- 1Department of Marine Science, University of Otago, Dunedin, New Zealand
- 2Department of Microbiology, University of Otago, Dunedin, New Zealand
- 3NIWA/University of Otago Research Centre for Oceanography, Dunedin, New Zealand
- 4Department of Functional and Evolutionary Ecology, University of Vienna, Vienna, Austria
- 5Department of Marine Microbiology and Biogeochemistry, NIOZ, Royal Netherlands Institute for Sea Research, Utrecht University, Utrecht, Netherlands
Marine microbes use extracellular phosphatases to hydrolyze phosphate from organic matter. Dissolved organic phosphorus (DOP) is typically present in higher concentrations than phosphate in oceanic surface waters. Yet, the fate and role of different DOP components, such as phosphomonoester and phosphodiester, are poorly understood. Most of the investigations on extracellular enzymatic hydrolysis of marine DOP have focused on phosphomonoesterase (MEA) activity (i.e., alkaline phosphatase), whereas phosphodiesterase (DEA) measurements are scarce. This limits our understanding of the ecological and biogeochemical role of DOP sources other than P-monoesters in the sea. We determined extracellular MEA and DEA activities including their cell-free fractions on a bimonthly basis over 14 months in surface and mesopelagic subantarctic waters, thus covering a wide range of phosphate availability levels (from <0.5 to 2.3 µM). We found that DEA and MEA exhibit similar hydrolysis rates in surface as well as in mesopelagic waters. The MEA:DEA ratio varied between 0.38 and 5.42 during the study period, indicating potential differences in function and/or expression among the two enzyme groups, potentially reflecting differences in the availability and/or utilization of P-monoester and P-diester pools. Interestingly, the MEA:DEA was negatively correlated to phosphate (r = −0.82, p = 0.02, R2=0.67) and positively with the inorganic N:P ratio (r = 0.84, p = 0.02, R2 = 0.67), suggesting that the relative importance of DEA vs. MEA is linked to inorganic P availability and the N:P ratio. DEA was also related to the N:P ratio, both at the surface and at depth, suggesting DEA alone is sensitive to changes in the N:P ratio. The majority (>70%) of extracellular MEA and DEA was found in the cell-free fraction, increasing with depth for MEA. Our results indicated that DOP hydrolysis mediated by DEA in the surface as well as in dark ocean is as important as the frequently measured MEA.
Introduction
Microbial communities are the main drivers of the global biogeochemical cycles (Falkowski et al., 2008). In contrast to terrestrial systems, the bulk of the organic matter pool available in the oceans is largely comprised of dissolved organic matter (DOM) (Arnosti et al., 2014). Microbes, due to their small size, have exclusive access to this DOM pool, recycling essential nutrients back into marine food webs (Azam et al., 1983; Fenchel 2008). Microbes utilize preferentially high molecular weight DOM as conceptualized by the “size-reactivity model” (Amon and Benner, 1996; Benner and Amon, 2015). High molecular weight DOM, however, needs to be enzymatically cleaved into small molecular weight compounds (<600 Da) outside the cell before they can be funnelled through the complex cell wall of heterotrophic bacteria (Nikaido and Vaara, 1987; Nakae, 1976; Weiss et al., 1991; Amon and Benner, 1996; Benner and Amon, 2015). This requirement for extracellular hydrolysis makes microbial extracellular enzymatic activity (EEA) a rate limiting step in the recycling of marine organic matter (Hoppe, 1991).
Although dissolved inorganic phosphate (Pi) is the preferred form of phosphorus for microbial growth, phosphorus in the epipelagic, and upper mesopelagic layers of the ocean is mainly present as dissolved organic phosphorus (DOP) (Björkman and Karl, 2003; Duhamel et al., 2010; Moore et al., 2013). Phosphate esters (including monoesters and diesters), along with phosphonates, are the main components of DOP (Kolowith et al., 2001). The limited number of studies available indicate that marine P-diesters exhibit similar concentrations as P-monoesters in oceanic waters (Suzumura et al., 1998; Monbet et al., 2009; Yamaguchi et al., 2019). Still, most of the research on the extracellular hydrolytic activity in the ocean has focused on P-monoesterase (MEA) (i.e., alkaline phosphatase), while very little is known about P-diesterase (DEA) activity. MEA and DEA can come from a variety of sources in the environment including phytoplankton as well as heterotrophic microbes (Hoppe, 2003; Yamaguchi et al., 2014). Published DEA research has largely been based on culture studies (Yamaguchi et al., 2014; Accoroni et al., 2017). Available environmental studies have indicated that both P-monoesters and P-diesters can act as phosphorus sources for marine microbes living in the epipelagic layer (Sato et al., 2013; Yamaguchi et al., 2019). However, there are no studies on the seasonal dynamics of DEA to date. Hence, the relative importance of MEA and DEA in DOP hydrolysis in the surface waters remains unknown. Even less is known about the relative importance of MEA vs. DEA in the meso- and bathypelagic ocean. There are some studies on mesopelagic MEAs, often reporting comparable MEA activities in surface and in deep waters (Koike and Nagata, 1997; Hoppe and Ullrich, 1999; Tamburini et al., 2002; Baltar et al., 2009), with cell-specific MEAs increasing with depth down to the bathypelagic zone (Hoppe and Ullrich, 1999; Baltar et al., 2010; Baltar et al., 2013). However, there are no measurements available of DEA in the meso- and bathypelagic waters.
