- 1State Key Laboratory of Freshwater Ecology and Biotechnology, Institute of Hydrobiology, Chinese Academy of Sciences, Wuhan, China
- 2College of Advanced Agricultural Sciences, University of Chinese Academy of Sciences, Beijing, China
Prolactin (PRL) has been considered a key regulator of ion uptake in zebrafish. The genes slc12a10.2 and slc12a3, which are Na+ and chloride Cl− co-transporters, have been reported to be regulated by PRL in freshwater fish. The integrative network of PRL signaling dissected from the knockout of tissue-specific downstream PRL ion transporters remains poor. In the present study, zebrafish models with increased endogenous levels of PRL were generated through the knockout of slc12a10.2 or slc12a3, and the developmental consequences were analyzed. The increased levels of pituitary PRL were observed in both slc12a10.2- and slc12a3-deficient fish. Unlike the slc12a3-deficient fish, which could survive to adulthood, the slc12a10.2-deficient fish began to die at 9 days post-fertilization (dpf) and did not survive beyond 17 dpf. This survival defect is a result of defective Cl− uptake in this mutant, indicating that Slc12a10.2 plays an essential role in Cl− uptake. Intriguingly, compared to the levels in control fish, no significant differences in the levels of Na+ in the body were observed in slc12a10.2- or slc12a3-deficient zebrafish. The upregulations of the PRL downstream transporters, slc9a3.2, slc12a10.2, and atp1a1a.5 were observed in slc12a3-deficient fish in both the gills/skin and the pronephric duct. However, this type of response was not observed in the pronephric duct of slc12a10.2-deficient fish, except under Na+-deprived conditions. Our results show that PRL is susceptible to deficiencies in downstream ion transporters. Moreover, both the gills/skin and pronephric duct show differential expression of downstream PRL targets in response to increased levels of pituitary PRL caused by the depletion of tissue-specific ion transporters.
Introduction
The endocrine system plays a central role in the homeostatic regulation of the salt and water balance in vertebrates (1). Several key neuroendocrine factors were identified as regulators of epithelial ion movement, including cortisol, catecholamines (adrenaline and noradrenaline), parathyroid hormone, and prolactin (PRL) (2). In mammals, PRL regulates salt and water balance by increasing intestinal water and salt absorption and reducing renal Na+ and K+ excretion through modulating Na+-K+ ATPase activity (3, 4). In fish, PRL has long been considered a key hormone for freshwater adaptation by preventing both the loss of ions and the uptake of water (5–10). The role of PRL in fish osmoregulation was first demonstrated in the 1950s by studies on killifish (Fundulus heteroclitus), as F. heteroclitus were unable to survive in freshwater following hypophysectomy; however, PRL treatment allowed hypophysectomized killifish to survive (8). This spawned an intensive investigation into the osmoregulatory role of PRL in a series of euryhaline species. PRL promotes the conservation of ions and secretion of water by acting on the gills/skin, pronephric duct, gut, and urinary bladder (7). Correspondingly, the highest prl receptor expression levels were observed in the primary osmoregulatory organs (kidney, gills, and intestine) (11–15). In zebrafish, PRL receptors (PRLRs), PRLRa and PRLRb, have been observed in the gills and pronephric duct, respectively. It has been demonstrated that PRL binds to PRLRs that are present in osmoregulatory organs (16–18). The multiple forms of PRL receptor family have been retained following genome duplication events (19), and have distinct expression patterns and capacities to activate intracellular signaling pathways (20–22). These results imply that PRL signaling is complicated and may be differentially regulated in disparate osmoregulatory organs.
The genetic understanding of the mechanisms through which PRL regulates ion transporters has only recently been investigated. Breves et al. demonstrated that slc12a10.2 represents a conserved transcriptional target of PRL in fishes, as pituitary-derived PRL regulates slc12a10.2 expression in ionocytes of the Mozambique tilapia (23). Our previous study also demonstrated that PRL is a positive regulator of ion absorption and reabsorption by maintaining the transcription of several transporters in the gill/skin and pronephric duct in zebrafish (17). The expression levels of certain key Na+/Cl− co-transporters in the gills (slc12a10.2), as well as, Na+/K+-ATPase subunits (atp1a1a.5), Na+/H+ exchangers (slc9a3.2) and Na+/Cl− transporters (slc12a3) in the pronephros in prl-deficient larvae were downregulated at 5 days post-fertilization (dpf), which caused defects in the uptake of Na+/K+/Cl− in prl-deficient zebrafish (17). Collectively, the previous studies have demonstrated that the transcriptional targets of PRL include slc12a10.2 in gills, and slc12a3, slc9a3.2, and atp1a1a.5 in pronephric duct. Although great progress has been made in previous studies supporting the hypothesis that PRL regulates the transcription of different ionocyte transporters, the regulatory network of PRL signaling has not been extensively investigated because the manipulation of ion transporters downstream of PRL remains poor.
Zebrafish has been recognized as a model organism for studying osmoregulation (24), as both Transcription Activator-Like Effector Nuclease (TALEN) and Clustered Regularly Interspaced Short Palindromic Repeats/CRISPR-associated system (CRISPR/Cas9) have been successfully adopted in zebrafish to knockout functional genes (25–27). Apart from the effective knockout strategy, zebrafish also possess several advantages as an experimental organism in osmoregulation research, including the applicability of molecular tools, ease of in vivo cellular observation, and rapid embryonic development (28). In particular, zebrafish possess specialized ionocytes, principally located in the gills of adults and in the skin of embryos and larvae, which facilitate active absorption of electrolytes from the medium (29). In freshwater fishes, the gills and pronephric duct are the most important organs responsible for ionoregulation. The gill and pronephric duct are involved in ion regulation owing to the presence of numerous ion channels, pumps, or exchangers (30). In a hypotonic freshwater environment, teleosts have to actively absorb ions from the water mainly via the gills and excrete large amounts of urine via the pronephric ducts in order to maintain the homeostasis of their body fluids (31, 32). In the zebrafish pronephric duct, ions are reabsorbed through active transcellular and passive paracellular transport processes. To eliminate the excess water gained from the environment, freshwater fish reabsorb approximately 95% of the NaCl that enters the glomerular filtrate and then produce diluted, hypoosmotic urine (30, 33, 34). Upon the coordinated corporation of the gills and pronephric duct, zebrafish have a high capacity for Na+/Cl− uptake, even living in hypoosmotic waters (35).
In zebrafish, four members of the slc12a family were identified: slc12a3, slc12a10.1, slc12a10.2, and slc12a10.3. Among the four members, slc12a3 is the ortholog to the mammalian slc12a3 (also known as ncc), whereas slc12a10.2 was recognized as ncc2b or ncc-like (36). During the larval stage, transcriptional abundance of slc12a10.2 was observed in the gill region and in the skin of the yolk sac, in addition to the observed transcriptional abundance of slc12a3 in the pronephric duct (17). In the present study, knockout of slc12a10.2 and slc12a3 was performed. Our results differ from previous findings obtained from the manipulation of the pituitary prl (5, 17). The developmental consequences of slc12a10.2- or slc12a3-deficent fish were analyzed. We found that increased levels of pituitary PRL was observed in both the slc12a10.2- and slc12a3-deficient fish, suggesting that the expression of PRL is susceptible to deficiencies in downstream ion transporters. We demonstrated that depletion of slc12a10.2 results in lethality, which is caused by defective Cl− uptake, whereas the slc12a3-deficient fish survived healthily into adulthood without impairments in the levels of Na+ and Cl−. We also observed an upregulation of the PRL target transporters, slc9a3.2, slc12a10.2, and atp1a1a.5, in the pronephric duct in slc12a3-deficient fish; however, upregulation of these genes were not observed in slc12a10.2-deficient fish, unless under Na+-deprived conditions. The integrative comparisons of the developmental consequences of slc12a10.2- and slc12a3-deficient fish demonstrate that the gills/skin and pronephric duct show differential responses of PRL transcriptional targets in response to the increased levels of PRL caused by the depletion of tissue-specific ion transporters.
Materials and Methods
Zebrafish Maintenance
All zebrafish (AB strain) were maintained in a circulated water system with a 14-h light and 10-h dark cycle at 28.5°C and fed newly hatched brine shrimp (Artemia salina). The developmental stages of zebrafish embryos were characterized as described previously (37). All fish experiments were conducted in accordance with the Guiding Principles for the Care and Use of Laboratory Animals and were approved by the Institute of Hydrobiology, Chinese Academy of Sciences (Approval ID: IHB2013724).
