- National Centre for Sustainable Coastal Management, Ministry of Environment, Forest and Climate Change, Chennai, India
Tropical seagrasses experience considerable spatio-temporal changes with light and other biogeochemical conditions and adopt species-specific acclimatization strategies. Variation in photo-acclimatory responses of three tropical seagrass species (Cymodocea serrulata, Thalassia hemprichii, and Enhalus acoroides) was studied by measuring photosynthetic electron transport rates (ETR) using Pulse Amplitude Modulated (PAM) fluorometry. Quantitative values of ETR and rates of photosynthetic O2 evolution (net O2 exchange corrected for dark respiration) were compared to establish species-specific relationships in shallow water conditions. The apparent average molar ratio of O2 evolution to ETR for the studied species found lower than the theoretical value (0.25). The highest photosynthetic activity (ETRmax) varied significantly among the three studied species in the decreasing order as: E. acoroides (59.27); T. hemprichii (54.06), and C. serrulata (46.72). The effective quantum yield (Y) of PS II (Photosystem II), one of the most useful indicators of stress conditions for seagrass, was observed to be significantly higher for E. acoroides compared to the other two species. It appears that the variations in water temperature and pCO2 could significantly alter the photosynthetic characteristics of these shallow water seagrass species. This study is useful in predicting the photo-acclimation strategies to any change in light availability and subsequent biogeochemical changes at the sea bed by these tropical seagrass species.
Introduction
Seagrass meadows are best known for their efficiency in capturing and converting light energy into organic matter, and its supply to other trophic levels (Hemminga and Duarte, 2000; Ow et al., 2015). Furthermore, seagrass has been identified as the real winner under the changing climate due to its ability to utilize additional dissolved inorganic carbon (DIC). Since tropical seagrass meadows exhibit a greater photosynthetic affinity for CO2 than HCO3–, increased availability of CO2 under ocean acidification can further boost the gross productivity (Durako, 1993). Alexandre et al. (2012) suggested that the global effects of CO2 increase on the DIC utilization of seagrass may be spatially heterogeneous and depend on the specific nitrogen availability of each system. This DIC utilization is largely regulated by light availability as other physico-chemical parameters are often altered through varying land runoff (Ow et al., 2015) in addition to the availability of water column producers. Earlier, Durako (1993) observed a significant increase in photosynthesis and subsequent growth in response to enhanced DIC concentrations in both tropical and temperate seagrass species.
Seagrass degradation is often associated with coastal water quality declines, eutrophication and enhanced water column turbidity which ultimately lead to limitation of available light to these primary producers (Orth et al., 2006; Waycott et al., 2009). Seagrass light acclimation may vary both on temporal and spatial scales, from the position along a single leaf blade to within the canopy and finally across the entire meadow. Leaf absorbance is often regulated by factors such as pigment content, morphology and physical properties, water depth, resource availability (Ralph et al., 2007). Additionally, the species-specific photo-acclimatory strategies (Schubert et al., 2018) often linked to the species’ life history strategies, may explain variations in inter responses to any disturbance. The light requirements of seagrass photosynthesis have been studied by a considerable number of researchers, indicating the possibility of inter-species level variations and the minimum light requirement among the seagrass species (Lee et al., 2007). These studies also concluded that the light requirements by seagrass may also vary within species, depending on conditions prevailing in the micro-environments (Czerny and Dunton, 1995). Even though most of these studies on light response of seagrasses are carried out under laboratory conditions, in situ studies in natural conditions are essential to evaluate the observations made from prior laboratory and mesocosm studies (Campbell and Fourqurean, 2013). Diving PAM fluorometer that deals with continuous short measuring-light pulses of red or blue light are successfully used to estimate photosynthetic parameters a. The maximal fluorescence emission (Fm) is achieved by applying saturating light pulse to the plant sample (van Kooten and Snel, 1990). In case of dark-adapted plants, the maximum photochemical efficiency of photosystem II (PS II) is termed as potential electron transfer quantum yield of PS II. This parameter, besides expressing the potential for PS II photochemistry, is commonly used to investigate the onset and recovery of stress situations due to its sensitivity to plant stress (Beer et al., 2001). When measured in ambient light, the ratio of the fluorescence parameter termed as the effective quantum yield for electron transfer by PS II. Further, photosynthesis rates under ambient conditions can also be efficiently measured based on fluorescence parameters by comparing the estimated photosynthetic electron transport rates (ETRs), with rates of O2 evolution during photosynthesis (Sundberg et al., 1997).
Seagrass ecosystems of India mostly exist in shallow waters and cover a total area of ∼517 km2 (Geevarghese et al., 2018) with a high potential to capture atmospheric CO2 through photosynthesis. Degradation of seagrass meadows by enhanced water column turbidity and proliferation of epiphytic macroalgae in certain areas (Kattumavadi in particular) along this coast has been reported by Purvaja et al. (2018). However, no quantitative information on existing seagrass health and the extent of ecosystem degradation are available along the coast. Further, a strong dependence of net ecosystem productivity over available light and dissolved CO2 was recorded in these meadows (Ganguly et al., 2017). Detailed understanding of the capacity of individual seagrass species to reduce their light requirement by photo-acclimatory efficiency of the green leaves (for optimized carbon balance) is therefore necessary. Evaluation of oxygen and inorganic carbon exchange during the photosynthetic processes are also important to determine the efficiency of the eco-physical process. Studies with regard to light response of different seagrass species either in situ or in vitro conditions are limited or non-existent in Indian coastal waters, which regularly experience strong seasonality and varying degree of anthropogenic influences. Further, an understanding of the photo-acclimatory efficiency and photosynthetic responses of these important marine macrophytes under future environmental changes could be useful in predicting their future sustainability. In the present study, differential photosynthetic responses of three tropical seagrass species (Cymodocea serrulata, Thalassia hemprichii, and Enhalus acoroides) to the solar irradiance, were studied along with the photosynthetic oxygen production, under natural conditions. Considering the variations in distribution pattern and species morphology, it was hypothesized that E. acoroides would show higher photosynthetic activity compared to the other two species. Additionally, it was hypothesized that changes in ambient water quality, would significantly influence the photosynthetic performance of these tropical seagrass species. It is expected that these results would be useful in predicting biological responses of tropical seagrass communities and in providing management strategies under future changes in the coastal environment.
Study Area
Seagrass along the Tamil Nadu coast, India, is represented by 14 seagrass species (Purvaja et al., 2018) and contribute more than 77% of the total seagrass cover in the country (Geevarghese et al., 2018). Palk Bay, located in Tamil Nadu, adjacent to the Gulf of Mannar and surrounded by Vaigai basin in the north west (Figure 1), is a shallow flat basin (mean depth 9 m) along the South East coast of India covering an area of ∼13500 km2 (Jyothibabu et al., 2014). The bay experiences stable climatic conditions with marginal spatio-temporal variability in terms of water quality and extremes in air/water temperature during dry season (February–September; Sridhar et al., 2008). The bay is known for diverse biological resources with luxuriant seagrass growth where the water column is shallow, and the bottom substratum is sandy or silty (Sridhar et al., 2010; Manikandan et al., 2011). The total seagrass cover surveyed during 2014 in Palk Bay waters was estimated to be ∼316 km2 (Geevarghese et al., 2018). The species diversity of seagrass in Palk Bay is reported to be highest in India (Parthasarathy et al., 1991) with the highest and lowest seagrass growth and ecosystem productivity reported during monsoon and post-monsoon (July–November; Govindasamy et al., 2013). The most dominant species occurring in this region are Cymodocea serrulata and Syringodium isoetifolium (Govindasamy and Arulpriya, 2011). In addition, beds of Halodule pinifolia, Enhalus sp., Thalassia sp., Cymodocea rotundata have also been sparsely distributed in various parts of the Bay (Purvaja et al., 2018). This region experiences semidiurnal tide, with spring tidal range of ∼0.6 m and neap tidal range of ∼0.16 m (Gowthaman et al., 2013). Tidal influence is limited, however, the diurnal changes in roughness of the surface water are highly influenced by wind in these shallow waters. The present study was conducted at three mono-species beds with a thick cover of C. serrulata (09°17′ 29″ N,79°07′44″E), T. hemprichii (10°05′44″N, 79°14′10.88″E) and E. acoroides (09°30′ 07″N,78°56′ 13″E), respectively. The average water column depth for both C. serrulata and T. hemprichii was ∼ 2 m, and for E. acoroides it was ∼1.5 m. Mature seagrass leaves, free of epiphytes of all the three studied species were selected for photosynthetic measurements to ensure consistency in the observed responses. The northern part of the bay with macroalgal canopy and dense epiphyte cover (Purvaja et al., 2018) was avoided and healthy seagrass meadows (around 2 m depth) parallel to the shoreline was selected (with no evidence of epiphytic growth) for the study.