Extracellular enzymes can either be associated to the cell surface or released into the environment, i.e., cell-free enzymes or dissolved enzymes (Hoppe et al., 2002; Baltar, 2018). It has been found that cell-free EEA represents a significant fraction of the total EEA (cell-associated plus cell-free EEA), often accounting for the majority of the total enzyme pool (Duhamel et al., 2010; Allison et al., 2012; Baltar et al., 2016b; Baltar et al., 2019). A large contribution of the cell-free EEA to the total EEA has important ecological and biogeochemical implications because cell-free enzymes can be temporally and/or spatially decoupled from the producing cell (Baltar et al., 2010; Arnosti, 2011; Baltar et al., 2016b). Since residence times for cell-free enzymes are on the scale of days to weeks (Li et al., 1998; Ziervogel et al., 2010; Arnosti, 2011; Steen and Arnosti, 2011; Baltar et al., 2013; Thomson et al., 2019), the history of the water mass may have more explanatory power than the in situ microbial community in understanding EEA dynamics (Kamer and Rassoulzadegan, 1995; Baltar et al., 2010; Arnosti 2011; Baltar et al., 2016b). It has been suggested that a high proportion of cell-free MEA might explain the paradox of “high alkaline phosphatase activity at high Pi concentration” (Thomson et al., 2019). Thus, to understand the role of a particular type of EEA it is important to determine the contribution of the cell-free to the total EEA, and how it changes in response to different environmental conditions. The contribution of cell-free to total DEA ranged from 13–49% in the only study where the cell-free fraction of DEA was studied (Sato et al., 2013). The percentage of cell-free to total MEA ranges from 12–100% throughout the water column (Hoppe, 1986; Li et al., 1998; Van Wambeke et al., 2002; Van Wambeke et al., 2009; Duhamel et al., 2011; Allison et al., 2012; Baltar et al., 2016b; Baltar et al., 2019; Thomson et al., 2019). The proportion of cell-free MEA (as well as of glucosidases and leucine aminopeptidase) tends to increase with depth from the epi- to bathypelagic waters (Baltar et al., 2010). This increase in the cell-free EEA with depth is consistent with a recent multi-omics study revealing a relative increase in the secretory peptidases and carbohydrate active enzymes (CAZymes) expression from the epi- to bathypelagic layers (Zhao et al., 2020). However, despite the importance of cell-free EEA in the dark ocean, there are no reports on the contribution of cell-free to total DEA and its relation to MEA in the dark ocean.
In this study, the seasonal dynamics of total and cell-free DEA and MEA are reported for epi- and mesopelagic waters. We hypothesized that the relative contribution of MEA and DEA varies from surface to mesopelagic waters and that the fraction of cell-free DEA covaries with the fraction of cell-free MEA.
Methods
Study Site and Sampling
Sampling was conducted bimonthly between November 2017 and January 2019 as part of MOTS (Munida Microbial Observatory Time-Series, https://www.otago.ac.nz/mots/about/index.html). MOTS is part of the well-established Munida Time Series Transect, where samples across the subtropical and subantarctic waters off the south east coast of New Zealand are taken for more than two decades (Currie and Hunter 1999; Currie et al., 2011; Baltar et al., 2015; Baltar et al., 2016a). For this study, samples were taken at the most offshore station (Station 8), located in subantarctic waters approximately 65 km off the Otago coast (−45.83°N, 171.54°E). Station 8 was chosen for this sampling as it is known to be subantarctic water consistently thought the year in the epi-and mesopelagic (Jones et al., 2013). Seawater samples were taken in triplicate for extracellular enzymatic activities from 2 m depth using an onboard continuous pump, and from the mesopelagic (500 and 1000 m) waters using Niskin bottles mounted on a rosette sampler. Temperature was logged on conductivity temperature depth profilers. Samples collected from 1000 m were only taken on the final two research cruises.
Nutrient and Chlorophyll-a Analyses
Nutrient analyses were performed on a SEAL AA3 HR auto-analyser (Seal Analytical, United States). All sampling equipment was rinsed three times with Milli-Q water before and after soaking in 10% HCl for > 6 h and oven dried at 60°C prior to collecting samples. Seawater samples were filtered (GF/F) then immediately frozen until analysis for dissolved reactive phosphorus and dissolved inorganic nitrogen. The detection limits were 0.1 mmol m−3 for dissolved inorganic nitrogen and 0.03 mmol m−3 for dissolved reactive phosphorus. Samples for chlorophyll-a analysis were filtered on-board using low vacuum (e.g., <200 mm Hg) through Whatman GF/F filters and frozen until analysis. Chlorophyll-a was extracted in 90% acetone for 16–24 h and measured using a calibrated Turner Designs Fluorometer following the procedures outlined by Parsons et al., (1984).
Bacterial Abundance
The bacterial abundance was determined by flow cytometry as described elsewhere (Gasol (1999), Gasol and Del Giorgio (2000) using a FACS Canto II (Becton & Dickinson) with a blue laser (488 nm wavelength). Samples (0.4 ml) preserved in 2% glutaraldehyde were stained with 1:10,000 final concentration of Sybr Green I in the dark for 15 min. Samples were analyzed at low speed for 2 min each and FL1 positive events counted on a FL1 vs. SSC plot using FlowJo10 software.
