- 1Department of Experimental, Diagnostic and Specialty Medicine (DIMES), University of Bologna, Bologna, Italy
- 2Interdepartmental Centre “L. Galvani” (CIG), University of Bologna, Bologna, Italy
- 3Venetian Institute of Molecular Medicine, Padova, Italy
- 4Department of Biomedical Sciences, University of Padova, Padova, Italy
- 5IRCCS Istituto delle Scienze Neurologiche di Bologna, Bologna, Italy
- 6Lobachevsky State University of Nizhny Novgorod, Nizhny Novgorod, Russia
Human aging is characterized by dramatic changes in body mass composition that include a general increase of the total fat mass. Within the fat mass, a change in the proportions of adipose tissues also occurs with aging, affecting body metabolism, and playing a central role in many chronic diseases, including insulin resistance, obesity, cardiovascular diseases, and type II diabetes. In mammals, fat accumulates as white (WAT) and brown (BAT) adipose tissue, which differ both in morphology and function. While WAT is involved in lipid storage and immuno-endocrine responses, BAT is aimed at generating heat. With advancing age BAT declines, while WAT increases reaching the maximum peak by early old age and changes its distribution toward a higher proportion of visceral WAT. However, lipids tend to accumulate also within lipid droplets (LDs) in non-adipose tissues, including muscle, liver, and heart. The excess of such ectopic lipid deposition and the alteration of LD homeostasis contribute to the pathogenesis of the above-mentioned age-related diseases. It is not clear why age-associated tissue remodeling seems to lean toward lipid deposition as a “default program.” However, it can be noted that such remodeling is not inevitably detrimental. In fact, such a programmed redistribution of fat throughout life could be considered physiological and even protective, in particular at extreme old age. In this regard, it has to be considered that an excessive decrease of subcutaneous peripheral fat is associated with a pro-inflammatory status, and a decrease of LD is associated with lipotoxicity leading to an increased risk of insulin resistance, type II diabetes and cardiovascular diseases. At variance, a balanced rate of fat content and distribution has beneficial effects for health and metabolic homeostasis, positively affecting longevity. In this review, we will summarize the present knowledge on the mechanisms of the age-related changes in lipid distribution and we will discuss how fat mass negatively or positively impacts on human health and longevity.
Introduction
Aging is a complex process characterized by progressive changes in body mass composition that lead to a functional decline at cellular and organ levels over time. With advancing age, lean mass and bone mineral density decrease, while total fat mass increases and changes its distribution, particularly in the abdominal region, often without concomitant changes in body mass index (BMI) (1). In mammals, fat mass accumulates as adipose tissue or ectopic lipid deposition. Adipose tissue is a dynamic organ involved in the regulation of energy homeostasis, mainly divided in three types, brown (BAT), white (WAT), and BEIGE which differ in embryogenesis, anatomy, and function (2–4). While BAT possesses high levels of mitochondria and is specialized in fat burning to generate heat, WAT is characterized by a low density of mitochondria and it is generally involved in lipid storage in two biological distinct compartments: subcutaneous (SAT) and visceral (VAT) adipose tissue. WAT is not only involved in the storage of lipids, but also plays an important role as immuno-endocrine organ (5). With advancing age, BAT mass declines, while WAT increases reaching the maximum peak by early old age and changing its distribution toward a higher proportion of VAT (2). WAT redistribution is also accompanied by an accumulation of fat mass in non-adipose tissues and organs, such as muscle, liver, heart, pancreas and others, that normally contain only small amounts of fat, stored within lipid droplets (LDs) (6). Adipose tissue shows also an extraordinary plasticity (7), in fact it can differentiate into another type of adipose tissue, such as BEIGE (8, 9) or replace the parenchyma of organs that undergo involution with age, such as the thymus.
It is well-described that increased proportions of fat mass affect body metabolism and play a central role in many chronic diseases, including insulin resistance, obesity, cardiovascular diseases, type II diabetes, and sarcopenia (1, 10, 11).
In this review, we will summarize the changes that occur in lipid distribution with increasing age, and we will propose that: (i) the generalized increased amount of fat (in form of adipose tissue or intracellular lipid droplets) should be interpreted as an adaptive response to environmental conditions and, as such, is not per se a detrimental phenomenon; (ii) it can be a case of antagonistic pleiotropy, i.e., while it has detrimental effects at old age, it can turn to be protective in extreme longevity.
The Role of Fat Mass in the Evolution and During AGING
Body fat storage has a long evolutionary history and represents a fundamental strategy to store energy fuel that is crucial for survival in conditions where food is not continuously available (12). All living organisms, from prokaryotes to mammals, have the ability to store energy that can be mobilized in response to a need, such as growth, metabolism, and reproduction (13). While simple organisms obtain and use energy only in response to an immediate need, more complex organisms have developed a mechanism to store energy in form of adipose tissue or ectopic lipid deposition (5). In insects, fat bodies represent not only lipid deposits but also organs able to perform complex endocrine and exocrine functions similar to those of the liver (14). Fat bodies play their biosynthetic and metabolic activities by the production of circulating proteins, acting as hormones, necessary in several physiological conditions, such as morphogenesis, egg maturation, and lipid and carbohydrate metabolism (15).
In mammals, fat mass distribution has reached a quite high degree of complexity. In these organisms, fat mass is widely distributed in the whole body and it is involved in many physiological processes, i.e., energy supply during periods of starvation or undernutrition, regulation of metabolic homeostasis, reproduction, thermoregulation, immune response with production of cytokines and chemokines (13, 16). However, fat mass does not remain constant during lifespan, but changes in content and distribution from birth to extreme old age (17). These changes regard both adipose tissue and intracellular lipid stores (LDs) in non-adipose sites, and some of them, as in the case of thymic involution occurring at puberty, have to be considered as physiologically programmed. Much less is known about the fat mass changes occurring at advanced age. The “thrifty genotype theory” (18) explains the accumulation of adipose tissue as a strategy of metabolic adaptation, shaped by natural selection, to survive conditions of food scarcity. However, the existence of a specific genetic program leading to lipid deposition during aging is unlikely, considering that, according to the more advanced theories, aging in mammals is neither programmed nor selected by evolution. Thus, it seems more plausible that the age-associated lipid deposition is rather a phenomenon of adaptive remodeling in response to environmental conditions. Like many other adaptive phenomena it is conceivable that it may have both beneficial and detrimental effects. A growing body of evidence demonstrates that high levels of fat mass are associated with the development of several metabolic diseases (10), for this reason very often the fat deposition is considered tout court purely detrimental for the organism, and every age-associated weight gain bad for health. However, several lines of evidence demonstrate that also the deficiency of adipose tissue, as observed in transgenic mice or during lipodystrophy, results in the development of metabolic dysfunctions (19–21). For example, human lipodystrophies are characterized by genetic defects in lipid storage with total or partial loss of fat mass and related metabolic abnormalities such as insulin resistance and hypertension (22). Moreover, transgenic mice expressing dominant-negative protein A-ZIP/F (19) are characterized by the absence of WAT, reduced amount of BAT, elevated serum glucose, insulin, free fatty acids (FFA), triglycerides and type II diabetes (19). Consistently, adipose tissue results to have beneficial protective effects toward metabolic syndromes (19, 23–25). We argue that also during aging fat deposition can have beneficial effects, in particular at very old age, when it can be an important reserve of strategic energy crucial for resilience and recovery from stress and, eventually, for survival.
Fat Mass Distribution in Human Aging
In normal aging total fat mass increases over the adult lifespan with a peak at about 65–70 years, while in extreme old age fat mass decreases (26). In the following paragraphs, we will describe the different types of body fat mass (adipose tissue and ectopic lipid depots) and the re-distribution of such fat mass during aging.
White Adipose Tissue (WAT)
WAT is a complex tissue composed by unilocular adipocytes, other cellular types, such as immune and stem cells, and connective tissue (4). The main role of WAT is the storage of energy and, as such, it actively controls the energy metabolism of all organs and tissues. In fact WAT secretes cytokines and proteins that communicate with other organs, such as brain, liver, muscle, or pancreas (27). As mentioned in the introduction, the two major WAT depots are SAT and VAT. In human body, SAT is present in the hypodermis of abdominal, gluteal, and femoral districts, while the counterpart VAT resides within abdominal cavity (omental, mesenteric, retroperitoneal, gonadal fat) and mediastinum. Moreover, VAT is also present around specific organs such as heart, stomach and blood vessels. With aging WAT increases and re-distributes, in particular a relative decline of SAT in the abdomen and limb region (thigh, calve), and a concomitant increase of VAT can be appreciated (4, 28). While SAT is considered protective, being the main source of adiponectin (29), VAT is considered detrimental as it produces pro-inflammatory mediators such as leptin, that boost the status of chronic, subclinical inflammation typically found in the elderly and indicated as inflammaging (30). Aging also entails a dysregulation in WAT and a consequent excess of circulating FFA. Higher levels of circulating FFA lead to lipotoxicity and the development of metabolic disorders (31, 32).