Methodology
Palk Bay annually experiences two major seasons, northeast monsoon comprising the wet season (October–January) and southwest monsoon, the dry season (February–September). During dry season, the bay water experiences surface current in the clockwise direction (range 0.05–0.3 m/s), and reverses during the wet season (unpublished data). In order to understand photosynthetic responses to light availability of the three dominant seagrass species in Palk Bay, three hydrologically stable stations (annual salinity range 31.08–34.96) with thick mono-species cover of C. serrulata (Station 1), T. hemprichii (Station 2), and E. acoroides (Station 3) were selected (Figure 1). Seasonal fluctuations in the extent of seagrass cover was not found prominent for Palk Bay region (Geevarghese et al., 2018). In situ measurements were carried out at each station characterized by healthy seagrass cover, from morning 6 am to 11 am in July, 2017 (dry season), under low to moderate light availability. As the day progressed, enhanced light intensity, higher wind speed and rough surface conditions caused unsuitable conditions for in situ photosynthetic measurements in the shallow aquatic environment.
Seagrass leaf length and width at all the sampling locations were measured in situ. Air temperature and wind speed was measured at 15-minute intervals with automatic weather monitor stations (i.e., DAVIS 7440) mounted on the anchored boats at the study location. Photosynthesis was measured by chlorophyll fluorescence using a Diving-PAM underwater fluorometer (Walz, Effeltrich, Germany). Photosynthetically active radiation (PAR) was estimated in parallel to the fluorometer using a PAR sensor attached with the Diving-PAM. Healthy, clean seagrass leaves (five replicates for each sampling) from independent shoots were carefully placed directly on the tip of the fluorometer fiber optic using the leaf holder. The exposure time at each irradiance was 15 s, separated by a saturating flash (0.8 s, ∼6,000 mmol m–2 s–1). Photosynthetic parameters (e.g., effective quantum yield of PS II, ETR) were determined at ambient conditions, from each sample. The effective quantum yield (Y) of PS II, ΔF/Fm’, is one of the most useful indicators of stress conditions for seagrass and therefore, is extensively used in different studies depicting a wide range of stress situations (Silva et al., 2009). It was measured using the following equation:
where Fv = variable fluorescence and Fm’ = maximal fluorescence of dark-acclimated samples (for dark acclimation, seagrass leaves were placed for 5 min (Necchi, 2005) directly on the tip of the fiber optic using the supplied dark leaf clip), F is the normal fluorescence in the ambient light.
ETR was calculated as:
where PAR is the actinic irradiance in μmol m–2 s–1. Further, to determine the within-shoot variability in AF, measurements were conducted with respect to difference in seagrass leaf lengths (matured leaves) based on their position in the shoot. These AF differed from species to another and from the standard value (0.85), and were therefore used to calculate the absolute electron-transport rates of the respective species.
Absorption factor for individual seagrass species was measured by placing each leaf (according to the length) over the PAR quantum sensor of the fluorometer, irradiating perpendicularly at a fixed distance with the instrument’s optic fiber. Three measurements were taken for each leaf, at the base, the middle and the leaf tip, respectively. The mean value was then calculated. Irradiance reaching the sensor with and without leaf was recorded, and AF was calculated as the percentage of light absorbed by the leaf. The use of this specific AF instead of the instrument predefined and commonly used value of 0.84, allowed for the calculation of absolute ETR (μmol electrons m2s–1) instead of relative ETRs.
Photosynthesis-irradiance (P-I) curves, as rapid light curves (RLCs) (White and Critchley, 1999), consisted of the fluorescence responses to nine increasing irradiances from 76 to 2334 mmol m–2 s–1 (76, 226, 310, 424, 545, 759, 996, 1630, and 2334), using the “light curve” option of the Diving-PAM. RLCs were calculated assuming a fixed distance between samples and actinic source and the average of at least nine RLCs was obtained for each species at the same location. Each of these RLCs was performed on healthy and individual seagrass that had not been measured previously. Ralph et al. (1998) showed that so-called rapid light curves could adequately identify adaptations of various species to their natural irradiance. It is pertinent to mention that RLCs are used as an effective tool for detecting the operational photo-physiology of seagrass at the time of measurements. It provides information on the dissipation of energy from limiting levels of irradiance through to saturating levels and can act as a proxy for the electron transport rate through PS II (Burdett et al., 2012). RLCs differ from traditional P-I curves in that they measure the actual, rather than the optimal, photosynthetic state, as steady state is not achieved during each light step duration (Ralph and Gademann, 2005; Perkins et al., 2010). Curves of PI were generated using the light versus ETR data for all the three studied species. Photosynthetic efficiency α was determined by linear fitting using the first three points of the ETR versus irradiance curve (Conde-Álvarez et al., 2002). The maximum rate of photosynthetic electron transport or photosynthetic capacity (ETRmax) was derived by fitting the RLCs to the double exponential decay function (Platt et al., 1980), using a least-squares non-linear curve-fitting algorithm:
where Ps value is a scaling factor which was in turn used to calculate ETRmax, α (the initial slope of the light limited relationship) and β (down-regulation)
This lower limit of saturating radiation (Ik, μmol quanta m–2 s–1) was calculated as Ik = ETRmax/α.
Simultaneous measurement of water quality parameters viz. salinity, pH (NBS scale), oxidation-reduction potential (ORP), dissolved oxygen (DO) and its saturation state (LDO%), and chlorophyll-a (Chl-a) were carried out, ∼ 0.1 m above the seagrass canopy at 15-minute intervals using pre-calibrated multiparametric Hydrolabsonde. A LI-COR underwater cosine sensor (Li192SA) attached to the sonde was used to measure irradiance at intervals during vertical descent and ascent through the water column, and the light attenuation coefficient Kd (expressed in m–1) was calculated following the methodology by Kirk (1994).
Gross photosynthetic O2 evolution was estimated and was compared with the absolute ETRs (Beer et al., 2001) calculated by measuring the absorption factor for individual species (Durako, 2007). Several authors have earlier reported that aquatic vegetation shows good agreement between ETR and O2 evolution within the moderate irradiance under the in situ conditions (Longstaff et al., 2002; Beer and Axelsson, 2004; Figueroa et al., 2013). For the measurement of total alkalinity (TA), water samples were collected at every 30-minute interval and potentiometric titration was performed using Auto titrator (Mettler Toledo-Compact Titrator G20). The dissolved inorganic carbon (DIC; sum of CO32–, HCO3–and aqueous CO2 concentration), and pCO2, were calculated with the CO2SYS program (version 14) using in situ pH (NBS), TA, salinity, temperature, and nutrients (phosphate and silicate) data (Lewis and Wallace, 1998). The CO2 dissociation constants given by Millero et al. (2006) for the 0 to 40 salinity range were used for this computation.