Extracellular Enzymatic Activity Assays
Extracellular enzymatic activities were assessed based on the hydrolysis of the fluorogenic substrate analogues (Hoppe, 1983). The fluorogenic substrates 4-methylumbelliferyl (MUF)-phosphate, and Bis(MUF)-phosphate were used to assess phosphomonoesterase (MEA, i.e., alkaline phosphatase) and phosphodiesterase (DEA) activities. DEA estimates using Bis(MUF)-phosphate can theoretically be overestimated because one molecule of Bis-MUP can release two molecules of 4-methylumbelliferone, the latter of which is catalyzed by monoesterase, although in practice it was shown that it was overestimated by at most 5–16% in a large transect extending from the North to the South Pacific Ocean (Sato et al., 2013). Saturating substrate concentrations of 100 μM were used based on pre-established kinetics for MEA with sample from this study site. Since P-diesters are usually at lower concentrations than P-monoesters in the ocean (Yamaguchi et al., 2019), and therefore what is saturating for MEA should also be so for DEA, we decided to use the same saturating concentration of 100 µM for DEA in order to compare MEA to DEA rates under the same conditions. These concentrations are saturating, and hence they only represent potential activity rates in the environment. The saturating concentrations we obtained in our preliminary test (which ranged between 50 and 100 µM) are consistent with those from many other previous marine studies (Baltar et al., 2010; Celussi and Del Negro, 2012; Steen et al., 2016; Celussi et al., 2019). Greiner Bio-one 96-well non-protein binding microplates were filled with six technical replicates of the fluorogenic substrates (10 μl) and seawater (290 μl) reaching final concentrations of 100 μM. Standards were prepared using 0.22 μm filtered seawater. Plates were read in a Spectramax M2 spectrofluorometer (Molecular Devices, United States) with excitation and emission wavelengths of 365 and 445 nm, respectively, both before and after an incubation time of 3 h. The wavelength bandwidth was 9 nm with a detection limit of 3.0 fmol/well in 200 μl FITC 96 wells. Six replicate samples without substrate addition served as blanks in each plate. Incubations were performed in the dark at the mean seawater temperature at the time of sampling. To separate the cell-free fraction from the total EEA pool, samples were gently filtered through low protein binding 0.22 μm PES Millex-GP syringe filters following published protocols (Kim et al., 2007; Baltar et al., 2010). Cell-specific extracellular enzymatic activities were calculated by dividing the EEA rates by the abundance of bacterial cells.
Statistical Analyses
Pearson’s correlations were carried out using the software package Sigma Stat 2.03 (SPSS). When tests for normality (Kolmogorov-Smirnov) were not met, data were square root transformed prior to analysis. When transformations were not possible Spearman’s correlations were applied.
Results and Discussion
Temperatures ranged from 8.7 to 17.4°C in the epipelagic layer and around 7°C in the mesopelagic waters (500 m) during the study period (Figure 1A). There was a shift in the inorganic nutrient concentrations in July 2018 in the epi- and mesopelagic waters, which separated the study into two periods (pre- and post-July 2018) (Figure 1A). In the epipelagic waters, mean phosphate and nitrate concentrations increased in July 2018 (from 0.51–1.03 µM and 8.1–11.8 µM for phosphate and nitrate, respectively), remaining at about these levels for the rest of the study (Figure 1A). In contrast, in the mesopelagic layer phosphate and nitrate concentrations decreased from February 2018 to April 2018. This increase in surface waters and decrease in mesopelagic waters of inorganic nutrients might be due to the austral winter overturning and mixing of surface and mesopelagic waters, which would cause a relative dilution of deep water and an enrichment in surface waters. This is consistent with the seasonal characterisation of our study region/transect, in which the mixed layer of our Station 8 (SAW) varied from 20 m in summer to >200 m in winter (Jones et al., 2013). Chlorophyll-a concentrations indicated the presence of an austral autumn and spring-summer phytoplankton bloom in March-April and November 2018, respectively, coinciding with an increase in bacterial abundance (Figure 1B).
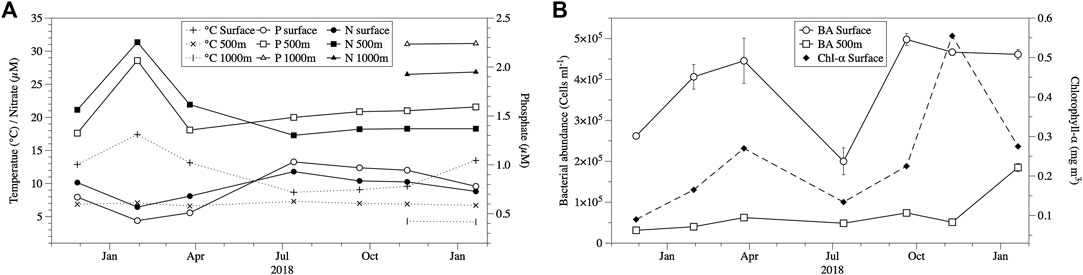
FIGURE 1. Seasonal variability in temperature, phosphate, and nitrate (A), and chlorophyll-a and mean (±SE) bacterial abundance (B) in the epi- and mesopelagic layer at the study site in subantarctic waters.
MEA and DEA activities were always detected throughout the study (both in the epi- and mesopelagic waters despite the observed differences in temperature and phosphate concentrations (Figure 2). MEA and DEA were in the same range in both epi- and mesopelagic waters (Figure 2A). MEA ranged between 2.75–12.23 nmol L−1 h−1 (mean ± SE: 8.6 ± 1.5 nmol L−1 h−1), and DEA between 1.43–20.44 nmol L−1 h−1 (10.9 ± 4.7 nmol L−1 h−1). The MEA rates measured in the subantarctic waters were similar to the MEA rates measured previously in this water mass (Thomson et al., 2019) and in the North Atlantic by Steen et al. (2016) but were frequently lower than some reports from the Mediterranean (Celussi and Del Negro, 2012; Celussi et al., 2019). In addition, our MEA rates were higher than those reported from the North Atlantic by (Baltar et al., 2009; Baltar et al., 2010; Baltar et al., 2013), North Pacific (Koike and Nagata, 1997), Indian Ocean (Tamburini et al., 2002) and Mediterranean Sea (Hoppe and Ullrich, 1999) which typically exhibit MEA rates < 4 nmol L−1 h−1. The DEA rates measured in the subantarctic waters were comparable at times to values reported by Yamaguchi et al. (2019) from the North Pacific but were generally higher overall and were much higher (1–2 orders of magnitude) than those reported by Sato et al. (2013) for the North and South Pacific.
