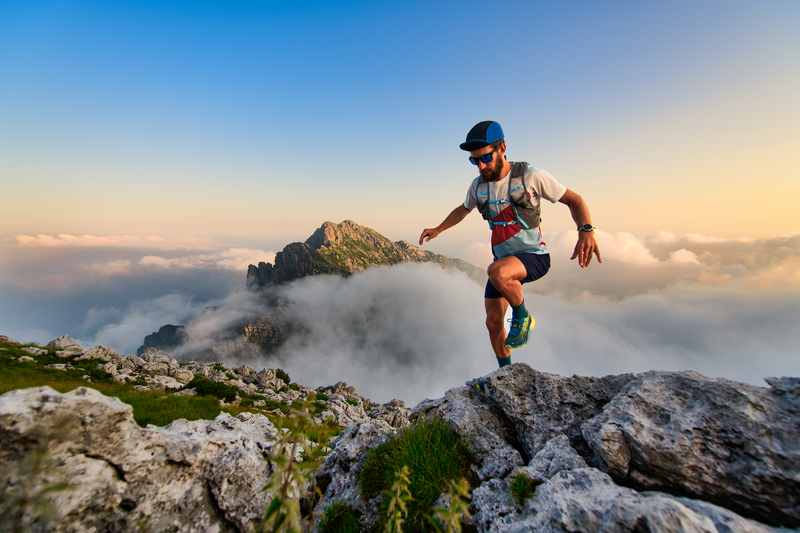
94% of researchers rate our articles as excellent or good
Learn more about the work of our research integrity team to safeguard the quality of each article we publish.
Find out more
ORIGINAL RESEARCH article
Front. Earth Sci. , 02 September 2020
Sec. Soil Processes
Volume 8 - 2020 | https://doi.org/10.3389/feart.2020.00350
This article is part of the Research Topic Legacy Phosphorus in Agriculture: Role of Past Management and Perspectives for the Future View all 12 articles
The forms in which phosphorus (P) accumulates in soils are dependent on management practices, fertilizer sources, and methods of application, which may promote distinct P solubility and plant uptake. We aimed here to evaluate how soil tillage and phosphate fertilization strategies affected soil P fractions over 17 years and to identify best management practices for improving labile P fractions. The experiment was conducted in a very clayey Rhodic Ferralsol (Oxisol) with initially very low P availability, during 17 years under soybean and corn, fertilized with 35 kg P ha–1 year–1. Treatments were two soil management systems (CT-conventional tillage and NT-no-till) and four phosphate fertilization strategies (TSP – triple superphosphate or RRP – reactive rock phosphate, applied to the crop furrow or broadcast). Soil samples were taken at five depth layers, and organic (Po), inorganic (Pi), and total P (Pt) were determined by Hedley’s sequential fractionation. CT resulted in a more homogeneous distribution of Pi fractions throughout the soil profile, while under NT there was a steep depth gradient characterized by Pi accumulation in the fertilizer application zone. NT resulted in accumulation of Pi in more labile fractions and higher accumulation of Po physically protected by aggregation, both compared to CT. Also, under NT with RRP, there was a great accumulation of Pi associated with calcium (HCl Pi) compared to TSP, especially when the fertilizer was broadcast applied. An accumulation of Po down to 20 cm (CT) and 10 cm (NT) was also detected, compared to Cerrado natural soil. NT and RRP positively affected legacy P fractions and can be recommended as strategies to improve P fertilizer use efficiency.
Brazilian Cerrado (savanna) soils originally present low phosphorus (P) availability for cropping, making the addition of phosphate fertilizers mandatory, firstly to build up P availability to acceptable levels before cropping and secondly to maintain the available levels during subsequent growing seasons, through periodic fertilization to replace the P exported by harvesting (Kurihara et al., 2016). The reason is that Cerrado soils have a high solid phase P retention capacity, due to their high Fe and Al sesquioxide constitution, capable of retaining phosphate ions in less labile forms (Fink et al., 2014, 2016a). Consequently, a high fertilizer P input, at a rate above that exported by the crop harvested products, is demanded when establishing the cropping system under these low initial soil test P (STP) soils, to improve P contents and compensate the soil solid phase P sink (de Oliveira et al., 2020a), which is significantly higher than plant demand (Novais et al., 2007). This high P input is usually called corrective P fertilization, and P rates depend on STP and soil buffer capacity (Sousa et al., 2010). Excessive fertilization after the P correction phase or incorrect agricultural practices, as observed for soybean monoculture under conventional tillage (de Oliveira et al., 2019) and/or in the absence of cover crop in the rotation system (Sousa et al., 2010), may promote even more legacy P accumulation, with very low P use efficiency under these production systems.
The soil P dynamics are interdependent and related to an organic and inorganic equilibrium and are under the influence of various factors related to the climate, soil type, and management practice. Regarding climate, the main factors are those related to fertilizer solubilization and SOM mineralization, such as the water regime and temperature (Sharpley, 1985). The soil’s mineralogy and texture define the kind of P chemical reaction after fertilizer addition, as well as the intensity of accumulation under distinct fractions (Sharpley, 1985; Huffman et al., 1996; Rheinheimer and Anghinoni, 2001, 2003; Pavinato et al., 2009). In terms of management practices, soil tillage system (Zheng et al., 2001; Tiecher et al., 2012a,b), the chemical and physical nature of the fertilizer (Zheng et al., 2001; Pavinato et al., 2017), the degree of fertilizer–soil contact (Santos et al., 2008), the rate applied (Zhang et al., 2004; Gatiboni et al., 2007), and the contribution of plant biomass (Tiecher et al., 2012a,b) are the most influential factors. All of these factors, combined, may affect soil legacy P characteristics.
The minimal soil disturbance under no-till (NT) has promoted the accumulation of nutrients in the top surface, especially those of low mobility, such as P from fertilizers and amendment residues (Selles et al., 1997; De Maria et al., 1999; Santos and Tomm, 2003). This phenomenon is intensified when the phosphate fertilizer is broadcast applied and/or slow-release sources are used, such as natural reactive rock phosphates (RRP) (Nunes et al., 2011b). Otherwise, the conventional tillage system (CT) results in the homogenization of P and SOM in the plowed layer (Selles et al., 1997; De Maria et al., 1999; Santos and Tomm, 2003), which exposes P to new adsorption sites (Sousa and Volkweiss, 1987a) and accelerates fertilizer sorption (Miranda et al., 2005), as well as SOM mineralization (Nunes et al., 2011a).