Statistical Analysis
In order to examine the variations in water quality (PAR, pH, DO, DIC, pCO2, Chl-a) and photosynthetic parameter (Y) with time among the studied species, one-way ANOVA with post hoc Tukey HSD test was performed using SPSS 20.0 (IBM Corporation, Armonk, NY, United States). P < 0.05 demonstrated significant difference. Prior to all analyses, normality of data was tested 223 using the Shapiro–Wilk test and examination of frequency histograms. In addition, Levene’s test was performed to assess the homogeneity of variances without any transformation. Water quality and photosynthetic parameters were observed to be normally distributed and exhibited homogeneity of variances (p > 0.05).
All data are presented as mean (±standard deviation). In order to assess the biogeochemical dependence of Y on the ambient water quality parameters stepwise Multiple Regression Analysis was performed. Water temperature, salinity, DIC and pCO2, were considered as independent variables. All the statistical tests were performed using Minitab 16 software (except ANOVA post hoc Tukey HSD test), whereas graphs and calculations from PI curve parameters were made using Microsoft Excel.
Results
Hydrological Conditions of the Sampling Sites
Morphometric characteristics of the three seagrass species measured (leaf length, width, etc.) at the study locations are given in Table 1. The mean environmental conditions at the three different stations is given in Supplementary Table S1. The water temperature showed typical summer character as salinity remained near oceanic levels and varied significantly (p < 0.05) throughout the day (30.7 and 32.7). In situ light availability (PAR) ranged between 60 and 545 μmol m–2 s–1 for the three selected species. Wind direction (160–280°) similar to southwest monsoon with a significant increase in wind speed after 9 am was recorded in all the three locations. As expected, light attenuation coefficient (kd) showed an increasing trend from morning to noon and the mean value was significantly higher for E. acoroides than the other two species. Although the available PAR did not show any significant difference (p > 0.05) among the three sampling stations, the spatial variations in kd during the study was significant (p < 0.05). Among other measured parameters seagrass photosynthetic efficiency, DIC and water column chl-a varied significantly among the species.
chlorophyll-a concentrations indicated mostly oligotrophic conditions of the waters with significantly higher (p < 0.001) concentrations in the E. acoroides meadows. The water column was mostly saturated with respect to O2 expect for C. serrulata where the water showed minor CO2 super saturation with respect to atmospheric concentrations. As the day progressed, DIC and pCO2 showed consistent decrease, contrary to O2 saturation in these shallow waters. During the study a decrease of 6, 4, and 7% from the initial DIC corresponded to a decrease of 47, 27, and 57% of the initial pCO2 for C. serrulata, T. hemprichii and E. acoroides, respectively. The in situ changes in DO, DIC, pH and pCO2, above the seagrass canopy was prominent (Figure 2) and their trends under ambient conditions were found statistically significant for the individual species (p < 0.05). Changes in available photosynthetic active radiation and photosynthetic efficiency under ambient conditions during the study were also plotted with time (Figure 2). Results indicated the possible impact of light depended seagrass photosynthesis on the water quality of the shallow meadows.
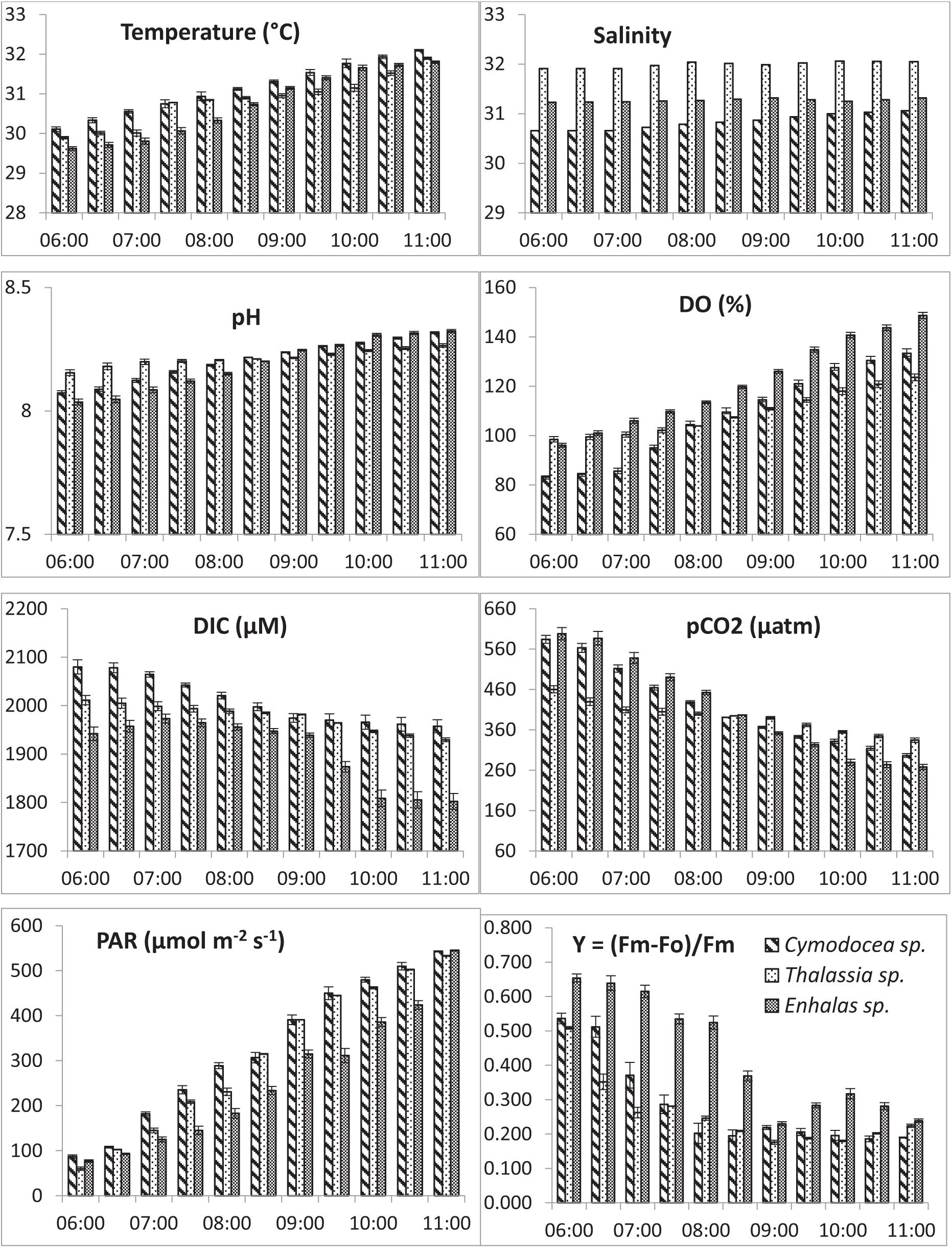
Figure 2. Hourly variations in water quality; Temperature, salinity, pH, dissolved Oxygen saturation (DO%), Dissolved Inorganic Carbon (DIC), partial pressure of carbon dioxide (pCO2), available Photosynthetically Active Radiation (PAR) and effective quantum yield (Y) for the three studied species in Palk bay.
Light Response of Seagrass
Results on photosynthetic responses of the three seagrass species are given in Table 2. The AF for C. serrulata, T. hemprichii, and E. acoroides were moderately lower than the default AF of 0.85. Based on both in situ light conditions and fluorescence yields or rapid light curves ETRs were calculated for three species (Figure 3), which provided similar results for the individual species at low to moderate irradiance levels. However, significant differences in photosynthetic parameters among the three species were recorded (Table 2). In the present study, as the day progressed from early morning to noon, Y, representing the percent of light energy absorbed by the green leaves (Deyanova et al., 2017), showed a gradual decrease (p = 0.014, Table 3) along with the changes of other water quality parameters for all three seagrasses studied.

