6, 12, and 24 h after the initiation of temperature drop to 15°C. Total RNA and DNA were extracted from these samples with Trizol and RT samples prepared were then used for real-time PCR measurement of target gene expression as described in the preceding section.
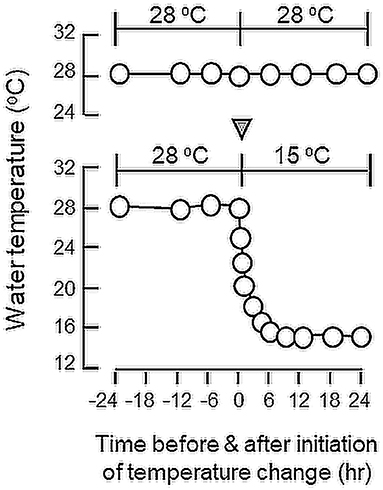
Figure 1. Profile of temperature change during the short-term acclimation of goldfish from summer temperature (28°C) to winter temperature (15°C). Goldfish were maintained in 28°C water for 4 weeks during the summer (Jun–July, 2017) prior to the activation of the cooling system linked to the water tank to gradually reduce the water temperature to 15°C (as indicated by the inverted triangle). The cooling system could allow for a gradual drop in water temperature from 28 to 15°C within 6 h without the need of transferring the fish during the experiment. Without activating the cooling system (as shown in upper panel), water temperature was maintained at 28°C without noticeable change over a 24-h period.
Data Transformation and Statistical Analysis
For measurement of feeding behaviors, cumulative counts for different types of feeding behaviors were scored every 10 min continuously over a period of 2 h. Food consumption over the 2-h period was normalized as the mass of food pellets taken by the fish over 60 min and used as an index for food intake after thermal acclimation. For real-time PCR of target gene expression, standard curves constructed with serial dilutions of plasmid DNA carrying the ORF/amplicons for target genes with a dynamic range of ≥105, amplification efficiency ≥98% and correlation coefficient ≥0.95 were used for data calibration with RotorGene Q-Rex software (Qiagen). To adjust for variations in the amount of tissues used in RNA extraction, raw data for transcript expression (in femtomole mRNA detected) were expressed as a ratio of genomic DNA (per μg DNA) detected in the same sample. Since the internal controls for β actin and EF-Iα did not show significant difference after long-term/short-term acclimation, the normalized data were presented directly or transformed as a percentage of mean values in the reference control. For the data obtained from seasonality study or experiments with 4-week/24-h acclimation to summer/ winter temperature (with temperature change as the variable), statistical analysis with Student's t-test or one-way ANOVA followed by Tukey post-hoc test was performed. For the time-course study on gene expression with temperature drop from 28 to 15°C (with time and temperature change as two variables), the data were analyzed by two-way ANOVA prior to Tukey test. In both cases, data presented are expressed as mean ± SEM (n = 10–16) and differences between treatment groups were considered as significant at p < 0.05.
Results
Seasonal Change in Feeding and Its Correlation With Water Temperature
In goldfish subjected to seasonal change in temperature during the transition from summer to winter, except for a lack in response for incomplete feeding/food spitting activity, the cumulative counts for feeding behaviors, including complete feeding/surface foraging and bottom feeding/ bottom foraging, were found to be reduced gradually from the summer (Jul–Aug, 2016), autumn (Sept–Oct, 2016), early-mid phase of the winter (Nov–Dec, 2016) to the peak phase of winter (Jan–Feb, 2017) (Figure 2A). During the same period, water temperature was reduced from 28.4 ± 2.2°C in summer to 15.1 ± 2.6°C during the peak phase of winter with a gradual drop in food consumption (Figure 2B). In the same study, Pearson's analysis also revealed a positive correlation between the drop in water temperature and the gradual decline in food consumption during the progression from summer to winter period (Figure 2C).
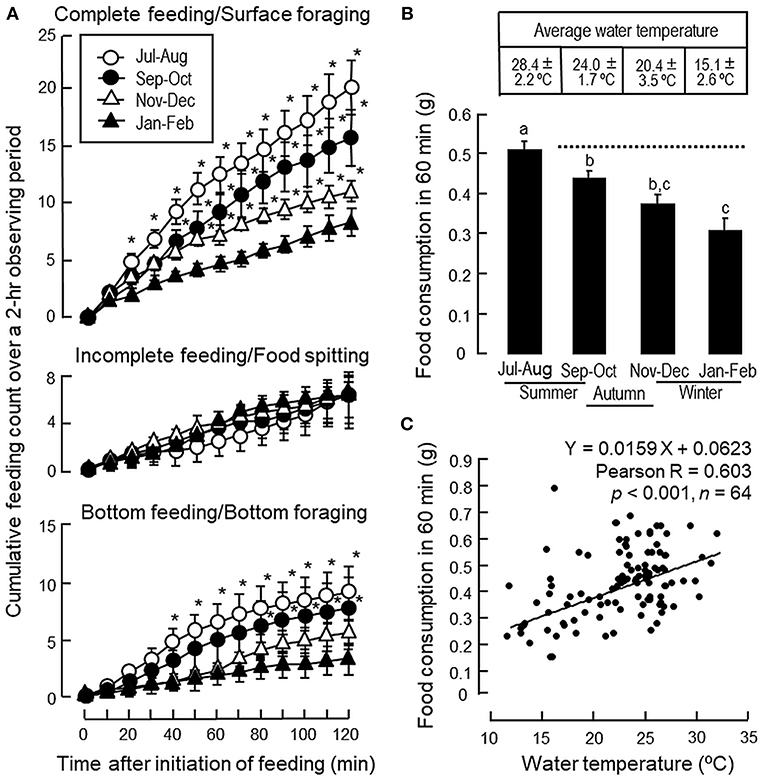
Figure 2. Seasonal changes of feeding behaviors and food intake in goldfish during the transition from summer to winter. (A) Seasonal changes of complete feeding/surface foraging, incomplete feeding/food spitting and bottom feeding/bottom foraging during the transition from the summer (Jul–Aug, 2016), autumn (Sep–Oct, 2016), early-mid phase of the winter (Nov–Dec, 2016) to the peak phase of the winter (Jan–Feb, 2017). (B) Seasonal change of food consumption related to the temperature drop in the environment during the same period. (C) Positive correlation of the gradual decline in food intake observed during the transition from summer to winter months as shown in (B) with the parallel drop in water temperature as revealed by Pearson product-moment regression analysis. Data presented, including feeding behaviors, food consumption and water temperature are expressed as mean ± SEM (n = 14–16). Feeding behaviors were scored over a period of 2 h and the data of feeding counts obtained during the summer, autumn and early-mid phase of the winter were compared with the corresponding data of the same time point from the group scored during the peak phase of the winter using Student's t-test. For food intake occurred during the same period, the data for food consumption from different groups were analyzed by one-way ANOVA followed by Tukey post-hoc test. Differences between treatment groups were considered as significant at p < 0.05.
Long-Term Thermal Acclimation on Feeding and Gene Expression of Feeding Regulators
To test if temperature change can serve as the cause for seasonal variations in feeding, long-term acclimation of goldfish for 4 weeks to either summer (28°C) or winter temperature (15°C) were performed. In this case, the cumulative counts for complete feeding/surface foraging and bottom feeding/bottom foraging in the group acclimated at 28°C were found to be notably higher than the group maintained at 15°C (Figure 3A). Similar to the results of seasonal change in feeding behaviors, the counts for incomplete feeding/food spitting were not affected by variation in water temperature. When compared to the group at 28°C, a parallel drop in food consumption was also noted with thermal acclimation to 15°C (Figure 3B), which was in agreement with the decline in foraging activity occurring both at the surface and bottom levels.