Soil P fractions have been successfully grouped and quantified (although not identified) by sequential chemical extractions, with the widely used Hedley’s P fractionation procedure, by its ability to concomitantly determine Pi and Po fractions (Hedley et al., 1982). The procedure involves subjecting the P in the soil to successive extractions in solutions of increasing extraction strength. Accordingly, each fraction of P relates to a chemical form of P in the soil (Hedley et al., 1982; Cross and Schlesinger, 1995), resulting in estimates of its lability. In this way, this procedure helps assess how management practices may transform P dynamics in the soil.
There are many reasons for adopting the NT system in tropical weathered soils, and many studies have evaluated its benefits in soil biology, physics, and diverse aspects of soil chemistry and fertility. However, the dynamics of P in soil and the relative contribution of its fractions to crop nutrition have not yet been specifically related to the positive benefits of this conservationist system in tropical soils. In addition, the use of rock phosphates has been restricted to corrective fertilization or low-cost fertilizations, due to concerns related to calcium-bound phosphate source efficiency, especially in NT, even though there is a lack of experiments assessing these concerns.
This study aimed to evaluate how soil tillage, phosphate source, and mode of application affected soil P fractions after 17 cropping years in an Oxisol considered highly adsorptive for P. These three management factors are directly related to the different reactions of P in soil and consequently may significantly affect legacy P characteristics, including depth distribution and lability and, ultimately, potential bioavailability to plants. This information is useful for orientating P management decisions in agricultural systems where significant legacy P occurs.
The experiment was conducted at the experimental area of Embrapa Cerrados, Planaltina-DF, Brazil (15°36′S; 47°42′W; 1014 m elevation). The regional climate is classified as Cwa according to Köppen (Alvares et al., 2013), with an average annual precipitation of 1570 mm and temperature ranging between 15.9 and 26.4°C. The soil is classified as Latossolo Vermelho Distrófico according to the Brazilian Soil Classification System (EMBRAPA, 2013), or Rhodic Ferralsol (IUSS Working Group WRB, 2006), containing 64% clay, with a predominance of kaolinite (634 g kg–1), gibbsite (195 g kg–1), and hematite (108 g kg–1) in the clay fraction. Naturally, it is a nutrient-poor soil (Table 1), whose initial P content in the 0–20 cm layer (0.9 mg kg–1; Mehlich-1) is very low (Sousa and Lobato, 2004). The original vegetation was Cerrado, with smooth undulating relief. For experiment establishment, all deficiencies besides P were corrected with the application of dolomitic limestone to increase the base saturation to 50%, agricultural phosphogypsum (3,000 kg ha–1), potassium (42 kg K ha–1), and micronutrients to obtain high annual crop yields, according to recommendations for the region (Sousa and Lobato, 2004). According to these authors, phosphate fertilizer recommendation, for the clay content and initial Mehlich-1 P levels found in the experimental area, would be 183 kg P ha–1 as corrective fertilization plus 35 kg ha–1 as maintenance fertilization.
Table 1. Soil chemical parametersa (0–20 cm layer) in two moments prior to the experiment establishment, before and after liming.
A randomized split-plot block design was used, with two soil management systems, CT and NT, as main plots and four strategies of phosphate fertilization management, combining two sources of P (TSP and RRP) and two placement methods (crop furrow and broadcast application) as subplots. The trial, therefore, consisted of eight treatments, with three replicates, all fertilized annually with 35 kg ha–1 of total P. This P dose was chosen so that it would allow a small but continuous soil P stock gain according to the expected yields. Plots measured 16 × 8 m (128 m2) and were split into subplots of 8 × 4 m (32 m2). In the center of each subplot, a useable area of 15 m2 for soybean and 12 m2 for maize was delimited for harvest procedures.
The experiment was initiated in the 1994/95 season, with the cultivation of soybean/corn as main (summer) crops in the following chronological order: soybean for the first nine years, corn in the 10th and 11th years, and then rotation of soybean and corn until the 17th crop (corn). After 2003 (9th soybean cultivation), millet (Pennisetum glaucum) was cultivated as a winter cover crop, with fertilization of 45 kg ha–1 of N in the form of urea. Plots under CT were plowed annually with a disk plow (to a depth of up to 20 cm) and harrow leveled before sowing the summer crop. Plots under NT were always directly sown over the crop residues. Soybeans and corn grains were harvested, while crop residues remained in the respective plots. The cover crop plant (millet) residues remained as mulch in NT and were incorporated in the soil in CT. These crop sequences are similar to those adopted by farmers in the region, involving a sequence of soybean crops after clearing a native vegetation area, followed by eventually introducing the corn crop.
The TSP used contained 20.8% total P, 92% of which was soluble in a 2% citric acid solution. The RRP consisted of 12.3% total P, 44% of which was soluble in 2% citric acid (1:100 phosphate:solution ratio, RRP particles analyzed at <0.063 mm). Thus, the RRP used in this study was highly reactive, as a consequence of the high substitution rate of carbonate to phosphate in the crystal structure (Zapata and Roy, 2004). Most RRP particles applied to the field were between 0.5 and 2.8 mm.
Maintenance fertilization was done annually in the summer crop with 66.7 kg ha–1 of K in the form of KCl, 30 kg ha–1 of S as phosphogypsum, and in the case of corn, 30 kg ha–1 of N was applied in the planting furrow along with two posterior applications with 60 kg ha–1 of N at 4–5 and 7–8 open leaves in the form of urea, according to Sousa and Lobato (2004).