Table 2. The absorption factor (AF), photosynthetic parameters derived from the rapid light curve and O2/ ETR ratios under in situ light irradiance for three studied seagrass species
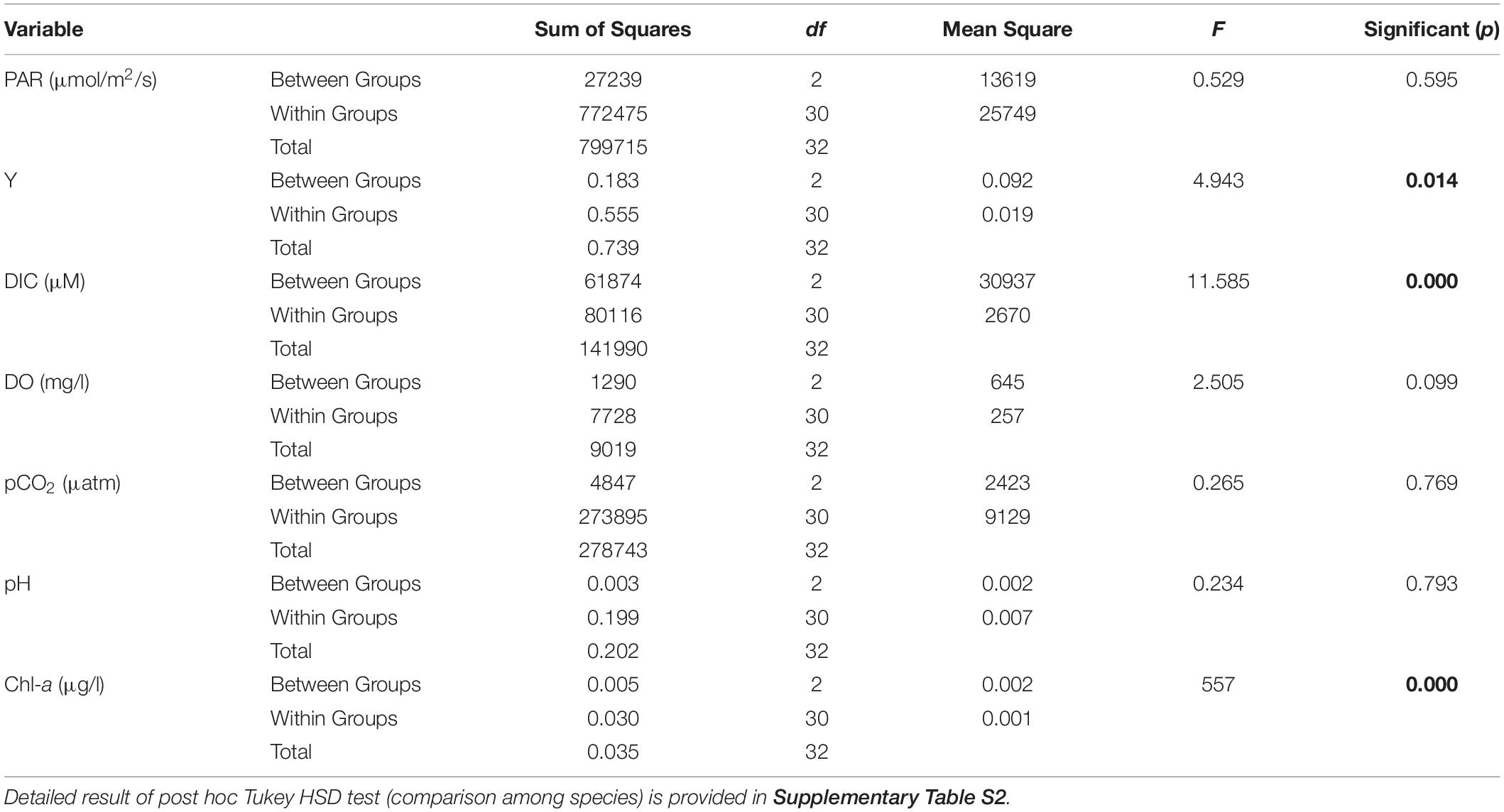
Table 3. Results of One-Way Repeated Measures ANOVA between selected parameters [Photosynthetically Active Radiation (PAR), effective quantum yield (y), dissolved inorganic carbon (DIC), Dissolved oxygen (DO), partial pressure of CO2 (pCO2), pH and Chl-a] recorded from (1) Cymodocea serrulata, (2) Thalassia hemprichii, (3) Enhalus acoroides (values in bold indicate statistically significant results).
A reduction in the Y is typically indicative of a decline in PS II activity (or photo-inhibition). Changes in Y was proportional with DIC and pCO2 of water, whereas the reverse was recorded with water temperature, DO and availability of PAR. The Post Hoc test (Tukey HSD) revealed (Supplementary Table S2) that Y, DIC and water column Chl-a for Enhalus acoroides varied significantly (Table 3) from the other two species. Temporal changes in other water quality parameters showed no variation between the studied species. Maximum rate of photosynthetic electron transport (ETRmax) was recorded for E. acoroides, followed by T. hemprichii and C. serrulata (p < 0.001). The minimum saturating irradiance (Ik) was highest in E. acoroides. However, the light limited photosynthetic efficiency (α) was the highest for T. hemprichii, followed by C. serrulata and E. acoroides (p < 0.001). Under ambient water quality conditions, the highest down regulation of the photosynthetic process, under enhanced light conditions was recorded for E. acoroides (11 ± 2 × 10–3) followed by T. hemprichii (5 ± 1 × 10–3) and C. serrulata (4 ± 1 × 10–3).
It was observed from stepwise multiple regression analysis that variability of y (effective quantum yield (Y) of PS II) in the seagrass meadows during day can significantly be explained by ambient temperature (19.8%) and pCO2 (66.5%) (Table 4) and the regression equation is:
Photosynthetic O2 evolution at three different locations in each of the seagrass species were compared with the corresponding ETR values under the ambient light conditions (low to moderate irradiance). Significant linear relationship with varying degree of variance between in situ O2 evolution and ETR were recorded for all the three species. The molar ratio between photosynthetic O2 evolution and ETR for C. serrulata was maximum (0.23) among the three species (Table 2) and was close to the theoretical stoichiometric ratio of 0.25 (i.e. 4 mol electrons are used to reduce 1 mol CO2; and 1 mol of O2 is released in the process). In contrast, the ratio for T. hemprichii and E. acoroides were recorded to be relatively lower than 0.25.
Discussion
Globally, several studies have been successfully conducted to depict the carbon regulatory mechanism in seagrass ecosystems, including a few from the Indian subcontinent (Kaladharan and Raj, 1989; Johnson and Johnstone, 1995; Singh et al., 2015; Ganguly et al., 2017). However, studies on the photosynthetic responses against available irradiance for individual species within this geographical area remained untouched. The present work is a pioneering attempt to establish the impact of light limitation on the sub-tidal distribution of three seagrass species in Indian coastal waters. Among the three studied species the highest photosynthetic activity was recorded for E. acoroides followed by T. hemprichii and C. serrulata. Identical trends of effective quantum yield (Y) of PS II with dissolved inorganic carbon and pCO2 in ambient water indicated similar physiological acclimatization, in response to changes in carbonate chemistry for the all the three seagrass species.
The results could be useful to assess the potential impacts of altered light availability caused by changes in water turbidity on seagrass growth, survival and associated water chemistry in the future. Manikandan et al. (2011) reported a decrease in the shoot density and biomass of 14 species of seagrass from near-shore, to off-shore zone of Palk Bay region, largely due to increasing water depth, resulting in limited light penetration. Increase in water column turbidity and high epiphyte loads may further impact the seagrass biomass by reducing available light conditions in these meadows.
Comparison of photosynthetic performance among the three tropical seagrass species was made under similar environmental conditions. The results suggested that among the three studied species, T. hemprichii is highly adapted in the low light conditions followed by C. serrulata. Additionally, the lowest Ik derived for T. hemprichii indicated its higher photosynthetic efficiency under the ambient condition. In contrast, E. acoroides, with low photosynthetic efficiency and higher ERTmax and Ik, suggested that it is adapted to high light conditions. Further, E. acoroides, with relatively higher leaf thickness, higher electron transport rates showed higher energy dissipation capacity through down regulation under light stress conditions as observed by Schumann et al. (2017). Down regulations of photosynthesis allow plants to withstand and utilize the rapidly changing light environment, either by decreasing the absorption of light energy or by providing alternative energy sinks through a complex group of rapid responses (MacIntyre et al., 2000). During low tide, E. acoroides., often gets exposed to direct sunlight and receives high irradiance at several places at Palk Bay. The present study confirms that frequent exposure to the light stress makes them better acclimated to high light condition with better photo-protective response.