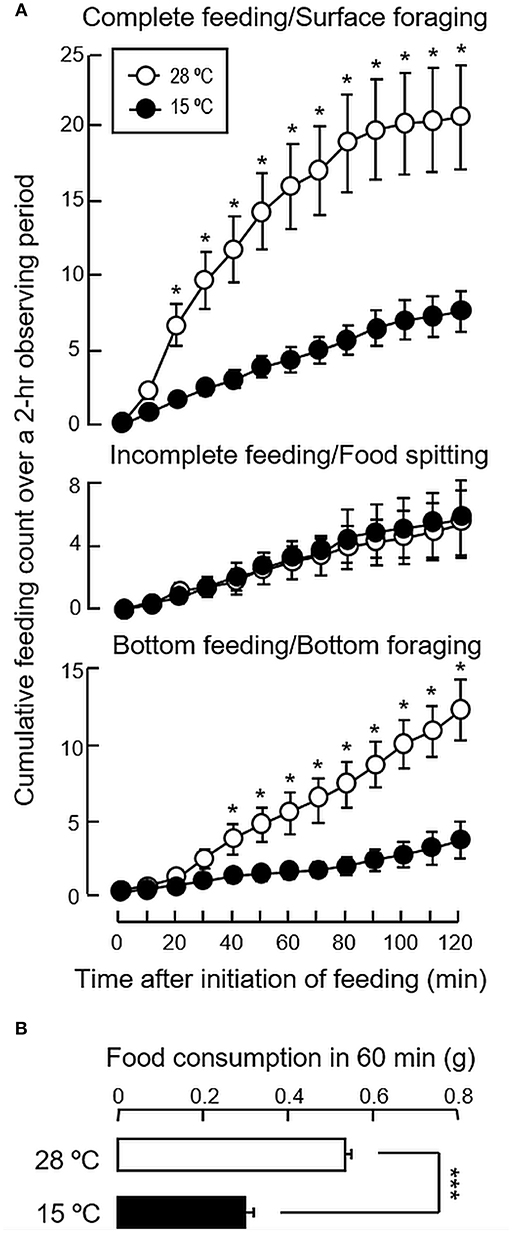
Figure 3. Long-term acclimation to the summer temperature (28°C) and winter temperature (15°C) on feeding behaviors and food consumption in goldfish. Goldfish acclimated to 20°C during the autumn months (Sep–Oct, 2017) were maintained for 4 weeks in 28 and 15°C water tanks respectively prior to the measurement of (A) feeding behaviors and (B) food consumption. In this experiment, the feeding counts for the three types of feeding behaviors, namely complete feeding, incomplete feeding and bottom feeding, as well as the food intake occurred during the same period were compared between the two groups using Student's t-test. Data presented are expressed as mean ± SEM (n = 12) and the difference between the two groups was considered as significant at p < 0.05 (*p < 0.05 and ***p < 0.001).
In parallel study using goldfish acclimated at 28°C during the summer as a reference control, acclimation of the fish to 15°C during the winter did not alter transcript expression of β actin and EF-Iα in the liver as well as in brain areas including the telencephalon, hypothalamus and optic tectum (Figure 4). In the telencephalon, however, parallel rises in LepR, CART, CCK and POMC mRNA levels were noted with no significant changes in transcript expression for leptin I, leptin II, NPY, orexin and apelin (Figure 4A). A similar pattern of transcript expression was also observed in the hypothalamus except that 15°C acclimation during winter did not alter CART expression but induced an elevation in MCH with a concurrent drop in orexin mRNA level (Figure 4B). In the optic tectum, unlike the responses in telencephalon/hypothalamus, except for the rise in LepR mRNA, significant changes in transcript expression for the other target genes examined were not apparent (Figure 4C). In the same study, interestingly, acclimation at 15°C during the winter was effective in increasing leptin I and II mRNA levels in the liver but with no concurrent change in LepR gene expression at the hepatic level (Figure 4D).
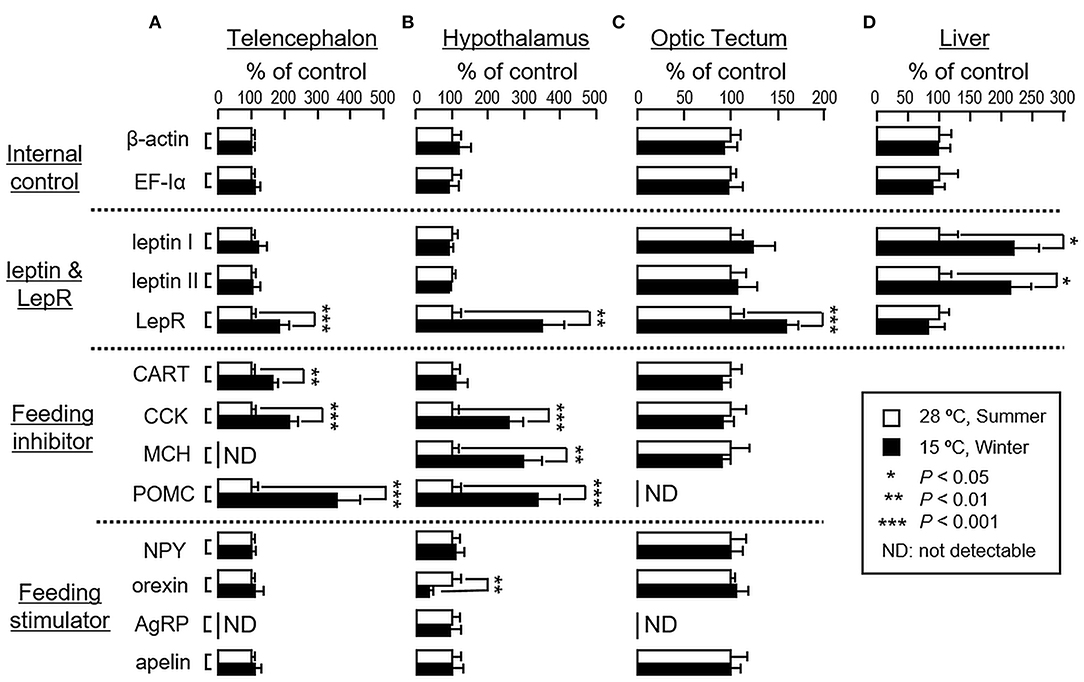
Figure 4. Transcript expression of orexigenic and anorexigenic factors in the liver and brain areas involved in feeding control in goldfish during the summer and winter months. To avoid the variability of daily fluctuation in water temperature, goldfish were maintained for 4 weeks at 28°C during the summer (July–Aug, 2016) and at 15°C during the winter (Jan–Feb, 2017). After that, the liver and brain areas, including the telencephalon, hypothalamus and optic tectum, were harvested and used for RNA isolation. RT samples were then prepared and used for real-time PCR for the respective gene targets. In this experiment, parallel measurement of β actin and EF-Iα mRNA expression were also conducted to serve as the internal control. Data presented (mean ± SEM, n = 12) were compared with Student's t-test and the difference between the two groups was considered as significant at p < 0.05 (*p < 0.05, **p < 0.01 and ***p < 0.001).
Short-Term Thermal Acclimation on Feeding and Gene Expression of Feeding Regulators
As shown in Figure 5A, a notable reduction in the counts for complete feeding/surface foraging and bottom feeding/bottom foraging was observed following a 24-h exposure to 15°C water in goldfish previously acclimated at 28°C, while the opposite was true with parallel transfer of goldfish acclimated at 15°C to 28°C water for 24 h in the reciprocal experiment. Consistent with the results for long-term acclimation, short-term changes in water temperature (from 28 to 15°C/from 15 to 28°C for 24 h) were not effective in altering incomplete feeding/food spitting activity. Of note, modifications in foraging activity were also reflected by corresponding changes in food intake. In this case, food consumption was reduced in 28°C fish after transfer to 15°C water but increased in 15°C fish after transfer to 28°C water (Figure 5B). In contrast, parallel transfer of goldfish to water tanks with “acclimated temperature” (i.e., 28°C to 28°C and 15°C to 15°C) did not trigger any noticeable changes in feeding behaviors/food intake, indicating that the feeding responses observed were not caused by handling stress during the experiment.