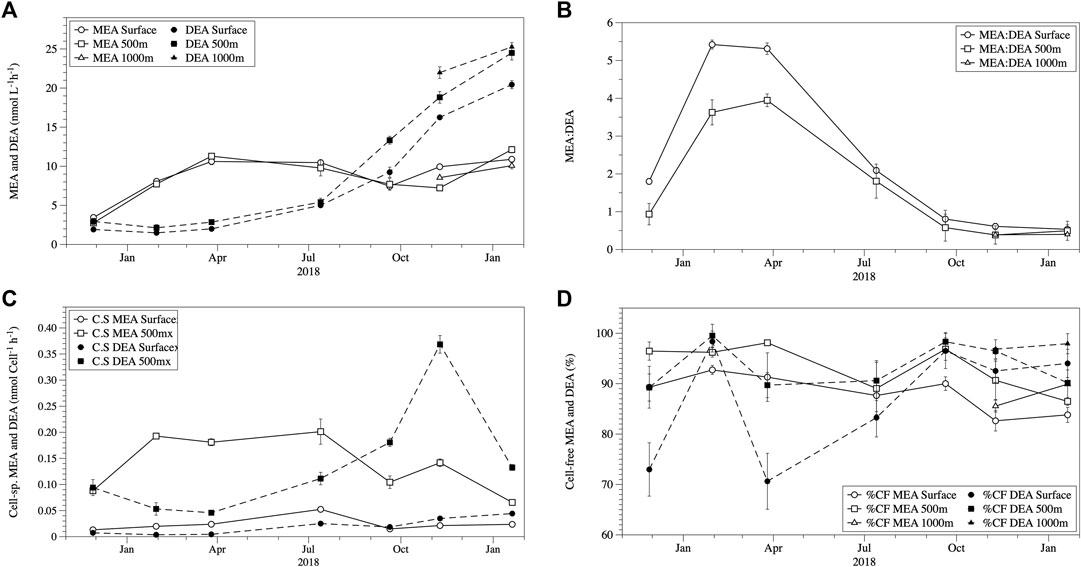
FIGURE 2. Seasonal variation in mean (±SE) total phosphomonoesterase activity (MEA) and phosphodiesterase activity (DEA) (A), the ratio of MEA to DEA (±SE) (B), the mean (±SE) cell-specific MEA and DEA given in nmol/cell−1/h−1(C), and the mean (±SE) cell-free (CF) fractions for MEA and DEA (D) in the epi- and mesopelagic layer at the study site.
Prior to July 2018, MEA was higher than DEA, however, this was reversed after July 2018 (Figure 2A). The ratio MEA:DEA decreased from 5.3 to 2.1 in the epipelagic and from 4.0 to 1.8 in the mesopelagic waters by July 2018 and decreased to MEA:DEA ratios <1 from November 2018 onwards (Figure 2B). The shift in the MEA:DEA ratio also indicates potential changes in the availability of different P-esters and/or microbial enzyme groups. The distribution pattern for the two P-esters has been shown to differ latitudinally and with depth in the open ocean (Yamaguchi et al., 2019), which might explain the changes in the dominance from MEA prior to July to DEA after July (Figure 2B). Unfortunately, no measurements of phosphate esters are available from the study site. The shift in the MEA:DEA ratio coincides with the increase in inorganic P and N concentrations in July. Although no significant correlations were found for the MEA or DEA and any of the environmental parameters, a negative correlation between the MEA:DEA ratio and phosphate concentration was observed in surface waters (Table 1, Pearson’s R2 = 0.67, p = 0.02) along with a positive correlation between the N:P ratio and MEA:DEA (Pearson’s R2 = 0.67, p = 0.02). These relationships between the MEA:DEA ratio, phosphate, and the N:P ratio were not found in the mesopelagic, which is reasonable considering the fairly high phosphate concentration in the mesopelagic waters. The N:P ratio also showed negative correlations with total DEA in the epipelagic waters (Table 1, Pearson’s R2 = 0.58, p = 0.02) and in the mesopelagic layer (Spearman’s R2 = 0.59, p = 0.03). It is not clear why the negative correlations between DEA and the N:P ratio exist. Sato et al. (2013) found no relationship between phosphate concentration and total MEA and DEA in the South Pacific, but a negative relationship in the North Pacific. These findings from the South Pacific are consistent with the observations in this study, as we also did not find any relation between total MEA or DEA and phosphate. Collectively, these results show that DEA can, at times, be as important as MEA for DOP hydrolysis, indicating a key role of phosphate availability in the relative role of MEA vs. DEA.