Soil samples were taken from the 0–2.5, 2.5–5.0, 5–10, 10–20, 20–30, and 30–40 cm layers after the 17th cultivation, using a soil core sampler auger to remove intact samples for the three first layers and a Dutch auger for the two deeper layers. 15–20 subsamples were randomly taken within the useable area plots to make up the composite sample of each plot in the treatments with broadcast spread fertilization. For the treatments with band-applied fertilization in the furrow, the composite samples of each plot were formed by subsampling according to Nicolodi et al. (2002) and CQFS-RS/SC (2004). This method involves sampling from seven points across a crop row: one right over the row, and three equally spaced to each side, up to the center of the corn inter-row. The soil from each layer of these seven positions was then mixed to form a subsample. This procedure was repeated three times per plot, which were mixed to form the plot sample. This methodology was adopted because it better considers the localized effects of band-applying P fertilizers. An area under natural Cerrado vegetation located 50 m from the experiment was chosen as reference and was sampled in three replications with 20 subsamples each.
Total digestion P (Ptdig) was determined in soil samples by acid digestion with H2SO4 and H2O2 in the presence of an MgCl2 saturated solution (Brookes and Powlson, 1981; Hedley et al., 1982), analyzed by spectrophotometry at 820 nm (Murphy and Riley, 1962).
Total organic P (Po) in the soil was determined according to the ignition method (Hance and Anderson, 1962; Olsen and Sommers, 1982). Two subsamples were submitted to extraction for 16 h with H2SO4 2.0 mol L–1, in the 1:8 soil:solution ratio. One subsample was previously submitted to ignition at 550°C for 1.5 h, in order to obtain total ignition P (Ptign) and the other not. Total Po was then obtained by the difference in P content between the two subsamples, analyzed by spectrophotometry at 820 nm (Thermo Fisher Scientific model Helios Omega) (Murphy and Riley, 1962).
Chemical fractionation of P was performed using the procedure originally proposed by Hedley et al. (1982) with modifications, as described below. Samples of 1.5 g soil were subjected to successive extractions with NaHCO3 0.5 mol L–1, NaOH 0.1 mol L–1, HCl 1.0 mol L–1, and NaOH 0.5 mol L–1. After extraction, the remaining soil was oven-dried at 40°C, and one part was subjected to digestion with H2SO4 + H2O2 + MgCl2 (Brookes and Powlson, 1981; Hedley et al., 1982) to obtain the residual Pt (Ptres). To obtain the residual Po (Pores), the soil remaining after fractionation was subjected to the ignition method described above (Hance and Anderson, 1962; Olsen and Sommers, 1982). The residual Pi (Pires) was obtained by subtracting Pores from Ptres.
In Hedley’s fractionation scheme of alkaline extracts (NaHCO3 and NaOH fractions), Pi was obtained by acidifying the extract and centrifuging to precipitate the organic compounds; acidified extract Pi concentration was then determined by spectrophotometry at 820 nm (Murphy and Riley, 1962). Total P for these Hedley’s fractions, on the other hand, was obtained by digesting the extract with H2SO4 + (NH4)2S2O8 in an autoclave (103.4 kPa, 121°C, 2 h) (USEPA, 1971) and determined by inductively coupled plasma atomic emission spectrometry (ICP/AES; model iCAP 6000, Thermo Fisher Scientific). The Po from these extracts was calculated by subtracting Pi from total P. The P fractions from Hedley’s fractionation scheme were then categorized as geochemical (Pgeo) and biological P (Pbio), according to Cross and Schlesinger (1995); the first was obtained by summing all fractions of Pi and the second by summing all fractions of Po. The sum of Pi and Po obtained by the fractionation procedure was denoted PtΣ frac.
Total P applied over the 17 years consisted of fertilizer P (17 years × 35 kg ha–1 year–1 P = 595 kg P ha–1) plus P provided by phosphogypsum application (13 kg P ha–1), totaling 608 kg P ha–1. To estimate the soil’s input–output residual P, total P applied was discounted by the quantity of P exported in the grains, assessed by wet digestion using HNO3 + HClO4 (3:1 v/v) (EMBRAPA, 1999).
The variation in P stocks in the 0–40 cm layer, compared to the natural Cerrado soil, was also assessed. To perform these soil P balance calculations, soil bulk densities were evaluated in all treatments and the reference area of Cerrado in the 0–2.5, 2.5–5, 5–10, 10–15, 15–20, 20–30, and 30–40 cm layers, after the 17th cultivation according to the volumetric ring method (EMBRAPA, 2005). To calculate the inventories and consequent P balance, the methodology of mass equivalence was applied (Ellert and Bettany, 1995). P balance was determined for both Ptdig and Ptign fraction contents. It was considered a discount in mass in the last sampled layer (30–40 cm), which was not affected by soil tillage or P fertilizer management. The equivalent mass in the 30–40 cm layer was calculated as follows:
where Mt(30–40) is the real soil mass in a given treatment in the 30–40 cm layer, given by its soil density multiplied by the volume occupied in a hectare; Mt(0–40)is the sum of soil mass in the different layers evaluated down to 40 cm in the given treatment; and Mr(0–40) is the sum of soil mass in the different layers down to 40 cm in the reference area (natural Cerrado vegetation soil).
Soil P stocks for each layer were then calculated, multiplying its P content by soil mass in the respective layer, considering the equivalent mass in the case of the 30–40 cm layer. The sum of P stocks in all evaluated layers minus that found in the reference area of the Cerrado is the final Pbalance. Soil bulk densities, P contents, and mass equivalence for the different layers and treatments are shown in Supplementary Table S5.
P recovery calculated for a given total P fraction (Ptdig or Ptign) was defined as follows:
where Pex is the amount of P exported in grains after 17 crops (kg ha–1); Pbalance is the gain of P in soil in the 0–40 cm layer compared to native vegetation soil (kg ha–1), for a given soil P fraction analysis method; and Papplied is the total amount of P applied as fertilizer (608 kg P ha–1).
To analyze the variance in grain yield and recovery of P, the following model was used:
To analyze the variance in P levels at the different dependent variable depth, the following model was used:
where μ = overall data mean; B = block (i = 1, 2, 3); S = management system (j = 1, 2); F = source of P (k = 1, 2); M = type of application (l = 1, 2); C = layer (m = 1, 2, 3, 4, 5); and error = experimental error.