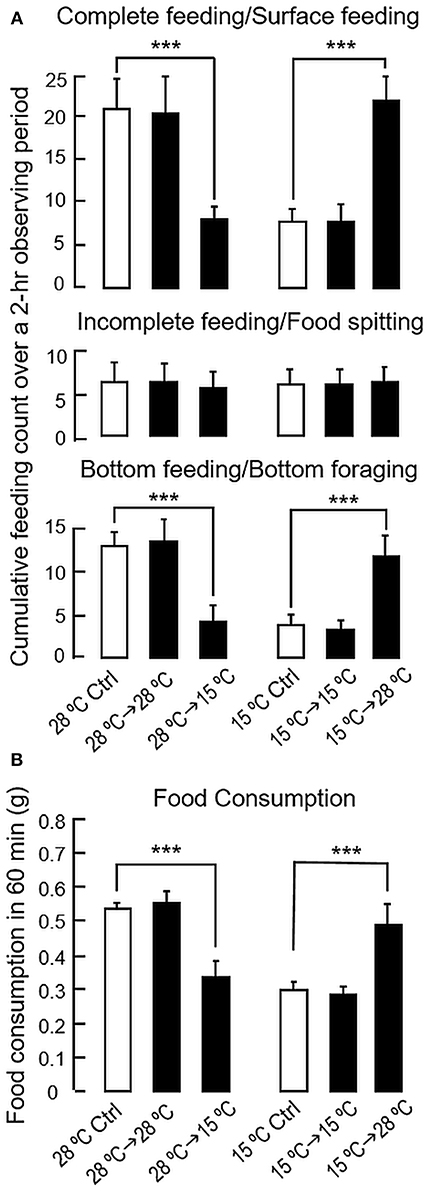
Figure 5. Short-term acclimation to the summer temperature (28°C) and winter temperature (15°C) on feeding behaviors and food consumption in goldfish. Goldfish acclimated to 20°C during the autumn months (Sep–Oct, 2017) were maintained for 4 weeks in 28 and 15°C water tanks, respectively. After that, the fish acclimated to 28°C were transferred to water tanks at 15°C for 24 h. In reciprocal experiment, the fish acclimated to 15°C were transferred to water tanks at 28°C during the same period. As control treatment, parallel experiments without transferring the fish or with parallel transfer into water tanks with the same acclimation temperature (i.e., from 28 to 28°C/from 15 to 15°C) were also conducted. Following the short-term exposure to temperature change, measurement of different types of feeding behaviors (A) and food intake (B) were performed according to the standard protocols. The data obtained (mean ± SEM, n = 10–12) were analyzed with one-way ANOVA followed by Tukey post-hoc test. Difference between groups was considered as significant at p < 0.05 (***p < 0.001).
To shed light on the mechanisms for feeding control by short-term temperature change, a time-course experiment was conducted in goldfish acclimated at 28°C with a gradual drop of water temperature from 28°C to 15°C. In our system, water temperature could be reduced to 15°C within the first 6 h after initiation of temperature change (Figure 1). Similar to our seasonality study, short-term exposure to 15°C could trigger differential changes in transcript expression of feeding regulators in the liver as well as in different brain areas. In the telencephalon, CART, CCK, POMC and LepR mRNA levels were found to be elevated in a time-dependent manner with no significant changes in β actin, NPY, orexin, leptin I and leptin II gene expression (Figure 6). The pattern of transcript expression in the hypothalamus, including the rises in CCK, POMC, and LepR gene expression, was comparable with that of the telencephalon. Interestingly, a drop in orexin mRNA with a parallel rise in MCH transcript level were also noted, which were absent in the telencephalon (Figure 7). In the optic tectum, except for the rise in LepR mRNA, no significant changes were observed regarding the gene expression for β actin, NPY, orexin, CART, CCK, MCH, leptin I, leptin II, and LepR (Figure 8). In the same study, however, leptin I and II mRNA levels were found to be elevated in the liver but without parallel change in β actin and LepR gene expression (Figure 9).
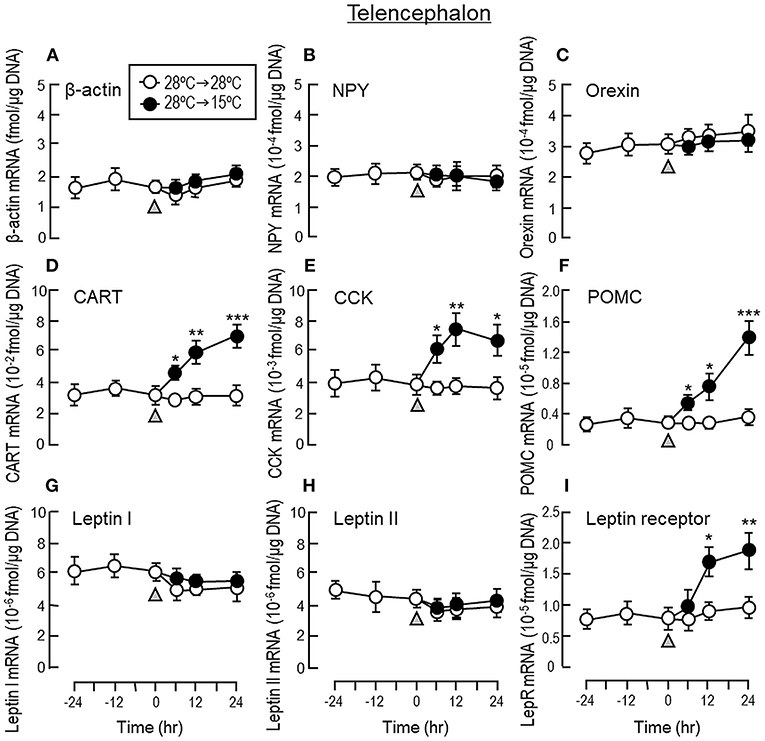
Figure 6. Transcript expression of orexigenic and anorexigenic factors within the telencephalon of goldfish with short-term exposure to winter temperature (15°C). Water temperature for goldfish acclimated at 28°C was gradually reduced to 15°C over a 24-h period using a cooling system linked with the water tank. The telencephalon was harvested from individual fish at different time points before and after the activation of the cooling system (as indicated by gray triangle). Total RNA was isolated, reversely transcribed and used for real-time PCR for respective gene targets, including (A) β actin, (B) NPY, (C) Orexin, (D) CART, (E) CCK, (F) POMC, (G) leptin I, and (H) leptin II and (I) leptin receptor. Parallel experiment with goldfish maintained at 28°C water without activation of the cooling system was used as the control treatment. Similar to the previous study on seasonality of orexigenic/anorexigenic signals, transcript expression of β actin was used as the internal control. For our time course study, the data obtained (mean ± SEM, n = 12) were analyzed using two-way ANOVA followed by Tukey test. Difference between groups was considered as significant at p < 0.05 (*p < 0.05, **p < 0.01, and ***p < 0.001).