Establishment of slc12a10.2 and slc12a3 Knockout Lines
The slc12a10.2 and slc12a3 knockout lines of zebrafish were generated via CRISPR/Cas9 technology. Guide RNA (gRNA), generated against the slc12a10.2 or slc12a3 target sequence in the first exon, was designed using an online software tool (http://zifit.partners.org/ZiFiT) (38). gRNA was synthesized from the PCR products of the pMD19T-gRNA plasmid using primers containing the target sequence and nominated sequence for the plasmid. PCR products were directly used for gRNA synthesis using the TranscriptAid T7 High Yield Transcription Kit following the manufacturer's constructions (K0441, Thermo Fisher Scientific, Waltham, MA, USA). Cas9 mRNA was synthesized from the pXT7-Cas9 plasmid using the T7 mMESSAGE mMACHINE mRNA Transcription Synthesis Kit (AM1344, Thermo Fisher Scientific) and purified using RNeasy Mini Kit (#74106, Qiagen, Hilden, Germany). The Cas9 mRNA and gRNA for slc12a10.2 or slc12a3 were combined for injection immediately before use (27). For mutation examination, the PCR products from the genomes of fish were run in PAGE gel after denaturation and annealing. The PAGE gel was stained with ethidium bromide for 15 min before examination. The homoduplexes and heteroduplexes were separated with heteroduplexes being slower in mobility (39). The primers for mutation examination are listed in Table 1. The heterozygous males and females in the F1 population were crossed to generate an F2 population that contained slc12a10.2 or slc12a3 homozygotes.
Whole Mount in situ Hybridization (WISH)
We performed WISH as described previously (40). cDNA of the following genes were used as anti-sense digoxigenin-labeled probes in our study: prl, slc12a10.2, slc12a3, slc9a3.2, and atp1a1a.5.
Sodium Influx Analysis
The sodium uptake by gill ionocytes was visualized using sodium green staining (S6901, Thermo Fisher Scientific) as previously described (17). Live larvae at 6 dpf were incubated in 10 μM sodium green in egg water containing 0.1% dimethyl sulfoxide (DMSO) for 1 h at 37°C. The larvae were anesthetized for photography (NOL-LSM 710 microscope, Carl Zeiss, Oberkochen, Germany).
Whole Body Ion Concentration Measurement
Ion measurement of the whole body was performed as previously described (17). Briefly, zebrafish were rinsed with double-deionized water and dried out at 56°C for 6 h. Then 100 μL nitric acid (71%) was added to the samples for digestion at 65°C overnight. For Na+, K+, Ca2+, and Mg2+ measurements, the digested solution was diluted with double-deionized water to a volume of 5 mL and measured using inductively coupled plasma-optical emission spectroscopy (ICP-OES, PerkinElmer-OPTIMA 8000DV, Waltham, MA, USA). For Cl− measurements, zebrafish were sonicated in 0.5 mL double-deionized water for 10 min until homogeneous, and the supernatant was collected after centrifuging at 14,000 rpm for 10 min. The Cl− concentrations of the supernatants were measured using a Chloride Colorimetric Assay Kit (K530-100, Biovision, San Francisco, CA, USA) according to the manufacturer's instructions.
The Rescue Experiment With Brackish Water and Artificial Water With Different Compounds
In the rescue experiment, 50 larvae of the F2 population were maintained in system water with sea salt (5 g sea salt added to 1 L system water), Mix 1 (3.336 g NaCl, 0.113 g KCl, 0.311 g CaCl2, and 1.378 g MgCl2 to 1 L system water), Mix 2 (4.054 g Na2SO4, 0.132 g K2SO4, 0.364 g CaSO4, and 0.816 g MgSO4 to 1 L system water), or Mix 3 (0.113 g KCl, 4.500 g CaCl2, and 1.378 g MgCl2 to 1 L system water). The survival ratio was recorded for 20 days.
The Hypo-Na+ Mediated Na+ Uptake Stress With Different Concentrations of Na+ in the Artificial Rearing Medium
Twenty control or slc12a10.2-deficient larvae from the F2 population were maintained in artificial water with ultrahigh Na+ concentration (3.336 g NaCl, 0.113 g KCl, 1.378 g MgCl2, and 0.311 g CaCl2 to 1 L purified water), high Na+ concentration (2.502 g NaCl, 0.113 g KCl, 1.378 g MgCl2, and 1.360 g CaCl2 to 1 L purified water), medium Na+ concentration (1.668 g NaCl, 0.113 g KCl, 1.378 g MgCl2, and 2.409 g CaCl2 to 1 L purified water), low Na+ concentration (0.834 g NaCl, 0.113 g KCl, 1.378 g MgCl2, and 3.458 g CaCl2 to 1 L purified water), or 0-Na+ concentration (0.113 g KCl, 1.378 g MgCl2, and 4.507 g CaCl2 to 1 L purified water). At 14 dpf, the control or slc12a10.2-deficient larvae reared at the above medium were harvested for RNA extraction and quantitative real-time PCR (qPCR) (see below).
RNA Extraction and qPCR
Total RNA was extracted using TRIzol reagent, and cDNA was synthesized using an oligo (dT) 18 primer and RevertAid First-strand cDNA Synthesis Kit (K1622, Thermo Scientific, Waltham, MA, USA) according to the manufacturer's instructions. qPCR primers for atp1a1a.5, slc12a3, slc9a3.2, slc12a10.2, prl, prlra, prlrb, and β-actin were designed using the National Center for Biotechnology Information (NCBI) primer BLAST service, and are listed in Table 1. All mRNA levels were calculated as fold expression relative to the housekeeping gene, β-actin. Each sample was run in triplicate, and the results were expressed according to the method described previously (41). The primers for qPCR were validated by DNA sequencing and agarose gel electrophoresis of PCR products.
Statistical Analysis
Detailed information regarding the number of zebrafish used per experiment is provided in each individual experiment and the corresponding figure. All analyses were performed with the GraphPad Prism 5.0 software program, and the differences were assessed using the Student's t-test. The results were expressed as the mean ± SD. For all statistical comparisons, a P < 0.05 was used to indicate a statistically significant difference.
Results
Cas9-Mediated Knockout of slc12a10.2
WISH staining using a probe against slc12a10.2 demonstrated that slc12a10.2 is expressed in the gills/skin at 4 dpf (Figure 1A). A mutant line was obtained with an 11 base pair (bp) deletion in the first exon of slc12a10.2 (Figure 1B). To evaluate the effects of slc12a10.2-deficiency on the Na+ and Cl− concentrations in the entire zebrafish body, we measured the Na+ and Cl− concentrations in slc12a10.2-deficient fish at 6 dpf. We observed unaffected Na+ concentration in the slc12a10.2-deficient fish (Figures 1C,D). This result demonstrates that the Na+ absorption is normally maintained in slc12a10.2-deficient fish. However, we did observe a significantly decreased Cl− concentration in the slc12a10.2-deficient fish, suggesting an essential role of Slc12a10.2 in maintaining Cl− levels within the body (Figure 1E). Sodium staining demonstrates that the slc12a10.2-deficient larvae at 6 dpf have normal Na+ uptake in gills (Figures 1F,G).
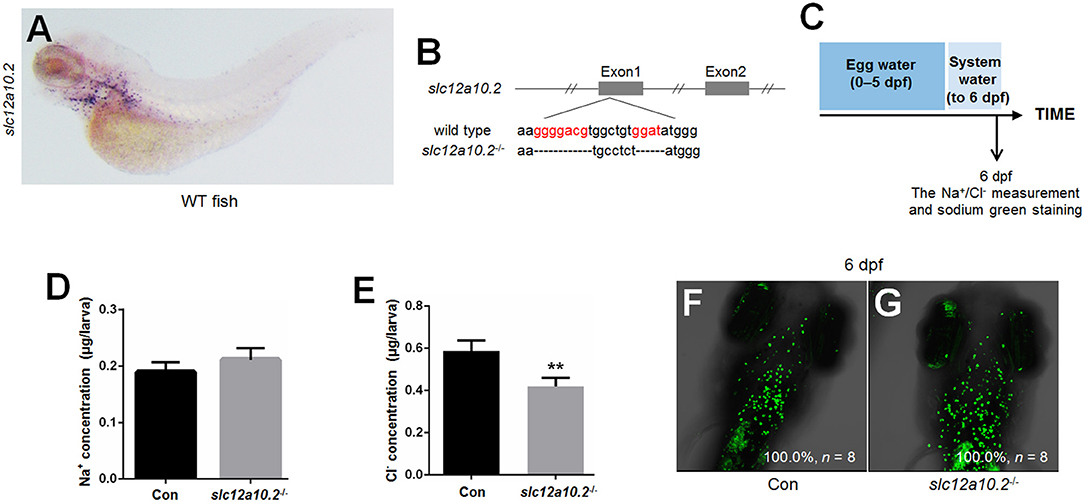
Figure 1. Generation of slc12a10.2-deficient zebrafish. (A) WISH analysis using the slc12a10.2 probe in wild-type larvae at 4 dpf. (B) Targeted depletion of the slc12a10.2 gene. The CRISPR/Cas9 target site is located at exon 1. A genotype with an 11-bp deletion was used to establish the slc12a10.2 knockout line (highlighted in red). (C) Schematic diagram of the rearing timeline. Larvae were reared in egg water from 0 to 5 dpf and in system water after 5 dpf. The fish at 6 dpf were harvested for Na+ and Cl− measurements. (D,E) Na+ and Cl− concentrations were measured in control and slc12a10.2-deficient larvae at 6 dpf. **P < 0.01. (F,G) Sodium green staining in the gills of control and slc12a10.2-deficient larvae at 6 dpf (ventral views).