The estimated Ik for C. serrulata derived from the light curve in the present study was comparable with those reported for Cymodocea nodosa (149.2 ± 45.3 μmol m–2 s–1) from tropical/sub-tropical waters (Lee et al., 2007). The observed Ik values were significantly higher than those reported for the leaf segment of tropical Thalassia testudinum (98.5 ± 11.5 μmol m–2 s–1; Lee et al., 2007). The highest potential quantum yield recorded during low light and high pCO2 conditions (early morning) for all the three species indicated reduced light requirements to achieve the metabolic balances (Schwarz et al., 2000; Long et al., 2004). These results are in agreement with those reported by Ow et al. (2015) who observed greater CO2 dependence of seagrass photosynthesis under low light conditions. Photobiological fitness of seagrass vegetation (mostly C3 plants) under CO2 enriched conditions enhances carboxylation capacity of Rubisco and subsequent photosynthetic activities (Repolho et al., 2017). However, extended shading, due to enhanced water column turbidity can cause substantial reductions in seagrass areal productivity and associated daily carbon balance (Deyanova et al., 2017). A likely increase in global mean sea level of around 50 cm has been projected by the end of the century (Jackson and Jevrejeva, 2016; Le Bars et al., 2017). Based on the elevation in the mean level of the ocean surface, increase in the tidal variability around that mean and local geomorphology, the net rise in the regional sea level will primarily increase the depth of water. A subsequent reduction in available light to the benthic community could significantly impair photosynthetic efficiencies of seagrass species (York et al., 2013). The greatest impact of this alteration in light availability will be seen on the seagrasses, located in the coastal edges (deeper waters) where, the existing light availability only just meets the minimum light requirements (Abal and Dennison, 1996). Similarly, it is expected that, with an increase in depth, seagrass species in the shallow regions, characterized by low light penetration (mostly due to high turbidity), could experience higher oxidative stress (Silva et al., 2013). Using the measured light attenuation co-efficient (Kd) measured from the shallow waters of Palk bay, in the equations given by Gallegos and Moore (2000), it is estimated that an increase of 50 cm depth, could reduce available light availability by 15, 10 and 10% in the existing E. acoroides, C. serrulata, T. hemprichii beds, respectively. These results further indicated that future increases in attenuation of light (Kd), caused by the greater phytoplankton abundance, higher riverine inputs of suspended particulate matter and colored dissolved organic matter could substantially alter seagrass growth and distribution in the shallow coastal waters. Seagrass species that are able to adapt better in low light regimes will have reduced competition in the altered coastal environment.
Significant dependence of Y on the ambient temperature (<33°C) and pCO2 suggested that enhanced CO2 availability will improve the photosynthetic performance of the studied seagrass (Borum et al., 2016). Collier et al. (2017) showed that tropical species, including C. serrulata are generally well adapted within their distributional range with a high thermal optimum (35°C on average) for gross photosynthesis. At all three sampling locations, seagrass species showed predictable response with a decrease in ΔF/Fm’ ratio to daytime increase in ambient PAR levels (Beer et al., 1998; Kahn et al., 2013). In general reduction in ΔF/Fm’ ratios under enhanced light conditions are considered as natural photo-protective response to PS II photo-damage (Major and Dunton, 2002; Durako et al., 2003). Under elevated light (illumination) conditions plant leaves with greater adaptivity, show high photosynthetic capacity and active photo-protective mechanisms (Demmig-Adams et al., 1999; Park et al., 2016). However, it is difficult in the field conditions to isolate the impacts of heat stress from those caused by changes in light availability on the metabolic activities of seagrass measured by changes in dissolved oxygen concentration in water column (Pedersen et al., 2016).
Linear relationship between photosynthetic O2 evolution and ETR for C. serrulata was close to the values derived theoretically, throughout the entire range of available irradiance. At sub-saturating irradiances several aquatic plants have shown an O2/ETR ratio close to 0.25 (Nielsen and Nielsen, 2008). At high light intensities, the linear relationship between ETR and O2 evolution can deteriorate, primarily due to various regulation processes that act as sink for electrons increase (Beer et al., 1998; Beer and Bjork, 2000; Silva and Santos, 2004). Further, during the photosynthetic rate measurements O2/ETR deviates significantly from the theoretical value below a threshold value of Y which further depends on the irradiance relative to the growth conditions of the aquatic plant, rather than the absolute irradiance (Beer and Axelsson, 2004). Relatively lower molar ratios between photosynthetic O2 evolution and ETR for T. hemprichii and, E. acoroides is likely due to difference in physiology of the seagrass and can be attributed to light dependence and alternative electron pathways that consume additional O2. These processes include photo-protective cyclic electron flow around PS II (Prasil et al., 1996; Lavaud et al., 2002), the Mehler reaction (O2 reduction at PS I) (Schreiber et al., 1995), photorespiration and chlororespiration (Beardall et al., 2003) etc. Light-enhanced dark respiration at high irradiances could also result in increased mitochondrial O2 consumption (Reddy et al., 1991; Beardall et al., 1994; Lis and Attaia, 2003; Napoleon and Claquin, 2012). Additionally, as the day progressed, a consistent increase in pH and decrease in DIC and pCO2 was recorded in the shallow coastal waters of Palk Bay. With increasing irradiance, some species showed decreased gross O2 evolution and ETR ratio within a range of low to moderate irradiances. This response by several plants including seagrass species is perhaps due to lack of explicit CO2 concentrating mechanisms (Beer et al., 1998; Beer and Bjork, 2000). This decrease substantially reduces net O2 production, indicating a possible increase in photorespiration rates. Enhanced photorespiration resulting a significant decline in seagrass primary production was also reported by Buapet et al. (2013) under natural carbon limitations caused by in situ photosynthetic activities. Other environmental conditions such as temperature, nutrients (which vary with various bio-physical modifications in water quality), intra-specific competition among shoots (Rose and Dawes, 1999) could also regulated seagrass productivity in these shallow waters. Further, the photosynthetic response due to increase of surface water pCO2 (ocean acidification) could have variable impact on seagrasses and the associated epiphyte (especially crustose coralline algae) cover (Cox et al., 2015). Reduction of these coralline algae could enhance the light availability and subsequent photosynthetic activities by these tropical seagrasses. It is expected that an enhancement in physiological competitiveness among all these seagrass species, capable of utilizing high CO2 conditions (Repolho et al., 2017), would determine their future distribution, productivity, and community composition. In order to understand future survival of tropical seagrass species, in-depth studies on adaptive photosynthetic responses under changing environment is essential.
Conclusion
In situ light responses of three tropical seagrass species in Indian coastal waters have been studied for the first time in association with their net ecosystem productivity. Among the studied seagrass species T. hemprichii showed the highest photosynthetic efficiency, however, highest light saturation was recorded for E. acoroides. The photosynthetic O2 evolution to ETR ratio was found highest and close to the theoretical stoichiometric ratio for C. serrulata. Results indicated that tropical seagrasses modify their photosynthetic performance in different proportion in response to the availability of in situ light conditions. Water temperature and pCO2 were found to be the two most significant factors that can explain the variability of the effective quantum yield pf PS II under the ambient conditions for the studied seagrass species. Future alterations in environmental conditions (natural - e.g., water temperature, wind direction, speed, rainfall, light availability and anthropogenic- e.g., ocean acidification, algal bloom, suspended particle etc.) may significantly alter the photosynthetic performance of tropical seagrasses.