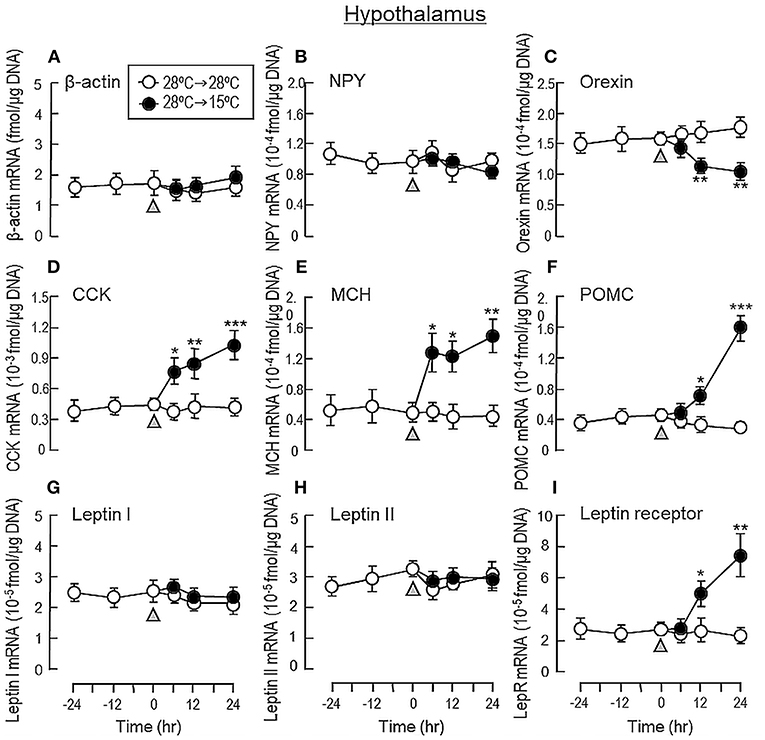
Figure 7. Transcript expression of orexigenic and anorexigenic factors within the hypothalamus of goldfish with short-term exposure to winter temperature (15°C). Water temperature for goldfish acclimated at 28°C was gradually reduced to 15°C over a 24-h period using a cooling system linked with the water tank. The hypothalamus was harvested from individual fish at different time points before and after the activation of the cooling system (as indicated by gray triangle). Total RNA was isolated, reversely transcribed and used for real-time PCR for respective gene targets, including (A) β actin, (B) NPY, (C) Orexin, (D) CCK, (E) MCH, (F) POMC, (G) leptin I, and (H) leptin II and (I) leptin receptor. Parallel experiment with fish maintained at 28°C water without activation of the cooling system was used as the control treatment. For our time course study, the data obtained (mean ± SEM, n = 12) were analyzed with two-way ANOVA followed by Tukey test. Difference between groups was considered as significant at p < 0.05 (*p < 0.05, **p < 0.01, and ***p < 0.001).
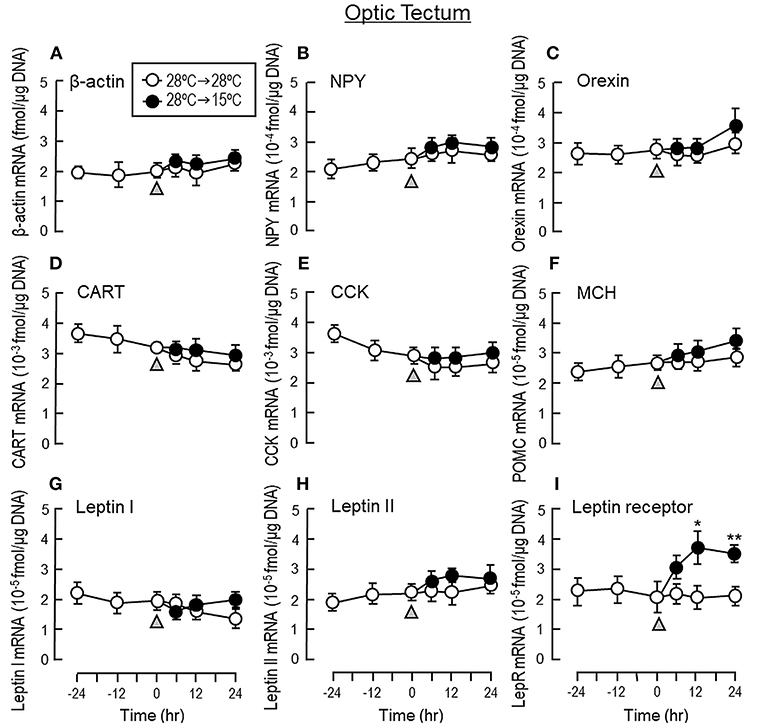
Figure 8. Transcript expression of orexigenic and anorexigenic factors within the optic tectum of goldfish with short-term exposure to winter temperature (15°C). Water temperature for goldfish acclimated at 28°C was gradually reduced to 15°C over a 24-h period using a cooling system linked with the water tank. The optic tectum was harvested from individual fish at different time points before and after the activation of the cooling system (as indicated by gray triangle). Total RNA was isolated, reversely transcribed and used for real-time PCR for respective gene targets, including (A) β actin, (B) NPY, (C) Orexin, (D) CART, (E) CCK, (F) MCH, (G) leptin I, and (H) leptin II and (I) leptin receptor. Parallel experiment with goldfish maintained at 28°C water without activation of the cooling system was used as the control treatment. For our time course study, the data obtained (mean ± SEM, n = 12) were analyzed with two-way ANOVA followed by Tukey test. Difference between groups was considered as significant at p < 0.05 (*p < 0.05, **p < 0.01, and ***p < 0.001).
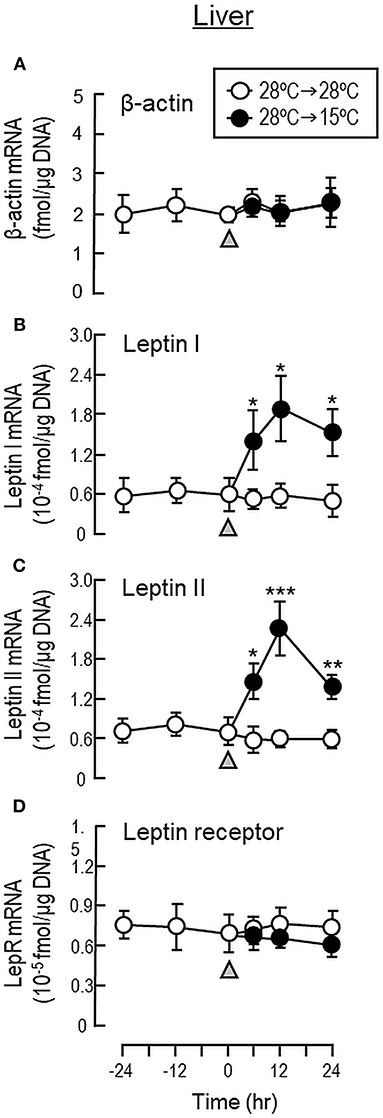
Figure 9. Transcript expression of leptin and leptin receptor in the liver of goldfish with short-term exposure to winter temperature (15°C). Water temperature for goldfish acclimated at 28°C was reduced to 15°C over a 24-h period using a cooling system linked with the water tank. The liver was harvested from individual fish at different time points before and after the activation of the cooling system (as indicated by gray triangle). Total RNA was isolated, reversely transcribed and used for real-time PCR for respective gene targets, including (A) β actin, (B) leptin I, (C) leptin II and (D) leptin receptor. Parallel experiment with goldfish maintained at 28°C water without activation of the cooling system was used as the control treatment. For our time course study, the data obtained (mean ± SEM, n = 12) were analyzed with two-way ANOVA followed by Tukey test. Difference between groups was considered as significant at p < 0.05 (*p < 0.05, **p < 0.01, and ***p < 0.001).