Depletion of slc12a10.2 Resulted in Lethality in Freshwater
When slc12a10.2-deficient larvae were reared under the standard conditions (egg water for the first 5 days and system water for the following 15 days), lethality occurred from 9 to 17 dpf (Figures 2A,B). The lethality of the slc12a10.2-deficient larvae occurred later than the prl-deficient larvae, as the lethality of the prl-deficient larvae occurred from 6 to 11 dpf (17) (Figure 2B). These results suggest that, compared to prl-deficient larvae, a more suitable ion concentration in the slc12a10.2-deficient zebrafish may be the reason for the delayed lethality in these fish. Based on our morphological observations of the early developmental processes of the fish at 6 and 8 dpf, no obvious defects could be found in the slc12a10.2-deficient fish (Figures 2C–F). However, significant body curvature and shrink defects were observed in the majority of the slc12a10.2-deficient fish at 10 dpf, and the defective conditions of these larvae continuously worsened over time (Figures 2G,H).
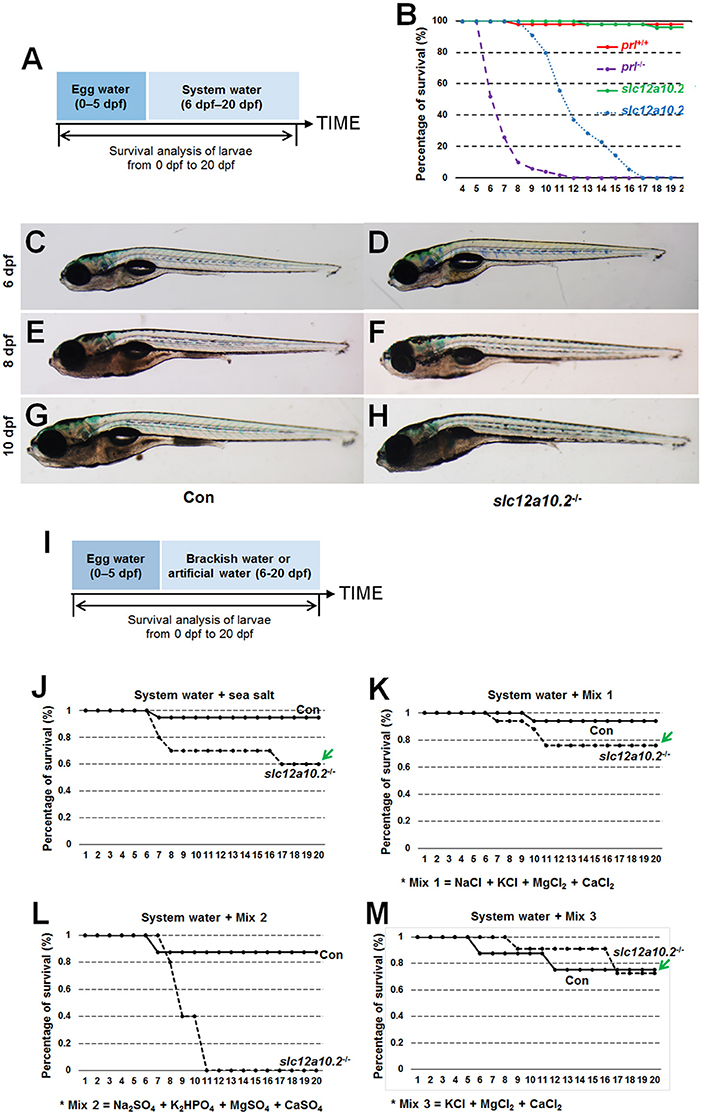
Figure 2. The lethality of the slc12a10.2-deficient zebrafish is rescued by exogenous Cl− supplement. (A) Schematic diagram of the rearing timeline. Larvae were reared in egg water from 0 to 5 dpf, and in system water after 5 dpf. The survival ratio of fish reared under this condition was analyzed until 20 dpf. (B) Comparison of the survival ratios of prl+/+, prl−/−, slc12a10.2+/+, and slc12a10.2−/− larvae (n = 25 for each genotype). (C–H) The general morphological observations of control larvae and slc12a10.2-deficient larvae at 6, 8, and 10 dpf. (C,E,G) Control larvae. (D,F,H) slc12a10.2-deficient larvae. (I) Schematic diagram of the rearing timeline. Larvae were reared in egg water from 0 to 5 dpf and in brackish or artificial water after 5 dpf. The survival ratios of fish reared under different conditions were analyzed until 20 dpf. (J–M) The survival ratios of control and slc12a10.2-deficient larvae in system water + sea salt, system water + Mix 1, system water + Mix 2, and system water + Mix 3.
Our further analysis showed that the slc12a10.2-deficient zebrafish were able to survive healthily in sea salt-supplemented system water (Figures 2I,J). By providing additional Na+, K+, Mg2+, Ca2+, and Cl− in the artificial water, the slc12a10.2-deficient zebrafish could survive healthily (Figure 2K). The rescue of lethality was observed when Na+ was depleted in the artificial water; however, the rescue failed when Cl− in the artificial water was replaced with (Figures 2L,M). These results demonstrate that the slc12a10.2-deficient fish died owing to the defective uptake of Cl−, not Na+, clearly indicating an essential role of Slc12a10.2 in Cl− uptake.
The Depletion of slc12a10.2 Upregulates PRL Rather Than PRL Transcriptional Targets in the Pronephric Duct
In order to better elucidate the changes in the PRL network in osmoregulation when Slc12a10.2 is absent, the expressions of downstream PRL targets were examined in control and slc12a10.2-deficient larvae at 4 dpf (Figure 3A). In slc12a10.2-deficient fish, the upregulation of pituitary prl and gills/skin atp1a1a.5 was observed (Figures 3B–E). Intriguingly, the expressions of the PRL transcriptional targets, atp1a1a.5, slc9a3.2, and slc12a3, were unaffected in the pronephric duct in slc12a10.2-deficient fish (Figures 3D–I). These results demonstrate that atp1a1a.5 in the gills most likely compensates for Na+ uptake in slc12a10.2-deficient fish rather than the other Na+ transporters in the pronephric duct. The upregulated gene expression of atp1a1a.5 was also confirmed with qPCR (Figure 3J). It is also worth noting that prl and prlra were upregulated in slc12a10.2-deficient zebrafish compared to control fish (Figure 3J). In light of the previous studies reporting that prlra is the downstream target of PRL (5, 23, 42), this data suggests that PRL signaling is amplified in the slc12a10.2-deficient zebrafish.
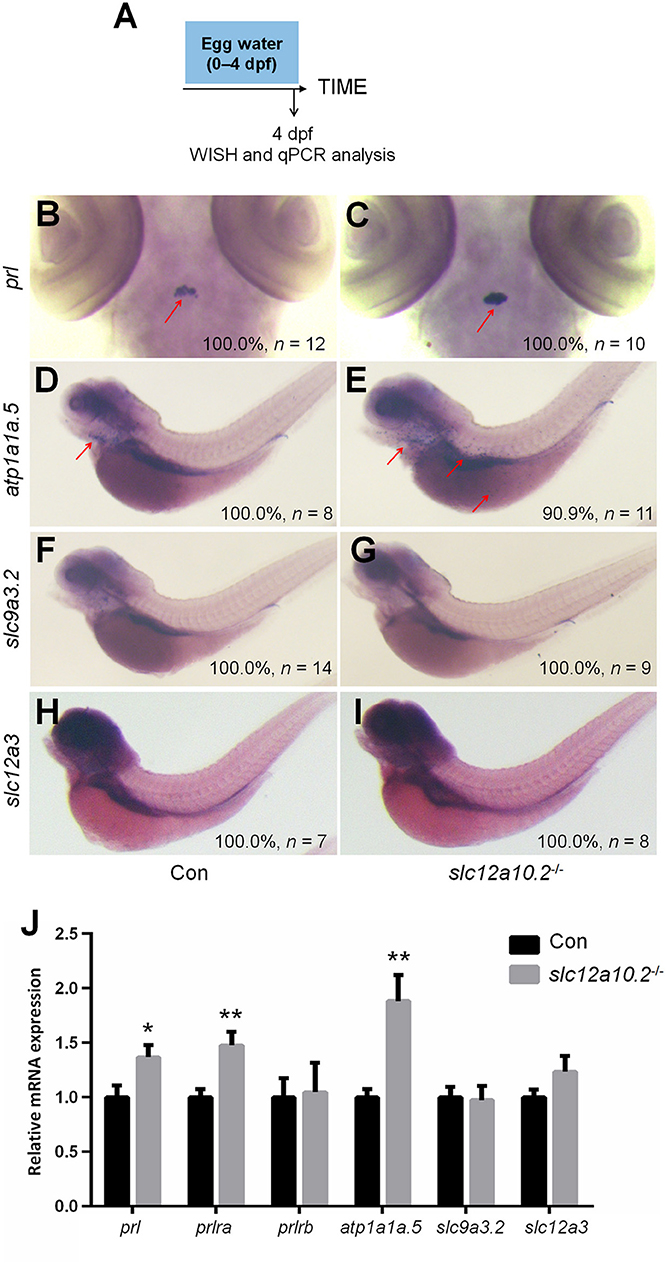
Figure 3. Expressions of prl and PRL targets in control and slc12a10.2-deficient larvae at 4 dpf. (A) Schematic diagram of the rearing timeline. Larvae were harvested at 4 dpf for WISH and qPCR analysis. (B,C) WISH analysis using the probe of prl in control and slc12a10.2-deficient larvae at 4 dpf. (D,E) WISH analysis using the probe of atp1a1a.5 in control and slc12a10.2-deficient larvae at 4 dpf. (F,G) WISH analysis using the probe of slc9a3.2 in control and slc12a10.2-deficient larvae at 4 dpf. (H,I) WISH analysis using the probe of slc12a3 in control and slc12a10.2-deficient larvae at 4 dpf. Red arrows indicate the expressions of the nominated genes. (J) Expression levels of prl, prlra, prlrb, atp1a1a.5, slc9a3.2, and slc12a3 in control larvae and slc12a10.2-deficient larvae at 4 dpf were examined with qPCR. *P < 0.05. **P < 0.01.