Data Availability Statement
The raw data supporting the conclusions of this article will be made available by the authors, without undue reservation, to any qualified researcher.
Author Contributions
RP was involved in conceptualization, sampling, and review of the manuscript. DG was involved in the sampling, sample analysis, data processing, and manuscript preparation. GH was involved in the underwater sample collection in the field (Diving) and preparation of the maps, graphs, and tables used in the manuscript. KA was involved in sample collection and measurement of water quality parameters. RR supervised the work and involved in the review and editing of the manuscript. All authors contributed to the article and approved the submitted version.
Conflict of Interest
The authors declare that the research was conducted in the absence of any commercial or financial relationships that could be construed as a potential conflict of interest.
Acknowledgments
This study was undertaken as part of the in-house research study of NCSCM on “Blue carbon ECosystems: Offsetting Carbon Emissions (BECOCE)” studies (Sanction Number IR12008). We acknowledge the financial support of the Ministry of Environment, Forest and Climate Change, Government of India, and the World Bank under the India ICZM Project (P097985).
Supplementary Material
The Supplementary Material for this article can be found online at: https://www.frontiersin.org/articles/10.3389/feart.2020.467548/full#supplementary-material
References
Abal, E. G., and Dennison, W. C. (1996). Seagrass depth range and water quality in southern Moreton bay, Queensland, Australia. Mar. Freshw. Res. 47, 763–771. doi: 10.1071/mf9960763
Alexandre, A., Silva, J., Buapet, P., Bjork, M., and Santos, R. (2012). Effects of CO2 enrichment on photosynthesis, growth, and nitrogen metabolism of the seagrass Zostera noltii. Ecol. Evol. 2, 2625–2635. doi: 10.1002/ece3.333
Beardall, J., Burger-Wiersma, T., Rijkeboer, M., Sukenik, A., Lemoalle, J., Dubinsky, Z., et al. (1994). Studies on enhanced post-illumination respiration in microalgae. J. Plankton Res. 16, 1401–1410. doi: 10.1093/plankt/16.10.1401
Beardall, J., Quigg, A., and Raven, J. A. (2003). “Oxygen consumption: photorespiration and chlororespiration,” in Photosynthesis in Algae, of Advances in photosynthesis and respiration, Vol. 14, eds A. W. D. Larkum, S. E. Douglas, and J. A. Raven (Berlin; Dordrecht: Springer; Kluwer), 157–181.
Beer, S., and Axelsson, L. (2004). Limitations in the use of PAM fluorometry for measuring photosynthetic rates of macroalgae at high irradiances. Eur. J. Phycol. 39, 1–7. doi: 10.1080/0967026032000157138
Beer, S., and Bjork, M. (2000). Measuring rates of photosynthesis of two tropical seagrasses by pulse amplitude modulated (PAM) fluorometry. Aquat. Bot. 66, 69–76. doi: 10.1016/S0304-3770(99)00020-0
Beer, S., Bjork, M., Gademann, R., and Ralph, P. (2001). “Measurements of photosynthetic rates in seagrasses,” in Global, Seagrass Research Methods, eds F. T. Short and R. G. Coles (New York, NY: Elsevier), 183–198.
Beer, S., Vilenkin, B., Weil, A., Veste, M., Susel, L., and Eshel, A. (1998). Measuring photosynthetic rates in seagrasses by pulse amplitude modulated (PAM) fluorometry. Mar. Ecol. Prog. Ser. 174, 293–300. doi: 10.3354/meps174293
Borum, J., Pedersen, O., Kotula, L., Fraser, M. W., Statton, J., Colmer, T. D., et al. (2016). Photosynthetic response to globally increasing CO2 of co-occurring temperate seagrass species. Plant. Cell Environ. 39, 1240–1250. doi: 10.1111/pce.12658
Buapet, P., Rasmusson, L. M., Gullström, M., and Bjork, M. (2013). Photorespiration and carbon limitation determine productivity in temperate seagrasses. PLoS One 8:e83804. doi: 10.1371/journal.pone.0083804
Burdett, H. L., Hennige, S. J., Francis, F. T. Y., and Kamenos, N. A. (2012). The photosynthetic characteristics of red coralline algae, determined using pulse amplitude modulation (PAM) fluorometry. Bot. Mar. 55, 499–509. doi: 10.1515/bot-2012-0135
Campbell, J. E., and Fourqurean, J. W. (2013). Effects of in situ CO2 enrichment on the structural and chemical characteristics of the seagrass Thalassia testudinum. Mar. Biol. 160, 1465–1475. doi: 10.1007/s00227-013-2199-3
Collier, C. J., Ow Yan, X., Langlois, L., Uthicke, S., Johansson, C. L., O’Brien, K. R., et al. (2017). Optimum temperatures for net primary productivity of three tropical seagrass species. Front. Plant Sci. 8:1446. doi: 10.3389/fpls.2017.01446
Conde-Álvarez, R. M., Pérez-Rodríguez, E., Altamirano, M., Nieto, J. M., Abdala, R., Figueroa, F. L., et al. (2002). Photosynthetic performance and pigment content in the aquatic liverwort Riellahelicophylla under natural solar irradiance and solar irradiance without ultraviolet light. Aquat. Bot. 73, 47–61. doi: 10.1016/S0304-3770(02)00007-4
Cox, T. E., Schenone, S., Delille, J., D?az-Castaneda, V., Alliouane, S., Gattuso, J. P., et al. (2015). Effects of ocean acidification on Posidonia oceanica epiphytic community and shoot productivity. J. Ecol. 103, 1594–1609.
Czerny, A. B., and Dunton, K. H. (1995). The effects of in situ light reduction on the growth of two subtropical seagrasses. Estuaries 18, 418–427. doi: 10.2307/1352324
Demmig-Adams, B., Adams, W. I. I. I., Ebbert, V., and Logan, B. A. (1999). “Ecophysiology of the xanthophyll cycle,” in The Photochemistry of Carotenoids, eds H. A. Frank, A. J. Young, G. Britton, and R. J. Cogdell (Netherlands: Kluwer Academic Publishers), 245–269. doi: 10.1007/0-306-48209-6_14
Deyanova, D., Gullstrom, M., Lyimo, L. D., Dahl, M., Hamisi, M. I., Mtolera, M. S., et al. (2017). Contribution of seagrass plants to CO2 capture in a tropical seagrass meadow under experimental disturbance. PLoS One 12:e0181386. doi: 10.1371/journal.pone.0181386
Durako, M. J. (1993). Photosynthetic utilization of CO2 (aq) and HCO3- in Thalassia testudinum (Hydrocharitaceae). Mar. Biol. 115, 373–380. doi: 10.1007/bf00349834
Durako, M. J. (2007). Leaf optical properties and photosynthetic leaf absorptances in several Australian seagrasses. Aquat. Bot. 87, 83–89. doi: 10.1016/j.aquabot.2007.03.005
Durako, M. J., Kunzelman, J. I., Kenworthy, W. J., and Hammerstrom, K. K. (2003). Depth-related variability in the photobiology of two populations of Halophila johnsonii and Halophila decipiens. Mar. Biol. 142, 1219–1228. doi: 10.1007/s00227-003-1038-3
Figueroa, F. L., Jerez, C. G., and Korbee, N. (2013). Use of in vivo chlorophyll fluorescence to estimate photosynthetic activity and biomass productivity in microalgae grown in different culture systems. Latin Am. J. Aquat. Res. 41, 801–819. doi: 10.3856/vol41-issue5-fulltext-1
Gallegos, C. L., and Moore, K. A. (2000). “Factors contributing to water-column light attenuation,” in Chesapeake Bay Submerged Aquatic Vegetation Water Quality and Habitat-Based Requirements and Restoration Targets: A Second Technical Synthesis, ed. R. A. Batiuk (Annapolis, MD: EPA Chesapeake Bay Program), 35–54.
Ganguly, D., Singh, G., Purvaja, R., Paneer, S., Banerjee, K., and Ramesh, R. (2017). Seagrass metabolism and carbon dynamics in a tropical coastal embayment. Ambio 46, 667–679. doi: 10.1007/s13280-017-0916-8
Geevarghese, G. A., Akhil, B., Magesh, G., Krishnan, P., Purvaja, R., and Ramesh, R. (2018). A comprehensive geospatial assessment of seagrass distribution in India. Ocean Coast. Manag. 159, 16–25. doi: 10.1016/j.ocecoaman.2017.10.032
Govindasamy, C., and Arulpriya, M. (2011). Seasonal variation in seagrass biomass in Northern Palk Bay, India. Biodiversity 12, 223–231. doi: 10.1080/14888386.2011.653542
Govindasamy, C., Arulpriya, M., Anantharaj, K., Ruban, P., and Srinivasan, R. (2013). Seasonal variations in seagrass biomass and productivity in Palk Bay, Bay of Bengal, India. Int. J. Biodivers. Conserv. 5, 408–417. doi: 10.5897/IJBC12.132
Gowthaman, R., Sanil Kumar, V., Dwarakish, G. S., Mohan, S. S., Singh, S. M., and Ashok Kumar, K. (2013). Waves in Gulf of Mannar and Palk Bay around Dhanushkodi, Tamil Nadu, India. Curr. Sci. 104, 1431–1435.
Hemminga, M. A., and Duarte, C. M. (2000). Seagrass Ecology, 1st Edn. Cambridge, MA: Cambridge University Press.
Jackson, L. P., and Jevrejeva, S. (2016). A probabilistic approach to 21st century regional sea-level projections using RCP and High-end scenarios. Glob. Planet. Change 146, 179–189. doi: 10.1016/j.gloplacha.2016.10.006
Johnson, P., and Johnstone, R. (1995). Productivity and nutrient dynamics of tropical sea-grass communities in Puttalam Lagoon, Sri Lanka. Ambio 24, 411–417.
Jyothibabu, R., Madhu, N. V., Jagadeesan, L., and Anjusha, A. (2014). Why do satellite imageries show exceptionally high chlorophyll in the Gulf of Mannar and the Palk Bay during the Northeast Monsoon? Environ. Monit. Assess. 186, 7781–7792. doi: 10.1007/s10661-014-3966-4
Kahn, A. E., Beal, J. L., and Durako, M. J. (2013). Diurnal and tidal variability in the photobiology of the seagrass Halophila johnsonii in a riverine versus marine habitat. Estuar. Coasts 36, 430–443. doi: 10.1007/s12237-012-9571-2
Kaladharan, P., and Raj, I. D. (1989). Primary production of seagrass Cymodocea serrulata and its contribution to the productivity of Amini Atoll, Lakshadweep Islands. Indian J. Mar. Sci. 18, 215–216.
Kirk, J. T. O. (1994). Light and Photosynthesis in Aquatic Ecosystems. Cambridge, MA: Cambridge University Press.
Lavaud, J., Rousseau, B., van Gorkom, H. J., and Etienne, A. L. (2002). Influence of the diadinoxanthin pool size on photoprotection in the marine planktonic diatom Phaeodactylum tricornutum. Plant Physiol. 129, 1398–1406. doi: 10.1104/pp.002014
Le Bars, D., Drijfhout, S., and de Vries, H. (2017). A high-end sea level rise probabilistic projection including rapid Antarctic ice sheet mass loss. Environ. Res. Lett. 12:044013. doi: 10.1088/1748-9326/aa6512
Lee, K. S., Park, S. R., and Kim, Y. K. (2007). Effects of irradiance, temperature, and nutrients on growth dynamics of seagrasses: a review. J. Exp. Mar. Biol. Ecol. 350, 144–175. doi: 10.1016/j.jembe.2007.06.016
Lewis, E., and Wallace, D. W. R. (1998). Program Developed for CO2 System Calculations, ORNL/CDIAC-105. Oak Ridge, TN: Carbon Dioxide Information Analysis Center, Oak Ridge National Laboratory, U.S. Department of Energy.
Lis, R., and Attaia, A. (2003). Control of mitochondrial function via photosynthetic redox signals. Photosynth. Res. 79, 133–148. doi: 10.1023/B:PRES.0000015409.14871.68
Long, S. P., Ainsworth, E. A., Rogers, A., and Ort, D. R. (2004). Rising atmospheric carbon dioxide: plants FACE the future. Annu. Rev. Plant Biol. 55, 591–628. doi: 10.1146/annurev.arplant.55.031903.141610
Longstaff, B. J., Kildea, T., Runcie, J. W., Cheshire, A., Dennison, W. C., Hurd, C., et al. (2002). An in situ study of photosynthetic oxygen exchange and electron transport rate in the marine macroalga Ulvalactuca (Chlorophyta). Photosynth. Res. 74, 281–293.
MacIntyre, H. L., Kana, T. M., and Greider, R. J. (2000). The effect of water motion on short-termrates of photosynthesis by marine phytoplankton. Trends Plant Sci. 5, 12–17. doi: 10.1016/s1360-1385(99)01504-6
Major, K. M., and Dunton, K. H. (2002). Variations in light-harvesting characteristics of the seagrass, Thalassia testudinum: evidence for photo-acclimation. J. Exp. Mar. Biol. Ecol. 275, 173–189. doi: 10.1016/s0022-0981(02)00212-5
Manikandan, S., Ganesapandian, S., and Parthiban, K. (2011). Distribution and zonation of seagrasses in the Palk Bay, Southeastern India. J. Fish. Aquat. Sci. 6, 178–185. doi: 10.3923/jfas.2011.101.106
Millero, F. J., Graham, T. B., Huang, F., Bustos-Serrano, H., and Perrot, D. (2006). Dissociation constants of carbonic acid in seawater as a function of salinity and temperature. Mar. Chem. 100, 80–94. doi: 10.1016/j.marchem.2005.12.001
Napoleon, C., and Claquin, P. (2012). Multi-parametric relationships between PAM measurements and carbon incorporation, an in situ approach. PLoS One 7:e40284. doi: 10.1371/journal.pone.0040284
Necchi, O. JR. (2005). Light-related photosynthetic characteristics of lotic macroalgae. Hydrobiologia 525, 139–155. doi: 10.1023/B:HYDR.0000038861.18999.7b
Nielsen, H. D., and Nielsen, S. L. (2008). Evaluation of imaging and conventional PAM as a measure of photosynthesis in thin- and thick-leaved marine macroalgae. Aquat. Biol. 3, 121–131. doi: 10.3354/ab00069
Orth, R. J., Carruthers, T. J., Dennison, W. C., Duarte, C. M., Fourqurean, J. W., Heck, K. L., et al. (2006). A global crisis for seagrass ecosystems. Bioscience 56, 987–996.
Ow, Y. X., Collier, C. J., and Uthicke, S. (2015). Responses of three tropical seagrass species to CO2 enrichment. Mar. Biol. 162, 1005–1017. doi: 10.1007/s00227-015-2644-6
Park, S. R., Kim, S., Kim, Y. K., Kang, C. K., and Lee, K. S. (2016). Photo-acclimatory responses of Zostera marina in the intertidal and subtidal zones. PLoS One 11:e0156214. doi: 10.1371/journal.pone.0156214
Parthasarathy, N., Ravikumar, K., Ganesan, R., and Ramamurthy, K. (1991). Distribution of seagrasses along the coast of Tamil Nadu, Southern India. Aquat. Bot. 40, 145–153. doi: 10.1016/0304-3770(91)90092-J
Pedersen, O., Colmer, T. D., Borum, J., Andrea, Z. P., and Gary, A. K. (2016). Heat stress of two tropical seagrass species during low tides – impact on underwater net photosynthesis, dark respiration and diel in situ internal aeration. New Phytol. 210, 1207–1218. doi: 10.1111/nph.13900
Perkins, R. G., Kromkamp, J. C., Serôdio, J., Lavaud, J., Jesus, B., Mouget, J. L., et al. (2010). “The application of variable chlorophyll fluorescence to microphytobenthic biofilms,” in Chlorophyll a Fluorescence in Aquatic Sciences: Methods and Applications. Developments in Applied Phycology, vol. 4, eds D. Suggett, O. Prášil, and M. Borowitzka (Dordrecht: Springer), 237–275.