Discussion
In poikilotherms, especially in fish species, body functions including somatic growth (8, 9, 17), reproduction (18, 19), metabolism (20), locomotor activity (21), stress responses (22), embryonic development (23), and immune functions (24) are known to be sensitive to temperature change. In fish models, circannual cycle in feeding pattern/food intake has been reported and can be associated with seasonal changes in water temperature and photoperiod (4). In general, elevation in feeding can be noted in fish species during the spring/summer months with higher temperature (25). This is at variance with the case in non-hibernating homeotherms, e.g., domesticated cats, with increased feeding in the late autumn/winter (26), which may be related to the elevated metabolic demand for thermogenesis at low temperature. The seasonal change in feeding observed in fish species is also in agreement with the results of previous studies showing that food intake can be reduced by low temperature, e.g., in catfish (Ictalurus punctatus) (27), halibut (Hippoglossus hippoglossus) (28), sickleback (Gasterosteus aculeatus) (29), turbot (Scophthalmus maximus) (30), and tench (Tinca tinca) (31). However, species-specific variations in feeding responses do exist in fish models. For examples, high temperature is known to induce voluntary anorexia in Atlantic salmon (Salmo salar) (11) and summer fasting can also be observed in some cold water fish, e.g., in cunner (Tautogolabrus adspersus) (32), suggesting that the “temperature effect” on feeding can be quite different between warm water and cold water species.
To confirm that seasonal change in feeding do exist in goldfish, a cyprinid species known to be well-adapted to a wide range of water temperature, its feeding behavior and food consumption were monitored over a period of 8 months covering the transition from summer to winter. In our study, a gradual decline in foraging behavior (both surface and bottom foraging) was noted during the progression from summer to winter with a parallel drop in water temperature. The decline in foraging activity also occurred with parallel reduction in food intake, which was found to have a positive correlation with the attenuation in water temperature during the same period, suggesting that the seasonal change in environmental temperature may contribute to the observed differences in feeding responses between the summer and winter months. In goldfish, regulation of food consumption can be achieved by alteration of foraging activity in water surface/at bottom level with concurrent modification in food spitting activity, e.g., after treatment with NPY (33) or spexin (14). However, food spitting activity did not exhibit significant changes in our seasonality study or parallel experiments with long-term/short-term acclimation to different temperatures and the involvement of this food rejection behavior in the seasonal cycle of feeding is rather unlikely. In our study, using the fish acclimated to summer temperature (28°C) as a reference, long-term and short-term acclimation to winter temperature (15°C) were both effective in mimicking the decrease in foraging activity and food intake observed during the seasonal change from summer to winter. The results of short-term acclimation (from 28 to 15°C and from 15 to 28°C) also reveal that the changes in feeding responses were highly reversible and rapid modifications in feeding behavior/food intake could be noted within 24 h exposure to temperature change. Our findings are highly comparable with the previous study in salmon parr showing that a short-term cold stress (>4 h) was sufficient to induce a rapid drop in food intake (34) and provide evidence that temperature change in the environment can trigger the seasonal cycle of feeding in goldfish, presumably via a rapid modulation in feeding behavior/foraging activity.
In homeotherms, including birds and mammals, modification of food intake by thermal stress (1, 35) is typically associated with corresponding changes in orexigenic/anorexigenic signals in the brain as well as in peripheral tissues (e.g., GI tract and adipose tissue) (2, 3, 36). In mammals (e.g., rat), the central effects of thermal regulation are commonly accepted to be mediated by the temperature-sensitive neurons within the hypothalamus (37), presumably via activation of thermo-TRP ion channels (38). In bony fish, the functional roles of orexigenic factors including NPY (33), orexin (39), AgRP (40), apelin (41), and ghrelin (42) and anorexigenic factors including CCK (43), CART (44), αMSH (45), MCH (46), and leptin (47) in appetite control are well-documented, but not much information is available for their regulation by temperature change. At present, only four studies have been reported on this topic in fish models. These include the previous studies showing up-regulation of CART in the hypothalamus of Atlantic cod (Gadus morhua) at low temperature (6) and reduction in hypothalamic levels of ghrelin receptor and NPY in salmon (Salmo salar) with parallel drops in plasma ghrelin at high temperature (11). Recently, two other reports have been published demonstrating that ghrelin and CCK expression in the brain could be elevated by high temperature in perch (Siniperca chuatsi) (12) and seahorse (Hippocampus erectus) (48), respectively. Unfortunately, the results from these studies are still limited and a common consensus has not been reached for temperature control of feeding based on the feeding regulators examined. In fish models, seasonal variations in central expression of orexigenic/ anorexigenic signals has been reported, e.g., for ghrelin (49), leptin (50), CCK (51), and NPY (52). Therefore, it would be tempting to speculate that their regulation by temperature can mediate the circannual cycle of food intake. However, the idea was not supported by the recent study in Arctic charr (Salvelinus alpinus), in which the seasonal patterns of NPY, AgRP, POMC, CART, and leptin expression in brain areas involved in appetite control did not match with its circannual rhythm of feeding (13). To date, the functional link between seasonal cycle of feeding and thermal regulation of orexigenic/anorexigenic signals in the fish brain remains unclear and further studies are highly warranted.
To shed light on the role of orexigenic/anorexigenic signals in seasonal change of feeding in cyprinid species, long-term acclimation of goldfish during the summer at 28°C and during the winter at 15°C were also conducted. In fish models, e.g., salmon (Salmo salar) (53), common carp (Cyprinus carpio) (54), and more recently in goldfish (Carassius auratus) (47), two forms of leptin, namely leptin I and II, have been identified, which are believed to be the result of fish-specific/3R whole genome duplication (55). Unlike mammals with leptin expressed mainly in adipose tissue, leptin is expressed at high levels in the liver of fish species (54–56) and exerts its effect as a satiety factor by regulating central expression of NPY, POMC and/or CCK, e.g., in goldfish (Carassius auratus) (57) and trout (Oncorhynchus mykiss) (58). When compared with its “summer counterpart” at 28°C, goldfish at 15°C during the winter was found to have notable elevations in leptin I and II mRNA levels in the liver with parallel rises of LepR gene expression in the telencephalon, hypothalamus and optic tectum, which are the major brain areas in goldfish involved in appetite control (7). Although the functional roles of NPY, AgRP, orexin, and apelin as orexigenic factors in fish models are well-documented (59) and their stimulatory effects on feeding have also been confirmed in goldfish (33, 41, 60), except for the drop in orexin mRNA occurring in the hypothalamus at 15°C, noticeable changes in gene expression for these feeding stimulators were not observed in the brain areas examined. In the same study, 15°C acclimation during the winter was found to up-regulate central expression of anorexigenic factors, including the transcript expression of CCK, CART, and POMC in the telencephalon and CCK, MCH, and POMC in the hypothalamus. In contrast, significant changes of leptin I, leptin II, CCK, CART, MCH, and POMC signals were not apparent in the optic tectum. A similar pattern of transcript expression observed in our seasonality study was also noted in our time-course experiment with a gradual drop of water temperature to 15°C within 6 h in goldfish acclimated at 28°C. In this case, similar to the rapid responses of foraging/food intake with short-term thermal acclimation, notable changes of transcript expression for leptin I and II in the liver as well as LepR and other feeding regulators expressed in different brain areas were also observed within 6–12 h exposure to temperature change and maintained up to 24 h during the course of the experiment. These results, as a whole, suggest that the reduction in foraging activity and food intake in goldfish caused by the seasonal change in water temperature may be mediated by the rises of leptin I and II signals in the liver with parallel enhancement in leptin sensitivity via LepR up-regulation in brain areas involved in feeding control. Meanwhile, central regulation of orexigenic/anorexigenic signals can also occur, with a down-regulation of orexin in the hypothalamus along with parallel rises of CCK, CART, MCH, and POMC expression in the telencephalon/hypothalamus. In our study, the orexigenic/anorexigenic factors expressed in the optic tectum did not exhibit seasonal change/noticeable responses to temperature drop. Presumably, this brain area is not a major site within the CNS for temperature sensing or thermal responses in goldfish.