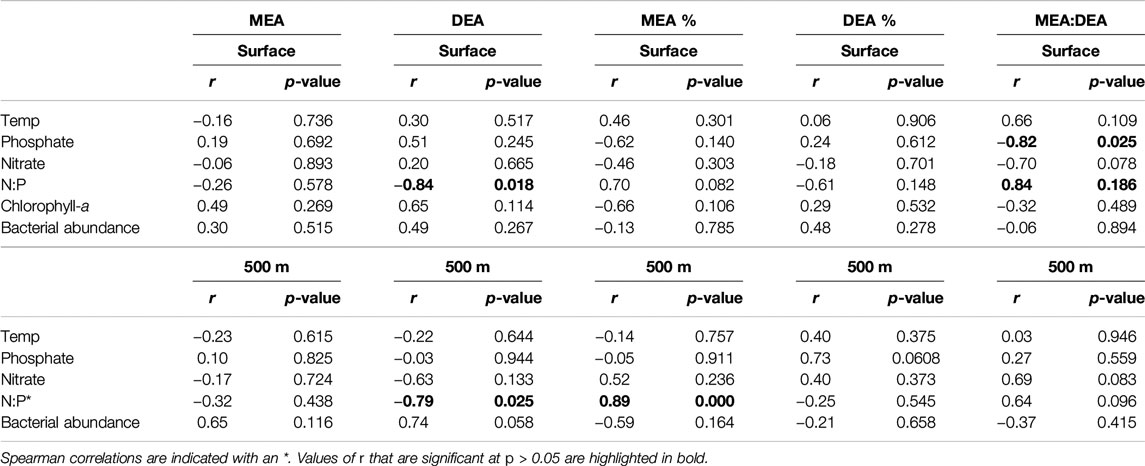
TABLE 1. Pearson’s correlation coefficient (r) between the total, cell-free fractions (%) and ratios of phosphomonoesterase activity (MEA) and/to phosphodiesterase activity (DEA) and temperature, phosphate, nitrate, chlorophyll-a, and bacterial abundance.
Interestingly, the temporal patterns in MEA and DEA described above were detected in the epi- as well as in the mesopelagic waters, indicating a strong link between these two layers in DOP cycling. The cell-specific (i.e., total enzymatic activities per bacterial cell) MEA and DEA activities increased with depth (Figure 2C), mainly caused by the decreasing bacterial abundance with depth (Figure 1B). This is consistent with increases in cell-specific MEA from the epi- to the bathypelagic waters reported for the North Atlantic and Indian Ocean (Hoppe and Ullrich, 1999; Baltar et al., 2010; Baltar et al., 2013). As there are no previous cell-specific DEA rates available from mesopelagic waters, our results are the first to confirm hydrolysis of DOP in the mesopelagic waters by DEA. In our particular study, the key observation that almost all MEA and DEA was found in the cell-free fraction implies that the activity that we measured was in fact not associated with any particular group of organisms. The high (and comparable to surface) MEA and DEA rates in the mesopelagic (where phytoplankton should not be active) further indicates that if these extracellular enzymes would be associated with some organism it is most likely that it will be to heterotrophic prokaryotes (at least in the deep waters). The intention of normalizing the MEA and DEA rates to some biological source (in this case we selected heterotrophic prokaryotes because of the reasons just mentioned above) was to see briefly how the relation was between the MEA and DEA rates and the presence of microorganisms with depth/time. However, this should be interpreted with some skepticism due to the complications arising from the cell-free fractions.
Cell-free enzymes accounted for the vast majority of both MEA and DEA in the epi- and mesopelagic waters throughout the study period (Figure 2D). In the epipelagic water, cell-free MEA contributed 88 ± 1.4% to total MEA and cell-free DEA 87 ± 4.3% to total DEA. Reports of the cell-free MEA proportion on total MEA vary widely ranging from as low as 12–100% of total MEA (Hoppe, 1986; Allison et al., 2012; Baltar et al., 2016b). Cell-free DEA contributed between 71 and 100% (91 ± 2%) to total DEA and was higher than reported in the only other published study (range: 13–49%, 38 ± 15%) in epipelagic waters (Sato et al., 2013). Possibly, the high cell-free DEA fraction in the present study might be associated with the low temperature characteristic of the subantarctic waters at our study site. The increase in the contribution of cell-free enzymes has been repeatedly linked to lower temperatures in the Atlantic Ocean (Baltar et al., 2010), in the Baltic Sea (Baltar et al., 2016b) and in laboratory experiments (Baltar et al., 2017). Lower temperatures are suggested to contribute to higher cell-free enzymatic activities due to the combined positive effects of cold preservation and reduced rates of heterotrophic consumption of dissolved proteins/enzymes (Baltar et al., 2016b; Baltar 2018).
In the mesopelagic layer, the contribution from cell-free MEA (92 ± 1.6%) was higher than in the epipelagic waters t(12) = −2.322, p = 0.039*, DEA was also higher (93 ± 1.4%) but was not statistically significant t(12) = −1.413, p = 0.163 (Figure 2D). This is consistent with previous studies reporting increasing cell-free proportions of MEA with depth (Baltar et al., 2010; Baltar et al., 2013). Although there are no other mesopelagic DEA estimates to make comparisons, the high contribution of cell-free MEA and DEA in the epi- and mesopelagic waters might explain the coupling observed between these two water layers, by transport of extracellular enzymes either via winter vertical overturning and mixing, and/or by fragmentation and dissolution of sinking particles (Koike and Nagata, 1997).
In conclusion, at the study site in South Pacific Antarctic waters, DEA rates can be comparable, at times, to the more frequently measured MEA. This suggests that DEA is relevant for the hydrolysis of DOP. The negative relationship between the MEA:DEA ratio and phosphate concentrations, and the positive relationship with the inorganic N:P ratio in epipelagic waters suggest that inorganic phosphorus availability along with the N:P ratio is the key parameter in controlling the relative importance of MEA vs. DEA in marine DOP hydrolysis. N:P ratios also showed negative relationships with DEA alone, both in the epi- and mesopelagic layer, suggesting DEA is sensitive to changes in the inorganic N:P ratio. Most of the DEA and MEA was found in the cell-free fraction and the contribution of the cell-free fractions to the total MEA and DEA was higher in the mesopelagic than in the epipelagic layer. These findings enhance our understanding of the marine ecological and biogeochemical mechanisms underpinning the cycling of phosphorus.
Data Availability Statement
All datasets presented in this study are included in the article.
Author Contributions
All authors contributed to the preparation of this manuscript
Funding
This research was supported by a University of Otago research grant and a Rutherford Discovery Fellowship (Royal Society of New Zealand) to FB.
Conflict of Interest
The authors declare that the research was conducted in the absence of any commercial or financial relationships that could be construed as a potential conflict of interest.
Acknowledgments
We thank the skipper of the RV Polaris II Bill Dickson and his crew for their help during the sampling events and the technical support by Dr. Doug Mackie, Linda Groenewegen, and Adam Brook at the Portobello Marine Laboratory.
References
Accoroni, S., Tartaglione, L., Dello Iacovo, E., Pichierri, S., Marini, M., Campanelli, A., et al. (2017). Influence of environmental factors on the toxin production of Ostreopsis cf. ovata during bloom events. Mar. Pollut. Bull. 123, 261–268. doi:10.1016/j.marpolbul.2017.08.049
Allison, S., Chao, Y., Farrara, J., Hatosy, S., and Martiny, A. (2012). Fine-scale temporal variation in marine extracellular enzymes of coastal southern California. Front. Microbiol. 3, 301. doi:10.3389/fmicb.2012.00301
Amon, R. M. W., and Benner, R. (1996). Bacterial utilization of different size classes of dissolved organic matter. Limnol. Oceanogr. 41, 41–51. doi:10.4319/lo.1996.41.1.0041
Arnosti, C. (2011). Microbial extracellular enzymes and the marine carbon cycle. Annu. Rev. Mar. Sci. 3, 401–425. doi:10.1146/annurev-marine-120709-142731
Arnosti, C., Bell, C., Moorhead, D. L., Sinsabaugh, R. L., Steen, A. D., Stromberger, M., et al. (2014). Extracellular enzymes in terrestrial, freshwater, and marine environments: perspectives on system variability and common research needs. Biogeochemistry 117, 5–21. doi:10.1007/s10533-013-9906-5
Azam, F., Fenchel, T., Field, J., Gray, J., Meyer-Reil, L., and Thingstad, F. (1983). The ecological role of water-column microbes in the sea. Mar. Ecol. Prog. Ser. 10, 257–263. doi:10.3354/meps010257
Baltar, F. (2018). Watch out for the “living dead”: cell-free enzymes and their fate. Front. Microbiol. 8, 2438. doi:10.3389/fmicb.2017.02438
Baltar, F., Arístegui, J., Gasol, J., Sintes, E., Van Aken, H., and Herndl, G. (2010). High dissolved extracellular enzymatic activity in the deep Central Atlantic Ocean. Aquat. Microb. Ecol. 58, 287–302. doi:10.3354/ame01377
Baltar, F., Arístegui, J., Gasol, J. M., Yokokawa, T., and Herndl, G. J. (2013). Bacterial versus archaeal origin of extracellular enzymatic activity in the northeast atlantic deep waters. Microb. Ecol. 65, 277–288. doi:10.1007/s00248-012-0126-7
Baltar, F., Arístegui, J., Sintes, E., Van Aken, H. M., Gasol, J. M., and Herndl, G. J. (2009). Prokaryotic extracellular enzymatic activity in relation to biomass production and respiration in the meso- and bathypelagic waters of the (sub)tropical Atlantic. Environ. Microbiol. 11, 1998–2014. doi:10.1111/j.1462-2920.2009.01922.x
Baltar, F., Currie, K., Stuck, E., Roosa, S., and Morales, S. E (2016a). Oceanic fronts: transition zones for bacterioplankton community composition. Environ. Microbiol. Rep. 8, 132–138. doi:10.1111/1758-2229.12362
Baltar, F., Legrand, C., and Pinhassi, J. (2016b). Cell-free extracellular enzymatic activity is linked to seasonal temperature changes: a case study in the Baltic Sea. Biogeosciences 13, 2815–2821. doi:10.5194/bg-13-2815-2016
Baltar, F., De Corte, D., Thomson, B., and Yokokawa, T. (2019). Teasing apart the differentsize pools of extracellular enzymatic activity in the ocean. Sci. Total Environ. 660, 690–696. doi:10.1016/j.scitotenv.2019.01.083
Baltar, F., Morán, X. A. G., and Lønborg, C. (2017). Warming and organic matter sources impact the proportion of dissolved to total activities in marine extracellular enzymatic rates. Biogeochemistry 133, 307–316. doi:10.1007/s10533-017-0334-9
Baltar, F., Stuck, E., Morales, S., and Currie, K. (2015). Bacterioplankton carbon cycling along the subtropical frontal zone off New Zealand. Prog. Oceanogr. 135, 168–175. doi:10.1016/j.pocean.2015.05.019
Benner, R., and Amon, R. M. W. (2015). The size-reactivity continuum of major bioelements in the ocean. Annu. Rev. Mar. Sci. 7, 185–205. doi:10.1146/annurev-marine-010213-135126
Björkman, K. M., and Karl, D. M. (2003). Bioavailability of dissolved organic phosphorus in the euphotic zone at Station ALOHA, North Pacific Subtropical Gyre. Limnol. Oceanogr. 48, 1049–1057. doi:10.4319/lo.2003.48.3.1049
Celussi, M., Zoccarato, L., Bernardi Aubry, F., Bastianini, M., Casotti, R., Balestra, C., et al. (2019). Links between microbial processing of organic matter and the thermohaline and productivity features of a temperate river-influenced Mediterranean coastal area. Estuar. Coast Shelf Sci. 228, 106378. doi:10.1016/j.ecss.2019.106378
Celussi, M., and Del Negro, P. (2012). Microbial degradation at a shallow coastal site: long-term spectra and rates of exoenzymatic activities in the NE Adriatic Sea. Estuar. Coast Shelf Sci. 115, 75–86. doi:10.1016/j.ecss.2012.02.002
Currie, K. I., and Hunter, K. A. (1999). Seasonal variation of surface water CO2 partial pressure in the Southland Current, east of New Zealand. Mar. Freshwater Res. 50, 375–382. doi:10.1071/mf98115
Currie, K. I., Reid, M. R., and Hunter, K. A. (2011). Interannual variability of carbon dioxide drawdown by subantarctic surface water near New Zealand. Biogeochemistry 104, 23–34. doi:10.1007/s10533-009-9355-3
Duhamel, S., Björkman, K. M., Van Wambeke, F., Moutin, T., and Karl, D. M. (2011). Characterization of alkaline phosphatase activity in the North and South Pacific Subtropical Gyres: implications for phosphorus cycling. Limnol. Oceanogr. 56, 1244–1254. doi:10.4319/lo.2011.56.4.1244
Duhamel, S., Dyhrman, S. T., and Karl, D. M. (2010). Alkaline phosphatase activity and regulation in the North Pacific Subtropical Gyre. Limnol. Oceanogr. 55, 1414–1425. doi:10.4319/lo.2010.55.3.1414
Falkowski, P., Fenchel, T., and Delong, E. (2008). The microbial engines that drive earth's biogeochemical cycles. Science 320, 1034–1039. doi:10.1126/science.1153213
Fenchel, T. (2008). The microbial loop – 25 years later. J. Exp. Mar. Biol. Ecol. 366, 99–103. doi:10.1016/j.jembe.2008.07.013
Gasol, J. M. (1999). How to count picoalgae and bacteria with the FACScalibur flow cytometer. Barcelona, Spain: Institut de Ciències del Mar, 1–51.
Gasol, J. M., and Del Giorgio, P. A. (2000). Using flow cytometry for counting natural planktonic bacteria and understanding the structure of planktonic bacterial communities. Sci. Mar. 64, 197–224. doi:10.3989/scimar.2000.64n2197
Hoppe, H. G. (1983). Significance of exoenzymatic activities in the ecology of brackish water: measurements by means of methylumbelliferyl-substrates. Mar. Ecol. Prog. Ser. 11, 299–308. doi:10.3354/meps011299
Hoppe, H.-G. (1986). “Relations between bacterial extracellular enzyme activities and heterotrophic substrate uptake in a brackish water environment,” in Proceeding of 2nd International Colloquium on Marine Bacteriology, Actes de Colloque 3, Brest, France, October 1–5, 1986.
Hoppe, H.-G. (1991). “Microbial extracellular enzyme activity: a new key parameter in aquatic ecology,” in Microbial enzymes in aquatic environments. Editor Chróst, R. J. (New York, NY: Springer), 60–83.
Hoppe, H.-G. (2003). Phosphatase activity in the sea. Hydrobiologia 493, 187–200. doi:10.1023/A:1025453918247.
Hoppe, H.-G., Arnosti, C., and Herndl, G. F. (2002). “Ecological significance of bacterial enzymes in the marine environment,” in Enzymes in the environment. Editors Burns, R. G., and Dick, R. P. (New York, NY: Marcel Dekker), 73–107.
Hoppe, H., and Ullrich, S. (1999). Profiles of ectoenzymes in the Indian Ocean: phenomena of phosphatase activity in the mesopelagic zone. Aquat. Microb. Ecol. 19, 139–148. doi:10.3354/ame019139
Jones, K. N., Currie, K. I., McGraw, C. M., and Hunter, K. A. (2013). The effect of coastal processes on phytoplankton biomass and primary production within the near-shore Subtropical Frontal Zone. Estuarine. Estuar Coast Shelf Sci. 124, 44. doi:10.1016%2Fj.ecss.2013.03.003
Kamer, M., and Rassoulzadegan, F. (1995). Extracellular enzyme activity: indications for high short-term variability in a coastal marine ecosystem. Microb. Ecol. 30, 143–156. doi:10.1007/BF00172570
Kim, C., Nishimura, Y., and Nagata, T. (2007). High potential activity of alkaline phosphatase in the benthic nepheloid layer of a large mesotrophic lake: implications for phosphorus regeneration in oxygenated hypolimnion. Aquat. Microb. Ecol. 49, 303–311. doi:10.3354%2Fame01137
Koike, I., and Nagata, T. (1997). High potential activity of extracellular alkaline phosphatase in deep waters of the central Pacific. Deep Sea Res. Part II Top. Stud. Oceanogr. 44, 2283–2294. doi:10.1016/S0967-0645(97)00025-8
Kolowith, L. C., Ingall, E. D., and Benner, R. (2001). Composition and cycling of marine organic phosphorus. Limnol. Oceanogr. 46, 309–320. doi:10.4319/lo.2001.46.2.0309
Li, H., Veldhuis, M., and Post, A. (1998). Alkaline phosphatase activities among planktonic communities in the northern Red Sea. Mar. Ecol. Prog. Ser. 173, 107–115. doi:10.3354%2Fmeps173107
Monbet, P., McKelvie, I. D., and Worsfold, P. J. (2009). Dissolved organic phosphorus speciation in the waters of the Tamar estuary (SW England). Geochem. Cosmochim. Acta 73, 1027–1038. doi:10.1016/j.gca.2008.11.024.
Moore, C. M., Mills, M. M., Arrigo, K. R., Berman-Frank, I., Bopp, L., Boyd, P. W., et al. (2013). Processes and patterns of oceanic nutrient limitation. Nat. Geosci. 6, 701. doi:10.1038%2FNGEO1765
Nakae, T. (1976). Identification of the outer membrane protein of E. coli that produces transmembrane channels in reconstituted vesicle membranes. Biochem. Biophys. Res. Commun. 71, 877–884. doi:10.1016/0006-291x(76)90913-x
Nikaido, H., and Vaara, M. (1987). Escherichia coli and Salmonella typhimurium: cellular and molecular biology. Washington, DC: American Society for Microbiology, Vol. 1, 7–22.