We subsequently performed qPCR to examine the mRNA expressions of Na+ uptake-related transporters, i.e., atp1a1a.5, slc12a3, and slc9a3.2, in the slc12a10.2-deficient fish when reared in artificial medium with different Na+ concentrations (ultrahigh-, high-, medium-, low-, and 0-Na+ concentration) from 6 to 14 dpf. In control fish reared in the artificial medium with ultrahigh- or high-Na+ content, the expressions of PRL transcriptional targets, atp1a1a.5, slc12a3, and slc9a3.2, were comparable with that in the slc12a10.2-deficient fish. However, these genes are significantly upregulated in slc12a10.2-deficient fish reared in the artificial medium with low- or 0-Na+ content relative to control fish (Figures 4A–D). These results suggest that, though pituitary prl was upregulated in the slc12a10.2-deficient fish when reared under standard conditions, the expressions of the PRL downstream targets in the pronephric duct were not similarly upregulated. However, when the fish inhabit a Na+-poor environment, the PRL downstream targets, i.e., the Na+ transporters in pronephric duct, would be upregulated to relieve the stress of Na+ absorption in the gills.
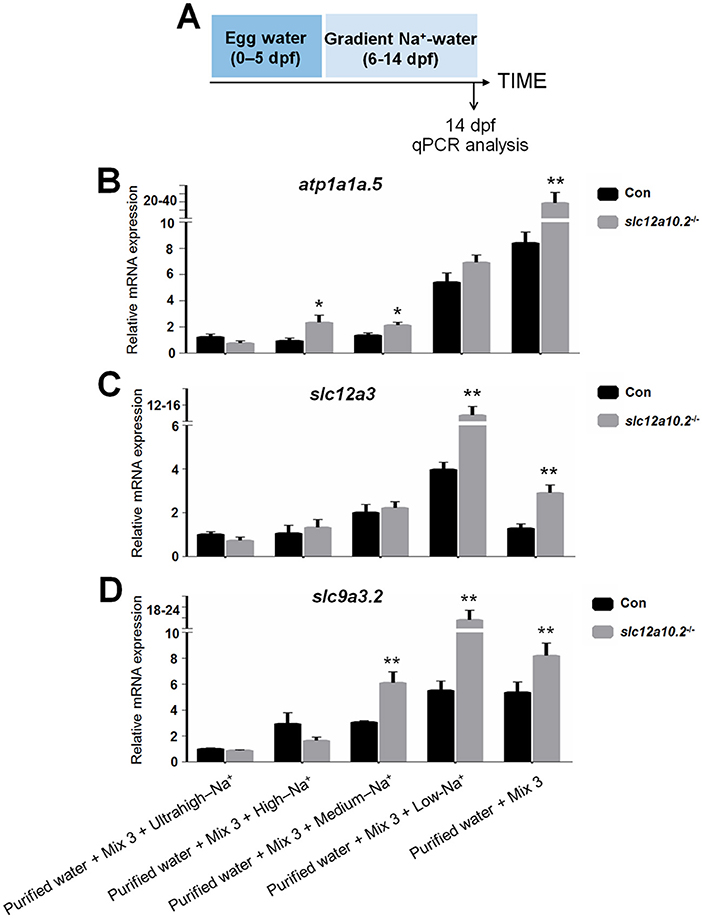
Figure 4. Hypo-Na+, not standard conditions, upregulates the expressions of the transporters. (A) Schematic diagram of the rearing timeline. Larvae were harvested at 14 dpf for qPCR analysis. (B–D) Expression levels of atp1a1a.5, slc12a3, and slc9a3.2 were examined in the control and slc12a10.2-deficient larvae reared in purified water + Mix 3 + ultrahigh-Na+, purified water + Mix 3 + high-Na+, purified water + Mix 3 + medium-Na+, purified water + Mix 3 + low-Na+, and purified water + Mix 3, from 6 to 14 dpf. *P < 0.05. **P < 0.01.
Cas9-Mediated Knockout of slc12a3
WISH staining using a probe against slc12a3 demonstrated that slc12a3 is expressed in the pronephric duct (Figure 5A). A mutant line with an 8 bp deletion in the first exon of slc12a3 was obtained (Figure 5B). To assess the effects of slc12a3 on the Na+ and Cl− concentrations in the entire zebrafish body, we measured the Na+ and Cl− concentrations in slc12a3-deficient fish at 2 months post-fertilization (mpf) (Figure 5C). Surprisingly, the concentrations of Na+ and Cl− were unaffected in the slc12a3-deficient zebrafish (Figures 5D,E). We also found that both male and female slc12a3-deficient fish were capable of surviving to adulthood in a freshwater habitat without any obvious defect (Figures 5F–I) or any significant difference in body weight and body length compared to their control siblings (Figures 5J,K).
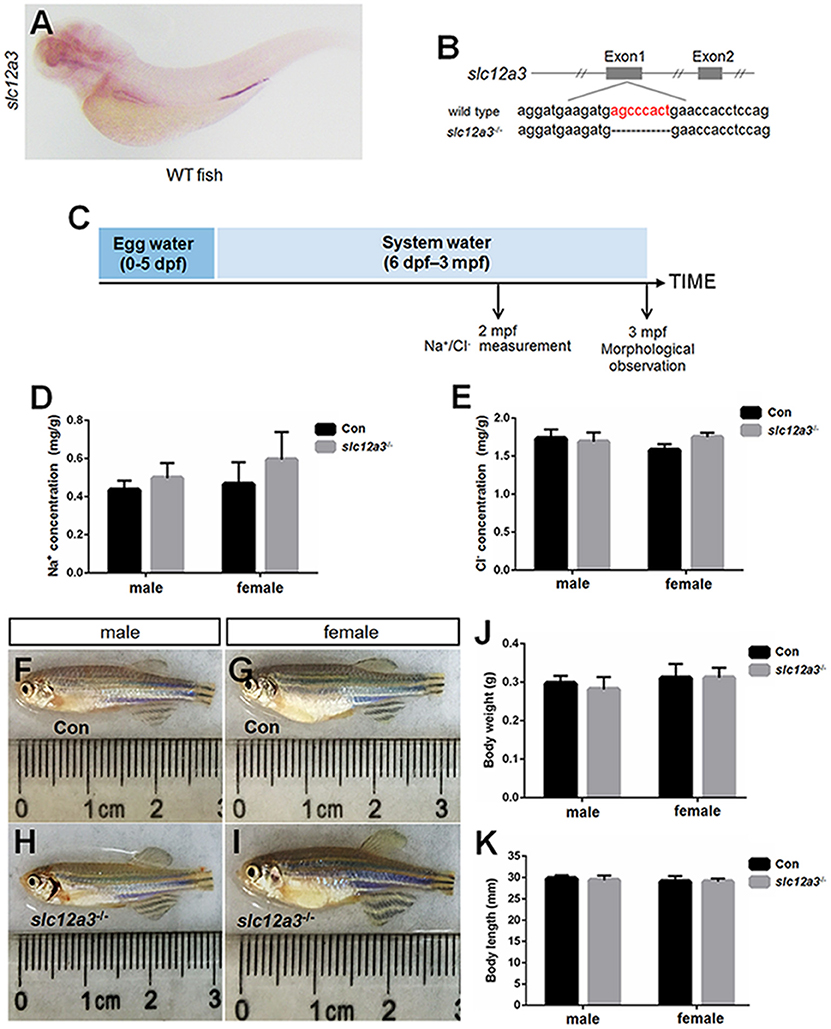
Figure 5. Generation of slc12a3-deficient zebrafish. (A) WISH analysis using the slc12a3 probe in wild-type larvae at 4 dpf. (B) Targeted depletion of the slc12a3 gene. The CRISPR/Cas9 target site is located at exon 1. A genotype with an 8-bp deletion was used to establish the slc12a3 knockout line (highlighted in red). (C) Schematic diagram of the rearing timeline. Larvae were reared in egg water from 0 to 5 dpf and in system water after 5 dpf. The fish at 2 mpf were harvested for Na+ and Cl− measurements. The fish at 3 mpf were harvested for morphological observation. (D,E) Na+ and Cl− concentrations were measured in control and slc12a3-deficient male and female fish at 2 mpf, respectively. (F–I) The general morphological observations of control and slc12a3-deficient male and female at 3 mpf, respectively. (F,G) Male and female control fish at 3 mpf. (H,I) Male and female slc12a3-deficient fish at 3 mpf. (J,K) Body weight and body length of control and slc12a3-deficient male and female at 3 mpf.