Analysis of variance was carried out using SAS PROC MIXED, and when significant, the Fischer’s least significant difference method (p < 0.05) was used to differentiate the means. Moreover, a paired t-test was applied at the 5% significance level to compare differences in P stocks in the weighted 0–30 cm layer mean between each treatment and the reference area under natural vegetation. This analysis was applied using the software R (version 3.4.0) (R Core Team, 2017).
The soil and fertilization management methods, as well as the phosphate sources used over the 17 years of cropping, resulted in differences in crop yield, grain P export, and consequently residual P stocks in the soil (Table 2). Under NT, total grain yield was higher for soybean and maize using TSP (mean of 97.2 Mg ha–1 using TSP compared to 89.0 Mg ha–1 for RRP, mean of both application methods) (Table 2). Treatments with lower values of grain yield resulted in higher values of calculated (input–output) residual P. Average soybean production throughout the experiment varied between 1.06 Mg ha–1 in the first year, when P stocks in soil were still low, and 3.76 Mg ha–1 in the 14th year, which is similar to regional yields. For corn, the average yield was 11.5 Mg ha–1, which is in line with high technology systems in the region.
Table 2. Soil P balance in a clayey Oxisol cultivated for 17 years under distinct soil management systems, phosphate fertilization sources, and application methods.
P stocks gain in the soil in Table 2 was calculated considering soil P contents determined by each extraction method, and soil densities, adjusting stocks by the methodology of mass equivalence (Ellert and Bettany, 1995). Total P by the ignition method (Ptign) consists in extraction with a relatively dilute H2SO4 solution (2.0 mol L–1) of soil samples subjected to ignition at 550°C, according to Hance and Anderson (1962) and Olsen and Sommers (1982), while total P by acid digestion (Ptdig) uses a concentrated H2SO4 solution (18 mol L–1) plus H2O2 under heating (Hedley et al., 1982), allowing for more complete access to total soil P. As a result, P gain in the soil by the ignition method resulted, on average, in a P recovery of 67% under CT, with no difference between fertilizer sources and management methods. In contrast, the same balance performed using acid digestion (Ptdig) resulted in an average 95% P recovery under the same tillage system.
Under NT, the P recovery by Ptign resulted in an average of 95% of the P applied, with a better response under broadcast application by both phosphate fertilizer sources (Table 2). Considering Ptdig, P recovery was 100% under NT, with no difference (p > 0.05) between the two systems, phosphate sources and fertilization methods.
The difference between the two methods in P recovery noticed for CT but not for NT indicates an accumulation of P in more labile forms under the conservationist system, where the soil is not revolved. Under CT, some 28% of the P applied over the 17 years was in chemical forms beyond the absorption capacity of the plants, a P form accessed by the digestion method (Ptdig) but not the ignition method (Ptign). In addition, through the digestion method, it can be seen that practically all the P applied to the soil over the 17-year cropping period and not taken up by the grains remained in the soil under both systems.
The variance analysis (Supplementary Table S1) showed that inorganic fractions, rather than organic, were the most influenced by the treatments and their interaction, but residual P fractions were less influenced than the others. Also, depending on the soil layer, the most common order in which the factors influenced P fractions was first the soil management system, then the source of phosphate fertilizer, and finally the method of application. The interaction of phosphate fertilizer management systems with the soil management system is probably related to the fact that CT and NT may provide different degrees of soil/fertilizer contact, possibly modifying the dynamics of the fertilizer solubilization, especially for slowly soluble sources like RRP, which requires more time and greater soil surface contact for dissolution (Pavinato et al., 2017; de Oliveira et al., 2019). In NT, the fertilizer application method directly influences P contents in the different soil layers, while CT minimizes the effect of the placement of the phosphate applied in previous growing seasons.
The various Pi and Po fraction contents throughout the different soil layers are shown in Supplementary Figures S1–S5. Here summarized as geochemical (Pgeo) and biological P (Pbio), respectively, it was noted that the accumulation in these distinct compartments was influenced by soil tillage and fertilizer management (Figure 1). Tillage and fertilizer management did not affect P fractions in the 30–40 cm soil layer, because tillage was limited to about 20 cm deep. For this reason, data is shown up to the 20–30 cm layer, with fairly similar Pgeo and Pbio values for all treatments.
Figure 1. Distribution of geochemical P (Pi) and biological P (Po) in five layers of a very clayey Oxisol after 17 years of cultivation in different soil tillage systems and phosphate fertilizer sources and application methods. CT, conventional tillage; NT, no-tillage; TSP, triple superphosphate; RRP, reactive rock phosphate; B, broadcast; F, furrow. The bars represent the LSD at 5% probability by Fischer’s test.
The highest Pgeo and Pbio levels were found in the 0–2.5 cm layer, especially when RRP was broadcast applied under NT. Under CT, P contents presented a more homogeneous distribution over the soil profile, whereas under NT there was a steep depth gradient, with layers down to 5 cm showing higher values than under CT, similar values in the 5–10 cm layer, and lower values under NT compared to CT in the 10–20 cm layer. The tillage system adopted was thus the main factor affecting Pgeo and Pbio contents at depth. Dynamics of fertilizer solubilization and SOM mineralization are factors closely related to tillage that may explain these differences.
For the weighted average of all layers down to 30 cm, geochemical fractions (Pgeo) represented some 64% and biological fractions (Pbio) represented 36% of soil PtΣ frac, with a mean of 348 mg kg–1, and very similar values for the different soil and fertilization management systems. However, there were some punctual variations: for instance, where the RRP fertilizer was applied under NT by broadcast application, the amount of Pgeo was 80% of PtΣ frac in the 0–2.5 cm layer. Otherwise, in the Cerrado soil, 53 and 47% of PtΣ frac were respectively present as Pgeo and Pbio, with a PtΣ frac mean of 268 mg kg–1 (Supplementary Table S4). Tiecher et al. (2012a), working in an area cropped for 23 years under NT and annual fertilization, found a reduction in the proportion of Pgeo (from 57 to 49%) and consequently an increase in the proportion of Pbio (from 43 to 51%) in PtΣ frac. These authors ascribed the Po gains to a balanced P fertilization, with no excessive application beyond plant demand, and the adoption of management systems with high potential for boosting SOM using cover crops. In their work, like in Rheinheimer et al. (2019), increasing total P (Pt) and SOM contents were found to contribute to the transformation of Pi into Po under NT. In contrast, in our work, the nine years of initial soybean cultivation without cover crop rotation, when P offtake was significantly greater than P offtake (data not shown), resulted in a high accumulation of Pt (Table 2) and a small plant biomass input that contributed to maintaining the fertilizer P especially in inorganic fractions.
The adoption of NT soil management was able to promote more P accumulation in labile + moderately labile forms compared to CT in the top surface layers (Figure 2). Under CT, the labile + moderately labile fractions with the average weighted by the most influential fertilized layer (0–20 cm) represented around 62% of PtΣ frac (average of 393 mg kg–1). Otherwise, under NT with the average weighted by the most influential fertilization layer in this system (0–10 cm), this value was 69% of PtΣ frac (average of 539 mg kg–1), reaching 75% in the 0–2.5cm layer with broadcast TSP and 82% in the same layer with broadcast RRP.
Figure 2. Participation of labile + moderately labile and residual fractions of P in relation to PtΣfrac in different layers of a very clayey Oxisol after 17 years of cultivation under distinct soil management systems, phosphate fertilizer sources, and application methods. CT, conventional tillage; NT, no-tillage; TSP, triple superphosphate; RRP, reactive rock phosphate; B, broadcast fertilizer application; F, furrow fertilizer application. Different letters represent significant differences between treatments in a given soil layer and P fraction, according to Fischer’s LSD test (P < 0.05).
Many studies account for the increase in P lability under NT in terms of gains in labile Po fractions (Rheinheimer and Anghinoni, 2003; Tiecher et al., 2012a). However, in our study, the greater proportion of labile + moderately labile fractions in PtΣ frac evaluated under NT was resultant from Pi fraction increase, not Po. This can be seen in Supplementary Figure S6A, where the Pi levels of each fraction are shown in terms of the relative proportion of total Pi obtained in fractionation (Pgeo), and in Supplementary Figure S6B, where the Po levels of each fraction are shown in terms of the relative proportion of the total fractionation scheme Po (Pbio). Under NT, Pi was present in greater amounts as labile and moderately labile Pi fractions in Pgeo in comparison to CT (Supplementary Figure S6A). However, the proportion of labile and moderately labile Po fractions in Pbio was even slightly lower for NT when compared to CT (Supplementary Figure S6B). This means that NT has preferably accumulated available Pi instead of labile Po.
Under CT, the fertilizer incorporation when plowing the soil resulted in a homogeneous distribution of Pi fractions. When fertilizer was broadcast in this system, due to thorough mixing with the soil, we hypothesize that differences in solubilization speed between TSP and RRP were minimized, resulting in complete solubilization of RRP. This is shown by the similarity between these two sources in the fractions of NaHCO3 Pi and HCl Pi when in CT, but not in NT (Supplementary Figure S6).
In NT, TSP showed a greater proportion of Pi and Po in the labile fraction NaOH 0.1 M in relation to RRP (Supplementary Figure S6A). Moreover, fertilizer furrow application resulted in more Pi in the region of fertilizer placement (between 2.5 and 10 cm) compared to the broadcast application. The application on soil surface has limited the solubilization of RRP, shown by the high levels of HCl Pi in the 0–2.5 cm layer (Supplementary Figure S6A), related to unsolubilized rock phosphate.
In CT, since the soil was completely homogenized every year together with plant root and shoot waste, the differences that may exist in the dynamics of P under CT are ephemeral, and merely the results of the year in question. Also, CT helps expose Pi to new adsorption sites (Sousa and Volkweiss, 1987a), favoring the transformation of more labile forms of Pi, such as NaHCO3 Pi and NaOH 0.1 M Pi, into forms of lower lability (Pires), impairing their lability in the system, especially in soils with an elevated sink effect (Novais et al., 2007).
Under NT, the differences between fertilizer management methods were more intense in the application layers (0–5.0 cm for broadcast and 5.0–10 cm for furrow) and are more evident than under CT. There was a superficial accumulation of Pi under NT, especially under broadcast application, due to the preservation of the fertilized layer and due to P cycling through the crops over the years (Santos and Tomm, 2003) through the decomposition of plant tissue waste deposited on the surface or absorbed by the roots (Costa et al., 2010). This Pi is of higher lability in these layers compared to the subsurface layers. This could be due to the higher accumulation of SOM on the surface under NT (Zhou et al., 2019), reducing Pi retention in the soil due to organic ions competing for adsorption sites (Sibanda and Young, 1986; Hue, 1991; Mesquita Filho and Torrent, 1993; Ohno and Erich, 1997; Nziguheba et al., 1998; Fink et al., 2016b), and the accumulation of P in these layers partially saturates adsorption sites (Guertal et al., 1991), so that the remaining Pi may be adsorbed with lower energy, possibly facilitating subsequent desorption, as observed by Barrow et al. (2018) and Tiecher et al. (2012b). In fact, this was observed by de Oliveira et al. (2020b) after cultivating this area without P input during successive corn crops, where despite about 100% of crop nutrition was supplied by the 0–5.0 cm layer under broadcast P application, compared to 70% under band application, P offtake and grain yield were similar between both application methods. Continuous broadcast P fertilization under NT could even increase yields in highly adsorptive weathered soils (de Oliveira et al., 2020a).
The reaction of the fertilizer itself is usually slower under NT (Kunishi et al., 1982). This might have resulted in the accumulation of non-reacted forms of calcium phosphate in our experiment (Sousa and Volkweiss, 1987b), and the possible formation of secondary calcium phosphates (Magid, 1993) observed in HCl Pi. It may protect P under this system, helping to maintain P in more labile forms. In the superficial layer under NT, the neoformation of calcium phosphates could be boosted by the high levels of Ca and high pH resulting from superficial liming, as also reported by Guo et al. (2000); Rheinheimer and Anghinoni (2001), and Tiecher et al. (2012b). RRP results in a high accumulation of these calcium phosphates and also P forms adsorbed on Fe and Al oxides and clay minerals of lower lability compared to TSP, evidenced by both the higher levels of HCl Pi and NaOH 0.5 M Pi, as previously reported by Soltangheisi et al. (2018) in a similar Oxisol. Although these fractions are considered to be of lower lability, an elevated residual effect and P use efficiency was observed from RRP fertilization after cultivating this area without P input during successive corn crops (de Oliveira et al., 2019).
As a result of this complex P dynamics in soil under the different tillage systems and phosphate fertilization strategies, it is noticed that NT and RRP use could be recommended as strategies to improve legacy P bioavailability in highly P adsorptive Oxisols in the tropics. This is new in the way which previous studies in CT systems were not able to observe the special importance of rock phosphates in highly adsorptive acid soils when soil is minimally disturbed. NT, combined with RRP applications, promotes efficient P nutrition while probably protecting P from adsorption due to calcium-bound forms.
While CT stimulates fertilizer reaction with colloids in the soil and speeds up SOM decomposition (Nunes et al., 2011a), favoring the accumulation of Pires, there is less accumulation of Pores under this system for the same reasons. Tillage and fertilization management little influenced the proportion of the Pores fraction in Pbio, with values around 30% of Pbio (Supplementary Figure S6). Furthermore, the proportion of the NaHCO3 Po fraction was fairly stable across the treatments at around 8% of Pbio. Under NT, even the more labile fraction of Po did not accumulate, probably due to rapid turnover (Bowman and Cole, 1978).
In general, a greater proportion of the less labile fraction NaOH 0.5 M and a smaller proportion of the more labile NaOH 0.1 M under NT when compared to CT were observed (Supplementary Figure S6B). This was especially evident when the P source was RRP. Although counterintuitive, this fact might be related to the intensified microbial activity under NT (Mendes et al., 2003), responsible for SOM chemical stabilization reactions and improved soil structure (Bertol et al., 2004). This physical protection (Nunes et al., 2011a; Figueiredo et al., 2013) might boost Po stability in the soil, evidenced by the NaOH 0.5 Po fraction, and therefore results in Po accumulation. This is a new observation which may add to the positive benefits of the NT system that generally results in increased crop yields. Labile and moderately labile fractions of Po under CT, on the other hand, are more easily mineralized and may constitute the main source of P for cropping under this system (Tiecher et al., 2018).
Otherwise, the accumulation of Po in more stable forms observed by RRP fertilization is intriguing. One hypothesis proposed by Santos et al. (2008) is that the replenishment of labile P, previously made by less labile Po fractions, could be made by the slow RRP P release in soils fertilized with this source. This would leave stable Po fraction contents relatively unaltered and able to accumulate.
Compared to the soil under natural Cerrado vegetation (Supplementary Table S4), gains in Pgeo were observed throughout the soil profile, irrespective of soil tillage and fertilizer management, ranging from 14 to 700 mg kg–1 (Figure 3). On the other hand, gains in Pbio did not occur in all soil layers and were significantly less than those of Pgeo. Even so, gains in Pbio in some layers were considerable, reaching 75 mg kg–1.
Figure 3. Variation in geochemical and biological P in relation to natural Cerrado in five layers of a very clayey Oxisol after 17 years of cultivation under distinct soil management systems and phosphate fertilizer sources and application methods. CT, conventional tillage; NT, no-tillage; TSP, triple superphosphate; RRP, reactive rock phosphate; B, broadcast; F, furrow. *Significant according to the Student’s t-test at P < 0.05.
Under CT, intermediate layers (2.5–5, 5–10, and 10–20 cm) exhibited net gains in Pbio, mostly due to the NaOH 0.1 M fraction, whereas NaOH 0.5 M Po and residual Po presented a negative balance (Figure 4). Under NT, in most treatments and layers down to 10 cm, there were expressive gains in Pbio compared to Cerrado, especially in the NaOH 0.5 M fraction. Below 10 cm, there were losses of Pbio, especially in the NaOH 0.1 M and residual fractions.
Figure 4. Variation in the Po pool in relation to natural Cerrado in five layers of a very clayey Oxisol after 17 years of cultivation under distinct soil management systems and phosphate fertilizer sources and application methods. CT, conventional tillage; NT, no-tillage; TSP, triple superphosphate; RRP, reactive rock phosphate; B, broadcast; F, furrow. *Significant according to the Student’s t-test at P < 0.05.
These differences in P gains between both tillage systems are related to the soil depth affected by the management practices in each system: under CT, the soil is revolved up to 20 cm deep, while in NT soil disturbance was limited to about 10 cm deep due to sowing operations and fertilization, in the case of furrow application. This way, some losses of Po below 20 cm under CT and below 10 cm under NT are explained by no effect of the management system on these layers, whereas in the Cerrado soil the deep root systems of the various species make a steady contribution of Po at deeper layers in the profile.
These results challenge the idea that Po is not altered by phosphate fertilization in highly weathered clayey soils, as reported by Conte et al. (2003) under NT after 5 years of cropping, and Pavinato et al. (2009) under CT and NT after 13 years of cropping. Possibly, management system duration plays a major role in producing this effect on Po, as reported by Costa et al. (2010), who observed the effect of the phosphate fertilizer application method on Po in an Argisol cropped for 18 years under CT and NT.
Considering the layers influenced by the soil management system (0–20 cm under CT and 0–10 cm under NT), it can be seen that Pbio gains under both CT and NT become fairly significant. Taking into account the fact that the superficial layer, especially under NT, exhibits the highest accumulation of nutrients (De Maria et al., 1999), roots (Costa et al., 2010), and microbial activity (Mendes et al., 2003), the effect of the soil and fertilization management system on Po has significant potential to influence the dynamics of P nutrition for plants, corroborating to Redel et al. (2007).
After 17 years of grain crop cultivation and fertilizer use, legacy P accumulated in the soil was influenced by soil tillage and phosphate fertilization management.
There was an accumulation of Pi and Po under both tillage systems compared to Cerrado natural soil. CT resulted in a more homogeneous distribution of Pi fractions throughout the profile, minimizing the effects of P fertilizer management.
Despite a strong P content stratification through the soil profile, with Pi accumulation in the fertilizer application zone, the NT system promoted P lability and net Po accumulation in layers where roots predominantly develop (0–10 cm). This is especially relevant considering that the conservationist system increased P exports in grains by 21% when compared to CT, after the 17 crops. This is a NT system benefit not previously observed in depth in tropical soils.
There was a high accumulation of Pi associated with calcium (HCl Pi) in soil under NT with RRP application, especially when this fertilizer was broadcast applied. This P fraction may act as a source of slow-release legacy P, which could not be observed in previous experiments involving CT only.
NT and RRP positively affected legacy P fractions and can be recommended as strategies to improve P fertilizer use efficiency.
The datasets generated for this study are available on request to the corresponding author.
RN: substantial work elaboration, writing, and data interpretation. DS: experimental design elaboration and laboratorial analyses. WG: contribution in experimental design elaboration and writing. LO: critical analysis of content and contributions in writing and statistics. PP: corrections in writing and significant scientific approach insights. TP: data interpretation and figure making. All authors contributed to the article and approved the submitted version.
This research was supported by the Embrapa Cerrados.
The authors declare that the research was conducted in the absence of any commercial or financial relationships that could be construed as a potential conflict of interest.
RN and LO acknowledge financial assistance granted by the Coordenação de Aperfeiçoamento de Pessoal de Nível Superior-Brasil (CAPES) – Finance Code 001.
The Supplementary Material for this article can be found online at: https://www.frontiersin.org/articles/10.3389/feart.2020.00350/full#supplementary-material
Alvares, C. A., Stape, J. L., Sentelhas, P. C., De Moraes Gonçalves, J. L., and Sparovek, G. (2013). Köppen’s climate classification map for Brazil. Meteorol. Zeit. 22, 711–728. doi: 10.1127/0941-2948/2013/0507
Barrow, N. J., Barman, P., and Debnath, A. (2018). Three residual benefits of applying phosphate fertilizer. Soil Sci. Soc. Am. J. 82, 1168–1176. doi: 10.2136/sssaj2018.03.0115
Bertol, I., Albuquerque, J. A., Leite, D., Amaral, A. J., and Zoldan Junior, W. A. (2004). Propriedades físicas do solo sob preparo convencional e semeadura direta em rotação e sucessão de culturas, comparadas às do campo nativo. Rev. Bras. Ciênc. Solo 28, 155–163. doi: 10.1590/S0100-06832004000100015
Bowman, R. A., and Cole, C. V. (1978). An exploratory method for fractionation of organic phosphorus from grassland soils. Soil Sci. 125, 95–101. doi: 10.1097/00010694-197802000-00006
Brookes, P. C., and Powlson, D. C. (1981). Preventing phosphorus losses during perchloric acid digestion of sodium bicarbonate soil extracts. J. Sci. Food Agric. 32, 671–674. doi: 10.1002/jsfa.2740320707
Conte, E., Anghinoni, I., and Rheinheimer, D. S. (2003). Frações de fósforo acumuladas em Latossolo argiloso pela aplicação de fosfato no sistema plantio direto. Rev. Bras. Ciênc. Solo 27, 893–900. doi: 10.1590/S0100-06832003000500014
Costa, S. E. V. G. A., Souza, E. D., de Anghinoni, I., Flores, J. P. C., Vieira, F. C. B., Martins, A. P., et al. (2010). Patterns in phosphorus and corn root distribution and yield in long-term tillage systems with fertilizer application. Soil Till. Res. 109, 41–49. doi: 10.1016/j.still.2010.04.003
Cross, A. F., and Schlesinger, W. H. (1995). A literature review and evaluation of the Hedley fractionation: applications to the biogeochemical cycle of soil phosphorus in natural ecosystems. Geoderma 64, 197–214. doi: 10.1016/0016-7061(94)00023-4
De Maria, I. C., Nnabude, P. C., and Castro, O. M. (1999). Long-term tillage and crop rotation effects on soil chemical properties of a Rholic Ferrasol in southern Brazil. Soil Till. Res. 51, 71–79. doi: 10.1016/j.still.2003.12.003
de Oliveira, L. E. Z., Nunes, R., de Sousa, D. M. G., Busato, J. G., and de Figueiredo, C. C. (2019). Response of maize to different soil residual phosphorus conditions. Agron. J. 111, 1–10. doi: 10.2134/agronj2018.11.0710
de Oliveira, L. E. Z., de Sousa, D. M. G., de Figueiredo, C. C., Nunes, R. S., and Malaquias, J. V. (2020a). Long-term phosphate fertilization strategies evaluation in a Brazilian Oxisol. Agron. J. 1–18. doi: 10.1002/agj2.20324
de Oliveira, L. E. Z., Nunes, R., de Sousa, D. M. G., and de Figueiredo, C. C. (2020b). Dynamics of residual phosphorus forms under different tillage systems in a Brazilian Oxisol. Geoderma 367, 114254. doi: 10.1016/J.GEODERMA.2020.114254
Ellert, B. H., and Bettany, J. R. (1995). Calculation of organic matter and nutrients stored in soils under contrasting management regimes. Can. J. Soil Sci. 75, 529–538. doi: 10.4141/cjss95-075
Embrapa (1997). Manual de Métodos de Análise de Solo. Empresa Brasileira de Pesquisa Agropecuária. Rio de Janeiro: Centro Nacional de Pesquisa de Solos.
EMBRAPA (1999). Handbook for Chemical Analysis of Soils, Plants and Fertilizers (In Portuguese). Empresa Brasileira de Pesquisa Agropecuaìria. Brasília: Embrapa Informação Tecnoloìgica.
EMBRAPA (2005). Manual de Laboratórios: Solo, Água, Nutrição Animal e Alimentos. São Carlos: Embrapa Pecuária Sudeste.
EMBRAPA (2013). Sistema Brasileiro de Classificação de solos. Empresa Brasileira de Pesquisa Agropecuária. Brasília: Embrapa Informação Tecnoloìgica.
Figueiredo, C. C., Resck, D. V. S., Carneiro, M. A. C., Ramos, M. L. G., and Sá, J. C. M. (2013). Stratification ratio of organic matter pools influenced by management systems in a weathered Oxisol from a tropical agro-ecoregion in Brazil. Soil Res. 51, 133–141. doi: 10.1071/SR12186
Fink, J. R., Inda, A. V., Bayer, C., Torrent, J., and Barrón, V. (2014). Mineralogy and phosphorus adsorption in soils of south and central-west Brazil under conventional and no-tillage systems. Acta Sci. Agron. 36, 379–387. doi: 10.4025/actasciagron.v36i3.17937
Fink, J. R., Inda, A. V., Bavaresco, J., Barrón, V., Torrent, J., and Bayer, C. (2016a). Adsorption and desorption of phosphorus in subtropical soils as affected by management system and mineralogy. Soil Tillage Res. 155, 62–68. doi: 10.1016/j.still.2015.07.017
Fink, J. R., Inda, A. V., Tiecher, T., and Barrón, V. (2016b). Iron oxides and organic matter on soil phosphorus availability. Ciênc. Agrotec. 40, 369–379. doi: 10.1590/1413-70542016404023016
Gatiboni, L. C., Kaminski, J., Rheinheimer, D. S., and Flores, J. P. C. (2007). Biodisponibilidade de formas de fósforo acumuladas em solo sob sistema plantio direto. Rev. Bras. Ciênc. Solo 31, 691–699. doi: 10.1590/S0100-06832007000400010
Guertal, E. A., Eckert, D. J., Traina, S. J., and Logan, T. J. (1991). Differential phosphorus retention in soil profiles under no-till crop production. Soil Sci. Soc. Am. J. 55, 410–413. doi: 10.2136/sssaj1991.03615995005500020020x
Guo, F., Yost, R. S., Hue, N. V., Evensen, C. I., and Silva, J. A. (2000). Changes in phosphorus fractions under intensive plant growth. Soil Sci. Soc. Am. J. 64, 1681–1689. doi: 10.2136/sssaj2000.6451681
Hance, R. J., and Anderson, G. (1962). A comparative study of methods of estimating soil organic phosphate. J. Soil Sci. 13, 225–230. doi: 10.1111/j.1365-2389.1962.tb00700.x
Hedley, M. J., Stewart, J. W. B., and Chauhan, B. S. (1982). Changes in inorganic and organic soil phosphorus fractions induced by cultivation practices and by laboratory incubations. Soil Sci. Soc. Am. J. 46, 970–976. doi: 10.2136/sssaj1982.03615995004600050017x
Hue, N. V. (1991). Effects of organic acids/anions on P sorption and phytoavailability in soils with different mineralogies. Soil Sci. 152, 463–471. doi: 10.1097/00010694-199112000-00009
Huffman, S. A., Cole, C. V., and Scott, N. A. (1996). Soil texture and residue addition effects on soil phosphorus transformation. Soil Sci. Soc. Am. J. 60, 1095–1101. doi: 10.2136/sssaj1996.03615995006000040019x
IUSS Working Group WRB (2006). World Reference Base for Soil Resources: A Framework for International Classification, Correlation and Communication. World Soil Resources Reports, n.103. Rome: FAO.
Kunishi, H. M., Bandel, V. A., and Mulford, F. R. (1982). ANO measurement of available soil phosphorus under conventional and no-till management. Commun. Soil Sci. Plant Anal. 13, 607–618. doi: 10.1080/00103628209367299
Kurihara, C. H., Silva, W. M., Dias, M. M., Tsujigushi, B. P., and Silva, J. V. S. (2016). Gradual correction of phosphorus availability in the no-tillage system. Rev. Ceres 63, 256–264. doi: 10.1590/0034-737X201663020018
Magid, J. (1993). Vegetation effects on phosphorus fraction in set-aside soils. Plant Soil 149, 111–119. doi: 10.1007/bf00010768
Mendes, I. C., Souza, L. V., Resck, D. V. S., and Gomes, A. C. (2003). Propriedades biológicas em agregados de um Latossolo Vermelho-escuro sob plantio convencional e direto no Cerrado. Rev. Bras. Ciênc. Solo 27, 435–443. doi: 10.1590/S0100-06832003000300005
Mesquita Filho, M. V., and Torrent, J. (1993). Phosphate sorption as related to mineralogy of a hydrosequence of soils from the cerrado region (Brazil). Geoderma 58, 107–123. doi: 10.1016/0016-7061(93)90088-3
Miranda, L. N., De Miranda, J. C. C., Rein, T. A., and Gomes, A. C. (2005). Utilização de calcário em plantio direto e convencional de soja e milho em Latossolo Vermelho. Pesq. Agropec. Bras. 40, 563–572. doi: 10.1590/S0100-204X2005000600006
Murphy, J., and Riley, J. P. (1962). A modified single solution method for the determination of phosphate in natural waters. Anal. Chim. Acta 27, 31–36. doi: 10.1016/S0003-2670(00)88444-5
Nicolodi, M., Anghinoni, I., and Salet, R. L. (2002). Alternativa à coleta de uma secção transversal, com pá de corte, na largura da entrelinha, na amostragem do solo em lavouras com adubação em linha no sistema plantio direto. Rev. Plant. Direto 69, 22–28.
Novais, R. F., Smyth, T. J., Nunes, F. N., Fósforo, I. N., Novais, R. F., Alvarez, V. H., et al. (2007). Fertilidade do Solo. Viçosa: Sociedade Brasileira de Ciência do Solo, 471–550.
Nunes, R. S., Lopes, A. A. C., Sousa, D. M. G., and Mendes, I. C. (2011a). Sistemas de manejo e os estoques de carbono e nitrogênio em latossolo de Cerrado com a sucessão soja-milho. Rev. Bras. Ciênc. Solo 35, 1407–1419. doi: 10.1590/S0100-06832011000400035
Nunes, R. S., Sousa, D. M. G., Goedert, W. J., and Vivaldi, L. (2011b). Distribuição de fósforo no solo em razão do sistema de cultivo e manejo da adubação fosfatada. Rev. