- 1Department of Glaciology and Climate, Geological Survey of Denmark and Greenland, Copenhagen, Denmark
- 2Department of Earth Sciences, University of New Brunswick, Fredericton, New Brunswick, Canada
- 3Department of Marine Geology, Geological Survey of Denmark and Greenland, Copenhagen, Denmark
- 4Paleoceanography and Paleoclimate Group, Aarhus University, Aarhus, Denmark
- 5Arctic Research Centre, Aarhus University, Aarhus, Denmark
- 6iClimate, Aarhus University Interdisciplinary Centre for Climate Change, Aarhus University, Aarhus, Denmark
Four marine sediment cores from two sites in the Independence Fjord system near the Wandel Sea in eastern North Greenland were analyzed for their dinoflagellate cyst (dinocyst) and foraminiferal fossil content to gain insight into the water mass properties and evolution of the outer fjord system over the Holocene and Last Glacial Period. While regarded as a climate-sensitive region, the climatic history of the area remains largely unknown and has been documented through the study of two composite marine sediment cores only once before. The results presented here reveal that Atlantic waters entered the Independence Fjord episodically during the studied interval. High concentrations of dinocysts and foraminifers in the upper few centimeters of the cores are in line with oceanographic measurements clearly illustrating that Atlantic-sourced waters make up the lower part of the water column in the area in modern times. Radiocarbon dating of foraminiferal tests and increasing microfossil concentrations and diversity toward the top of the cores suggest that this inflow has been occurring for at least 2,000 years and intensified toward recent times. The core sections below the upper few centimeters are devoid of (Quaternary) dinocysts and calcareous foraminifera with the exception of the lowermost segments of the longer cores. While low foraminiferal test quantities in these lowermost core sections prevent precise age determination, their radiocarbon ages reveal that they were deposited prior to 30,000 years ago, indicating the existence of a pathway for the occasional intrusion of Atlantic-sourced waters into, and thus relatively small local ice caps around, the fjord system prior to the Last Glacial Maximum. The previously documented early Holocene inflow of Atlantic-sourced waters was not detected in our records, likely suggesting a strong topographical and deglacial control on the routing of these water masses during the early Holocene.
Introduction
The Independence, Hagen and Danmark fjords—hereafter simply referred to as the Independence Fjord system—located in eastern North Greenland between Peary Land and Kronprins Christian Land, constitute a large system of outlet glaciers at the northeastern edge of the Greenland Ice Sheet (Figure 1). The Independence Fjord system is about 150 km long and is under the influence of two glaciers, the Academy Glacier and Hagen Glacier, which discharge from the Greenland Ice Sheet into the Independence and Hagen fjords, respectively. At present, the glacier fronts are situated well inland of the fjord mouths, but the system functioned as a major outlet for the Greenland Ice Sheet during the last deglaciation, draining into the Wandel Sea (e.g., Nørgaard-Pedersen et al., 2003; Nørgaard-Pedersen et al., 2008). The area is located just north of Fram Strait, the most important pathway for water and heat exchange between the Arctic Ocean and the Nordic Seas. Relatively warm and saline waters of Atlantic origin move northwards through eastern Fram Strait into the Arctic Ocean, while fresh and cold waters from the Arctic Ocean, consisting of variable amounts of arctic shelf-sourced, recirculated Atlantic, and Pacific waters, flow southward along the east coast of Greenland, where they are joined with and exert an influence on the wider North Atlantic ocean circulation.
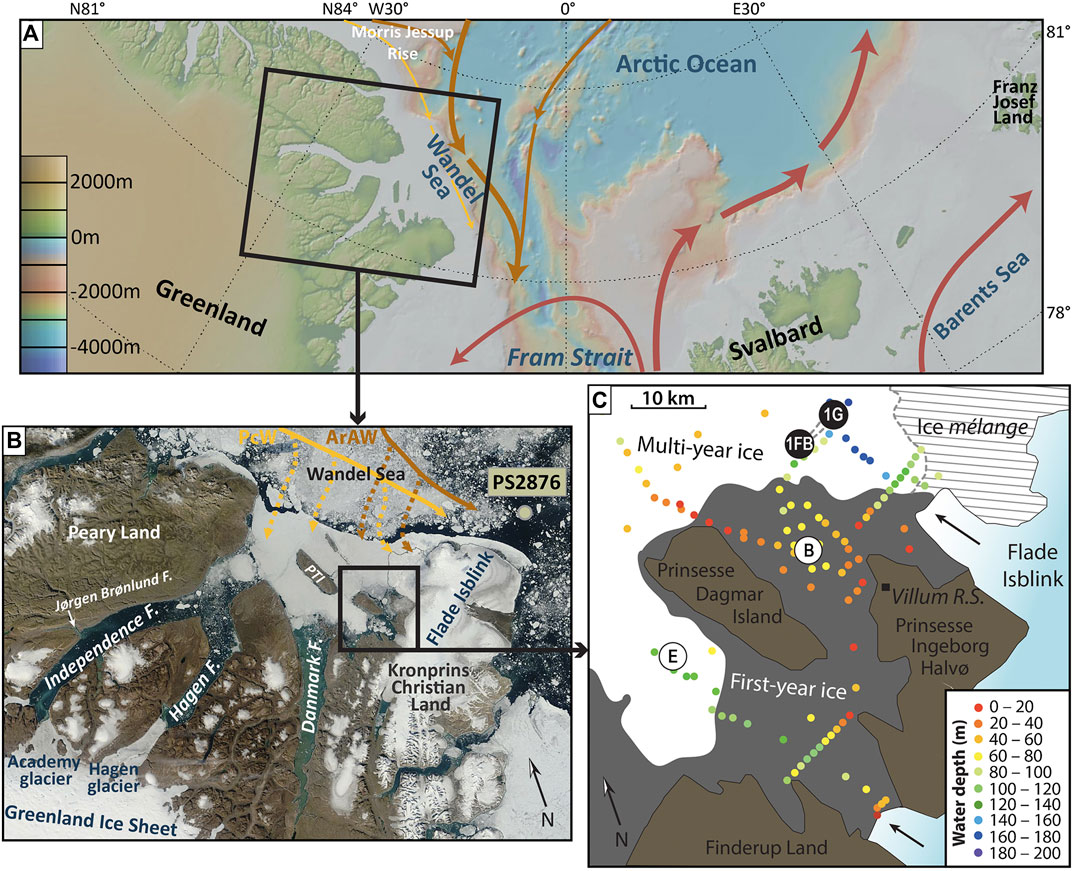
FIGURE 1. (A) Map of the larger study area with its main surface currents. Red arrows indicate relatively warm and saline Atlantic water, orange arrows modified, Arctic-derived Atlantic water, and yellow arrows Pacific water. Basemap created using GeoMapApp (www.geomapapp.org)/CC BY/CC BY (Ryan et al., 2009). (B) Satellite image of the fjord system and ice cover around the study area (image from August 13, 2015; credit: NASA Worldview: https://worldview.earthdata.nasa.gov). Also shown are the location of core PS2876 just off the Wandel Sea shelf (Nørgaard-Pedersen et al., 2003) and the flow paths of Pacific water (PcW; yellow) and Arctic-derived Atlantic water (ArAW; orange) along and on the shelf (modified from Kirillov et al., 2017). PTI = Prinsesse Thyra Island. (C) Detailed view of the study area around the Villum Research Station at Station Nord, showing the location of sites 1Fb (cores K6 and R1) and 1G (cores K9 and R3). Also shown are the locations of sites E and B from Nørgaard-Pedersen et al. (2008). Arrows indicate the flow direction of the two main western outlet glaciers from the Flade Isblink icecap.
The present-day oceanography of the area has recently been investigated by Dmitrenko et al. (2017), Kirillov et al. (2017) and Bendtsen et al. (2017), the latter two studies focusing on the water column properties directly at the tidewater glacier terminus of the Flade Isblink icecap (Figure 1). In the fjord mouth area around Station Nord, the water column is structured into five distinguishable layers (Dmitrenko et al., 2017). Underneath a thin (∼15 m) and fresh (salinity 1–21) upper layer formed by runoff and meltwater lies a cold (−1.5 to −1.75°C) and low-salinity (30–31.2) “halostad” consisting of waters of Pacific origin. This water mass reaches down to about 65 m and overlies a ∼35 m thick halocline, across which temperature increases to −0.65°C and salinity increases to ∼34. Temperature and salinity continue to increase gradually throughout the underlying “Atlantic-modified polar water”—Atlantic water that was modified as it circulated the Arctic basin before exiting again through Fram Strait—and eventually exceed 0°C and 34 respectively, identifying the presence of Atlantic Water below ∼140 m water depth (Dmitrenko et al., 2017).
During the Last Glacial Maximum (LGM)—the ultimate stage of the last ice age (the Weichselian glaciation) between ∼19 and 23 ka BP (e.g., Clark and Mix, 2002)—the ice sheet discharged directly onto the Wandel Sea shelf (Nørgaard-Pedersen et al., 2003; Nørgaard-Pedersen et al., 2008, and references therein). Following this period of maximum ice cover, land-based evidence such as raised marine deposits, driftwood, and lake sediment cores, have revealed that the coastal regions of southern Peary Land and eastern Kronprins Christian Land were deglaciated by about 11 to 9.7 ka BP at the latest (e.g., Bennike & Björck, 2002, and references therein). The limited data available from within the fjord system suggest that Finderup Land, on northwestern Kronprins Christian Land (Figure 1), was deglaciated by 10.8 ± 0.2 ka BP at the latest (Strunk et al., 2018) and that the ice sheet had retreated to at least the Jørgen Brønlund Fjord halfway the northern Independence Fjord by 10.2 ka BP, while the Hagen glacier reached its present extent only by ∼7.6 ka BP (Bennike and Björck, 2002). As a result of ice sheet retreat, the ice cover on Kronprins Christian Land became isolated and persisted as a local ice cap, the Flade Isblink, which at present still drains into the fjord system via two main outlets to the northeast and south of Station Nord (Figure 1). Recent findings suggest that the outlets of the Flade Isblink retreated inland beyond their present position from ∼9.4 to 0.2 ka BP, but also that the icecap never disappeared completely during the Holocene (Larsen et al., 2019). The few available marine sediment records show that Atlantic-sourced waters followed the retreating ice sheet and spread across the Wandel Sea shelf and into the Independence Fjord system during the early Holocene (Nørgaard-Pedersen et al., 2008; Strunk et al., 2018). During the Holocene Thermal Maximum (∼8–5 ka BP), sea-ice free waters are believed to have prevailed during much of the summer months (e.g., Funder et al., 2011a; Funder et al., 2011b). However, the hydrography of the Independence Fjord system during much of this Holocene period is unclear, mainly due to low sedimentation rates and the scarcity of radiocarbon-datable material recovered at the few studied sites so far.
The paucity of available records from the Independence Fjord system can easily be explained by its remoteness. Even if the area was intermittently inhabited by the pre-Inuit cultures Independence I and Greenlandic Dorset during the mid to late Holocene (Grønnow and Sørensen, 2006; Grønnow, 2016; Jensen, 2016), the semi-permanent fast ice cover in the fjords prevents ships from entering them, and the first (scientific) explorations, using dog sledges, only took place in the early 20th century, by illustrious adventurers such as Robert Peary, Ludvig Mylius-Erichsen and Lauge Koch (e.g., Amdrup, 1912–1917; E. Mikkelsen in Amdrup, 1913; Koch, 1928). In the 1950s, “Station Nord” was established on northern Kronprins Christian Land as a weather and telecommunication station, evolving into a military base from which scientific activity increasingly took place from the early 1970s on. It was not until 2005 that the first marine sediment cores from the fjord system were recovered (Nørgaard-Pedersen et al., 2008), and with the opening of the Villum Research Station at Station Nord in 2015 also came the first comprehensive oceanographic and marine geoscience research campaign in the Independence Fjord system (Nørgaard-Pedersen et al., 2016; Dmitrenko et al., 2017; Limoges et al., 2018). The present study is the first report on the sedimentological and microfossil analysis of four sediment cores recovered from the deeper parts of the Independence Fjord system during the 2015 campaign. The overall aim of this study is thus to shed light on the ocean-cryosphere interactions during the late Quaternary evolution of this remote area at the fringe of the Arctic Ocean.
Materials and Methods
Sediment Core Sampling
Four sediment cores were recovered in 2015 from two sites east of Prinsesse Dagmar Island about 20 km north of Station Nord and the present front of the southern Flade Isblink outlet glacier (Figure 1). At each of the two sites, a short and a more voluminous longer core were recovered by drilling through the—in this case—first-year ice and using a Kajak Corer and Rumohr Lot Corer, respectively, attached to a tripod with a top-mounted winch (Nørgaard-Pedersen et al., 2016). At site 1Fb (81.766°N, 16.825°W), 17.5 cm-long core K6 and 54 cm-long core R1 were recovered in this way, from a water depth of 128 m. Cores K9 and R3 from site 1G (81.779°N, 16.596°W) measure 11 and 41 cm, respectively, and were recovered from a water depth of 154.5 m (Limoges et al., 2018). The sediment consists of brown silty clay, gradually changing to dark gray silty clay around ∼30 cm in the longer cores R1 and R3, with scattered rare granules and some irregular small mottling throughout (Figures 2, 3). Prior to subsampling, cores R1 and R3 were X-rayed and color line scanned using a ITRAX core scanner at Aarhus University. Samples for organic palynomorph and calcareous microfossil content analyses were taken as 1 cm-thick slices at a 2–8 cm interval. The samples were stored at −20°C before further handling.
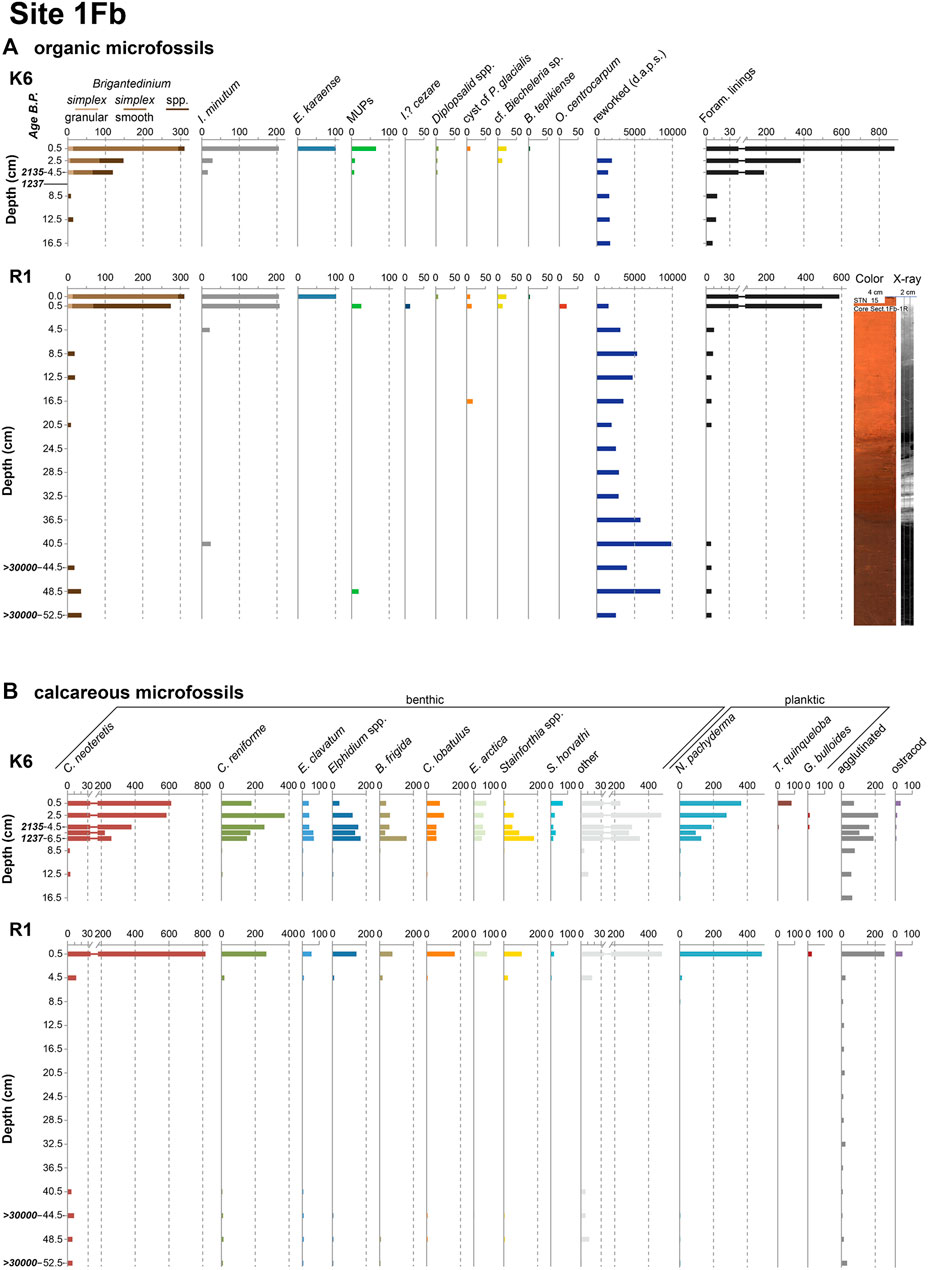
FIGURE 2. (A) Palynological and (B) foraminiferal and other calcareous microfossil concentrations (specimens per gram dry sediment) for cores K6 and R1, site 1Fb. The reworked palynomorphs include pre-Quaternary dinocysts, acritarchs, pollen, and spores (d.a.p.s.). Also shown are the color and X-ray scans of the longer core R1. All levels that were analyzed are indicated with a tick mark and a label on the depth axis, and as such also include barren samples. Calibrated radiocarbon ages are also indicated.