The slc12a3-Depletion Upregulates prl and PRL Transcriptional Targets in the Pronephric Duct
In slc12a3-deficient fish at 4 dpf, the upregulation of prl in the pituitary, as well as, atp1a1a.5 and slc12a10.2 in the gills/skin were observed (Figures 6A–G), which are partly similar to the observations in the slc12a10.2-deficient larvae (Figures 3B–E). We observed upregulated expressions of downstream PRL targets, including slc9a3.2 and atp1a1a.5, in pronephric duct (Figures 6F–I). The upregulated expressions of slc12a10.2, atp1a1a.5, and slc9a3.2 were also confirmed by qPCR analysis (Figure 6J). The expressions of prl and prlra were also significantly upregulated in slc12a3-deficient zebrafish compared to control fish, again reflecting the fact that PRL is increased in slc12a3-deficient fish compared with control fish (Figure 6J). The overall response of the downstream PRL targets, both in the gills/skin and pronephric duct, to slc12a3-deficiency are quite different with the observations in slc12a10.2-deficient larvae (Figure 3).
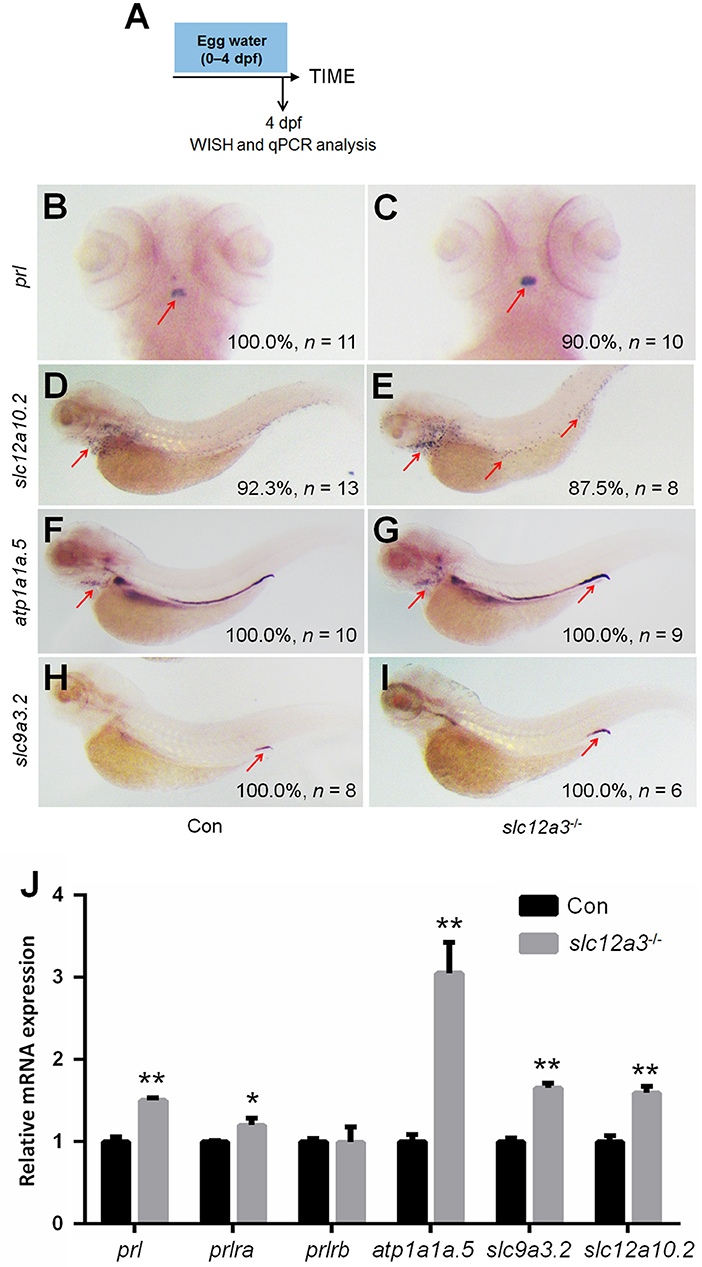
Figure 6. Expression patterns of prl and PRL targets in the slc12a3-deficient larvae at 4 dpf. (A) Schematic diagram of the rearing timeline. Larvae were harvested at 4 dpf for WISH analysis. (B,C) WISH analysis using the probe of prl in control and slc12a3-deficient larvae at 4 dpf. (D,E) WISH analysis using the probe of slc12a10.2 in control and slc12a3-deficient larvae at 4 dpf. (F,G) WISH analysis using the probe of atp1a1a.5 in control and slc12a3-deficient larvae at 4 dpf. (H,I) WISH analysis using the probe of slc9a3.2 in control and slc12a3-deficient larvae at 4 dpf. Red arrows indicate the expressions of the nominated genes. (J) Expression levels of prl, prlra, prlrb, atp1a1a.5, slc9a3.2, and slc12a10.2 in control larvae and slc12a3-deficient larvae at 4 dpf were examined with qPCR assay. *P < 0.05. **P < 0.01.
Discussion
In mammals, NCC (encoded by slc12a3), a thiazide-sensitive membrane protein, is a critical transporter promoting salt reabsorption in the apical membranes of the mammalian distal convoluted tubules of the pronephric duct (43). In zebrafish, two members of the slc12a family, slc12a10.2 (encodes NCC-2b or NCC-like) and slc12a3 (encodes NCC), expressed in the gills/skin and pronephric duct, respectively, are both identified as PRL transcriptional targets (17, 35, 36). In the present study, models with increased endogenous PRL signaling were generated by the knockout of slc12a10.2 and slc12a3. By investigating the functional roles of slc12a10.2 and slc12a3 in zebrafish, the novel regulatory network of PRL signaling was elucidated. Specifically, we demonstrated differential expression of downstream targets in the gills and pronephric duct in zebrafish deficient in slc12a10.2 or slc12a3.
In the present study, the increased expression of pituitary prl was observed in both the slc12a10.2- and slc12a3-deficient zebrafish, supporting the notion that PRL is the key mediator in maintaining the expressions of slc12a10.2 and slc12a3. This study also provides new insight that suggests that PRL is susceptible to deficiencies in downstream ion transporters, as evidenced by the increased transcriptional levels of prl from WISH and qPCR analysis (Figures 3, 6). It has been reported that intraperitoneal injection of ovine PRL increased prlra transcript (5). Intriguingly, the upregulation of prlra was observed in both of the knockout lines, indicating that PRL is increased when the downstream ion transporter was depleted (Figures 3, 6, 7). Although the protein levels of PRL were not examined in the present study, the amplified PRL signaling was still determined in the two knockout lines.
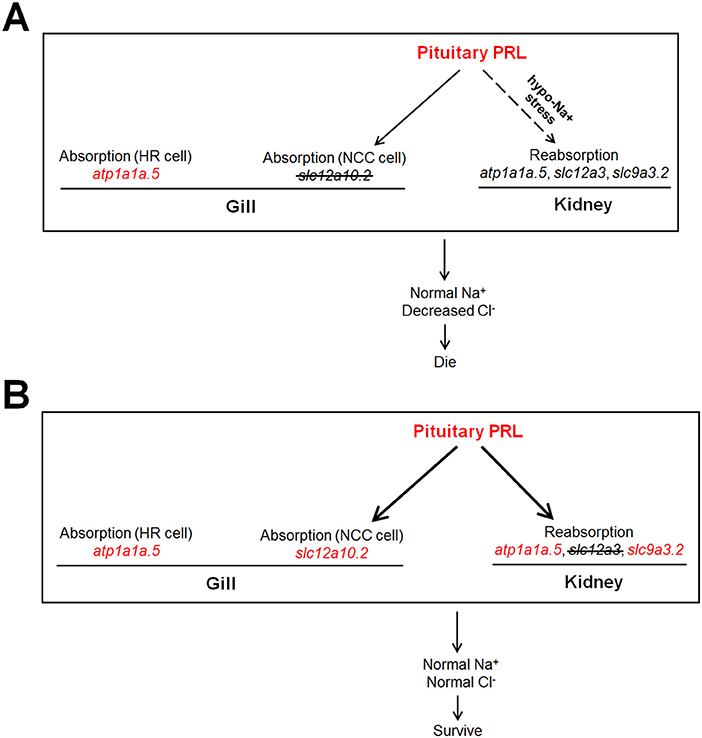
Figure 7. The dissected regulatory network of PRL signaling in the slc12a10.2- and slc12a3-deficient fish, respectively. (A,B) The generations of the model with increased endogenous PRL in zebrafish. Either depletion of slc12a10.2 or slc12a3 could result in increased endogenous PRL effectively. (A) Although pituitary prl is upregulated in the slc12a10.2-deficient fish, the responses of PRL downstream targets in pronephric duct to upregulated prl is not observed, as this pathway could only be conditionally activated under the hypo-Na+ stress. The Na+ absorption maintenance by upregulated atp1a1a.5 in the gills/skin of the slc12a10.2-deficient larvae is evidently observed, which probably compensate to maintain the systemic Na+ concentration. However, the PRL downstream targets in the pronephric duct failed to respond to the increased pituitary PRL. (B) The overall responses of Na+ and Cl− absorption both in the gills/skin and reabsorption in the pronephric duct compensate to maintain normal levels of Na+ and Cl− in the slc12a3-deficient fish. The upregulated expressions of the nominated genes are highlighted in red.
No significant difference in systemic Na+ levels was observed in slc12a10.2- or slc12a3-deficient zebrafish. From the results of WISH, we identified other underlying compensators for Na+ uptake, i.e., upregulated atp1a1a.5 and atp1a1a.5/slc12a10.2 in the gills/skin of the slc12a10.2-deficient fish and slc12a3-deficient fish, respectively. The expressions of PRL transcriptional targets to increased levels of pituitary PRL in the gills/skin and pronephric duct were observed in the slc12a3-deficient zebrafish: elevated expressions of slc9a3.2 (in the pronephric duct), slc12a10.2 (in the gills/skin), and atp1a1a.5 (both in the gills and pronephric duct) were simultaneously observed in slc12a3-deficient fish (Figure 6). Interestingly, the expressions of PRL targets in response to elevated levels of endogenous PRL in the pronephric duct observed in slc12a3-deficient fish were not seen in slc12a10.2-deficient fish. It is clear that the regulation of systemic salt uptake are more complex than previously anticipated, as the absorption of both Na+ and Cl− take place in the pronephric duct and gills/skin, and these two organs work cooperatively to regulate the systemic levels of Na+ and Cl− (9, 44). It has been reported that slc12a10.2 is the transcriptional target of PRL, as both knockdown and knockout of prl result in the downregulation of slc12a10.2 in the gills/skin of zebrafish (10, 17). It has been shown that the ovine PRL supplement is sufficient to upregulate slc12a10.2 expression both in vivo and in vitro. The upregulation of slc12a10.2 by ovine PRL indicates that slc12a10.2 can be upregulated by exogenous PRL supplementation (5). These previous results are in agreement with the observed upregulation of slc12a10.2 in response to elevated levels of endogenous PRL in the gills/skin of slc12a3-deficient fish (Figure 6).
Unlike the gill slc12a10.2 and pronephric slc9a3.2, which are competent to respond to elevated levels of endogenous PRL caused by slc12a3 depletion, the pronephric duct slc12a3 did not respond to elevated levels of PRL caused by the depletion of slc12a10.2 (Figures 3, 6). It seems that the pronephric duct transporters, slc12a3, slc9a3.2, and atp1a1a.5, undergo differential transcriptional regulation of PRL signaling in the gills/skin of slc12a10.2-deficient zebrafish. This, therefore, leads to the failed response of the pronephric duct genes slc12a3 and slc9a3.2 to the increased levels of pituitary PRL in the slc12a10.2-deficient fish when reared under the standard conditions (Figures 3F–I). The differential response of the gills/skin and pronephric duct to manipulated PRL signaling is in agreement with the previous study. Although the expressions of prl, slc12a10.2, and slc9a3.2, as well as, the plasma levels of PRL, increase in response to a low salinity environment (18, 45–47), exogenous supplementation with ovine PRL, which is sufficient to upregulate slc12a10.2, failed to upregulate slc9a3.2 (5). Taken together, in regard to the slc12a10.2-deficiency, we conclude that when fish are reared under standard conditions with suitable levels of Na+, the pronephric duct transporters do not respond to the elevated pituitary PRL caused by the depletion of slc12a10.2 (Figures 3F–I). However, slc12a10.2-deficient zebrafish need the intact response in the gills/skin and pronephric duct to cope with hypo-Na+ stress. Thus, this PRL pathway potentially helps zebrafish rapidly acclimate to dramatic reductions in Na+ concentrations and adapt to natural conditions (Figures 4A–D).
Cl− is the main anion with biological functions, such as cell volume regulation, transport of salt and fluid across epithelia, muscle contraction, charge compensation, and acidification of intracellular organelles (48). In humans, mutation of the chloride transporters, clc-1 or clc-kb, causes myotonia and Bartter's syndrome, respectively. In mice, knockout of clc-k1 leads to diabetes insipidus (49, 50), whereas the clc-2 knockout mice demonstrated blindness, sterility, and leukodystrophy by unknown reasons (51–53). More specifically, knockout of the chloride transporter clcc1 in zebrafish leads to damaged retinal morphology and function and lethality at 11 dpf (54). It has been shown that both slc12a10.2 and slc12a3 are responsible for Cl− uptake, and gill mRNA expression of slc12a10.2 was induced by a low-Cl− environment; however, a normal Cl− concentration in slc12a3-deficient fish at 2 mpf was observed, which probably resulted from the upregulation of slc12a10.2 in the gills/skin. We also demonstrated that slc12a10.2-deficiency in fish was lethal due to defective Cl− uptake. This result clearly demonstrated that Slc12a10.2 plays an essential role in Cl− uptake; however, the role Slc12a3 plays in Cl− absorption could not be completely concluded, as the potential compensatory effect of Slc12a10.2 may contribute to chloride absorption.
The naturally occurring mutations found in the human SLC12A3 gene have been identified in patients suffering from a salt-wasting disorder called Gitelman's syndrome (55). It is interesting that, unlike the mammals, zebrafish possess multiple subtypes of NCC encoded by distinct mRNAs (36). In larval fish, the relative abundance of slc12a10.2 and slc12a3 differ during early embryonic development (Figures 1, 5). Although downregulation of slc12a10.2 and slc12a3 were observed in prl-knockdown or prl-knockout fish (10, 17), distinct expression patterns suggest that they may undergo a complicated regulatory network of PRL signaling. The phenotypes associated with the SLC12A3 gene mutation is in sharp contrast to our observations of the slc12a10.2- and slc12a3-deficient fish. This suggests that overlapping roles of Slc12a10.2 and Slc12a3 in maintaining Na+/Cl− absorption may exist in zebrafish. However, the different phenotypes of slc12a10.2- and slc12a3-deficient fish also suggest that Slc12a10.2 and Slc12a3 exhibit diversified functions in maintaining Na+/Cl− absorption. The different results obtained from the slc12a10.2- and slc12a3-deficient fish, e.g., the conditional and non-conditional-activated PRL signaling, may be caused by the diversified functions of the two transporters in maintaining Na+/Cl− absorption. Such differences between the two transporters in osmoregulation in teleosts were also observed in a tilapia PRL study, where tilapia PRL188 injection resulted in a dose-dependent effect on ion retention, as evidenced by an increase in the plasma levels of Na+ and Cl−, but PRL177 injection caused only slight increases in plasma Na+ and Cl− that were not dose-dependent (56).