Overall, WAT is involved in several physiological processes, as demonstrated by different studies on animal models. Studies on leptin-deficient ob/ob mice, with metabolic and immune dysfunctions, demonstrated that the transplantation of WAT normalizes high glucose levels, body weight and fertility (33), as well as thymus/spleen cellularity and inflammatory parameters like IL-6 (21). Moreover, a novel protective role for WAT in the immune response has been proposed (34). In particular, WAT from mice infected with bacteria represents a reservoir of memory T cell populations and promotes a protective memory response to infections (34). Studies in humans, and in particular in healthy centenarians, demonstrated that WAT becomes crucial in the extreme old age, as it secretes circulating factors such as adiponectin, that are associated with a protective metabolic and anti-inflammatory phenotype (35, 36).
As a whole, all these data suggest that WAT remodeling with aging may have not only negative but also positive effects on health.
Brown Adipose Tissue (BAT)
BAT is a highly vascularized, heat-producing tissue, aimed at protecting animals from hypothermia through thermogenesis. This role is prominent in small size animals and newborns. It is distributed in cervical, supraclavicular, axillary, paravertebral, mediastinal, and upper abdominal regions (4). BAT is characterized by the presence of multilocular adipocytes containing abundant mitochondria that express high levels of UCP1 through which dissipate the proton gradient across the inner membrane and produce heat (37, 38). In addition to the traditional role of BAT in thermogenesis, recent data suggest that BAT plays an important endocrine role through the release of several endocrine factors, particularly in response to thermogenic activation (39). All these signaling molecules control metabolic processes via autocrine, paracrine, and endocrine mechanisms. These factors include (i) vascular endothelial growth factors (VEGFs) and insulin-like growth factor I (IGF-I), which, respectively, favor angiogenesis and increase the number of brown adipocyte precursor cells; (ii) several bone morphogenetic proteins (BMPs), implicated in the regulation of adipocyte differentiation and energy expenditure; (iii) thyroid hormone (triiodothyronine, T3), a well-recognized regulator of thermogenesis; iv. interleukin-6 (IL-6) and fibroblast growth factor 21 (FGF21). Although IL-6 is commonly considered a pro-inflammatory cytokine, studies of BAT transplantation demonstrate the beneficial effects of IL-6 derived from BAT in the control of metabolism (40). IL-6 is a key mediator to improve glucose homeostasis and insulin sensitivity, and contributes to the increase of circulating levels of FGF21 that plays an important role in the control of glucose and lipid metabolism (41).
In humans, BAT is abundant in newborns and infants, while it gradually declines from adolescence to adulthood (42, 43). In adults the amount of BAT is modulated by several factors, such as hormones, physical activity, cold exposure, and diet (44, 45), however the responsiveness to these stimuli declines during aging (46). Due to the endocrine role of BAT, several evidences demonstrate that its induction plays a protective role in counteracting age-related metabolic diseases. While the loss of BAT predisposes to WAT accumulation and weight gain (47), the transplantation of BAT in murine models induces an enhancement of energy expenditure, weight loss and insulin sensitivity, and prevents or even reverses obesity (48, 49). It was also shown that caloric restriction is able to stimulate BAT growth, conferring protection against the major age-related pathologies, such as cardiovascular diseases, cancer, and neurodegenerative disorders (50, 51). Moreover, increased levels of BAT mass promote longevity and enhance metabolism (52).
Beige Adipose Tissue and Browning
BEIGE or BRITE (“brown in white”) adipose tissue is a subset of WAT with features of BAT. In particular, BEIGE tissue is composed of adipocytes derived from differentiation of WAT pre-adipocytes or trans-differentiation of WAT adipocytes, a phenomenon known as “WAT browning” (53, 54). In adults BEIGE tissue is localized within white fat depots, at inguinal and neck levels, where acts as WAT (55), while under particular stimuli, beige adipocytes acquire brown-like functions (56). The stimuli inducing the browning are not still well-understood, however it is known that they influence several molecular factors to orchestrate browning and thermogenesis mechanisms. Briefly, browning and thermogenesis are generally mediated by chronic cold exposure or hormones and peptide factors that activate the β-3-adrenergic receptor, an adipose tissue-selective adrenergic receptor (57). Moreover, the zinc finger protein PRDM16 and the mitochondrial protein UCP1 are considered the key contributors to prompt browning activity in the new BEIGE tissue (58). Noteworthy, a reduced expression of PRDM16 and UCP1 leads to the reversion of beige adipocytes into white adipocytes (59–61). Browning is a reversible mechanism pointing to the extraordinary plasticity of the adipose tissue. The process of browning is impaired during aging leading to a loss of BEIGE tissue and to a progressive decline in metabolic activity. This phenomenon is in part due to a reduction in the response to the β-adrenergic stimuli with aging (62). In fact, some studies have demonstrated that the loss of β-3-adrenergic receptor leads to an incapacity of white adipocytes to differentiate in beige adipocytes upon cold exposure (63, 64). Other studies in knockout or transgenic mice for different molecular regulators of browning underline the importance of this thermogenic tissue on age-associated metabolic dysregulations. As an example, in old mice the ablation of the winged helix factor FOXA3, a factor inducing the increase of adiposity and decrease of BAT mass with aging, induces browning, increases thermogenic capacity, decreases WAT expansion, thus leading to improved insulin sensitivity and lifespan extension (65). Other factors involved in the formation of brown adipocytes and in the regulation of lifespan are the BMPs. For example, BMP4, BMP7, and BMP8b control beige adipocyte development. In particular, mice treated with these factors increase browning processes and are protected from insulin resistance (29, 66).
Ectopic Lipid Depots
Several tissues, such as bone marrow, skeletal muscle, liver and pancreas, make use of fatty acids as energy source, and these fatty acids accumulate within LDs in form of neutral lipids, mainly as triacylglycerols (TAGs) and steryl esters. LDs play an evolutionarily conserved role from yeasts to multicellular eukaryotic organisms (67). The physiological role of LDs is the maintenance of cellular energy homeostasis and the protection from lipotoxicity caused by an excess of FFA accumulation (68). An alteration in LD homeostasis leads to an excess of lipid intermediates, such as diacylglycerols, which in turn perturb metabolic pathways and cellular functions causing inflammation, mitochondrial stress and increase of reactive oxygen species (ROS) (68). The amount and size of LDs increase with age and contribute to the development of several age-related metabolic diseases such as type II diabetes, obesity, hepatic steatosis, and sarcopenia (68–75). Nevertheless, the primary causes of age-dependent ectopic fat accumulation remain largely unknown. However, not only the excess but also the deficiency of LDs leads to the onset of metabolic disorders (6, 20), in fact a growing body of evidence suggests that a balance between neutral lipid accumulation and their degradation is essential for the health and longevity of the organism. Numerous studies of model organisms, such as yeasts, nematodes, insects, and mice indicate that LDs are involved in the regulation of several longevity-related mechanisms, most of which are evolutionary conserved. It has been demonstrated that LDs control metabolic and lipid homeostasis and stimulate the release of different molecular mediators, such as lipophilic hormones that act as aging regulators and promote longevity (76). As an example, LD accumulation in the intestine of Caenorhabditis elegans contributes to the secretion of hormonal steroid pregnenolone, also present in humans, that extends lifespan (77–79). Consistently, the accumulation of TAGs has been reported as a novel pro-longevity factor in yeast. In fact, genetic manipulations leading to an increase in TAG content (by either the decrease of TAG lipases or the increase of TAG biosynthesis enzymes), extend yeast chronological lifespan (80, 81) independently of other lifespan regulators, such as dietary restriction (82). Moreover, LDs are involved in the adaptive stress responses and cell survival by the production of signaling molecules involved in the immune response pathway (83). As an example, in humans LDs serve as main storage site for arachidonic acid, which is the precursor of signaling molecules, such as eicosanoids or retinoic acids, which regulate inflammation (84).
All these findings indicate that the balance of fat accumulation in non-adipose tissues is important for the maintenance of a healthy status, in fact, both the excess and scarcity of fat are linked to the development of pathologies (20).
The Endocrine Role of Adipose Tissue During Aging
Adipose tissue is an important endocrine organ that controls numerous physiological functions such as appetite, body weight, insulin sensitivity, fat distribution, glucose and lipid metabolism, neuroendocrine functions. This endocrine activity influences the whole body metabolism by releasing FFA, adipokines, cytokines and other molecular factors (85) that play a pleiotropic function on different tissues such as the liver, skeletal muscle, heart, lung, blood vessels, and sensory receptors, such as olfactory ones. Recent studies demonstrate that olfactory system is closely linked to adipose tissue in the regulation of energy balance (86, 87). In particular, the olfactory mucosa and bulb are provided with receptors for adipose-derived factors, such as leptin and adiponectin through which they influence body metabolism (88). How the age-related changes in adiposity modify olfactory function remains unclear. However, it is reasonable to think that the adipose tissue dysfunction with aging may affect energy homeostasis by perturbing the olfactory system through the production of these adipokines, and, in turn, outputs from the olfactory tract are among the causes of adipose tissue modifications.
WAT regulates also immune functions. In this regards, a link between white adiposity (in particular of VAT) and immune aging was found. In WAT, there are different types of immune cells that under stress conditions, such as obesity, impair their immune capacity increasing the pro-inflammatory behaviors in adipose tissue (89). Pro-inflammatory mediators can be produced also by senescent cells that accumulate in adipose tissue during aging (10). As for the olfactory system, the regulation of immune cell function by the adipose tissue depends on the secretion of adipokines such as leptin and adiponectin, that were originally classified as pro- or anti-inflammatory, respectively. The primary function of leptin is the regulation of appetite and energy expenditure by acting at the level of the central nervous system. It is known that higher circulating levels of leptin are present in obese and overfeeding individuals (90, 91). However, studies in ob/ob mice have demonstrated that the absence of leptin leads to obesity, hyperlipidaemia and insulin resistance, while leptin administration reverses these metabolic perturbations (92, 93). Accordingly, leptin administration has been proposed as a possible therapy to ameliorate glycemic control and dyslipidaemia in human patients affected by lipodystrophy or congenital leptin deficiency (94, 95). Likewise, adiponectin displays protective metabolic functions such as stimulating fatty acid oxidation and improving insulin sensitivity (96). High plasma levels of adiponectin are associated with low fat mass (97) and low incidence of metabolic disorders, including type II diabetes (98). Thus, leptin and adiponectin play different roles that converge on the maintenance of balanced energy levels and fat stores. Data from animal models highlight the critical functions of adipokines in metabolic homeostasis and longevity. In particular, these murine models, characterized by mutation or knockout in genes involved in metabolism, exhibit an extended lifespan associated to higher levels of plasma adiponectin, reduced adiposity, and lower-fasting insulin concentration (99–104). All these findings indicate that there is a clear association between elevated circulating levels of adiponectin and longevity, where the key mediator appears to be the maintenance of adiponectin-dependent insulin sensitivity. A recent cross-sectional study in caloric restricted mice of different age shows that longevity phenotype is linked to a specific adiponectin isoform with high molecular weight (105). This isoform was recognized as the most effective in enhancing the insulin sensitivity in humans (106). It is reported that the circulating levels of adiponectin and leptin (only in males), but not of resistin, increase with age (107). Noteworthy, also centenarians display higher levels of adiponectin associated to preserved insulin sensitivity. In particular, high adiponectin blood levels are associated with high levels of HDL-cholesterol and low levels of glycated hemoglobin (HbA1c) and C-reactive protein (35). Moreover elevated adiponectin levels are inversely correlated with the HOMA-IR, a risk factor for the onset of age-related metabolic diseases (108, 109).
Another factor secreted by adipose tissue is FGF21, an important metabolic regulator. FGF21 is in fact involved in the transcription of adiponectin in WAT, and these two factors together act to control energy metabolism and insulin sensitivity in other organs, such as liver and skeletal muscle (110, 111). FGF21 increases insulin sensitivity, energy expenditure, and weight loss also by inducing browning activity (112). Moreover, FGF21 has been also proposed as an antiaging hormone, in fact the overexpression of FGF21 in mice extends the lifespan (113). However, very recently we found that circulating levels of FGF21 in humans increase with age from 21 to 100+ years in healthy individuals and these high levels are related to worsened biochemical health parameters in old persons and decreased survival in extreme longevity (114). Accordingly, the role of FGF21 as a pro-longevity hormone has been questioned, as it appears to be responsible for the phenotype of accelerated aging in Opa1-deficient mice (115). This apparent contradiction could be explained in the framework of the hormetic paradigm. In other words, FGF21 is per se protective and likely able to recover a mild stress, but when the stress becomes stronger, it overcomes the beneficial effects of FGF21 and possibly an excessive production of FGF21 can have detrimental effects on the health status (114). WAT secretes also various bioactive compounds, indicated as Volatile Organic Compounds (VOCs) (116). VOCs are low-weight molecules produced by cellular metabolism that are involved in different physiological processes. VOCs mirror normal or abnormal physiological processes and reflect the metabolic condition of the organism and might have promise as diagnostic tools for a number of diseases as well as the metabolic condition of elderly people, as they seem to be characterized by a modified production of VOCs (117–119). Human fat can produce consistent amounts of VOCs detectable in breath, skin and sweat, and fluids, such as blood, urine, saliva, and in feces. It has been demonstrated that metabolic disorders or other diseases are characterized by specific VOCs profiles (120). Moreover, recent data demonstrate that the exposure to a new profile of VOCs contributes to an increase of pro-inflammatory cytokines involved in the development of metabolic diseases (116, 121). Therefore, it is reasonable to think that the age-related rearrangement of adipose tissue is at least in part responsible not only of the alteration of fat hormones production, but also of VOCs profiles, with at present unpredictable effects on the health status.
As a whole, adipose tissue and its derived adipokines have a critical role in controlling whole body energy metabolism, as well as some of the major age-related dysfunctions and longevity.
Organ Involution and Fat Infiltration With Aging
One of the most universal manifestations of aging is the progressive atrophy or involution of many organs and tissues. This phenomenon is characterized by a loss of mass due to the concomitant reduction of cell proliferation and the increase of cell death rates, together with the accumulation of senescent cells (122). In some cases, this age-related loss of organ parenchyma does not lead to mere organ shrinkage, but is rather paralleled by an infiltration of adipocytes and sometimes by a complete replacement of organ with adipose tissue (122). The reasons why the age-related organ involution is accompanied by a replacement of adipose tissue remains poorly understood. As described above, adipose tissue has a crucial role in metabolic processes. Therefore, the adipose tissue substitution during organ involution is not a simple replacement with a neutral tissue, but it has to be considered as an adaptive response with dramatic biological consequences, as adipose tissue plays an active role as a source of hormones and adipokines that may regulate the homeostasis of the adjacent tissues (2, 91, 123). The substitution of parenchyma with adipose tissue is particularly evident in thymus, bone marrow, but also in muscle and pancreas, and generally begins between 20 and 50 years of age, excepting the thymic involution that begins much earlier, around late infancy-puberty (122). However, all human organs are gradually invaded by fat cells from birth onwards.
Thymus and bone marrow are two organs highly subjected to age-associated changes. They represent primary lymphoid organs, playing a fundamental role to provide cellular components of immune system during lifespan (124). The thymus is responsible for thymocyte differentiation and maturation into T cells, while bone marrow is the main site for the generation of all blood cells and for the maturation of B cells. The thymus progressively loses its functionality with age, in a process termed thymic involution or atrophy (125, 126). This phenomenon begins relatively soon after birth and results in a significant loss in thymic mass and a replacement with WAT within the thymus but also in the peripheral areas (127, 128). The organ replacement with WAT seems to occur in all species that possess the thymus indicating that this process is not only evolutionary ancient and conserved (129) but also important to counterbalance the loss of thymus by maintaining the size of this organ throughout life (125).
Aging induces adipocyte accumulation also in bone marrow cavities resulting in a loss of hemopoietic activity and bone loss disorders. Mesenchymal stem cells (MSC) of bone marrow can give rise to adipocytes or bone-forming osteoblasts. With age, these MSC tend to differentiate more into adipocytes that can become the prevalent cellular population of the marrow. Several studies suggest that this process leads to an impairment of osteogenic and hematopoietic activities (130–132) and is associated with the development of a great number of age-related diseases, such as osteoporosis and type II diabetes (133, 134). It is however suggested that the presence of adipose tissue in the marrow is necessary and beneficial for the health of the bone, especially when it has features of BAT (135).
As previously mentioned, aging is also associated with adipose tissue infiltration at non-adipose sites that normally are not involved in the fat storage. This age-related ectopic adiposity is associated with the progressive impairment of organs and tissues. This phenomenon affects, in particular, skeletal muscle, liver, pancreas, and heart. In skeletal muscle, elevated adipose deposition is observed at both intra- and inter-muscular sites. These two types of ectopic adiposity negatively impact on muscle strength and quality, as demonstrated in previously studies from our laboratory and others (75, 136, 137). In older people intra-muscular lipid content is associated with insulin resistance and the development of metabolic disorders (138). Moreover, in skeletal muscle, a subset of stem cells indicated as fibro-adipogenic progenitors (FAPs) appears to play a major role in inter-muscular adipose tissue (IMAT) infiltration, a phenomenon linked to progressive muscle dysfunction. FAPs appear to be driven toward adipogenic differentiation by muscle inactivity (139). It is therefore plausible to consider the increased adiposity of skeletal muscle observed with age as an adaptive response to mutated organismal requests.
Pancreas undergoes several alterations with aging, not only in volume but also structure. In particular, a significant reduction of pancreatic antero-posterior diameter, an increase of pancreatic lobulation and a decline of parenchymal component with a concomitant parenchymal fat mass increase (fatty replacement or lipomatosis) have been observed. This fatty replacement in pancreas is characterized by the infiltration of adipose tissue, as interlobular fat, between pancreatic lobules, accumulating around vessels (140, 141). The increase of pancreatic fat infiltration with aging is not well-understood and is still under debate. The degree of fatty replacement varies among subjects and depends on the condition, physiological, or pathological, in which each individual is involved. However, in any case, like in other organs, also the age-related atrophy of pancreas is associated to a replacement with fat mass.
In liver, an increase of intracellular fat mass in hepatocytes appears to be associated only with a progressive dysfunction of hepatic organ. Several evidence indicate that hepatic fat infiltration is associated with an increase of oxidative stress, inflammatory response, and cellular senescence, leading to the alteration of hepatic structure and the onset of non-alcoholic fatty liver diseases (NAFLD) (142, 143). Studies on the causes or effects of aging on hepatic adiposity are still limited. However, several evidence suggest that the progression of NAFLD is associated with telomere shortening, increased p21 expression and increased M1 macrophage inflammatory responses that are considered specific markers of cellular aging (144, 145). The increased expression levels of these markers are also observed in adipocytes, indicating that adipocytes under oxidative stress exhibit increased levels of ROS, shortened telomeres and switch to senescent/pro-inflammatory phenotype with the decline in insulin sensitivity (146).