In summary, we have confirmed that seasonal change of feeding with a parallel reduction in foraging activity and food intake do exist in goldfish as a result of temperature drop during the transition from summer to winter period. These feeding responses can occur rapidly and are highly reversible with respect to temperature change, and may involve the leptin output from the liver with differential modifications of orexigenic/anorexigenic signals and leptin responsiveness in brain areas for appetite control. To our knowledge, our study represents the first report on (i) thermal regulation of leptin expression in the liver and (ii) involvement of leptin/LepR system in seasonal change of feeding induced by temperature drop in a fish model. Although our studies have provided new insights on the mechanisms for seasonal change of feeding in fish species, the functional components for thermal detection, e.g., through the thermal sensing neurons within the hypothalamus (37) or vagus nerve network (61), have yet to be determined. Of note, POMC expression induced by CCK (43) and CART expression induced by leptin (62) and MCH (63) have been reported in goldfish, especially in brain areas responsible for feeding control, and the possibility for functional interactions among different feeding regulators in the seasonal cycle of feeding cannot be excluded. Besides temperature change, photoperiod is another environmental cue known to affect feeding in fish species (4). Given that melatonin has been shown to inhibit food intake in goldfish (64) and stimulate leptin expression in the liver (65) with parallel changes of orexigenic/anorexigenic signals in the brain of zebrafish (Danio rerio) (66), the functional interplay between photoperiod and temperature via a “crosstalk” of melatonin with other feeding regulators for sure can be an interesting topic to follow up for the seasonal change of feeding in fish models.
Data Availability
The raw data supporting the conclusions of this manuscript will be made available by the authors, without undue reservation, to any qualified researcher.
Ethics Statement
The study was carried out in accordance with the recommended guidelines for the care and use of laboratory animals for research and teaching at the University of Hong Kong (Hong Kong). The protocol used in our study (CULATR 4608-18) was approved by the Committee on the Use of Live Animal for Teaching and Research, University of Hong Kong.
Author Contributions
AW was the PI and grant holder. AW and TC were responsible for project planning and data analysis. TC and MW were involved in the experiments for seasonal studies and thermal acclimation. Manuscript preparation was done by AW and BC.
Funding
The project was supported by GRF grants (17128215 & 17117716, & 17113918) from the Research Grant Council (Hong Kong) and HMRF grant (13142591) from the Food and Health Bureau (Hong Kong Government).
Conflict of Interest Statement
The authors declare that the research was conducted in the absence of any commercial or financial relationships that could be construed as a potential conflict of interest.
Acknowledgments
Support from the School of Biological Sciences, University of Hong Kong (Hong Kong), in the form of postgraduate studentship (to TC and MW) is acknowledged. We also thank Mr. C. P. Wat for his help in setting up the system for measurement of foraging activity and food intake in goldfish and Dr. N. K. Wong for proof reading and editing of the manuscript.
Abbreviations
AgRP, Agouti-Related Peptide; α-MSH, Alpha Melanocyte-Stimulating Hormone; CART, Cocaine- and Amphetamine-Regulated Transcript; CCK, Cholecystokinin; MCH, Melanin-Concentrating Hormone; NPY, Neuropeptide Y; POMC, Proopiomelanocortin; LepR, Leptin Receptor; CNS, Central Nervous System.
References
1. Charlot K, Faure C, Antoine-Jonville S. Influence of hot and cold environments on the regulation of energy balance following a single exercise session: a mini-review. Nutrients. (2017) 9:E592. doi: 10.3390/nu9060592
2. He X, Lu Z, Ma B, Zhang L, Li J, Jiang Y, et al. Effects of chronic heat exposure on growth performance, intestinal epithelial histology, appetite-related hormones and genes expression in broilers. J Sci Food Agric. (2018) 98:4471–8. doi: 10.1002/jsfa.8971
3. Kojima C, Sasaki H, Tsuchiya Y, Goto K. The influence of environmental temperature on appetite-related hormonal responses. J Physiol Anthropol. (2015) 34:22. doi: 10.1186/s40101-015-0059-1
4. Volkoff H, Hoskins LJ, Tuziak SM. Influence of intrinsic signals and environmental cues on the endocrine control of feeding in fish: potential application in aquaculture. Gen Comp Endocrinol. (2010) 167:352–9. doi: 10.1016/j.ygcen.2009.09.001
5. Bendiksen EA, Jobling M, Arnesen AM. Feed intake of Atlantic salmon parr Salmo salar L. in relation to temperature and feed composition. Aquacul Res. (2002) 33:525–32. doi: 10.1046/j.1365-2109.2002.00737.x
6. Kehoe AS, Volkoff H. The effects of temperature on feeding and expression of two appetite-related factors, neuropeptide Y and cocaine- and amphetamine-regulated transcript, in Atlantic cod, Gadus morhua. J World Aquacul Soc. (2008) 39:790–6. doi: 10.1111/j.1749-7345.2008.00215.x
7. Volkoff H, Xu M, Macdonald E, Hoskins L. Aspects of the hormonal regulation of appetite in fish with emphasis on goldfish, Atlantic cod and winter flounder: notes on actions and responses to nutritional, environmental and reproductive changes. Comp Biochem Physiol A Mol Integr Physiol. (2009) 153:8–12. doi: 10.1016/j.cbpa.2008.12.001
8. Gabillard JC, Weil C, Rescan PY, Navarro I, Gutierrez J, Le Bail PY. Environmental temperature increases plasma GH levels independently of nutritional status in rainbow trout (Oncorhynchus mykiss). Gen Comp Endocrinol. (2003) 133:17–26. doi: 10.1016/S0016-6480(03)00156-4
9. Gabillard JC, Weil C, Rescan PY, Navarro I, Gutierrez J, Le Bail PY. Effects of environmental temperature on IGF1, IGF2, and IGF type I receptor expression in rainbow trout (Oncorhynchus mykiss). Gen Comp Endocrinol. (2003) 133:233–42. doi: 10.1016/S0016-6480(03)00167-9
10. Gabillard JC, Yao K, Vandeputte M, Gutierrez J, Le Bail PY. Differential expression of two GH receptor mRNAs following temperature change in rainbow trout (Oncorhynchus mykiss). J Endocrinol. (2006) 190:29–37. doi: 10.1677/joe.1.06695
11. Hevroy EM, Waagbo R, Torstensen BE, Takle H, Stubhaug I, Jorgensen SM, et al. Ghrelin is involved in voluntary anorexia in Atlantic salmon raised at elevated sea temperatures. Gen Comp Endocrinol. (2012) 175:118–34. doi: 10.1016/j.ygcen.2011.10.007
12. Song Y, Zhao C, Liang XF, He S, Tian C, Cheng X, et al. Effects of fasting, temperature, and photoperiod on preproghrelin mRNA expression in Chinese perch. Fish Physiol Biochem. (2017) 43:803–12. doi: 10.1007/s10695-016-0335-y
13. Striberny A, Ravuri CS, Jobling M, Jorgensen EH. Seasonal differences in relative gene expression of putative central appetite regulators in Arctic charr (Salvelinus alpinus) do not reflect its annual feeding cycle. PLoS ONE. (2015) 10:e0138857. doi: 10.1371/journal.pone.0138857
14. Wong MK, Sze KH, Chen T, Cho CK, Law HC, Chu IK, et al. Goldfish spexin: solution structure and novel function as a satiety factor in feeding control. Am J Physiol Endocrinol Metab. (2013) 305:E348–366. doi: 10.1152/ajpendo.00141.2013
15. Volkoff H, Peter RE. Effects of CART peptides on food consumption, feeding and associated behaviors in the goldfish, Carassius auratus: actions on neuropeptide Y- and orexin A-induced feeding. Brain Res. (2000) 887:125–33. doi: 10.1016/S0006-8993(00)03001-8
16. Ma A, He M, Bai J, Wong MK, Ko WK, Wong AO. Dual role of insulin in spexin regulation: functional link between food intake and spexin expression in a fish model. Endocrinology. (2017) 158:560–77. doi: 10.1210/en.2016-1534
17. Luckenbach JA, Murashige R, Daniels HV, Godwin J, Borski RJ. Temperature affects insulin-like growth factor I and growth of juvenile southern flounder, Paralichthys lethostigma. Comp Biochem Physiol A Mol Integr Physiol. (2007) 146:95–104. doi: 10.1016/j.cbpa.2006.09.024
18. Baroiller JF, D'cotta H, Bezault E, Wessels S, Hoerstgen-Schwark G. Tilapia sex determination: where temperature and genetics meet. Comp Biochem Physiol A Mol Integr Physiol. (2009) 153:30–8. doi: 10.1016/j.cbpa.2008.11.018
19. David D, Degani G. Temperature affects brain and pituitary gene expression related to reproduction and growth in the male blue gouramis, Trichogaster trichopterus. J Exp Zool A Ecol Genet Physiol. (2011) 315:203–14. doi: 10.1002/jez.663
20. Kullgren A, Jutfelt F, Fontanillas R, Sundell K, Samuelsson L, Wiklander K, et al. The impact of temperature on the metabolome and endocrine metabolic signals in Atlantic salmon (Salmo salar). Comp Biochem Physiol A Mol Integr Physiol. (2013) 164:44–53. doi: 10.1016/j.cbpa.2012.10.005
21. Lopez-Olmeda JF, Sanchez-Vazquez FJ. Zebrafish temperature selection and synchronization of locomotor activity circadian rhythm to ahemeral cycles of light and temperature. Chronobiol Int. (2009) 26:200–18. doi: 10.1080/07420520902765928
22. Qin G, Johnson C, Zhang Y, Zhang H, Yin J, Miller G, et al. Temperature-induced physiological stress and reproductive characteristics of the migratory seahorse Hippocampus erectus during a thermal stress simulation. Biol Open. (2018) 7:bio032888. doi: 10.1242/bio.032888.