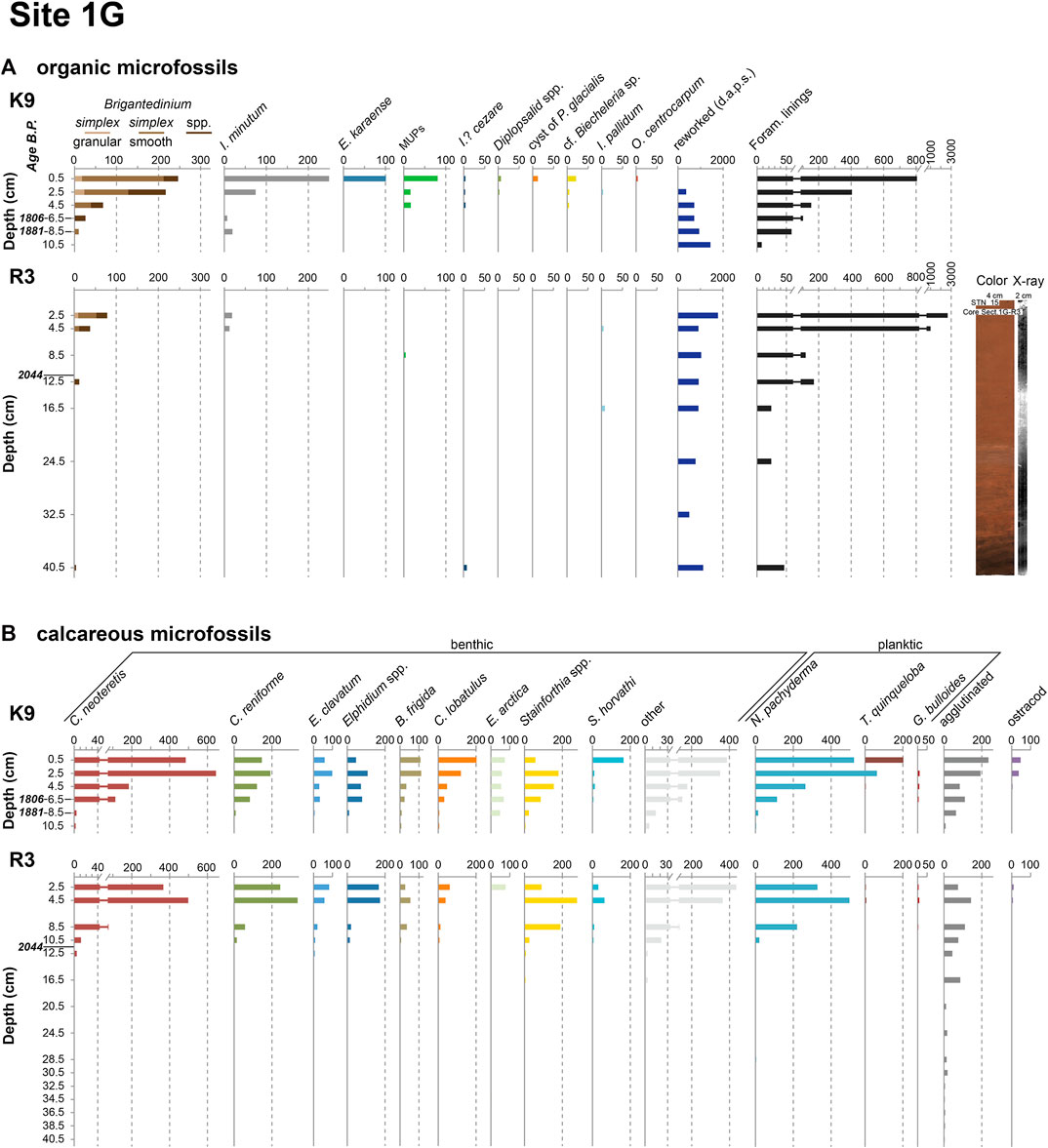
FIGURE 3. (A) Palynological and (B) foraminiferal and other calcareous microfossil concentrations (specimens per gram dry sediment) for cores K9 and R3, site 1G. The reworked palynomorphs include pre-Quaternary dinocysts, acritarchs, pollen, and spores (d.a.p.s.). Also shown are the color and X-ray scans of the longer core R3. All levels that were analyzed are indicated with a tick mark and a label on the depth axis, and as such also include barren samples. Calibrated radiocarbon ages are also indicated.
Palynological Analysis
Following freeze-drying, the samples were processed according to standard procedures at the Palynological, Micropalaeontological and Geobotanical laboratory at GEUS in Copenhagen. The organic fraction, including dinoflagellate cysts (dinocysts), pollen and spores, and various other palynomorphs, was isolated from the samples using cold HCl (2 N) and cold HF (40%) to dissolve the calcareous and siliceous fractions, respectively, and sieving through a 11 µm nylon mesh. A known number of Lycopodium clavatum spores were added as marker grains to each sample at the beginning of the chemical treatment to allow the calculation of absolute palynomorph concentrations (Stockmarr, 1971; Price et al., 2016; Lund University batch #1031, 20,848 spores/tablet). The residue was mounted between microscopy slide and coverslip using glycerin gelatin, and studied at 400× to 1,000× magnification with an Olympus BX41 upright light microscope using differential interference contrast optics.
Cysts with and without visible cell contents were counted separately but regrouped here for statistical treatment. Similarly, specimens were identified to the highest taxonomic rank possible following Fensome et al. (2019), but regrouped here for practical purposes and when unfavourable orientation or preservation hampered identification. This concerns notably Brigantedinium spp., which comprises brown spherical spineless cysts that could not be attributed unambiguously to Brigantedinium simplex, and diplopsalids spp., which encompasses closely similar spherical spineless cysts with a thin brown wall presumably produced by dinoflagellates belonging to the diplopsalioideans (e.g., Liu et al., 2017). In addition, other unspecified protoperidinian cysts and one single specimen attributable to the genus Lejeunecysta were grouped as “miscellaneous unspecified protoperidinians”. Cysts identified here as “cf. Biecheleria sp.” correspond to those previously recovered from the study area (Limoges et al., 2018) and elsewhere in the Arctic (e.g., Heikkilä et al., 2014), and closely resemble cysts of Biecheleria baltica known from the Baltic Sea (Kremp et al., 2005, as Woloszynskia halophila; Moestrup et al., 2009). Since the cyst-theca relationship of the arctic cysts remains unknown, the comparative identification “cf. Biecheleria sp.,” is also used here, following the recommendation by Limoges et al. (2020). Operculodinium centrocarpum as used here refers to the cyst morphotype sensu Wall and Dale (1966). Other identified and counted palynomorphs include organic foraminiferal linings, reworked pre-Quaternary dinocysts and acritarchs, and both fresh and reworked pollen and spores. The surface sample data for cores K6 and K9 are from Limoges et al. (2018).
Foraminiferal Analysis
The subsamples selected for foraminiferal census analysis were oven-dried at ∼50°C for all cores except K9, for which the samples had been freeze-dried. Both methods may negatively impact the preservation of agglutinated foraminifera, but have the advantage over wet samples that calculations of foraminiferal concentrations are more reliable. Based on our results, we have no reason to believe that freeze-drying introduced bias in our results compared to oven drying, since the agglutinated foraminifera dominantly occur fragmented in both the freeze-dried and oven-dried samples and show similar concentrations and trends in all cores (see Results). Once dry, the samples were weighed and wet-sieved over 63 and 100 µm mesh sieves, and the fractions were left to dry again in the oven at ∼50°C. The census analysis was done in the dry 63–100 and >100 µm fractions separately using a gridded picking tray. As such, the absolute abundance of taxa in each of the fractions, and thus also the total sample abundance, could be calculated. Planktic and benthic foraminifera were identified down to species level whenever possible. Specimens of Stainforthia concava, Stainforthia fusiformis and Stainforthia loeblichi were observed and distinguished in the >100 µm fraction, but were notably abundant in the 63–100 µm fraction. Since it can be hard to distinguish these species for such small specimens, they are grouped here as Stainforthia spp. Specimens of the genus Buccella were identified as Buccella frigida but may include rare specimens of closely similar Buccella hannai subsp. arctica. Agglutinated foraminifera (mostly fragments) and ostracod shells were also counted but not identified to genus or species level. The surface sample data for cores K6 and K9 are from Limoges et al. (2018).
Chronology
After census analysis of the calcareous fraction, benthic and planktic foraminifera were picked from the fraction for radiocarbon dating of selected samples (Table 1). This usually involved isolating the entire foraminiferal content from the sample because of the scarcity of calcareous microfossils in high arctic marine settings. The samples were dated at the Tandem Laboratory at Uppsala University, Sweden, which has particular expertize in dating low quantities of organic carbon. Accurate dates could be acquired in this way for the upper part of the cores, but in the deepest sections, where only 30–50 specimens could be isolated, the radiocarbon dates could only reveal that those particular samples have a minimum age of 30,000 years (Table 1), thus pre-dating the Late Weichselian and most likely deposited during (the second half of) the Middle Weichselian (ca. 70–27 ka BP; Mangerud, 2004). The dates from the upper core sections were calibrated with Calib 7.1 using the MARINE13 calibration curve (Reimer et al., 2013) and applying a total reservoir correction of 550 years (ΔR = 150 years) following Nørgaard-Pedersen et al. (2008).