In the present study, a zebrafish model was used for the dissection of the PRL regulatory signaling network, as zebrafish inhabit hypotonic freshwater environments and have emerged as an important model for investigating osmoregulation, especially with regard to the genetic editing and manipulation. To our knowledge, there has been no report of a knockout model with increased endogenous PRL signaling or the evaluation of responsive expressions of ion transporters in zebrafish. Unlike the previous studies established from the knockout or knockdown experiments of pituitary prl, the present study regarding the knockout of slc12a10.2 and slc12a3 in zebrafish are more integrative to the dissection of the regulatory network of PRL signaling by exploring the transcriptional expressions of PRL targets in response to increase in pituitary PRL caused by the depletion of ion transporters that are tissue-specific (Figure 7). To further our understanding of the physiological roles of NCC transporters, it would be useful if future studies obtain the slc12a10.2/slc12a3 double-knockout fish. Moreover, the detailed mechanisms concerning the failed responses of the transporters in the pronephric duct to elevated levels of pituitary PRL in the slc12a10.2-deficient fish require further research. Thus, it would be a challenging, but important issue to investigate whether there is any other hypo-Na+-mediated transcriptional factor that may be responsible for the conditionally-activated transcription of the transporters in the pronephric duct of slc12a10.2-deficient fish (Figure 7).
Author Contributions
TS and YS conducted most of the experiments for this work. YG, XJ, and JH provided help with genotyping and fish rearing. GZ performed training and provided insights for this work. GZ wrote the paper and prepared all of the figures. ZY initiated and supervised the research team and revised the paper. All the authors approved the final manuscript.
Funding
This work was supported by the National Natural Science Foundation of China (No. 31501857 to GZ and 31530077 to ZY), the National Basic Research Program of China (973 Program, 2014CB138602 to ZY), the Pilot Program A Project from the Chinese Academy of Sciences (XDA08010405 to ZY), and the Knowledge Innovation Program of the Chinese Academy of Sciences (to GZ).
Conflict of Interest Statement
The authors declare that the research was conducted in the absence of any commercial or financial relationships that could be construed as a potential conflict of interest.
References
1. McCormick SD, Bradshaw D. Hormonal control of salt and water balance in vertebrates. Gen Comp Endocrinol. (2006) 147:3–8. doi: 10.1016/j.ygcen.2005.12.009
2. Guh YJ, Hwang PP. Insights into molecular and cellular mechanisms of hormonal actions on fish ion regulation derived from the zebrafish model. Gen Comp Endocrinol. (2017) 251:12–20. doi: 10.1016/j.ygcen.2016.08.009
3. Bachelot A, Binart N. Reproductive role of prolactin. Reproduction (2007) 133:361–9. doi: 10.1530/REP-06-0299
4. Bole-Feysot C, Goffin V, Edery M, Binart N, Kelly PA. Prolactin (PRL) and its receptor: actions, signal transduction pathways and phenotypes observed in PRL receptor knockout mice. Endocrine Rev. (1998) 19:225–68. doi: 10.1210/edrv.19.3.0334
5. Breves JP, Serizier SB, Goffin V, McCormick SD, Karlstrom RO. Prolactin regulates transcription of the ion uptake Na+/Cl- cotransporter (ncc) gene in zebrafish gill. Mol Cell Endocrinol. (2013) 369:98–106. doi: 10.1016/j.mce.2013.01.021
6. Manzon LA. The role of prolactin in fish osmoregulation: a review. Gen Comp Endocrinol. (2002) 125:291–310. doi: 10.1006/gcen.2001.7746
8. Pickford GE, Phillips JG. Prolactin, a factor in promoting survival of hypophysectomized killifish in fresh water. Science (1959) 130:454–5. doi: 10.1126/science.130.3373.454
9. Guh YJ, Lin CH, Hwang PP. Osmoregulation in zebrafish: ion transport mechanisms and functional regulation. EXCLI J. (2015) 14:627–59. doi: 10.17179/excli2015–246
10. Breves JP, McCormick SD, Karlstrom RO. Prolactin and teleost ionocytes: new insights into cellular and molecular targets of prolactin in vertebrate epithelia. Gen Comp Endocrinol. (2014) 203:21–8. doi: 10.1016/j.ygcen.2013.12.014
11. Edery M, Young G, Bern HA, Steiny S. Prolactin receptors in tilapia (Sarotherodon mossambicus) tissues: binding studies using 125I-labeled ovine prolactin. Gen Comp Endocrinol. (1984) 56:19–23. doi: 10.1016/0016-6480(84)90056-X
12. Weng CF, Lee TH, Hwang PP. Immune localization of prolactin receptor in the mitochondria-rich cells of the euryhaline teleost (Oreochromis mossambicus) gill. FEBS Lett. (1997) 405:91–4. doi: 10.1016/S0014-5793(97)00162-2
13. Sandra O, Sohm F, de Luze A, Prunet P, Edery M, Kelly PA. Expression cloning of a cDNA encoding a fish prolactin receptor. Proc Natl Acad Sci USA. (1995) 92:6037–41. doi: 10.1073/pnas.92.13.6037
14. Santos CR, Ingleton PM, Cavaco JE, Kelly PA, Edery M, Power DM. Cloning, characterization, and tissue distribution of prolactin receptor in the sea bream (Sparus aurata). Gen Comp Endocrinol. (2001) 121:32–47. doi: 10.1006/gcen.2000.7553
15. Prunet P, Sandra O, Le Rouzic P, Marchand O, Laudet V. Molecular characterization of the prolactin receptor in two fish species, tilapia Oreochromis niloticus and rainbow trout, Oncorhynchus mykiss: a comparative approach. Can J Physiol Pharmacol. (2000) 78:1086–96. doi: 10.1139/cjpp-78-12-1086
16. Chen M, Huang X, Yuen DS, Cheng CH. A study on the functional interaction between the GH/PRL family of polypeptides with their receptors in zebrafish: evidence against GHR1 being the receptor for somatolactin. Mol Cell Endocrinol. (2011) 337:114–21. doi: 10.1016/j.mce.2011.02.006
17. Shu Y, Lou Q, Dai Z, Dai X, He J, Hu W, et al. The basal function of teleost prolactin as a key regulator on ion uptake identified with zebrafish knockout models. Sci Rep. (2016) 6:18597. doi: 10.1038/srep18597
18. Liu NA, Liu Q, Wawrowsky K, Yang Z, Lin S, Melmed S. Prolactin receptor signaling mediates the osmotic response of embryonic zebrafish lactotrophs. Mol Endocrinol. (2006) 20:871–80. doi: 10.1210/me.2005–0403
19. Fukamachi S, Meyer A. Evolution of receptors for growth hormone and somatolactin in fish and land vertebrates: lessons from the lungfish and sturgeon orthologues. J Mol Evolu. (2007) 65:359–72. doi: 10.1007/s00239–07–9035–7
20. Huang X, Jiao B, Fung CK, Zhang Y, Ho WK, Chan CB, et al. The presence of two distinct prolactin receptors in seabream with different tissue distribution patterns, signal transduction pathways and regulation of gene expression by steroid hormones. J Endocrinol. (2007) 194:373–92. doi: 10.1677/JOE-07–076
21. Fiol DF, Sanmarti E, Sacchi R, Kultz D. A novel tilapia prolactin receptor is functionally distinct from its paralog. J Exp Biol. (2009) 212 (Pt 13):2007–15. doi: 10.1242/jeb.025601
22. Breves JP, Seale AP, Helms RE, Tipsmark CK, Hirano T, Grau EG. Dynamic gene expression of GH/PRL-family hormone receptors in gill and kidney during freshwater-acclimation of Mozambique tilapia. Comp Biochem Physiol Part A Mol Integr Physiol. (2011) 158:194–200. doi: 10.1016/j.cbpa.2010.10.030
23. Breves JP, Watanabe S, Kaneko T, Hirano T, Grau EG. Prolactin restores branchial mitochondrion-rich cells expressing Na+/Cl- cotransporter in hypophysectomized Mozambique tilapia. Am J Physiol Regul Integr Comp Physiol. (2010) 299:R702–10. doi: 10.1152/ajpregu.00213.2010
24. Hwang PP. Ion uptake and acid secretion in zebrafish (Danio rerio). J Exp Biol. (2009) 212 (Pt 11):1745–52. doi: 10.1242/jeb.026054
25. Huang P, Xiao A, Zhou M, Zhu Z, Lin S, Zhang B. Heritable gene targeting in zebrafish using customized TALENs. Nat Biotechnol. (2011) 29:69–700. doi: 10.1038/nbt.1939