23. Osterauer R, Kohler HR. Temperature-dependent effects of the pesticides thiacloprid and diazinon on the embryonic development of zebrafish (Danio rerio). Aquat Toxicol. (2008) 86:485–94. doi: 10.1016/j.aquatox.2007.12.013
24. Mateus AP, Costa RA, Cardoso JCR, Andree KB, Estevez A, Gisbert E, et al. Thermal imprinting modifies adult stress and innate immune responsiveness in the teleost sea bream. J Endocrinol. (2017) 233:381–94. doi: 10.1530/JOE-16-0610
25. Lall SP, Tibbetts SM. Nutrition, feeding, and behavior of fish. Vet Clin North Am Exot Anim Pract. (2009) 12:361–372, xi. doi: 10.1016/j.cvex.2009.01.005
26. Serisier S, Feugier A, Delmotte S, Biourge V, German AJ. Seasonal variation in the voluntary food intake of domesticated cats (Felis catus). PLoS ONE. (2014) 9:e96071. doi: 10.1371/journal.pone.0096071
27. Buentello JA, Gatlin DM, Neill WH. Effects of water temperature and dissolved oxygen on daily feed consumption, feed utilization and growth of channel catfish (Ictalurus punctatus). Aquaculture. (2000) 182:339–52. doi: 10.1016/S0044-8486(99)00274-4
28. Jonassen TM, Imsland AT, Kadowaki S, Stefansson SO. Interaction of temperature and photoperiod on growth of Atlantic halibut Hippoglossus hippoglossus L. Aquacul Res. (2000) 31:219–27. doi: 10.1046/j.1365-2109.2000.00447.x
29. Guderley H, Leroy PH, Gagne A. Thermal acclimation, growth, and burst swimming of threespine stickleback: enzymatic correlates and influence of photoperiod. Physiol Biochem Zool. (2001) 74:66–74. doi: 10.1086/319313
30. Burel C, Personleruyet J, Gaumet F, Leroux A, Severe A, Boeuf G. Effects of temperature on growth and metabolism in juvenile turbot. J Fish Biol. (1996) 49:678–92. doi: 10.1111/j.1095-8649.1996.tb00064.x
31. Guijarro AI, Delgado MJ, Pinillos ML, Lopez-Patino MA, Alonso-Bedate M, De Pedro N. Galanin and beta-endorphin as feeding regulators in cyprinids: effect of temperature. Aquacul Res. (1999) 30:483–9. doi: 10.1046/j.1365-2109.1999.00360.x
32. Babichuk NA, Volkoff H. Changes in expression of appetite-regulating hormones in the cunner (Tautogolabrus adspersus) during short-term fasting and winter torpor. Physiol Behav. (2013) 120:54–63. doi: 10.1016/j.physbeh.2013.06.022
33. Narnaware YK, Peyon PP, Lin X, Peter RE. Regulation of food intake by neuropeptide Y in goldfish. Am J Physiol Regul Integr Comp Physiol. (2000) 279:R1025–34. doi: 10.1152/ajpregu.2000.279.3.R1025
34. Folkedal O, Torgersen T, Olsen RE, Ferno A, Nilsson J, Oppedal F, et al. Duration of effects of acute environmental changes on food anticipatory behaviour, feed intake, oxygen consumption, and cortisol release in Atlantic salmon parr. Physiol Behav. (2012) 105:283–91. doi: 10.1016/j.physbeh.2011.07.015
35. Faure C, Charlot K, Henri S, Hardy-Dessources MD, Hue O, Antoine-Jonville S. Effect of heat exposure and exercise on food intake regulation: a randomized crossover study in young healthy men. Metabolism. (2016) 65:1541–9. doi: 10.1016/j.metabol.2016.07.004
36. Lei L, Hepeng L, Xianlei L, Hongchao J, Hai L, Sheikhahmadi A, et al. Effects of acute heat stress on gene expression of brain-gut neuropeptides in broiler chickens. J Anim Sci. (2013) 91:5194–201. doi: 10.2527/jas.2013-6538
37. Zhao Y, Boulant JA. Temperature effects on neuronal membrane potentials and inward currents in rat hypothalamic tissue slices. J Physiol. (2005) 564:245–57. doi: 10.1113/jphysiol.2004.075473
38. Dhaka A, Viswanath V, Patapoutian A. TRP ion channels and temperature sensation. Annu Rev Neurosci. (2006) 29:135–61. doi: 10.1146/annurev.neuro.29.051605.112958
39. Yan A, Zhang L, Tang Z, Zhang Y, Qin C, Li B, et al. Orange-spotted grouper (Epinephelus coioides) orexin: molecular cloning, tissue expression, ontogeny, daily rhythm and regulation of NPY gene expression. Peptides. (2011) 32:1363–70. doi: 10.1016/j.peptides.2011.05.004
40. Forlano PM, Cone RD. Conserved neurochemical pathways involved in hypothalamic control of energy homeostasis. J Comp Neurol. (2007) 505:235–48. doi: 10.1002/cne.21447
41. Volkoff H, Wyatt JL. Apelin in goldfish (Carassius auratus): cloning, distribution and role in appetite regulation. Peptides. (2009) 30:1434–40. doi: 10.1016/j.peptides.2009.04.020
42. Unniappan S, Lin X, Cervini L, Rivier J, Kaiya H, Kangawa K, et al. Goldfish ghrelin: molecular characterization of the complementary deoxyribonucleic acid, partial gene structure and evidence for its stimulatory role in food intake. Endocrinology. (2002) 143:4143–6. doi: 10.1210/en.2002-220644
43. Kang KS, Yahashi S, Azuma M, Matsuda K. The anorexigenic effect of cholecystokinin octapeptide in a goldfish model is mediated by the vagal afferent and subsequently through the melanocortin- and corticotropin-releasing hormone-signaling pathways. Peptides. (2010) 31:2130–4. doi: 10.1016/j.peptides.2010.07.019
44. Kehoe AS, Volkoff H. Cloning and characterization of neuropeptide Y (NPY) and cocaine and amphetamine regulated transcript (CART) in Atlantic cod (Gadus morhua). Comp Biochem Physiol A Mol Integr Physiol. (2007) 146:451–61. doi: 10.1016/j.cbpa.2006.12.026
45. Shimakura S, Miura T, Maruyama K, Nakamachi T, Uchiyama M, Kageyama H, et al. Alpha-melanocyte-stimulating hormone mediates melanin-concentrating hormone-induced anorexigenic action in goldfish. Horm Behav. (2008) 53:323–8. doi: 10.1016/j.yhbeh.2007.10.009
46. Matsuda K, Kojima K, Shimakura SI, Miura T, Uchiyama M, Ando H, et al. Relationship between melanin-concentrating hormone- and neuropeptide Y-containing neurons in the goldfish hypothalamus. Comp Biochem Physiol A Mol Integr Physiol. (2009) 153:3–7. doi: 10.1016/j.cbpa.2008.10.002
47. Yan AF, Chen T, Chen S, Ren CH, Hu CQ, Cai YM, et al. Goldfish leptin-AI and leptin-AII: function and central mechanism in feeding control. Int J Mol Sci. (2016) 17:E783. doi: 10.3390/ijms17060783.