In addition to the radiocarbon dates, 210Pb activity was measured at the Gamma Dating Center, Copenhagen University, in the upper 10 cm of cores K6 and K9, using 1 cm-thick samples. While we could confirm that the topmost samples of K6 and K9 are modern, the unsupported 210Pb activity proved to be very low with signs of mixing in the upper 5 cm and undetectable below 5 cm, and therefore, no 210Pb chronology was produced (see also Limoges et al., 2018).
Results
Since no 210Pb profiles could be established and only one or two levels in each core could be dated by accelerator mass spectrometry, it was not deemed opportune to construct an age-depth model for the four cores. Hence, the results from the microfossil analysis are shown against depth in Figures 2, 3, with indication of the age of the dated levels on the depth axis. Since low concentrations of microfossils in many samples did not allow counting the required number of specimens for statistically significant percentage calculations, the results of the down-core census analyses are given in absolute concentrations only.
Palynomorph Abundances
Highest dinocyst concentrations were found in the uppermost centimeters of all cores, with values fluctuating around 700 cysts g−1 dry sediment except for core R3 (site 1G) where concentrations are about one order of magnitude lower (Figures 2A, 3A). The assemblages in these samples are dominated by heterotrophic species, notably Brigantedinium cysts (mostly smooth-walled Brigantedinium simplex) and Islandinium minutum, with Echinidinium karaense as an important subordinate species. Accessory taxa consist of Islandinium? cezare, diplopsalids spp., and miscellaneous unspecified protoperidinians. The autotrophic component of the assemblages is made up by low but noticeable numbers of cf. Biecheleria sp. and cysts of Polarella glacialis, and the occasional occurrence of Operculodinium centrocarpum, Impagidinium pallidum and Bitectatodinium tepikiense. These assemblages persist through the first few centimeters of the cores, albeit with rapidly decreasing absolute concentrations, and the samples become quasi-barren below 5 cm core depth (i.e. roughly around 1,500–2,000 years BP). In the longer cores R1 and R3, the sections between ∼21 and 40 cm core depth are completely devoid of dinocysts. However, several specimens of Brigantedinium spp. and spiny brown cysts were recovered from the lowermost core sections pre-dating ∼30 ka BP.
The record of organic microforaminiferal linings shows a trend very similar to the dinocysts (Figures 2A, 3A): highest concentrations of 16,000–18,000 linings g−1 dry sediment (∼3,000 for R3) occur in the uppermost centimeters of the cores, but rapidly decrease downcore and reach near-zero levels around 5 cm core depth. These are completely absent between ∼21 and 40 cm in cores R1 and R3, but reappear together with the dinocysts in the lower sections of those cores. By contrast, reworked pre-Quaternary dinocysts and other reworked palynomorphs are present throughout all the analyzed cores.
Foraminiferal Abundances
Similar to the palynomorph abundances, foraminiferal test concentrations are highest in the uppermost few centimeters of the cores, varying between ∼1,300 and 2,200 tests g−1 dry sediment for the benthic foraminifera, and between ∼300 and 700 tests g−1 dry sediment for the planktic foraminifera (Figures 2B, 3B). The downcore decline of test concentrations is less steep than for the palynomorphs, in particular for core K6 (site 1Fb; Figure 2B) where total concentrations of over 1,400 calcareous tests g−1 persist down to ∼7 cm core depth. The assemblages in these uppermost few centimeters are dominated by Cassidulina neoteretis and Cassidulina reniforme, with Elphidium species (dominantly E. clavatum), Buccella frigida, Cibicides lobatulus, Epistominella arctica, Stetsonia horvathi and Stainforthia spp. (dominantly S. concava) as most frequent subordinate species. The latter taxon is particularly abundant at 8.5 and 10.5 cm depth in core R3 (site 1G), where it dominates the assemblages. It is worth mentioning that a significant number of C. lobatulus specimens look somewhat abraded, i.e., the outer edges of the tests and surface details appear variably rubbed out. A large number of other, rare species complete the assemblages (see Supplementary Material). The planktic assemblages are by far dominated by Neogloboquadrina pachyderma sinistral (sin), but low numbers of subpolar species (in particular Turborotalita quinqueloba and rare specimens of Globigerina bulloides) were also observed, in particular in the surface samples of K6 and K9. Note that occasional specimens of right-coiling Neogloboquadrina pachyderma were included with the left-coiling forms, since the right-coiling forms never made up more than 3% of the assemblages (cf. Darling et al., 2006).
Calcareous tests are very rare in all cores below 10 cm core depth, and they are completely absent from 20.5 cm down to the bottom of core R3 (site 1G), and between 8.5 and 36.5 cm in core R1 (site 1Fb) (Figures 2B, 3B). However, benthic foraminifera reappear in the four lowermost samples of core R1 covering 40.5–52.5 cm core depth, and planktic foraminifera in the three lowermost samples (44.5–52.5 cm), albeit in very low numbers (4–21 benthic tests g−1; 0.1–0.5 planktic tests g−1). All planktic specimens in these samples dating to 30 kyr ago or more belong to N. pachyderma (sin), while B. frigida, C. neoteretis, C. reniforme, E. clavatum, and Stainforthia spp. are the most frequent benthic species. A full list of all recovered species can be found in the Supplementary Material.
Fragments of agglutinated foraminifera are most abundant in the top few centimeters of the cores, but in contrast to the calcareous foraminifera, they also persist in low abundances all the way down to the bottom of all cores. Individual chambers of Reophax pilulifer occur down to 16.5 cm core depth at site 1G and down to 24.5 cm core depth at site 1Fb. Ostracod shells occur only in the upper ∼5 cm of the cores, where their abundances range from 1 to 45 individuals g−1 dry sediment (Figures 2B, 3B).
Discussion
Pre-Last Glacial Maximum Presence of Atlantic Waters
Data from within and offshore the Independence fjord system suggest that a relatively thin ice sheet extending onto the outer Wandel Sea shelf covered the area during the late Weichselian with a maximum extension during the LGM (Nørgaard-Pedersen et al., 2003, Nørgaard-Pedersen et al., 2008, and references therein) (Figure 4A). In line with recent data from the Northeast Greenland Ice Stream south of the Independence Fjord system, showing that the ice sheet margin likely was several tens of kilometers farther inland ∼41–26 kyr ago (Larsen et al., 2018), the radiocarbon ages of the benthic foraminifera recovered together with low numbers of dinocysts and planktic foraminifera from the bottom section of core R1 (Figure 2; Table 1; Supplementary Material) reveal that the ice sheet was not reaching the outer regions of the Independence Fjord system until at least 30 kyr ago. Instead, occasional intrusions of marine waters east of Prinsesse Dagmar Island occurred presumably during the second half of the Middle Weichselian. The dominance of C. neoteretis, C. reniforme and E. clavatum among the recovered specimens reveals the fingerprint of cooled subsurface Atlantic waters at the core site—similar to the latest Holocene assemblages albeit in much lower concentrations. This finds further support from marine mollusc shells recovered from Little Ice Age moraines around Station Nord and radiocarbon-dated to ∼52.7 to 43.1 ka BP (Larsen et al., 2019) (Figure 4A). Any further southward penetration of the marine waters along this pathway would then have been halted by glacial meltwater blocking and/or land exposed by the estimated 35–75 m sea-level drop at that time (e.g., Pico et al., 2016; Spratt and Lisiecki, 2016; Batchelor et al., 2019). While topography would not have made it impossible, the presently available data cannot provide insight into whether or not the marine waters also flowed to the west of Prinsesse Thyra Island and Prinsesse Dagmar Island at that time (Figure 4A).
The Greenland ice core stable oxygen isotope records show seven marked excursions indicative of higher atmospheric temperatures between ∼34 and 50 ka BP, corresponding to Greenland Interstadials (GI) 7 to 13 (Andersen et al., 2006; Svensson et al., 2008). Furthermore, abundant calcareous microfossils recovered from the Lomonosov Ridge and Morris Jesup Rise suggest intermittent inflow of North Atlantic waters through Fram Strait toward the central Arctic Ocean during intervals of the Middle Weichselian, notably between ∼34 and 50 ka BP, with the development of significant productive seasonal leads alternating with extended periods of severe, perennial sea-ice cover (Nørgaard-Pedersen et al., 1998; Spielhagen et al., 2004; Hanslik et al., 2010; Cronin et al., 2010). It is therefore tempting to associate the lower section of core R1, and the times of notable Atlantic water inflow north of Fram Strait, to one or more of these GIs. The pronounced and longer GI 12 (46.5 ka BP) in particular appears to be a probable candidate, as its timing furthermore fits best with the available radiocarbon dates and planktic foraminiferal abundance peaks from the Morris Jesup Rise and central Lomonosov Ridge (Nørgaard-Pedersen et al., 1998; Hanslik et al., 2010; Larsen et al., 2019). However, the low quantities of datable material only allow an approximate, minimum age of 30 kyr to be established for the lowermost section of core R1, implying that those sediments can have been deposited during any of GIs 7–13, and even GI 5 and 6 (32–34 ka BP), at times when Atlantic-sourced waters thus occasionally also seem to have reached into the Independence Fjord system.