Parsons, T., Maita, Y., and Lalli, C. (1984). A manual of chemical and biological methods for seawater analysis. Pergamon, Oxford sized algae and natural seston size fractions. Mar. Ecol. Prog. Ser. 199, 43–53. doi:10.1016/C2009-0-07774-5
Sato, M., Sakuraba, R., and Hashihama, F. (2013). Phosphate monoesterase and diesterase activities in the North and South Pacific Ocean. Biogeosciences 10, 7677. doi:10.5194%2Fbg-10-7677-2013
Steen, A. D., and Arnosti, C. (2011). Long lifetimes of β-glucosidase, leucine aminopeptidase, and phosphatase in Arctic seawater. Mar. Chem. 123, 127–132. doi:10.1016%2Fj.marchem.2010.10.006
Steen, A. D., Quigley, L. N., and Buchan, A. (2016). Evidence for the priming effect in a planktonic estuarine microbial community. Front. Mar. Sci. 3, 6. doi:10.3389%2Ffmars.2016.00006
Suzumura, M., Ishikawa, K., and Ogawa, H. (1998). Characterization of dissolved organic phosphorus in coastal seawater using ultrafiltration and phosphohydrolytic enzymes. Limnol. Oceanogr. 43, 1553–1564. doi:10.4319/lo.1998.43.7.1553
Tamburini, C., Garcin, J., Ragot, M., and Bianchi, A. (2002). Biopolymer hydrolysis and bacterial production under ambient hydrostatic pressure through a 2000 m water column in the NW Mediterranean. Deep Sea Res. Part II Top. Stud. Oceanogr. 49, 2109–2123. doi:10.1016%2FS0967-0645(02)00030-9
Thomson, B., Wenley, J., Currie, K., Hepburn, C., Herndl, G. J., and Baltar, F. (2019). Resolving the paradox: continuous cell-free alkaline phosphatase activity despite high phosphate concentrations. Mar. Chem. 214, 103671. doi:10.1016%2Fj.marchem.2019.103671
Van Wambeke, F., Christaki, U., Giannakourou, A., Moutin, T., and Souvemerzoglou, K. (2002). Longitudinal and vertical trends of bacterial limitation by phosphorus and carbon in the Mediterranean Sea. Microb. Ecol. 43, 119. doi:10.1007%2Fs00248-001-0038-4
Van Wambeke, F., Ghiglione, J. F., Nedoma, J., Mével, G., and Raimbault, P. (2009). Bottom up effects on bacterioplankton growth and composition during summer-autumn transition in the open NW Mediterranean Sea. Biogeosciences 6, 705–720. doi:10.5194%2Fbg-6-705-2009
Weiss, M. S., Abele, U., Weckesser, J., Welte, W., Schiltz, E., and Schulz, G. E. (1991). Molecular architecture and electrostatic properties of a bacterial Porin. Science 254, 1627–1630. doi:10.1126/science.1721242
Yamaguchi, H., Arisaka, H., Otsuka, N., and Tomaru, Y. (2014). Utilization of phosphate diesters by phosphodiesterase-producing marine diatoms. J. Plankton Res. 36, 281–285. doi:10.1093%2Fplankt%2Ffbt091
Yamaguchi, T., Sato, M., Hashihama, F., Ehama, M., Shiozaki, T., Takahashi, K., et al. (2019). Basin‐scale variations in labile dissolved phosphoric monoesters and diesters in the central North Pacific ocean. J. Geophys. Res. 124, 3058–3072. doi:10.1029/2018JC014763
Zhao, Z., Baltar, F., and Herndl, G. J. (2020). Linking extracellular enzymes to phylogeny indicates a predominantly particle-associated lifestyle of deep-sea prokaryotes. Sci. Adv. 6, eaaz4354. doi:10.1126%2Fsciadv.aaz4354
Keywords: extracellular enzymatic activity, phosphomonoesterase, phosphodiesterase, alkaline phosphatase activity, phosphorus
Citation: Thomson B, Wenley J, Lockwood S, Twigg I, Currie K, Herndl GJ, Hepburn CD and Baltar F (2020) Relative Importance of Phosphodiesterase vs. Phosphomonoesterase (Alkaline Phosphatase) Activities for Dissolved Organic Phosphorus Hydrolysis in Epi- and Mesopelagic Waters. Front. Earth Sci. 8:560893. doi:10.3389/feart.2020.560893
Received: 11 May 2020; Accepted: 31 August 2020;
Published: 24 September 2020.
Edited by:
Timothy Ferdelman, Max Planck Institute for Marine Microbiology (MPG), GermanyReviewed by:
Masahiro Suzumura, National Institute of Advanced Industrial Science and Technology (AIST), JapanFrance Van Wambeke, Centre National de la Recherche Scientifique (CNRS), France
Copyright © 2020 Thomson, Baltar, Herndl, Currie, Hepburn, Wenley, Lockwood and Twigg. This is an open-access article distributed under the terms of the Creative Commons Attribution License (CC BY). The use, distribution or reproduction in other forums is permitted, provided the original author(s) and the copyright owner(s) are credited and that the original publication in this journal is cited, in accordance with accepted academic practice. No use, distribution or reproduction is permitted which does not comply with these terms.
*Correspondence: Blair Thomson, YmxhaXIudGhvbXNvbkBvdGFnby5hYy5ueg==, Federico Baltar, ZmVkZXJpY28uYmFsdGFyQHVuaXZpZS5hYy5hdA==