48. Zhang H, Qin G, Sun J, Zhang B, Lin Q. The evolution and functional characterization of lined seahorse (Hippocampus erectus) CCKs involved in fasting and thermal stress response. Gen Comp Endocrinol. (2018) 255:56–63. doi: 10.1016/j.ygcen.2017.10.006
49. Froiland E, Murashita K, Jorgensen EH, Kurokawa T. Leptin and ghrelin in anadromous Arctic charr: cloning and change in expressions during a seasonal feeding cycle. Genl Comp Endocrinol. (2010) 165:136–43. doi: 10.1016/j.ygcen.2009.06.010
50. Trombley S, Mustafa A, Schmitz M. Regulation of the seasonal leptin and leptin receptor expression profile during early sexual maturation and feed restriction in male Atlantic salmon, Salmo salar L., parr. Gen Comp Endocrinol. (2014) 204:60–70. doi: 10.1016/j.ygcen.2014.04.033
51. Lohmus M, Raven PA, Sundstrom LF, Devlin RH. Disruption of seasonality in growth hormone-transgenic coho salmon (Oncorhynchus kisutch) and the role of cholecystokinin in seasonal feeding behavior. Horm Behav. (2008) 54:506–13. doi: 10.1016/j.yhbeh.2008.02.010
52. Singh Nee Priyadarshini P, Lal B. Seasonal variations in cellular expression of neuropeptide Y (NPY) in testis of the catfish, Clarias batrachus and its potential role in regulation of steroidogenesis. Peptides. (2018) 103:19–25. doi: 10.1016/j.peptides.2018.03.008
53. Ronnestad I, Nilsen TO, Murashita K, Angotzi AR, Gamst Moen AG, Stefansson SO, et al. Leptin and leptin receptor genes in Atlantic salmon: cloning, phylogeny, tissue distribution and expression correlated to long-term feeding status. Gen Comp Endocrinol. (2010) 168:55–70. doi: 10.1016/j.ygcen.2010.04.010
54. Huising MO, Geven EJW, Kruiswijk CP, Nabuurs SB, Stolte EH, Spanings F, et al. Increased leptin expression in common carp (Cyprinus carpio) after food intake but not after fasting or feeding to satiation. Endocrinology. (2006) 147:5786–97. doi: 10.1210/en.2006-0824
55. Gorissen M, Bernier NJ, Nabuurs SB, Flik G, Huising MO. Two divergent leptin paralogues in zebrafish (Danio rerio) that originate early in teleostean evolution. J Endocrinol. (2009) 201:329–39. doi: 10.1677/JOE-09-0034
56. Kurokawa T, Uji S, Suzuki T. Identification of cDNA coding for a homologue to mammalian leptin from pufferfish, Takifugu rubripes. Peptides. (2005) 26:745–50. doi: 10.1016/j.peptides.2004.12.017
57. Volkoff H, Eykelbosh AJ, Peter RE. Role of leptin in the control of feeding of goldfish Carassius auratus: interactions with cholecystokinin, neuropeptide Y and orexin A, and modulation by fasting. Brain Res. (2003) 972:90–109. doi: 10.1016/S0006-8993(03)02507-1
58. Murashita K, Uji S, Yamamoto T, Ronnestad I, Kurokawa T. Production of recombinant leptin and its effects on food intake in rainbow trout (Oncorhynchus mykiss). Comp Biochem Physiol B Biochem Mol Biol. (2008) 150:377–84. doi: 10.1016/j.cbpb.2008.04.007
59. Volkoff H, Canosa LF, Unniappan S, Cerda-Reverter JM, Bernier NJ, Kelly SP, et al. Neuropeptides and the control of food intake in fish. Gen Comp Endocrinol. (2005) 142:3–19. doi: 10.1016/j.ygcen.2004.11.001
60. Miura T, Maruyama K, Shimakura SI, Kaiya H, Uchiyama M, Kangawa K, et al. Regulation of food intake in the goldfish by interaction between ghrelin and orexin. Peptides. (2007) 28:1207–13. doi: 10.1016/j.peptides.2007.03.023
61. Fajardo O, Meseguer V, Belmonte C, Viana F. TRPA1 channels mediate cold temperature sensing in mammalian vagal sensory neurons: pharmacological and genetic evidence. J Neurosci. (2008) 28:7863–75. doi: 10.1523/JNEUROSCI.1696-08.2008
62. Volkoff H, Peter RE. Characterization of two forms of cocaine- and amphetamine-regulated transcript (CART) peptide precursors in goldfish: molecular cloning and distribution, modulation of expression by nutritional status, and interactions with leptin. Endocrinology. (2001) 142:5076–88. doi: 10.1210/endo.142.12.8519
63. Volkoff H. In vitro assessment of interactions between appetite-regulating peptides in brain of goldfish (Carassius auratus). Peptides. (2014) 61:61–8. doi: 10.1016/j.peptides.2014.09.002
64. Pinillos ML, De Pedro N, Alonso-Gomez AL, Alonso-Bedate M, Delgado MI. Food intake inhibition by melatonin in goldfish (Carassius auratus). Physiol Behav. (2001) 72:629–34. doi: 10.1016/S0031-9384(00)00399-1
65. Piccinetti CC, Migliarini B, Olivotto I, Simoniello MP, Giorgini E, and Carnevali O. Melantonin and peripheral circultries: insights on appeptite and metabolism in Danio rerio. Zebrafish. (2013) 10:275–82. doi: 10.1089/zeb.2012.0844
Keywords: appetite control, feeding behavior, temperature change, leptin and leptin receptor, orexigenic factors, anorexigenic factors, goldfish
Citation: Chen T, Wong MKH, Chan BCB and Wong AOL (2019) Mechanisms for Temperature Modulation of Feeding in Goldfish and Implications on Seasonal Changes in Feeding Behavior and Food Intake. Front. Endocrinol. 10:133. doi: 10.3389/fendo.2019.00133
Received: 05 December 2018; Accepted: 12 February 2019;
Published: 07 March 2019.
Edited by:
María Jesús Delgado, Complutense University of Madrid, SpainReviewed by:
Hélène Volkoff, Memorial University of Newfoundland, CanadaLingqing Zeng, Chongqing Normal University, China
Copyright © 2019 Chen, Wong, Chan and Wong. This is an open-access article distributed under the terms of the Creative Commons Attribution License (CC BY). The use, distribution or reproduction in other forums is permitted, provided the original author(s) and the copyright owner(s) are credited and that the original publication in this journal is cited, in accordance with accepted academic practice. No use, distribution or reproduction is permitted which does not comply with these terms.
*Correspondence: Anderson O. L. Wong, b2x3b25nQGhrdS5oaw==
†Present Address: Ting Chen, CAS Key Laboratory of Tropical Marine Bio-resources and Ecology, Guangdong Provincial Key Laboratory of Applied Marine Biology, South China Sea Institute of Oceanology, Chinese Academy of Sciences, Guangzhou, China