Atlantic Water Inflow During the Holocene
The dominance of the infaunal foraminifer species C. neoteretis and C. reniforme in the calcareous assemblages is a feature that is typically observed in the fjord and shallow shelf regions of eastern Greenland (e.g., Jennings and Helgadottir, 1994). Both species thrive in cool (2°C or less) but fairly saline (30–35) waters, and are therefore generally associated with cooled subsurface Atlantic waters spreading over the shelf and into the fjords at depth (e.g., Hald and Vorren, 1987; Mackensen and Hald, 1988; Seidenkrantz, 1995; Polyak and Mikhailov, 1996; Hald and Korsun, 1997; Lubinski et al., 2001). Recently obtained conductivity-temperature-depth profiles confirm that saline waters of Atlantic origin make up the bottom waters below 140 m over the Wandel Sea shelf (Dmitrenko et al., 2017). However, it is unclear whether and to what degree these Atlantic waters are responsible for maintaining local benthic productivity, or rather promote the transport of tests to the inner Wandel Sea shelf from productive waters further offshore (cf. Limoges et al., 2018). On the one hand, the abraded appearance of several specimens of the epibenthic species C. lobatulus might indeed indicate that these specimens have been transported, or at least subjected to strong bottom currents, the typically preferred conditions of this species (e.g., Wollenburg and Kuhnt, 2000). As noted earlier (Nørgaard-Pedersen et al., 2008; Limoges et al., 2018), the planktic foraminiferal tests recovered from the core sites were also likely advected rather than produced locally. Although N. pachyderma (sin) appears to calcify and spend most of its time in the upper ∼100 m of the water column—particularly in the seasonally ice-covered regions of western Fram Strait (Volkmann, 2000; Schiebel and Hemleben, 2005; Pados and Spielhagen, 2014; Pados et al., 2015)—it is rare in shallow shelf areas and, perhaps more importantly, its distribution is strongly controlled by food availability (Pados and Spielhagen, 2014). In fact, low concentrations of their primary food source, diatoms (Volkmann, 2000; Hemer et al., 2007), were found in the surface sediments of sites 1Fb and 1G (Limoges et al., 2018). While we have no downcore diatom data, the virtual absence of phototrophic dinocyst species argues against microalgal productivity being sufficient to wholly sustain a local planktic foraminiferal community. On the other hand, oligotrophic benthic species such as S. horvathi (Wollenburg and Kuhnt, 2000) depend less on a sustained phytodetritus transfer and might indeed be/have been living at sites 1Fb and 1G, and the good preservation of the abundant Cassidulina specimens argues against them being transported. Local benthic productivity is further suggested by the downcore offset between the dinocyst and foraminiferal trends, which implies active burying on-site by the benthic foraminifera (see Recent and Holocene Local Productivity). Thus, the above suggests that the calcareous communities are partly allochthonous, partly autochthonous, brought into the area and sustained by subsurface Atlantic waters reaching the study area.
While the modern presence of Atlantic bottom waters is evident, it is unclear for how long these have been reaching the inner Wandel Sea shelf. At face value, the few available radiocarbon dates in the upper sections of the cores suggest that the inflow has been occurring since at least ∼2,000 years. However, this is likely an underestimate, since the evident mixing in the upper few centimeters of the cores—well illustrated by the downcore age reversal of the radiocarbon dates in core K6 (Table 1; Figure 2)—implies that those ages result from a mixture of younger and older tests. The presence of agglutinated tests of R. pilulifer beyond the levels with (enough datable) calcareous tests would further suggest that Atlantic-sourced waters (Korsun et al., 1998) also reached the core sites before 2,000 years BP, albeit probably much less frequently and/or strongly (Figure 4). Reworked shells of the marine mollusc Astarte borealis collected from upthrusted marine sediments near Station Nord were dated between ∼4.2 and ∼8.7 ka BP (Larsen et al., 2019), noting however that this species can also thrive under much reduced salinities (e.g., Zettler, 2002 and references therein) and thus not necessarily indicates the presence of Atlantic waters. Reophax pilulifer was also recovered from the assumed Holocene 0.5 m of reddish brown marine mud at Site B from Nørgaard-Pedersen et al. (2008), south of our core sites (Figure 1). However, these marine muds were devoid of any calcareous fossils and therefore cannot give further indications about the absolute timing of Atlantic water inflow east of Prinsesse Dagmar Island. The Holocene age of the muds is implied from their position on top of a thick diamicton and through correlation with Holocene sediments west of Prinsesse Dagmar Island, to the southwest of our core sites (Site E from Nørgaard-Pedersen et al., 2008; Figure 1). There, abundant calcareous foraminiferal tests were recovered from a thin horizon at the top of the core (1.0–1.1 ka BP) and a thick section representing the early Holocene (8.9–10.0 ka BP), indicating the presence of subsurface Atlantic waters west of Prinsesse Dagmar Island during those times (Figures 4B,D). The fact that calcareous foraminifera are present during the early Holocene west of Prinsesse Dagmar Island (Site E) but not to the east (Sites 1G, 1Fb, and B) is likely to be attributed to the combined effect of the topography of the fjord system and the earlier (?) deglaciation of its northern part, forcing inflowing Atlantic waters to follow the deeper parts of the fjord off southeast Peary Land (cf. Nørgaard-Pedersen et al., 2008) and then southward to the west of the Prinsesse Thyra and Prinsesse Dagmar islands toward and across the then-submerged northernmost parts of Finderup Land (Strunk et al., 2018) (Figure 4B). Indeed, radiocarbon dates from raised marine deposits on southern and southeastern Peary Land indicate that glaciers had retreated from this area by 9.7–10.2 ka BP (Bennike, 1987; Bennike & Björck, 2002), when outlet glaciers from the Greenland Ice Sheet might still have been over or near the area off northwestern Kronprins Christian Land, much like the outlet glaciers from the Flade Isblink ice cap are today. Thus, the occasional presence of calcareous tests during the early Holocene (west) and during at least the last 2,000 years or so (east and west of Prinsesse Dagmar Island), and the complete lack of levels clearly datable to the mid Holocene at all sites, would suggest that Atlantic water inflow was patchy during the early and late Holocene and strongly suppressed or absent otherwise. The early and late Holocene intervals of evidenced Atlantic water advection into the Independence Fjord system fall within periods of generally strengthened flow of Atlantic water through Fram Strait into the southern Arctic basin (Werner et al., 2013).