Platt, T. G. C. L., Gallegos, C. L., and Harrison, W. G. (1980). Photo-inhibition of photosynthesis in natural assemblages of marine phytoplankton. J. Mar. Res. 38, 687–701.
Prasil, O., Kobler, Z., Berry, J. A., and Falkowski, P. G. (1996). Regular poper Cyclic electron flow around Photosystem II in vivo. Photosynth. Res. 48, 395–410. doi: 10.1007/BF00029472
Purvaja, R., Robin, R. S., Ganguly, D., Hariharan, G., Singh, G., Raghuraman, R., et al. (2018). Seagrass meadows as proxy for assessment of ecosystem health. Ocean Coast. Manag. 159, 34–45. doi: 10.1016/j.ocecoaman.2017.11.026
Ralph, P. J., Durako, M. J., Enríquez, S., Collier, C. J., and Doblin, M. A. (2007). Impact of light limitation on seagrasses. J. Exp. Mar. Biol. Ecol. 350, 176–193. doi: 10.1016/j.jembe.2007.06.017
Ralph, P. J., and Gademann, R. (2005). Rapid light curves: a powerful tool to assess photosynthetic activity. Aquat. Bot. 82, 222–237. doi: 10.1016/j.aquabot.2005.02.006
Ralph, P. J., Gademann, R., and Dennison, W. C. (1998). In situ seagrass photosynthesis measured using a submersible, pulse-amplitude modulated fluorometer. Mar. Biol. 132, 367–373. doi: 10.1007/s002270050403
Reddy, M. M., Vani, T., and Raghavendra, A. S. (1991). Light-enhanced dark respiration in mesophyll protoplasts from leaves of pea. Plant Physiol. 96, 1368–1371. doi: 10.1104/pp.96.4.1368
Repolho, T., Duarte, B., Dionísio, G., Jose, R. P., Ines, R., Rui, R., et al. (2017). Seagrass ecophysiological performance under ocean warming and acidification. Sci. Rep. 7:41443. doi: 10.1038/srep41443
Rose, C. D., and Dawes, C. J. (1999). Effects of community structure on the seagrass Thalassia testudinum. Mar. Ecol. Prog. Ser. 84, 83–95. doi: 10.3354/meps184083
Schreiber, U., Hormann, H., Asada, K., and Neubauer, C. (1995). “O2-dependent electron flow in spinach chloroplasts: properties and possible regulation of the Mehler-ascorbate peroxidase cycle,” in Photosynthesis: From Light to Biosphere, ed P. Mathis (Dordrecht: Kluwer).
Schubert, N., Freitas, C., Silva, A., Costa, M. M., Barrote, I., Horta, P. A., et al. (2018). Photo-acclimation strategies in northeastern Atlantic seagrasses: Integrating responses across plant organizational levels. Sci. Rep. 8:14825. doi: 10.1038/s41598-018-33259-4
Schumann, T., Paul, S., Melzer, M., Dörmann, P., and Jahns, P. (2017). Plant growth under natural light conditions provides highly flexible short-term acclimation properties toward high light stress. Front. Plant Sci. 8:681. doi: 10.3389/fpls.2017.00681
Schwarz, A. M., Bjork, M., Buluda, T., Mtolera, M., and Beer, S. (2000). Photosynthetic utilisation of carbon and light by two tropical seagrass species as measured in situ. Mar. Biol. 137, 755–761. doi: 10.1007/s002270000433
Silva, J., Barrote, I., Costa, M. M., Albano, S., and Santos, R. (2013). Physiological responses of Zostera marina and Cymodocea nodosa to light-limitation stress. PLoS One 8:e81058. doi: 10.1371/journal.pone.0081058
Silva, J., and Santos, R. (2004). Can chlorophyll fluorescence be used to estimate photosynthetic production in the seagrass Zostera noltii? J. Exp. Mar. Biol. Ecol. 307, 207–216. doi: 10.1016/j.jembe.2004.02.009
Silva, J., Sharon, Y., Santos, R., and Beer, S. (2009). Measuring seagrass photosynthesis: methods and applications. Aquat. Biol. 7, 127–141. doi: 10.3354/ab00173
Singh, G., Ganguly, D., Paneer Selvam, A., Kakolee, K., Purvaja, R., and Ramesh, R. (2015). Seagrass ecosystem and climate change: an Indian perspective. J. Clim. Change 1, 67–74. doi: 10.3233/jcc-150005
Sridhar, R., Thangaradjou, T., and Kannan, L. (2008). Comparative investigation of physico-chemical properties of coral reef and seagrass ecosystems of Palk Bay. Indian J. Mar. Sci. 37, 207–213.
Sridhar, R., Thangaradjou, T., and Kannan, L. (2010). Spatial and temporal variations in phytoplankton in coral reef and seagrass ecosystems of the Palk Bay, Southeast coast of India. J. Environ. Biol. 31, 765–777.
Sundberg, B., Campbell, D., and Palmquist, K. (1997). Predicting CO2 gain and photosynthetic light acclimation from fluorescence yield and quenching in cyano-hchens. Planta 201, 138–145. doi: 10.1007/bf01007698
van Kooten, O., and Snel, J. F. H. (1990). The use of chlorophyll fluorescence nomenclature in plant stress physiology. Photosynth. Res. 25, 147–150. doi: 10.1007/BF00033156
Waycott, M., Duarte, C. M., Carruthers, T. J., Orth, R. J., Dennison, W. C., Olyarnik, S., et al. (2009). Accelerating loss of seagrasses across the globe threatens coastal ecosystems. Proc. Natl. Acad. Sci. U.S.A. 106, 12377–12381. doi: 10.1073/pnas.0905620106
White, A. J., and Critchley, C. (1999). Rapid light curves: a new fluorescence method to assess the state of the photosynthetic apparatus. Photosynth. Res. 59, 63–72. doi: 10.1023/A:1006188004189
York, P. H., Gruber, R. K., Hill, R., Ralph, P. J., Booth, D. J., and Macreadie, P. I. (2013). Physiological and morphological responses of the temperate seagrass Zostera muelleri to multiple stressors: investigating the interactive effects of light and temperature. PLoS One 8:76377. doi: 10.1371/journal.pone.0076377
Keywords: seagrass, pulse amplitude modulated fluorometry, photosynthetic O2 evolution, biogeochemical changes, Palk Bay, India
Citation: Purvaja R, Ganguly D, Hariharan G, Arumugam K and Ramesh R (2020) In situ Photosynthetic Activities and Associated Biogeochemical Changes in Three Tropical Seagrass Species. Front. Earth Sci. 8:467540. doi: 10.3389/feart.2020.467548
Received: 23 April 2019; Accepted: 12 August 2020;
Published: 10 September 2020.
Edited by:
Luiz Drude Lacerda, Federal University of Ceará, BrazilReviewed by:
Zhijian Jiang, South China Sea Institute of Oceanology, Chinese Academy of Sciences, ChinaLuis Ernesto Arruda Bezerra, Instituto de Ciências do Mar, Brazil
Copyright © 2020 Purvaja, Ganguly, Hariharan, Arumugam and Ramesh. This is an open-access article distributed under the terms of the Creative Commons Attribution License (CC BY). The use, distribution or reproduction in other forums is permitted, provided the original author(s) and the copyright owner(s) are credited and that the original publication in this journal is cited, in accordance with accepted academic practice. No use, distribution or reproduction is permitted which does not comply with these terms.
*Correspondence: Ramachandran Purvaja, cHVydmFqYS5yYW1hY2hhbmRyYW5AZ21haWwuY29t