26. Bedell VM, Wang Y, Campbell JM, Poshusta TL, Starker CG, Krug RG II, et al. In vivo genome editing using a high-efficiency TALEN system. Nature (2012) 491:114–8. doi: 10.1038/nature11537
27. Hwang WY, Fu Y, Reyon D, Maeder ML, Tsai SQ, Sander JD, et al. Efficient genome editing in zebrafish using a CRISPR-Cas system. Nat Biotechnol. (2013) 31:227–9. doi: 10.1038/nbt.2501
28. Hwang PP, Chou MY. Zebrafish as an animal model to study ion homeostasis. Pflugers Archiv. (2013) 465:123–47. doi: 10.1007/s00424–013–1269–1
29. Garciaromeu F, Maetz J. The mechanism of sodium and chloride uptake by the gills of a fresh-water fish, Carassius Auratus. I. Evidence for an independent uptake of sodium and chloride ions. J Gen Physiol. (1964) 47:1195–207. doi: 10.1085/jgp.47.6.1195
30. Perry SF, Shahsavarani A, Georgalis T, Bayaa M, Furimsky M, Thomas SL. Channels, pumps, and exchangers in the gill and kidney of freshwater fishes: their role in ionic and acid-base regulation. J Exp Zool Part A Comp Exp Biol. (2003) 300:53–62. doi: 10.1002/jez.a.10309
31. Lin CH, Tsai RS, Lee TH. Expression and distribution of Na, K-ATPase in gill and kidney of the spotted green pufferfish, Tetraodon nigroviridis, in response to salinity challenge. Comp Biochem Physiol Part A Mol Integr Physiol. (2004) 138:287–95. doi: 10.1016/j.cbpb.2004.04.005
32. Takei Y, Hiroi J, Takahashi H, Sakamoto T. Diverse mechanisms for body fluid regulation in teleost fishes. Am J Physiol Regul Integr Compar Physiol. (2014) 307:R778–92. doi: 10.1152/ajpregu.00104.2014
33. Evans DH. Teleost fish osmoregulation: what have we learned since August Krogh, Homer Smith, and Ancel Keys. Am J Physiol Regul Integr Comp Physiol. (2008) 295:R704–13. doi: 10.1152/ajpregu.90337.2008
34. Miyazaki H, Kaneko T, Uchida S, Sasaki S, Takei Y. Kidney-specific chloride channel, OmClC-K, predominantly expressed in the diluting segment of freshwater-adapted tilapia kidney. Proc Natl Acad Sci USA. (2002) 99:15782–7. doi: 10.1073/pnas.242611099
35. Boisen AM, Amstrup J, Novak I, Grosell M. Sodium and chloride transport in soft water and hard water acclimated zebrafish (Danio rerio). Biochim Biophys Acta (2003) 1618:207–18. doi: 10.1016/j.bbamem.2003.08.016
36. Wang YF, Tseng YC, Yan JJ, Hiroi J, Hwang PP. Role of SLC12A10.2, a Na-Cl cotransporter-like protein, in a Cl uptake mechanism in zebrafish (Danio rerio). Am J Physiol Regul Integr Comp Physiol. (2009) 296:R1650–60. doi: 10.1152/ajpregu.00119.2009
37. Kimmel CB, Ballard WW, Kimmel SR, Ullmann B, Schilling TF. Stages of embryonic development of the zebrafish. Dev Dyn. (1995) 203:253–310. doi: 10.1002/aja.1002030302
38. Sander JD, Zaback P, Joung JK, Voytas DF, Dobbs D. Zinc finger targeter (ZiFiT): an engineered zinc finger/target site design tool. Nucleic Acids Res. (2007) 35:W599–605. doi: 10.1093/nar/gkm349
39. Zhang Z, Zhu B, Ge W. Genetic analysis of zebrafish gonadotropin (FSH and LH) functions by TALEN-mediated gene disruption. Mol Endocrinol. (2015) 29:76–98. doi: 10.1210/me.2014–1256
40. Zhai G, Gu Q, He J, Lou Q, Chen X, Jin X, et al. Sept6 is required for ciliogenesis in Kupffer's vesicle, the pronephros, and the neural tube during early embryonic development. Mol Cell Biol. (2014) 34:1310–21. doi: 10.1128/MCB.01409–13
41. Bustin SA, Benes V, Garson JA, Hellemans J, Huggett J, Kubista M, et al. The MIQE guidelines: minimum information for publication of quantitative real-time PCR experiments. Clin Chem. (2009) 55:61–2. doi: 10.1373/clinchem.2008.112797
42. Pierce AL, Fox BK, Davis LK, Visitacion N, Kitahashi T, Hirano T, et al. Prolactin receptor, growth hormone receptor, and putative somatolactin receptor in Mozambique tilapia: tissue specific expression and differential regulation by salinity and fasting. Gen Comp Endocrinol. (2007) 154:31–40. doi: 10.1016/j.ygcen.2007.06.023
43. Renfro JL. Water and ion transport by the urinary bladder of the teleost Pseudopleuronectes americanus. Am J Physiol. (1975) 228:52–61. doi: 10.1152/ajplegacy.1975.228.1.52
44. Wingert RA, Davidson AJ. The zebrafish pronephros: a model to study nephron segmentation. Kidney Int. (2008) 73:1120–7. doi: 10.1038/ki.2008.37
45. Hoshijima K, Hirose S. Expression of endocrine genes in zebrafish larvae in response to environmental salinity. J Endocrinol. (2007) 193:481–91. doi: 10.1677/JOE-07–03
46. Lee KM, Kaneko T, Aida K. Prolactin and prolactin receptor expressions in a marine teleost, pufferfish Takifugu rubripes. Gen Comp Endocrinol. (2006) 146:318–28. doi: 10.1016/j.ygcen.2005.12.003
47. Yada T, Hirano T, Grau EG. Changes in plasma levels of the two prolactins and growth hormone during adaptation to different salinities in the euryhaline tilapia, Oreochromis mossambicus. Gen Comp Endocrinol. (1994) 93:214–23. doi: 10.1006/gcen.1994.1025
48. Perez-Rius C, Gaitan-Penas H, Estevez R, Barrallo-Gimeno A. Identification and characterization of the zebrafish ClC-2 chloride channel orthologs. Pflugers Archiv. (2015) 467:1769–81. doi: 10.1007/s00424–014–1614-z
49. Kornak U, Kasper D, Bosl MR, Kaiser E, Schweizer M, Schulz A, et al. Loss of the ClC-7 chloride channel leads to osteopetrosis in mice and man. Cell (2001) 104:205–15. doi: 10.1016/S0092-8674(01)00206-9
50. Simon DB, Bindra RS, Mansfield TA, Nelson-Williams C, Mendonca E, Stone R, et al. Mutations in the chloride channel gene, CLCNKB, cause Bartter's syndrome type III. Nat Genet. (1997) 17:171–8. doi: 10.1038/ng1097–171
51. Blanz J, Schweizer M, Auberson M, Maier H, Muenscher A, Hubner CA, et al. Leukoencephalopathy upon disruption of the chloride channel ClC-2. J Neurosci. (2007) 27:6581–9. doi: 10.1523/JNEUROSCI.0338–07.2007
52. Bosl MR, Stein V, Hubner C, Zdebik AA, Jordt SE, Mukhopadhyay AK, et al. Male germ cells and photoreceptors, both dependent on close cell-cell interactions, degenerate upon ClC-2 Cl(-) channel disruption. EMBO J. (2001) 20:1289–9. doi: 10.1093/emboj/20.6.1289
53. Matsumura Y, Uchida S, Kondo Y, Miyazaki H, Ko SB, Hayama A, et al. Overt nephrogenic diabetes insipidus in mice lacking the CLC-K1 chloride channel. Nat Genet. (1999) 21:95–8. doi: 10.1038/5036
54. Li L, Jiao X, D'Atri I, Ono F, Nelson R, Chan CC, et al. Mutation in the intracellular chloride channel CLCC1 associated with autosomal recessive retinitis pigmentosa. PLoS Genet. (2018) 14:e1007504. doi: 10.1371/journal.pgen.1007504
55. Gagnon KB, Delpire E. Physiology of SLC12 transporters: lessons from inherited human genetic mutations and genetically engineered mouse knockouts. Am J Physiol Cell Physiol. (2013) 304:C693–714. doi: 10.1152/ajpcell.00350.2012
Keywords: prolactin, ion transporter, gills/skin, pronephric duct, slc12a10.2, slc12a3
Citation: Shu T, Shu Y, Gao Y, Jin X, He J, Zhai G and Yin Z (2018) Depletion of Tissue-Specific Ion Transporters Causes Differential Expression of PRL Targets in Response to Increased Levels of Endogenous PRL. Front. Endocrinol. 9:683. doi: 10.3389/fendo.2018.00683
Received: 06 September 2018; Accepted: 01 November 2018;
Published: 20 November 2018.
Edited by:
Gen He, Ocean University of China, ChinaReviewed by:
Shaojun Liu, Hunan Normal University, ChinaTakashi Yada, Japan Fisheries Research and Education Agency (FRA), Japan
Copyright © 2018 Shu, Shu, Gao, Jin, He, Zhai and Yin. This is an open-access article distributed under the terms of the Creative Commons Attribution License (CC BY). The use, distribution or reproduction in other forums is permitted, provided the original author(s) and the copyright owner(s) are credited and that the original publication in this journal is cited, in accordance with accepted academic practice. No use, distribution or reproduction is permitted which does not comply with these terms.
*Correspondence: Gang Zhai, emhhaWdhbmdAaWhiLmFjLmNu
Zhan Yin, enlpbkBpaGIuYWMuY24=
†These authors have contributed equally to this work