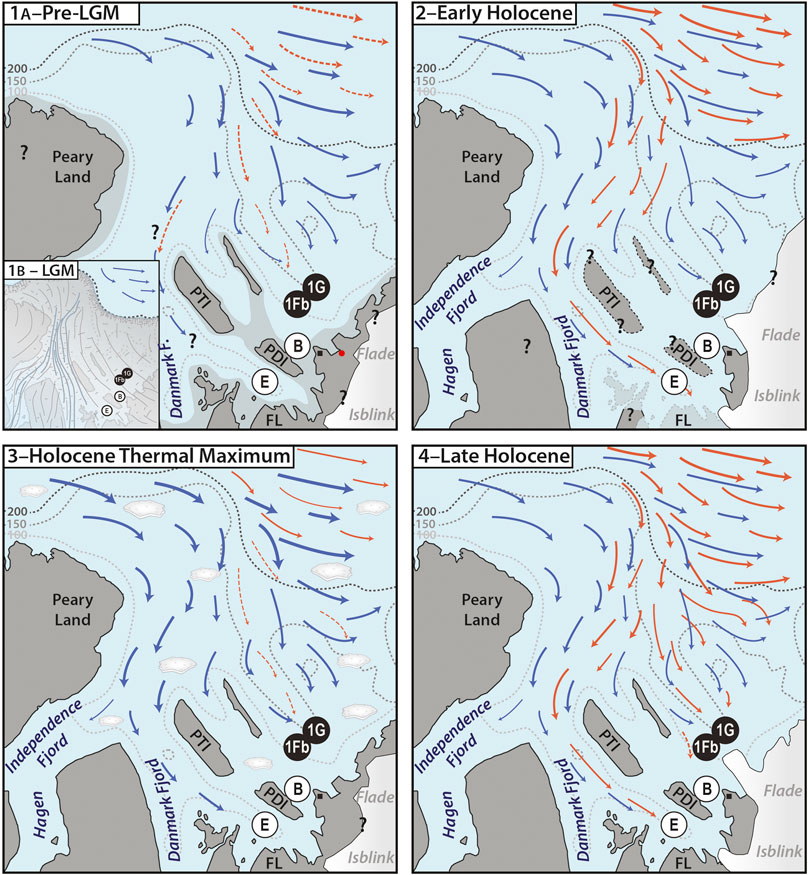
FIGURE 4. Schematic representation of water inflow into the Independence Fjord system during the past ca. 50 kyr as discussed in the text. The dotted gray lines represent the present-day 100, 150, and 200 m isobaths based on Nørgaard-Pedersen et al. (2008) and Dmitrenko et al. (2017)–note that these are to a large extent approximate since much of the area has not been covered by detailed bathymetric surveys. Orange arrows indicate Atlantic-sourced subsurface waters, blue arrows indicate polar surface waters, striped lines suggest occasional, intermittent inflow only. The black circles show the position of sites 1Fb and 1G studied here, the white circles show the position of sites E and B from Nørgaard-Pedersen et al. (2008), the black square shows the location of Station Nord. PDI = Prinsesse Dagmar Island, PTI = Prinsesse Thyra Island, and FL = Finderup Land. The question marks in the scenarios relay the fact that much still remains unknown about the latest Pleistocene–Holocene history of the area. (1A–Pre-LGM) Low but indicative numbers of dinocysts and/or calcareous benthic foraminifera, the latter having a radiocarbon age of at least 30 kyr, in the bottom sections of long cores from sites 1Fb and 1G, and reworked marine mollusc shells radiocarbon-dated to ∼52.7 to 43.1 ka BP recovered from a location (red dot) near one of the modern-day Flade Isblink outlet glaciers (Larsen et al., 2019), suggest episodic inflow of Atlantic-sourced waters to the northeast of PDI at one or several occasions during the Middle Weichselian. There is no information available about whether or not these waters also flowed to the west of PTI and PDI. The light-shaded gray suggests an approximate outline of exposed terrain during the Middle Weichselian based on the present-day bathymetry and a ca. 75 m sea-level drop (e.g., Spratt & Lisiecki, 2016), but Middle Weichselian global sea level might have dropped 30–40 m less than that (Pico et al., 2016; Batchelor et al., 2019). (1B–LGM, inset) The Independence Fjord system is covered by the Greenland Ice Sheet extending onto the outer Wandel Sea shelf. (2–Early Holocene) Steered by topography and the possibly slightly earlier deglaciation of the western fjord, Atlantic-sourced waters penetrate between Peary Land and PTI toward site E (Nørgaard-Pedersen et al., 2008) and across isostatically depressed FL (Strunk et al., 2018; Larsen et al., 2019). There is no evidence for Atlantic-sourced waters also reaching sites B, 1Fb and 1G during the early Holocene. (3–Holocene Thermal Maximum) Despite reduced ice cover along the east coast of Greenland (Funder et al., 2011b), inflow of Atlantic-sourced waters, if any at all, is intermittent and strongly suppressed. Under a positive Arctic Oscillation with strengthened and eastward-shifted Transpolar Drift, the water column at the fjord mouth might have been flooded by polar waters, furthermore bringing along ice floes from the Arctic that can freely drift into the fjord system and hamper primary production. Note that ice floes are shown in this cartoon only to emphasize their suggested increased presence and effect during the Holocene Thermal Maximum with respect to the other time intervals. (4–Late Holocene) Atlantic-sourced waters make up the subsurface water column in the deeper parts of the Independence Fjord system including sites E, 1G and 1Fb in modern times. The inflow appears to have started at least 2,000 years ago and gradually increased over that time, on occasion also influencing site B.
Recent and Holocene Local Productivity
While both the foraminiferal and dinocyst abundances show a rapid down-core decline, the foraminifera appear to maintain somewhat higher concentrations further down-core, in particular in the short “kayak cores” (K6 and K9; Figures 2, 3). This slight offset is likely explained by the active epifaunal to infaunal behavior of the benthic foraminifera burying into the sediment, in contrast to the dinocysts, which are passively mixed down the sediment. Thus, both the dinocysts and calcareous foraminifera can be assumed to represent approximately the same period of Atlantic water inflow. As with the foraminifera, the extent to which the dinocyst assemblages were either locally produced or advected is hard to determine. Local production would seem more likely to have occurred in more recent times, represented by the upper few centimeters of the cores with highest cyst abundances, including cysts with cell content (notably Brigantedinium spp., diplopsalids spp., E. karaense, cf. Biecheleria sp., and cysts of P. glacialis). The limited historical data available reveals that open water leads near the core sites–required for local primary production–were first observed in 1978 (through aerial photography; Higgins, 1991) and followed a period of permanent sea-ice cover spanning (at least) from 1950 to 1964, as documented by the Danish Meteorological Institute (Rysgaard et al., 2003). Satellite data further reveal that the glacial front of the outlet glacier south of the core site has retreated by about 13 km between 1991 and 2015 (Nørgaard-Pedersen et al., 2016), which might have relieved some of the pressure caused by the ice mélange and calving in front of the outlet glacier (Figure 1C), facilitating the seasonal formation of leads at the core sites, and thus increased productivity.
Taken at face value, the low concentrations in the downcore sections would seem to suggest that extensive, perennial sea-ice cover drastically limited primary production in the area prior to the mid-20th century, when benthic life must have been sustained mainly by subsurface outer-shelf water advection and, perhaps, the occasional occurrence of open water. In this respect, it is surprising that the records reveal no indications for enhanced local primary productivity during the Holocene Thermal Maximum, when warmer-than-present conditions are assumed to have resulted in seasonally open, sea ice free waters (Funder et al., 2011b) and glaciers were at or behind their present front (Nørgaard-Pedersen et al., 2008). There is no apparent mismatch between the number of foraminiferal tests and the organic linings that remain when the calcareous tests dissolve (Figures 2, 3), and thus dissolution is an unlikely explanation for the low fossil content. Either very slow sedimentation rates due to the halted influx of meltwater-derived sediments after 9 ka BP precluded the preservation of organic remains during that time (cf. Nørgaard-Pedersen et al., 2008), or conditions in the area were less favorable than suggested by the mere presence of local beach ridges and driftwood deposits (Funder et al., 2011a; Funder et al., 2011b). Indeed, while reduced seasonal ice cover characterized much of the Greenland coast during the Holocene Thermal Maximum, the driftwood as well as biogenic data also indicate a strong and westward-shifted Transpolar Drift due to a positive Arctic Oscillation index at that time (Funder et al., 2011b; Georgiadis et al., 2020). The consequent increased export of polar waters through Fram Strait might have suppressed the flow of Atlantic Waters to, and as such protist community growth in, the Independence Fjord system (Figure 4C). Furthermore, the reduced ice cover during the Holocene Thermal Maximum along the eastern Greenland coast might have facilitated a sustained inflow of drift ice deeper into the fjord during the summer months, advected through Fram Strait by the strengthened Transpolar Drift. This is in contrast with the present situation where multi-year ice largely blocks the entrance of the Fjord System for drift ice and thus allows the development of open water leads off Finderup Land and Prinsesse Ingeborg Halvø (Figure 1C).
Conclusion
Microfossil analysis of four sediment cores recovered through the sea ice at the mouth of the Independence Fjord system near Station Nord revealed that predominantly harsh conditions caused by heavy ice cover and/or polar water inflow, as suggested by quasi barren samples, are punctuated by three phases of recorded Atlantic-sourced water inflow into the fjord system during the Holocene and Last Glacial Period:
• Low but indicative numbers of organic and calcareous microfossils dated to at least 30,000 BP reveal the existence of a pathway for the intrusion of Atlantic waters during the second half of the Middle Weichselian, implying a local ice cap prior to the LGM with an extent similar to or smaller than at present.
• In contrast to previous studies, our sediment records show no indications of Atlantic water inflow during the early Holocene, implying that any inflow into the fjord system at that time was restricted to the western part of the Independence Fjord system, likely controlled by the local topography and deglacial history. Atlantic inflow appears to have been non-existent or strongly suppressed during the mid-Holocene.
• The modern-day presence in the Independence Fjord system of cooled subsurface waters of Atlantic origin is a feature that appears to have started at least ∼2,000 years ago, with protist diversity and productivity—either local and/or offshore on and along the Wandel Sea shelf—increasing toward recent times likely as a result of changes in sea-ice cover linked to modern warming.
Data Availability Statement
All datasets presented in this study are included in the article/Supplementary Material.
Author Contributions
NV: data production and analysis; writing—original draft; visualization. AL: data analysis; writing—editing; visualization. NN: sampling; data analysis. MS: writing—editing; resources. SR: data analysis; writing—editing; resources.
Funding
This study received financial support from the Villum Foundation, Denmark (grant no. VKR023454 to SR). Fieldwork was in part also funded by the Arctic Research Center, Aarhus University. MS was funded by the Danish Council for Independent Research (grant no. 7014-00113B/FNU; G-Ice project). AL received funding from NSERC-DG (2018-03984).
Conflict of Interest
The authors declare that the research was conducted in the absence of any commercial or financial relationships that could be construed as a potential conflict of interest.
Acknowledgments
The Department of Environmental Science, the Arctic Research Center, Aarhus University, and the staff of the Villum Research Station are acknowledged for providing logistics. We thank Kunuk Lennert, Jesper Hofmann, and Egon R. Frandsen for outstanding technical and logistical assistance in the field. We also appreciate the logistical support from the Station Nord Danish military personnel. We thank Jan Hennissen and Mingrui Qiang for their constructive reviews. This work is a contribution to the Arctic Science Partnership (ASP).
Supplementary Material
The Supplementary Material for this article can be found online at: https://www.frontiersin.org/articles/10.3389/feart.2020.565670/full#supplementary-material
References
Amdrup, G. C. (1912–1917). Danmark-ekspeditionen til Grønlands Nordøstkyst 1906–1908 under ledelse af L. Mylius Erichsen. Meddelelser om Grønland. 41–46. Copenhagen, DK: Bianco lunos Bogtrykkeri.
Amdrup, G. C. (1913). Mylius-Erichsen's report on the non-existence of the Peary Channel; information brought home by Ejnar Mikkelsen. Meddelser om Grønland 41-5.
Andersen, K. K., Svensson, A., Johnsen, S. J., Rasmussen, S. O., Bigler, M., and Röthlisberger, R. (2006). The greenland ice core chronology 2005, 15–42 ka. Part 1: constructing the time scale. Quat. Sci. Rev. 25, 3246–3257. doi:10.1016/j.quascirev.2006.08.002
Batchelor, C. L., Margold, M., Krapp, M., Murton, D. K., Dalton, A. S., and Gibbard, P. L. (2019). The configuration of Northern Hemisphere ice sheets through the quaternary. Nat. Commun. 10, 3713. doi:10.1038/s41467-019-11601-2
Bendtsen, J., Mortensen, J., Lennert, K., Ehn, J. K., Boone, W., and Galindo, V. (2017). Sea ice breakup and marine melt of a retreating tidewater outlet glacier in northeast Greenland (81°N). Sci. Rep. 7, 4941. doi:10.1038/s41598-017-05089-3
Bennike, O. (1987). Quaternary geology and biology of the Jørgen Brønlund fjord area, north Greenland. Meddelelser Grønland Geosci. 18, 23.
Bennike, O., and Björck, S. (2002). Chronology of the last recession of the Greenland ice sheet. J. Quat. Sci. 17, 211–219. doi:10.1002/jqs.670
Clark, P. U., and Mix, A. C. (2002). Ice sheets and sea level of the last glacial maximum. Quat. Sci. Rev. 21, 1–7. doi:10.1016/S0277-3791(01)00118-4
Cronin, T. M., Gemery, L., Briggs, W. M., Jr., Jakobsson, M., Polyak, L., and Brouwers, E. M. (2010). Quaternary sea-ice history in the Arctic Ocean based on a new Ostracode sea-ice proxy. Quat. Sci. Rev. 29, 3415–3429. doi:10.1016/j.quascirev.2010.05.024
Darling, K. F., Kucera, M., Kroon, D., and Wade, C. M. (2006). A resolution for the coiling direction paradox in Neogloboquadrina pachyderma. Paleoceanography 21, 3415–3429. doi:10.1029/2005pa001189
Dmitrenko, I. A., Kirillov, S. A., Rudels, B., Babb, D. G., Pedersen, L. T., and Rysgaard, S. (2017). Arctic Ocean outflow and glacier-ocean interactions modify water over the Wandel Sea shelf (northeastern Greenland). Ocean Sci. 13, 1045–1060. doi:10.5194/os-13-1045-2017
Fensome, R. A., Williams, G. L., and MacRae, R. A. (2019). The Lentin and Williams Index of fossil dinoflagellates 2019 edition. AASP Contributions Ser. 50, 1–1173.
Funder, S., Goosse, H., Jepsen, H., Kaas, E., Kjaer, K. H., and Korsgaard, N. J. (2011a). A 10,000-year record of Arctic Ocean sea-ice variability—view from the beach. Science 333, 747–750. doi:10.1126/science.1202760
Funder, S., Kjeldsen, K. K., Kjær, K. H., and Ó Cofaigh, C. (2011b). “The Greenland ice sheet during the past 300,000 years: a review”, in Quaternary glaciations–extent and chronology–a closer look. Developments in quaternary Sciences. Editors J. Ehlers, P. L. Gibbard, and P. D. Hughes (Amsterdam, Netherlands: Elsevier), Vol. 15, 699–714.
Georgiadis, E., Giraudeau, J., Jennings, A., Limoges, A., Jackson, R., and Ribeiro, S. (2020). Local and regional controls on Holocene sea ice dynamics and oceanography in Nares Strait, Northwest Greenland. Mar. Geol. 422, 106115. doi:10.1016/j.margeo.2020.106115
Grønnow, B. (2016). “Independence I and saqqaq: the first Greenlanders”, in The Oxford handbook of the prehistoric arctic. Editors M. Friesen and O. Mason (New York, NY: Oxford University Press). doi:10.1093/oxfordhb/9780199766956.013.33
Grønnow, B., and Sørensen, M. (2006). “Palaeo-eskimo migrations into Greenland: the Canadian connection,” in Proceedings of the SILA/NABO conference on arctic and north atlantic Archaeology, Copenhagen, Denmark, May 10–14, 2004. Editor B. Grønnow (PNM, National Museum), Vol. 10, 59–74.
Hald, M., and Korsun, S. (1997). Distribution of modern benthic foraminifera from fjords of Svalbard, European Arctic. J. Foramin. Res. 27, 101–122. doi:10.2113/gsjfr.27.2.101
Hald, M., and Vorren, T. O. (1987). Foraminiferal stratigraphy and environment of late Weichselian deposits on the continental shelf off Troms, northern Norway. Mar. Micropaleontol. 12, 129–160. doi:10.1016/0377-8398(87)90018-1
Hanslik, D., Jakobsson, M., Backman, J., Björck, S., Sellén, E., and O’Regan, M. (2010). Quaternary Arctic Ocean sea ice variations and radiocarbon reservoir age corrections. Quat. Sci. Rev. 29, 3430–3441. doi:10.1016/j.quascirev.2010.06.011
Heikkilä, M., Pospelova, V., Hochheim, K. P., Kuzyk, Z. Z. A., Stern, G. A., and Barber, D. G. (2014). Surface sediment dinoflagellate cysts from the Hudson Bay system and their relation to freshwater and nutrient cycling. Mar. Micropaleontol. 106, 79–109. doi:10.1016/j.marmicro.2013.12.002
Hemer, M. A., Post, A. L., O'Brien, P. E., Craven, M., Truswell, E. M., and Roberts, D. (2007). Sedimentological signatures of the sub-Amery Ice Shelf circulation. Antarct Sci. 19, 497–506. doi:10.1017/S0954102007000697
Higgins, A. K. (1991). North Greenland glacier velocities and calf ice production. Polarforschung 60, 1–23.
Jennings, A. E., and Helgadottir, G. (1994). Foraminiferal assemblages from the fjords and shelf of eastern Greenland. J. Foramin. Res. 24, 123–144. doi:10.2113/gsjfr.24.2.123
Jensen, J. F. (2016). “Greenlandic Dorset,” in The Oxford handbook of the prehistoric arctic. Editors M. Friesen and O. Mason (New York, NY: Oxford University Press) doi: 10.1093/oxfordhb/9780199766956.013.56
Kirillov, S., Dmitrenko, I., Rysgaard, S., Babb, D., Toudal Pedersen, L., and Ehn, J. (2017). Storm-induced water dynamics and thermohaline structure at the tidewater Flade Isblink Glacier outlet to the Wandel Sea (NE Greenland). Ocean Sci. 13, 947–959. doi:10.5194/os-13-947-2017
Koch, L. (1928). Contributions to the glaciology of North Greenland. Meddelelser om Grønland 65, 181–464.
Korsun, S., Hald, M., Panteleeva, N., Tarasov, G., and Båmstedt, U. (1998). Biomass of foraminifera in the st. Anna trough, Russian arctic continental margin. Sarsia 83, 419–431. doi:10.1080/00364827.1998.10413701
Kremp, A., Elbrächter, M., Schweikert, M., Wolny, J. L., and Gottschling, M. (2005). Woloszynskia halophila (Biecheler) comb. nov.: a bloom-forming cold-water dinoflagellate co-occurring with Scrippsiella hangoei (dinophyceae) in the Baltic Sea. J. Phycol. 41, 629–642. doi:10.1111/j.1529-8817.2005.00070.x
Larsen, N. K., Levy, L. B., Carlson, A. E., Buizert, C., Olsen, J., and Strunk, A. (2018). Instability of the northeast Greenland ice Stream over the last 45,000 years. Nat. Commun. 9, 1872. doi:10.1038/s41467-018-0431207
Larsen, N. K., Levy, L. B., Strunk, A., Søndergaard, A. S., Olsen, J., and Lauridsen, T. L. (2019). Local ice caps in Finderup land, north Greenland, survived the Holocene thermal maximum. Boreas 48, 551–562. doi:10.1111/bor.12384
Limoges, A., Ribeiro, S., Weckström, K., Heikkilä, M., Zamelczyk, K., and Andersen, T. J. (2018). Linking the modern distribution of biogenic proxies in high Arctic Greenland shelf sediments to sea ice, primary production, and Arctic-Atlantic inflow. J. Geophys. Res. 123, 760–786. doi:10.1002/2017JG003840
Limoges, A., Van Nieuwenhove, N., Head, M. J., Mertens, K. N., Pospelova, V., and Rochon, A. (Forthcoming 2020). A review of rare and less well known extant marine organic-walled dinoflagellate cyst taxa of the orders Gonyaulacales and Suessiales from the Northern Hemisphere. Mar. Micropaleontol. doi: 10.1016/j.marmicro.2019.101801
Liu, T., Mertens, K. N., and Gu, H. (2017). Cyst-theca relationship and phylogenetic positions of the diplopsalioideans (Peridiniales, Dinophyceae), with description of Niea and Qia gen. nov. Phycologia 54, 210–232, doi: 10.2216/14-94.1
Lubinski, D. J., Polyak, L., and Forman, S. L. (2001). Freshwater and Atlantic water inflows to the deep northern Barents and Kara seas since ca 13 14 Cka:. Quat. Sci. Rev. 20, 1851–1879. doi:10.1016/s0277-3791(01)00016-6
Mackensen, A., and Hald, M. (1988). Cassidulina teretis Tappan and C. laevigata d'Orbigny; their modern and late quaternary distribution in northern seas. J. Foramin. Res. 18, 16–24. doi:10.2113/gsjfr.18.1.16
Mangerud, J. (2004). “Ice sheet limits in Norway and on the Norwegian continental shelf,” in Developments in quaternary sciences Vol. 2, Quaternary glaciations extent and chronology Part I: Europe. Editors J. Ehlers and P. L. Gibbard, 271–294 (Elsevier B.V.). doi:10.1016/S1571-0866(04)80078-2
Moestrup, Ø., Lindberg, K., and Daugbjerg, N. (2009). Studies on woloszynskioid dinoflagellates IV: the genus Biecheleria gen. nov. Phycol. Res. 57, 203–220. doi:10.1111/j.1440-1835.2009.00540.x
Nørgaard-Pedersen, N., Mikkelsen, N., and Kristoffersen, Y. (2008). Late glacial and Holocene marine records from the independence fjord and Wandel Sea regions, north Greenland. Polar Res. 27, 209–221. doi:10.1111/j.1751-8369.2008.00065.x
Nørgaard-Pedersen, N., Ribeiro, S., Mikkelsen, N., Limoges, A., and Seidenkrantz, M.-S. (2016). Investigations of past climate and sea-ice variability in the fjord area by Station Nord, eastern North Greenland. Geol Surv Den Greenl 35, 67–70.
Nørgaard-Pedersen, N., Spielhagen, R. F., Erlenkeuser, H., Grootes, P. M., Heinemeier, J., and Knies, J. (2003). Arctic Ocean during the Last Glacial Maximum: Atlantic and polar domains of surface water mass distribution and ice cover. Paleoceanography 28, 1063. doi:10.1029/2002PA000781
Nørgaard-Pedersen, N., Spielhagen, R. F., Thiede, J., and Kassen, H. (1998). Central Arctic surface ocean environment during the past 80,000 years. Paleoceanography 13, 193–204. doi:10.1029/97pa03409
Pados, T., Spielhagen, R. F., Bauch, D., Meyer, H., and Segl, M. (2015). Oxygen and carbon isotope composition of modern planktic foraminifera and near-surface waters in the Fram Strait (Arctic Ocean)–a case study. Biogeosciences 12, 1733–1752. doi:10.5194/bg-12-1733-2015
Pados, T., and Spielhagen, R. F. (2014). Species distribution and depth habitat of recent planktic foraminifera in Fram Strait, Arctic Ocean. Polar Res. 33, 22483. doi:10.3402/polar.v33.22483
Pico, T., Mitrovica, J. X., Ferrier, K. L., and Braun, J. (2016). Global ice volume during MIS 3 inferred from a sea-level analysis of sedimentary core records in the Yellow River Delta. Quat. Sci. Rev. 152, 72–79. doi:10.1016/j.quascirev.2016.09.012
Polyak, L., and Mikhailov, V. (1996). “Post-glacial environments of the southeastern Barents Sea: foraminiferal evidence,” in Late quaternary palaeoceanography of the north Atlantic margins. Editors J. T. Andrews, W. E. N. Austin, H. Bergsten, and A. E. Jennings (Geological Society Special Publication), 323–337.
Price, A. M., Gurdebeke, P. R., Mertens, K. N., and Pospelova, V. (2016). Determining the absolute abundance of dinoflagellate cysts in recent marine sediments III: identifying the source of Lycopodium loss during palynological processing and further testing of the Lycopodium marker-grain method. Rev. Palaeobot. Palynol. 226, 78–90. doi:10.1016/j.revpalbo.2015.12.009
Reimer, P. J., Bard, E., Bayliss, A., Beck, J. W., Blackwell, P. G., and Ramsey, C. B. (2013). IntCal13 and Marine13 radiocarbon age calibration curves 0–50,000 Years cal BP. Radiocarbon 55, 1869–1887. doi:10.2458/azu_js_rc.55.16947
Ryan, W. B. F., Carbotte, S. M., Coplan, J. O., O'Hara, S., Melkonian, A., and Arko, R. (2009). Global multi-resolution topography synthesis. Geochem. Geophys. Geosyst. 10, 1–9. doi:10.1029/2008GC002332
Rysgaard, S., Vang, T., Stjernholm, M., Rasmussen, B., Windelin, A., and Kiilsholm, S. (2003). Physical conditions, carbon transport, and climate change impacts in a northeast Greenland fjord. Arctic Antarct. Alpine Res. 35, 301–312. doi:10.1657/1523-0430(2003)035[0301:pcctac]2.0.co;2
Schiebel, R., and Hemleben, C. (2005). Modern planktic foraminifera. Paläontol. Z. 79, 135–148. doi:10.1007/bf03021758
Seidenkrantz, M.-S. (1995). Cassidulina teretis Tappan and Cassidulina neoteretis new species (Foraminifera): stratigraphic markers for deep sea and outer shelf areas. J. Micropalaeontol. 14, 145–157. doi:10.1144/jm.14.2.145
Spielhagen, R., Baumann, K.-H., Erlenkeuser, H., Nowaczyk, N. R., Nørgaard-Pedersen, N., and Vogt, C. (2004). Arctic Ocean deep-sea record of northern Eurasian ice sheet history. Quat. Sci. Rev. 23, 1455–1483. doi:10.1016/j.quascirev.2003.12.015
Spratt, R. M., and Lisiecki, L. E. (2016). A Late Pleistocene sea level stack. Clim. Past 12, 1079–1092. doi:10.5194/cp-12-1079-2016
Stockmarr, J. (1971). Tablets with spores used in absolute pollen analysis. Pollen Spores 13, 616–621.
Strunk, A., Larsen, N. K., Nilsson, A., Seidenkrantz, M.-S., Levy, L. B., and Olsen, J. (2018). Relative sea-level changes and ice sheet history in Finderup Land, North Greenland. Front. Earth Sci. 6, 129. doi:10.3389/feart.2018.00129
Svensson, A., Andersen, K. K., Bigler, M., Clausen, H. B., Dahl-Jensen, D., and Davies, S. M. (2008). A 60 000 year Greenland stratigraphic ice core chronology. Clim. Past 4, 47–57. doi:10.5194/cp-4-47-2008
Volkmann, R. (2000). Planktic foraminifers in the outer Laptev sea and the Fram Strait--Modern distribution and ecology. J. Foramin. Res. 30, 157–176. doi:10.2113/0300157
Wall, D., and Dale, B. (1966). "Living fossils" in western Atlantic Plankton. Nature 211, 1025–1026. doi:10.1038/2111025a0
Werner, K., Spielhagen, R. F., Bauch, D., Hass, H. C., and Kandiano, E. (2013). Atlantic Water advection versus sea-ice advances in the eastern Fram Strait during the last 9 ka: multiproxy evidence for a two-phase Holocene. Paleoceanography 28, 283–313. doi:10.1002/palo.20028
Wollenburg, J. E., and Kuhnt, W. (2000). The response of benthic foraminifers to carbon flux and primary production in the Arctic Ocean. Mar. Micropaleontol. 40, 189–231. doi:10.1016/s0377-8398(00)00039-6
Keywords: Holocene, high arctic fjord, Greenland Ice Sheet, dinoflagellate cysts, foraminifera, Atlantic water, Weichselian
Citation: Van Nieuwenhove N, Limoges A, Nørgaard-Pedersen N, Seidenkrantz M-S and Ribeiro S (2020) Episodic Atlantic Water Inflow Into the Independence Fjord System (Eastern North Greenland) During the Holocene and Last Glacial Period. Front. Earth Sci. 8:565670. doi:10.3389/feart.2020.565670
Received: 25 May 2020; Accepted: 25 August 2020;
Published: 15 September 2020.
Edited by:
Daniel Nývlt, Masaryk University, CzechiaReviewed by:
Jan Alfons Irma Hennissen, British Geological Survey (BGS), United KingdomMingrui Qiang, South China Normal University, China
Copyright © 2020 Van Nieuwenhove, Limoges, Nørgaard-Pedersen, Seidenkrantz and Ribeiro. This is an open-access article distributed under the terms of the Creative Commons Attribution License (CC BY). The use, distribution or reproduction in other forums is permitted, provided the original author(s) and the copyright owner(s) are credited and that the original publication in this journal is cited, in accordance with accepted academic practice. No use, distribution or reproduction is permitted which does not comply with these terms.
*Correspondence: Sofia Ribeiro, sri@geus.dk