In heart, the epicardial adiposity increases in size between myocardium and pericardium (147). In healthy conditions, cardiac adipose tissue has physiological functions, including metabolic, thermogenic and mechanical functions (148). New findings demonstrate that epicardial fat is mainly composed of adipocytes with features similar to brown or beige adipocytes (149), playing a protective role against the development of metabolic diseases (150). In particular, these adipocytes act through paracrine secretion of anti-atherogenic cytokines, such as adiponectin and adrenomedullin. However, the epicardial fat is susceptible to age-associated changes. In fact, with aging brown/beige adipose tissue undergoes a brown-to-white transition becoming dysfunctional and contributing to the onset of several pathologies.
All these data suggest that the increase of fat mass with age (including the increase of volume of adipose tissue, replacement of parenchyma and ectopic lipid deposition) is an apparently universal phenomenon, linked in general (but not exclusively) to the onset of pathological conditions (Figure 1). In fact, it is considered that adipocytes in adipose tissue do not change in number with age but rather in size, thus resembling the phenomenon occurring in obesity (151, 152). Adipocytes from obese persons are larger and unilocular, they release high amounts of FFA, produce less adiponectin and are infiltrated with M1 macrophages and produces pro-inflammatory cytokines (152), thus representing a risk factor for many age-associated diseases. The same seems to occur with age, as the large majority of the persons show a remodeling of adipose tissue with these features. In this regard, obesity could be considered as a sort of accelerated aging of the WAT. However, adipose tissue remodeling with age is likely an adaptive response to environmental conditions, therefore is not necessarily detrimental, and a preserved balanced metabolism can maintain a physiological remodeling of adipose tissue, without excessive WAT hypertrophy, but also without excessive loss of adiposity. This could be a key feature for achieving successful aging and longevity.
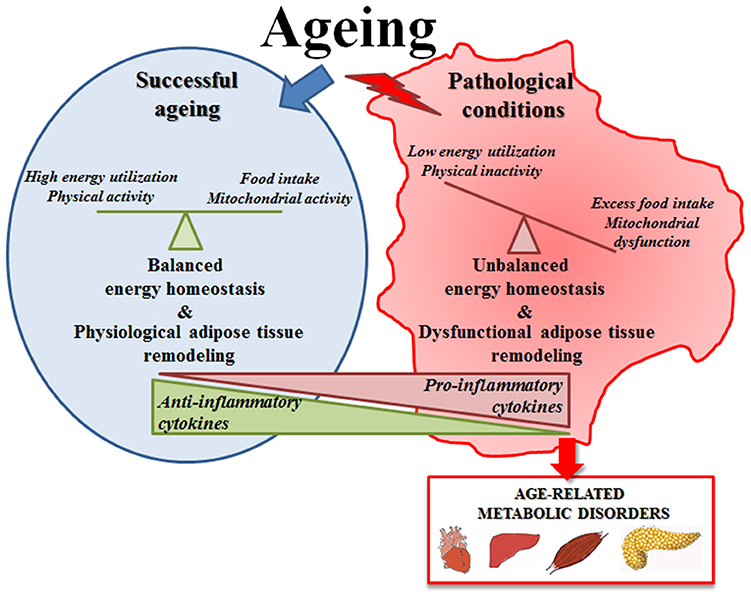
Figure 1. Schematic representation of adipose tissue function in successful aging or in pathological conditions. In successful aging a preserved balanced metabolism can maintain a physiological remodeling of adipose tissue that is likely an adaptive response to environmental conditions; in pathological conditions the unbalanced energy homeostasis leads to an excessive adipose tissue hypertrophy that becomes detrimental and contributes to the onset of age-related metabolic diseases.
Conclusions
The maintenance of a balanced amount of fat mass is crucial for health and survival, as discussed above. According to the “thrifty phenotype” theory, humans were selected to accumulate fat depots to face periods of food shortage. However, while a critical lower threshold of fat content exists, an upper threshold is apparently missing, and adipose tissue can accumulate in great amounts. The absence of an upper threshold for fat accumulation is probably due to the fact that this phenomenon did not occur in the wild frequently enough to undergo selection, or, alternatively, resulted neutral for the fitness of individuals.
With aging, the “thrifty phenotype” seems to emerge more dramatically, and the balance is tilted toward an increase of fat mass, at the level of VAT and SAT as well as in ectopic sites (liver, muscles, etc.) (Figure 2). This increase in fat deposition at the level of SAT and VAT can be considered an adaptive response to modified health conditions interacting with contingent environmental conditions, leading eventually to decreased energy expenditure. However, in some cases the storage of surplus energy can not be claimed as the reason for fat accumulation, especially when this occurs ectopically at the expenses of other tissue types with important vital functions, as in the case of thymic involution or skeletal muscle infiltration. In this case, it seems that fat deposition in form of WAT is a sort of physiological program (genetically determined?) for organs and tissues undergoing age-related atrophy or involution. We have mentioned the fact that different stem cell subpopulations such as muscle FAPs and bone marrow MSC preferentially differentiate to adipocyte with age, therefore, we are tempted to speculate that the pathway leading to this cell type is a sort of a default choice in involution processes. Should this speculation be verified, the reasons for this choice remain elusive.
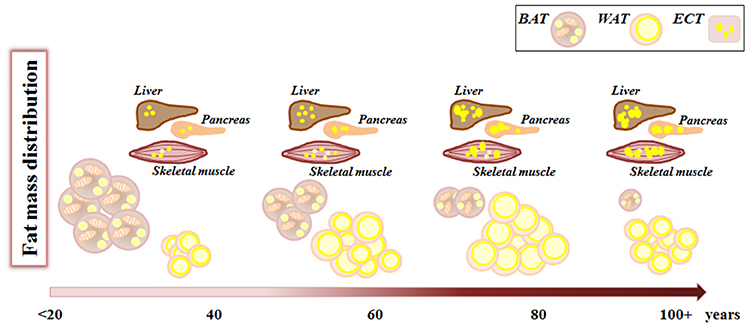
Figure 2. Fat mass remodeling with aging. During aging, from birth to advanced age, fat mass changes its amount and distribution. White adipose tissue (WAT) increases both in number and volume of adipocytes, while brown adipose tissue (BAT) decreases. Concomitantly there is an increase in lipid deposition in ectopic tissues (ECT) such as liver, muscles, pancreas where lipids are stored within lipid droplets.
The accumulation of WAT has been for long time viewed as detrimental, being the source of pro-inflammatory mediators and other important endocrine modulators and strongly associated with metabolic diseases such as insulin resistance and type II diabetes, cardiovascular diseases and cancer. However, it is not totally clear whether this negative role is present also in extreme old age. Actually, data on body composition in non-agenarians and centenarians are largely missing, even though the BMI of these people is usually lower than that of younger (70–80 years-old) persons. It is possible that, as for other risk factors like lipid serum profile and inflammatory parameters (153, 154), also the presence of a consistent amount of WAT can be important for survival at very advanced age. Further studies are needed to verify this hypothesis.
To sum up, human aging is characterized by a general tendency to increase adiposity, a phenomenon that in industrialized Countries can synergize with obesogenic conditions and become a health-threatening phenomenon. However, low adiposity is a risk factor too, as discussed, and a qualitatively adequate amount of fat is likely a key feature of long life.
Author Contributions
MC, MM and SS: concept, analysis of literature, and writing; MS and CF: analysis of literature and critical discussion.
Conflict of Interest Statement
The authors declare that the research was conducted in the absence of any commercial or financial relationships that could be construed as a potential conflict of interest.
The handling editor declared a shared affiliation, though no other collaboration, with several of the authors MC, MM, CF, SS.
Acknowledgments
This work has been partially supported by the Roberto and Cornelia Pallotti legacy for cancer research to SS; the European Union (EU)’S H2020 Project PROPAG-AGEING (grant agreement 634821), EU JPND ADAGE, EU FP7 HUMAN (grant agreement 602757), Russian Federation mega grant DPM-AGEING (grant 2017-220-06-4741) on Digitalized and Personalized Medicine of Healthy Aging, 2018–2021, at the Lobachevsky State University of Nizhny Novgorod to CF.
References
1. Kuk JL, Saunders TJ, Davidson LE, Ross R. Age-related changes in total and regional fat distribution. Ageing Res Rev. (2009) 8:339–48. doi: 10.1016/j.arr.2009.06.001
2. Saely CH, Geiger K, Drexel H. Brown versus white adipose tissue: a mini-review. Gerontology (2012) 58:15–23. doi: 10.1159/000321319
4. Cinti S. Obesity, Type 2 Diabetes and the Adipose Organ. Cham: Springer International Publishing AG (2018).
5. Ottaviani E, Malagoli D, Franceschi C. The evolution of the adipose tissue: a neglected enigma. Gen Comp Endocrinol. (2011) 174:1–4. doi: 10.1016/j.ygcen.2011.06.018
6. Onal G, Kutlu O, Gozuacik D, Dokmeci Emre S. Lipid droplets in health and disease. Lipids Health Dis. (2017) 16:128. doi: 10.1186/s12944-017-0521-7
7. Giordano A, Smorlesi A, Frontini A, Barbatelli G, Cinti S. White, brown and pink adipocytes: the extraordinary plasticity of the adipose organ. Eur J Endocrinol. (2014) 170:R159–71. doi: 10.1530/EJE-13-0945
8. Rui L. Brown and beige adipose tissues in health and disease. Compr Physiol. (2017) 7:1281–306. doi: 10.1002/cphy.c170001
9. Hepler C, Vishvanath L, Gupta RK. Sorting out adipocyte precursors and their role in physiology and disease. Genes. Dev. (2017) 31:127–40. doi: 10.1101/gad.293704.116
10. Tchkonia T, Morbeck DE, Von Zglinicki T, Van Deursen J, Lustgarten J, Scrable H, et al. Fat tissue, aging, and cellular senescence. Aging Cell. (2010) 9:667–84. doi: 10.1111/j.1474-9726.2010.00608.x
11. Buch A, Carmeli E, Boker LK, Marcus Y, Shefer G, Kis O, et al. Muscle function and fat content in relation to sarcopenia, obesity and frailty of old age–An overview. Exp Gerontol. (2016) 76:25–32. doi: 10.1016/j.exger.2016.01.008
12. Pond CM. An evolutionary and functional view of mammalian adipose tissue. Proc Nutr Soc. (1992) 51:367–77. doi: 10.1079/PNS19920050
13. Birsoy K, Festuccia WT, Laplante M. A comparative perspective on lipid storage in animals. J Cell Sci. (2013) 126:1541–52. doi: 10.1242/jcs.104992
14. Franchini A, Mandrioli M, Franceschi C, Ottaviani E. Morpho-functional changes of fat body in bacteria fed Drosophila melanogaster strains. J Mol Histol. (2012) 43:243–51. doi: 10.1007/s10735-011-9382-y
15. Arrese EL, Soulages JL. Insect fat body: energy, metabolism, and regulation. Annu Rev Entomol. (2010) 55:207–25. doi: 10.1146/annurev-ento-112408-085356
16. Norgan NG. The beneficial effects of body fat and adipose tissue in humans. Int J Obes Relat Metab Disord. (1997) 21:738–46. doi: 10.1038/sj.ijo.0800473
17. Zafon C. Oscillations in total body fat content through life: an evolutionary perspective. Obes Rev. (2007) 8:525–30. doi: 10.1111/j.1467-789X.2007.00377.x
18. Neel JV. Diabetes mellitus: a “thrifty” genotype rendered detrimental by “progress”? Am J Hum Genet. (1962) 14:353–62.
19. Moitra J, Mason MM, Olive M, Krylov D, Gavrilova O, Marcus-Samuels B, et al. Life without white fat: a transgenic mouse. Genes Dev. (1998) 12:3168–81.
20. Krahmer N, Farese RV Jr, Walther TC. Balancing the fat: lipid droplets and human disease. EMBO Mol Med. (2013) 5:973–83. doi: 10.1002/emmm.201100671
21. Sennello JA, Fayad R, Pini M, Gove ME, Fantuzzi G. Transplantation of wild-type white adipose tissue normalizes metabolic, immune and inflammatory alterations in leptin-deficient ob/ob mice. Cytokine (2007) 36:261–6. doi: 10.1016/j.cyto.2007.02.001
22. Huang-Doran I, Sleigh A, Rochford JJ, O’Rahilly S, Savage DB. Lipodystrophy: metabolic insights from a rare disorder. J Endocrinol. (2010) 207:245–55. doi: 10.1677/JOE-10-0272
23. Gunawardana SC. Benefits of healthy adipose tissue in the treatment of diabetes. World J Diabetes (2014) 5:420–30. doi: 10.4239/wjd.v5.i4.420
24. Grundy SM. Adipose tissue and metabolic syndrome: too much, too little or neither. Eur J Clin Invest. (2015) 45:1209–17. doi: 10.1111/eci.12519
25. Akinci B, Sahinoz M, Oral E. Lipodystrophy Syndromes: Presentation and Treatment. 24. In: De Groot LJ, Chrousos G, Dungan K, Feingold KR, Grossman A, Hershman JM, editors. Endotext Dartmouth, MA: MDText.com, Inc. (2018).
26. Graja A, Schulz TJ. Mechanisms of aging-related impairment of brown adipocyte development and function. Gerontology (2015) 61:211–7. doi: 10.1159/000366557
27. Trayhurn P, Beattie JH. Physiological role of adipose tissue: white adipose tissue as an endocrine and secretory organ. Proc Nutr Soc. (2001) 60:329–39. doi: 10.1079/PNS200194
28. Schwartz RS, Jaeger LF, Veith RC, Lakshminarayan S. The effect of diet or exercise on plasma norepinephrine kinetics in moderately obese young men. Int J Obes. (1990) 14:1–11.
29. Schosserer M, Grillari J, Wolfrum C, Scheideler M. Age-induced changes in white, brite, and brown adipose depots: a mini-review. Gerontology (2018) 64:229–36. doi: 10.1159/000485183
30. Franceschi C, Bonafè M, Valensin S, Olivieri F, De Luca M, Ottaviani E, et al. Inflamm-aging. An evolutionary perspective on immunosenescence. Ann N Y Acad Sci. (2000) 908:244–54. doi: 10.1111/j.1749-6632.2000.tb06651.x
31. Després JP, Lemieux I. Abdominal obesity and metabolic syndrome. Nature (2006) 444:881–7. doi: 10.1038/nature05488
32. Gavi S, Stuart LM, Kelly P, Melendez MM, Mynarcik DC, Gelato MC, et al. Retinol-binding protein 4 is associated with insulin resistance and body fat distribution in nonobese subjects without type 2 diabetes. J Clin Endocrinol Metab. (2007) 92:1886–90. doi: 10.1210/jc.2006-1815
33. Klebanov S, Astle CM, DeSimone O, Ablamunits V, Harrison DE. Adipose tissue transplantation protects ob/ob mice from obesity, normalizes insulin sensitivity and restores fertility. J Endocrinol. (2005) 186:203–11. doi: 10.1677/joe.1.06150
34. Han SJ, Glatman Zaretsky A, Andrade-Oliveira V, Collins N, Dzutsev A, Shaik J, et al. White adipose tissue is a reservoir for memory T cells and promotes protective memory responses to infection. Immunity (2017) 47:1154–68.e6. doi: 10.1016/j.immuni.2017.11.009
35. Arai Y, Nakazawa S, Kojima T, Takayama M, Ebihara Y, Shimizu K, et al. High adiponectin concentration and its role for longevity in female centenarians. Geriatr Gerontol Int. (2006) 6:32–9. doi: 10.1111/j.1447-0594.2006.00304.x
36. Arai Y, Hirose N. Adiponectin and healthy aging in centenarians. Anti-Aging Med. (2012) 9:1–5. doi: 10.3793/jaam.9.1
37. Cannon B, Nedergaard J. Brown adipose tissue: function and physiological significance. Physiol Rev. (2004) 84:277–359. doi: 10.1152/physrev.00015.2003
38. Kwok KH, Lam KS, Xu A. Heterogeneity of white adipose tissue: molecular basis and clinical implications. Exp Mol Med. (2016) 48:e215. doi: 10.1038/emm.2016.5
39. Wang GX, Zhao XY, Lin JD. The brown fat secretome: metabolic functions beyond thermogenesis. Trends Endocrinol Metab. (2015) 26:231–7. doi: 10.1016/j.tem.2015.03.002
40. Stanford KI, Middelbeek RJ, Townsend KL, An D, Nygaard EB, Hitchcox KM, et al. Brown adipose tissue regulates glucose homeostasis and insulin sensitivity. J Clin Invest. (2013) 123:215–23. doi: 10.1172/JCI62308
41. Villarroya F, Giralt M. The beneficial effects of brown fat transplantation: further evidence of an endocrine role of brown adipose tissue. Endocrinology (2015) 156:2368–70. doi: 10.1210/en.2015-1423
42. Au-Yong IT, Thorn N, Ganatra R, Perkins AC, Symonds ME. Brown adipose tissue and seasonal variation in humans. Diabetes (2009) 58:2583–7. doi: 10.2337/db09-0833
43. Rogers NH. Brown adipose tissue during puberty and with aging. Ann Med. (2015) 47:142–9. doi: 10.3109/07853890.2014.914807
44. Cinti S. The role of brown adipose tissue in human obesity. Nutr Metab Cardiovasc Dis. (2006) 16:569–74. doi: 10.1016/j.numecd.2006.07.009
45. Kajimura S, Saito M. A new era in brown adipose tissue biology: molecular control of brown fat development and energy homeostasis. Annu Rev Physiol. (2014) 76:225–49. doi: 10.1146/annurev-physiol-021113-170252
46. Mattson MP. Perspective: does brown fat protect against diseases of aging? Ageing Res Rev. (2010) 9:69–76. doi: 10.1016/j.arr.2009.11.004
47. Hansen JB, Kristiansen K. Regulatory circuits controlling white versus brown adipocyte differentiation. Biochem J. (2006) 398:153–68. doi: 10.1042/BJ20060402
48. Zhu Z, Spicer EG, Gavini CK, Goudjo-Ako AJ, Novak CM, Shi H. Enhanced sympathetic activity in mice with brown adipose tissue transplantation (transBATation). Physiol Behav. (2014) 125:21–9. doi: 10.1016/j.physbeh.2013.11.008
49. Liu X, Wang S, You Y, Meng M, Zheng Z, Dong M, et al. Brown adipose tissue transplantation reverses obesity in Ob/Ob mice. Endocrinology (2015) 156:2461–9. doi: 10.1210/en.2014-1598
50. Varady KA, Hellerstein MK. Alternate-day fasting and chronic disease prevention: a review of human and animal trials. Am J Clin Nutr. (2007) 86:7–13. doi: 10.1093/ajcn/86.1.7
51. Maalouf M, Rho JM, Mattson MP. The neuroprotective properties of calorie restriction, the ketogenic diet, and ketone bodies. Brain Res Rev. (2009) 59:293–315. doi: 10.1016/j.brainresrev.2008.09.002
52. Vatner DE, Zhang J, Oydanich M, Guers J, Katsyuba E, Yan L, et al. Enhanced longevity and metabolism by brown adipose tissue with disruption of the regulator of G protein signaling 14. Aging Cell (2018) 14:e12751. doi: 10.1111/acel.12751
53. Wu J, Boström P, Sparks LM, Ye L, Choi JH, Giang AH, et al. Beige adipocytes are a distinct type of thermogenic fat cell in mouse and human. Cell (2012) 150:366–76. doi: 10.1016/j.cell.2012.05.016
54. Harms M, Seale P. Brown and beige fat: development, function and therapeutic potential. Nat Med. (2013) 19:1252–63. doi: 10.1038/nm.3361
55. Lee P, Werner CD, Kebebew E, Celi FS. Functional thermogenic beige adipogenesis is inducible in human neck fat. Int J Obes. (2014) 38:170–6. doi: 10.1038/ijo.2013.82
56. Barquissau V, Beuzelin D, Pisani DF, Beranger GE, Mairal A, Montagner A, et al. White-to-brite conversion in human adipocytes promotes metabolic reprogramming towards fatty acid anabolic and catabolic pathways. Mol Metab. (2016) 5:352–65. doi: 10.1016/j.molmet.2016.03.002
57. Cypess AM, Weiner LS, Roberts-Toler C, Franquet Elía E, Kessler SH, Kahn PA, et al. Activation of human brown adipose tissue by a β3-adrenergic receptor agonist. Cell Metabol. (2015) 21:33–8. doi: 10.1016/j.cmet.2014.12.009
58. Bargut TCL, Souza-Mello V, Aguila MB, and Mandarim-de-Lacerda CA. Browning of white adipose tissue: lessons from experimental models. Horm Mol Biol Clin Investig. (2017) 31:20160051. doi: 10.1515/hmbci-2016-0051
59. Shabalina IG, Petrovic N, de Jong JM, Kalinovich AV, Cannon B, Nedergaard J. UCP1 in brite/beige adipose tissue mitochondria is functionally thermogenic. Cell Rep. (2013) 5:1196–203. doi: 10.1016/j.celrep.2013.10.044
60. Cohen P, Levy JD, Zhang Y, Frontini A, Kolodin DP, Svensson KJ, et al. Ablation of PRDM16 and beige adipose causes metabolic dysfunction and a subcutaneous to visceral fat switch. Cell (2014) 156:304–16. doi: 10.1016/j.cell.2013.12.021
61. Harms MJ, Ishibashi J, Wang W, Lim HW, Goyama S, Sato T, et al. Prdm16 is required for the maintenance of brown adipocyte identity and function in adult mice. Cell Metab. (2014) 19:593–604. doi: 10.1016/j.cmet.2014.03.007
62. Nedergaard J, Cannon B. The changed metabolic world with human brown adipose tissue: therapeutic visions. Cell Metab. (2010) 11:268–72. doi: 10.1016/j.cmet.2010.03.007
63. Jimenez M, Barbatelli G, Allevi R, Cinti S, Seydoux J, Giacobino JP, et al. Beta 3-adrenoceptor knockout in C57BL/6J mice depresses the occurrence of brown adipocytes in white fat. Eur J Biochem. (2003) 270:699–705. doi: 10.1046/j.1432-1033.2003.03422.x
64. Barbatelli G, Murano I, Madsen L, Hao Q, Jimenez M, Kristiansen K, et al. The emergence of cold-induced brown adipocytes in mouse white fat depots is determined predominantly by white to brown adipocyte transdifferentiation. Am J Physiol Endocrinol Metab. (2010) 298:E1244–53. doi: 10.1152/ajpendo.00600.2009
65. Ma X, Xu L, Gavrilova O, Mueller E. Role of forkhead box protein A3 in age-associated metabolic decline. Proc Natl Acad Sci USA. (2014) 111:14289–94. doi: 10.1073/pnas.1407640111
66. Kajimura S, Spiegelman BM, Seale P. Brown and beige fat: physiological roles beyond heat generation. Cell Metab. (2015) 22:546–59. doi: 10.1016/j.cmet.2015.09.007
67. Goldberg AA, Bourque SD, Kyryakov P, Boukh-Viner T, Gregg C, Beach A, et al. A novel function of lipid droplets in regulating longevity. Biochem Soc Trans. (2009) 37:1050–5. doi: 10.1042/BST0371050
68. Conte M, Franceschi C, Sandri M, Salvioli S. Perilipin 2 and age-related metabolic diseases: a new perspective. Trends Endocrinol Metab. (2016) 27:893–903. doi: 10.1016/j.tem.2016.09.001
69. Goodpaster BH, Carlson CL, Visser M, Kelley DE, Scherzinger A, Harris TB, et al. Attenuation of skeletal muscle and strength in the elderly: the health ABC study. J Appl Physiol. (2001) 90:2157–65. doi: 10.1152/jappl.2001.90.6.2157
70. Chang BH, Li L, Paul A, Taniguchi S, Nannegari V, Heird WC, et al. Protection against fatty liver but normal adipogenesis in mice lacking adipose differentiation-related protein. Mol Cell Biol. (2006) 26:1063–76. doi: 10.1128/MCB.26.3.1063-1076.2006
71. Cartwright MJ, Tchkonia T, Kirkland JL. Aging in adipocytes: potential impact of inherent, depot-specific mechanisms. Exp Gerontol. (2007) 42:463–71. doi: 10.1016/j.exger.2007.03.003
72. Yim JE, Heshka S, Albu J, Heymsfield S, Kuznia P, Harris T, et al. Intermuscular adipose tissue rivals visceral adipose tissue in independent associations with cardiovascular risk. Int J Obes. (2007) 31:1400–5. doi: 10.1038/sj.ijo.0803621
73. Delmonico MJ, Harris TB, Visser M, Park SW, Conroy MB, Velasquez-Mieyer P, et al. Health, aging, and body. Longitudinal study of muscle strength, quality, and adipose tissue infiltration. Am J Clin Nutr. (2009) 90:1579–85. doi: 10.3945/ajcn.2009.28047
74. Koehler EM, Schouten JN, Hansen BE, van Rooij FJ, Hofman A, Stricker BH, et al. Prevalence and risk factors of non-alcoholic fatty liver disease in the elderly: results from the Rotterdam study. J Hepatol. (2012) 57:1305–11. doi: 10.1016/j.jhep.2012.07.028
75. Conte M, Vasuri F, Trisolino G, Bellavista E, Santoro A, Degiovanni A, et al. Increased Plin2 expression in human skeletal muscle is associated with sarcopenia and muscle weakness. PLoS ONE (2013) 8:e73709. doi: 10.1371/journal.pone.0073709
76. Bustos V, Partridge L. Good Ol’ Fat: links between lipid signaling and longevity. Trends Biochem Sci. (2017) 42:812–23. doi: 10.1016/j.tibs.2017.07.001
77. Broué F, Liere P, Kenyon C, Baulieu EE. A steroid hormone that extends the lifespan of Caenorhabditis elegans. Aging Cell. (2007) 6:87–94. doi: 10.1111/j.1474-9726.2006.00268.x
78. Russell SJ, Kahn CR. Endocrine regulation of ageing. Nat Rev Mol Cell Biol. (2007) 8:681–91. doi: 10.1038/nrm2234
79. Aguilaniu H, Fabrizio P, Witting M. The role of dafachronic acid signaling in development and longevity in caenorhabditis elegans: digging deeper using cutting-edge analytical chemistry. Front Endocrinol. (2016) 7:12. doi: 10.3389/fendo.2016.00012
80. Li X, Handee W, Kuo MH. The slim, the fat, and the obese: guess who lives the longest? Curr Genet. (2017) 63:43–9. doi: 10.1007/s00294-016-0617-z
81. Medkour Y, Dakik P, McAuley M, Mohammad K, Mitrofanova D, Titorenko VI. Mechanisms underlying the essential role of mitochondrial membrane lipids in yeast chronological aging oxid. Med Cell Longev. (2017) 2017:2916985. doi: 10.1155/2017/2916985
82. Handee W, Li X, Hall KW, Deng X, Li P, Benning C, et al. An energy-independent pro-longevity function of triacylglycerol in yeast. PLoS Genet. (2016) 12:e1005878. doi: 10.1371/journal.pgen.1005878
83. Henne WM, Reese ML, Goodman JM. The assembly of lipid droplets and their roles in challenged cells. EMBO J. (2018) 37:e98947. doi: 10.15252/embj.201898947
84. Dichlberger A, Schlager S, Maaninka K, Schneider WJ, Kovanen PT. Adipose triglyceride lipase regulates eicosanoid production in activated human mast cells. J Lipid Res. (2014) 55:2471–8. doi: 10.1194/jlr.M048553
85. Kershaw EE, Flier JS. Adipose tissue as an endocrine organ. J Clin Endocrinol Metab. (2004) 89:2548–56. doi: 10.1210/jc.2004-0395
86. Riera CE, Tsaousidou E, Halloran J, Follett P, Hahn O, Pereira MMA, et al. The sense of smell impacts metabolic health and obesity. Cell Metab. (2017) 26:198–211.e5. doi: 10.1016/j.cmet.2017.06.015
87. Wu C, Hwang SH, Jia Y, Choi J, Kim YJ, Choi D, et al. Olfactory receptor 544 reduces adiposity by steering fuel preference toward fats. J Clan Invest. (2017) 127:4118–23. doi: 10.1172/JCI89344
88. Palouzier-Paulignan B, Lacroix MC, Aimé P, Baly C, Caillol M, Congar P, et al. Olfaction under metabolic influences. Chem Sens. (2012) 37:769–97. doi: 10.1093/chemse/bjs059
89. Shirakawa K, Yan X, Shinmura K, Endo J, Kataoka M, Katsumata Y, et al. Obesity accelerates T cell senescence in murine visceral adipose tissue. J Clin Invest. (2016) 126:4626–39. doi: 10.1172/JCI88606
90. Liuzzi A, Savia G, Tagliaferri M, Lucantoni R, Berselli ME, Petroni ML, et al. Serum leptin concentration in moderate and severe obesity: relationship with clinical, anthropometric and metabolic factors. Int J Obes Relat Metab Disord. (1999) 23:1066–73. doi: 10.1038/sj.ijo.0801036
91. Coelho M, Oliveira T, Fernandes R. Biochemistry of adipose tissue: an endocrine organ. Arch Med Sci. (2013) 9:191–200. doi: 10.5114/aoms.2013.33181
92. Shimomura I, Hammer RE, Ikemoto S, Brown MS, Goldstein JL. Leptin reverses insulin resistance and diabetes mellitus in mice with congenital lipodystrophy. Nature (1999) 401:73–6. doi: 10.1038/43448
93. Ouchi N, Parker JL, Lugus JJ, Walsh K. Adipokines in inflammation and metabolic disease. Nat Rev Immunol. (2011) 11:85–97. doi: 10.1038/nri2921
94. Oral EA, Simha V, Ruiz E, Andewelt A, Premkumar A, Snell P, et al. Leptin-replacement therapy for lipodystrophy. N Engl J Med. (2002) 346:570–8. doi: 10.1056/NEJMoa012437
95. Chong AY, Lupsa BC, Cochran EK, Gorden P. Efficacy of leptin therapy in the different forms of human lipodystrophy. Diabetologia (2010) 53:27–35. doi: 10.1007/s00125-009-1502-9
96. Galic S, Oakhill JS, Steinberg GR. Adipose tissue as an endocrine organ. Mol Cell Endocrinol. (2010) 316:129–39. doi: 10.1016/j.mce.2009.08.018
97. Schraw T, Wang ZV, Halberg N, Hawkins M, Scherer PE. Plasma adiponectin complexes have distinct biochemical characteristics. Endocrinology (2008) 149:2270–82. doi: 10.1210/en.2007-1561
98. Li S, Shin HJ, Ding EL, van Dam RM. Adiponectin levels and risk of type 2 diabetes: a systematic review and meta-analysis. JAMA (2009) 302:179–88. doi: 10.1001/jama.2009.976
99. Blüher M, Kahn BB, Kahn CR. Extended longevity in mice lacking the insulin receptor in adipose tissue. Science (2003) 299:572–4. doi: 10.1126/science.1078223
100. Berryman DE, List EO, Coschigano KT, Behar K, Kim JK, Kopchick JJ. Comparing adiposity profiles in three mouse models with altered GH signaling. Growth Horm IGF Res. (2004) 14:309–18. doi: 10.1016/j.ghir.2004.02.005
101. Wang Z, Al-Regaiey KA, Masternak MM, Bartke A. Adipocytokines and lipid levels in Ames dwarf and calorie-restricted mice. J Gerontol A Biol Sci Med Sci. (2006) 61:323–31. doi: 10.1093/gerona/61.4.323
102. Brooks NL, Trent CM, Raetzsch CF, Flurkey K, Boysen G, Perfetti MT, et al. Low utilization of circulating glucose after food withdrawal in Snell dwarf mice. J Biol Chem. (2007) 282:35069–77. doi: 10.1074/jbc.M700484200
103. Arai Y, Takayama M, Abe Y, Hirose N. Adipokines and aging. J Atheroscler Thromb. (2011) 18:545–50. doi: 10.5551/jat.7039
104. Bartke A, List EO, Kopchick JJ. The somatotropic axis and aging: benefits of endocrine defects. Growth Horm IGF Res. (2016) 27:41–5. doi: 10.1016/j.ghir.2016.02.002
105. Miller KN, Burhans MS, Clark JP, Howell PR, Polewski MA, DeMuth TM, et al. Aging and caloric restriction impact adipose tissue, adiponectin, and circulating lipids. Aging Cell. (2017) 16:497–507. doi: 10.1111/acel.12575
106. Turer AT, Scherer PE. Adiponectin: mechanistic insights and clinical implications. Diabetologia (2012) 55:2319–26. doi: 10.1007/s00125-012-2598-x
107. Bucci L, Yani SL, Fabbri C, Bijlsma AY, Maier AB, Meskers CG, et al. Circulating levels of adipokines and IGF-1 are associated with skeletal muscle strength of young and old healthy subjects. Biogerontology (2013) 14:261–72. doi: 10.1007/s10522-013-9428-5
108. Bik W, Baranowska-Bik A, Wolinska-Witort E, Martynska L, Chmielowska M, Szybinska A, et al. The relationship between adiponectin levels and metabolic status in centenarian, early elderly, young and obese women. Neuro Endocrinol Lett. (2006) 27:493–500.
109. Atzmon G, Pollin TI, Crandall J, Tanner K, Schechter CB, Scherer PE, et al. Adiponectin levels and genotype: a potential regulator of life span in humans. J Gerontol A Biol Sci Med Sci. (2008) 63:447–53. doi: 10.1093/gerona/63.5.447
110. Itoh N. FGF21 as a hepatokine, adipokine, and myokine in metabolism and diseases. Front Endocrinol. (2014) 5:107. doi: 10.3389/fendo.2014.00107
111. Hui X, Feng T, Liu Q, Gao Y, Xu A. The FGF21-adiponectin axis in controlling energy and vascular homeostasis. J Mol Cell Biol. (2016) 8:110–9. doi: 10.1093/jmcb/mjw013
112. Straub L, Wolfrum C. FGF21, energy expenditure and weight loss - How much brown fat do you need? Mol Metab. (2015) 4:605–9. doi: 10.1016/j.molmet.2015.06.008
113. Zhang Y, Xie Y, Berglund ED, Coate KC, He TT, Katafuchi T, et al. The starvation hormone, fibroblast growth factor-21, extends lifespan in mice. Elife (2012) 1:e00065. doi: 10.7554/eLife.00065
114. Conte M, Ostan R, Fabbri C, Santoro A, Guidarelli G, Vitale G, et al. Human aging and longevity are characterized by high levels of mitokines. J Gerontol A Biol Sci Med Sci. (2018). doi: 10.1093/gerona/gly153. [Epub ahead of print].
115. Tezze C, Romanello V, Desbats MA, Fadini GP, Albiero M, Favaro G, et al. Loss of OPA1 in muscle impacts muscle mass, metabolic homeostasis, systemic inflammation, and epithelial senescence. Cell Metab. (2017) 25:1374–89.e6. doi: 10.1016/j.cmet.2017.04.021
116. Mochalski P, Diem E, Unterkofler K, Mündlein A, Drexel H, Mayhew CA, et al. In vitro profiling of volatile organic compounds released by Simpson-Golabi-Behmel syndrome adipocytes. J Chromatogr B Analyt Technol Biomed Life Sci. (2019) 1104:256–61. doi: 10.1016/j.jchromb.2018.11.028
117. Mazzatenta A, Pokorski M, Di Giulio C. Real time analysis of volatile organic compounds (VOCs) in centenarians. Respir Physiol Neurobiol. (2015) 209:47–51. doi: 10.1016/j.resp.2014.12.014
118. Mazzatenta A, Pokorski M, Sartucci F, Domenici L, Di Giulio C. Volatile organic compounds (VOCs) fingerprint of Alzheimer’s disease. Respir Physiol Neurobiol. (2015) 209:81–4. doi: 10.1016/j.resp.2014.10.001
119. Kramer C, Mochalski P, Unterkofler K, Agapiou A, Ruzsanyi V, Liedl KR. Prediction of blood:air and fat:air partition coefficients of volatile organic compounds for the interpretation of data in breath gas analysis. J Breath Res. (2016) 10:017103. doi: 10.1088/1752-7155/10/1/017103
120. Shirasu M, Touhara K. The scent of disease: volatile organic compounds of the human body related to disease and disorder. J Biochem. (2011) 150:257–66. doi: 10.1093/jb/mvr090
121. Heindel JJ, Blumberg B, Cave M, Machtinger R, Mantovani A, Mendez MA, et al. Metabolism disrupting chemicals and metabolic disorders. Reprod Toxicol. (2017) 68:3–33. doi: 10.1016/j.reprotox.2016.10.001
122. Richardson RB, Allan DS, Le Y. Greater organ involution in highly proliferative tissues associated with the early onset and acceleration of ageing in humans. Exp Gerontol. (2014) 55:80–91. doi: 10.1016/j.exger.2014.03.015
123. Palmer AK, Kirkland JL. Aging and adipose tissue: potential interventions for diabetes and regenerative medicine. Exp Gerontol. (2016) 86:97–105. doi: 10.1016/j.exger.2016.02.013
124. Chinn IK, Blackburn CC, Manley NR, Sempowski GD. Changes in primary lymphoid organs with aging. Semin Immunol. (2012) 24:309–20. doi: 10.1016/j.smim.2012.04.005
125. Taub DD, Longo DL. Insights into thymic aging and regeneration. Immunol Rev. (2005) 205:72–93. doi: 10.1111/j.0105-2896.2005.00275.x
126. Dooley J, Liston A. Molecular control over thymic involution: from cytokines and microRNA to aging and adipose tissue. Eur J Immunol. (2012) 42:1073–9. doi: 10.1002/eji.201142305
127. Dowling MR, Hodgkin PD. Why does the thymus involute? A selection-based hypothesis. Trends Immunol. (2009) 30:295–300. doi: 10.1016/j.it.2009.04.006
128. Dixit VD. Thymic fatness and approaches to enhance thymopoietic fitness in aging. Curr Opin Immunol. (2010) 22:521–8. doi: 10.1016/j.coi.2010.06.010
129. Torroba M, Zapata AG. Aging of the vertebrate immune system. Microsc Res Tech. (2003) 62:477–81. doi: 10.1002/jemt.10409
130. Im GI, Shin YW, Lee KB. Do adipose tissue-derived mesenchymal stem cells have the same osteogenic and chondrogenic potential as bone marrow-derived cells? Osteoarthr Cartil. (2005) 13:845–53. doi: 10.1016/j.joca.2005.05.005
131. Zhou S, Greenberger JS, Epperly MW, Goff JP, Adler C, Leboff MS, et al. Age-related intrinsic changes in human bone-marrow-derived mesenchymal stem cells and their differentiation to osteoblasts. Aging Cell. (2008) 7:335–43. doi: 10.1111/j.1474-9726.2008.00377.x
132. Muruganandan S, Sinal CJ. The impact of bone marrow adipocytes on osteoblast and osteoclast differentiation. IUBMB Life (2014) 66:147–55. doi: 10.1002/iub.1254
133. Phetfong J, Sanvoranart T, Nartprayut K, Nimsanor N, Seenprachawong K, Prachayasittikul V, et al. Osteoporosis: the current status of mesenchymal stem cell-based therapy. Cell Mol Biol Lett. (2016) 21:12. doi: 10.1186/s11658-016-0013-1
134. Sundararaghavan V, Mazur MM, Evans B, Liu J, Ebraheim NA. Diabetes and bone health: latest evidence and clinical implications. Ther Adv Musculoskelet Dis. (2017) 9:67–74. doi: 10.1177/1759720X16687480
135. Muruganandan S, Govindarajan R, Sinal CJ. Bone marrow adipose tissue and skeletal health. Curr Osteoporos Rep. (2018) 16:434–42. doi: 10.1007/s11914-018-0451-y
136. Marcus RL, Addison O, Kidde JP, Dibble LE, Lastayo PC. Skeletal muscle fat infiltration: impact of age, inactivity, and exercise. J Nutr Health Aging. (2010) 14:362–6. doi: 10.1007/s12603-010-0081-2
137. Marcus RL, Addison O, Dibble LE, Foreman KB, Morrell G, Lastayo P. Intramuscular adipose tissue, sarcopenia, and mobility function in older individuals. J Aging Res. (2012) 2012:629637. doi: 10.1155/2012/629637
138. Hamrick MW, McGee-Lawrence ME, Frechette DM. Fatty infiltration of skeletal muscle: mechanisms and comparisons with bone marrow adiposity. Front Endocrinol. (2016) 7:69. doi: 10.3389/fendo.2016.00069
139. Pagano AF, Brioche T, Arc-Chagnaud C, Demangel R, Chopard A, Py G. Short-term disuse promotes fatty acid infiltration into skeletal muscle. J Cachexia Sarcopenia Muscle. (2018) 9:335–47. doi: 10.1002/jcsm.12259
140. Sato T, Ito K, Tamada T, Sone T, Noda Y, Higaki A, et al. Age-related changes in normal adult pancreas: MR imaging evaluation. Eur J Radiol. (2012) 81:2093–8. doi: 10.1016/j.ejrad.2011.07.014
141. Löhr JM, Panic N, Vujasinovic M, Verbeke CS. The ageing pancreas: a systematic review of the evidence and analysis of the consequences. J Intern Med. (2018) 283:446–60. doi: 10.1111/joim.12745
142. Abd, El-Kader SM, El-Den Ashmawy EM. Non-alcoholic fatty liver disease: the diagnosis and management. World J Hepatol. (2015) 7:846–58. doi: 10.4254/wjh.v7.i6.846
143. Ogrodnik M, Miwa S, Tchkonia T, Tiniakos D, Wilson CL, Lahat A, et al. Cellular senescence drives age-dependent hepatic steatosis. Nat Commun. (2017) 8:15691. doi: 10.1038/ncomms15691
144. Kim IH, Kisseleva T, Brenner DA. Aging and liver disease. Curr Opin Gastroenterol. (2015) 31:184–91. doi: 10.1097/MOG.0000000000000176
145. Tajiri K, Shimizu Y. Liver physiology and liver diseases in the elderly. World J Gastroenterol. (2013) 19:8459–67. doi: 10.3748/wjg.v19.i46.8459
146. Monickaraj F, Aravind S, Nandhini P, Prabu P, Sathishkumar C, Mohan V, et al. Accelerated fat cell aging links oxidative stress and insulin resistance in adipocytes. J Biosci. (2013) 38:113–22.
147. Silaghi A, Piercecchi-Marti MD, Grino M, Leonetti G, Alessi MC, Clement K, et al. Epicardial adipose tissue extent: relationship with age, body fat distribution, and coronaropathy. Obesity (2008) 16:2424–30. doi: 10.1038/oby.2008.379
148. Iacobellis G. Local and systemic effects of the multifaceted epicardial adipose tissue depot. Nat Rev Endocrinol. (2015) 11:363–71. doi: 10.1038/nrendo.2015.58
149. Sacks HS, Fain JN, Bahouth SW, Ojha S, Frontini A, Budge H, et al. Adult epicardial fat exhibits beige features. J Clin Endocrinol Metab. (2013) 98:E1448–55. doi: 10.1210/jc.2013-1265
150. Aldiss P, Davies G, Woods R, Budge H, Sacks HS, Symonds ME. ‘Browning’ the cardiac and peri-vascular adipose tissues to modulate cardiovascular risk. Int J Cardiol. (2017) 228:265–74. doi: 10.1016/j.ijcard.2016.11.074
151. Jo J, Gavrilova O, Pack S, Jou W, Mullen S, Sumner AE, et al. Hypertrophy and/or hyperplasia: dynamics of adipose tissue growth. PLoS Comput Biol. (2009) 5:e1000324. doi: 10.1371/journal.pcbi.1000324
152. Choe SS, Huh JY, Hwang IJ, Kim JI, Kim JB. Adipose tissue remodeling: its role in energy metabolism and metabolic disorders. Front Endocrinol. (2016) 7:30. doi: 10.3389/fendo.2016.00030
153. Baggio G, Donazzan S, Monti D, Mari D, Martini S, Gabelli C, et al. Lipoprotein(a) and lipoprotein profile in healthy centenarians: a reappraisal of vascular risk factors. FASEB J. (1998) 12:433–7. doi: 10.1096/fasebj.12.6.433
Keywords: adipose tissue, aging, lipid deposition, organ involution, inflammaging
Citation: Conte M, Martucci M, Sandri M, Franceschi C and Salvioli S (2019) The Dual Role of the Pervasive “Fattish” Tissue Remodeling With Age. Front. Endocrinol. 10:114. doi: 10.3389/fendo.2019.00114
Received: 22 November 2018; Accepted: 07 February 2019;
Published: 26 February 2019.
Edited by:
Antonello Lorenzini, University of Bologna, ItalyReviewed by:
Nina Mohorko, University of Primorska, SloveniaAndrea Mazzatenta, Università degli Studi G. d’Annunzio Chieti e Pescara, Italy
Copyright © 2019 Conte, Martucci, Sandri, Franceschi and Salvioli. This is an open-access article distributed under the terms of the Creative Commons Attribution License (CC BY). The use, distribution or reproduction in other forums is permitted, provided the original author(s) and the copyright owner(s) are credited and that the original publication in this journal is cited, in accordance with accepted academic practice. No use, distribution or reproduction is permitted which does not comply with these terms.
*Correspondence: Maria Conte, bS5jb250ZUB1bmliby5pdA==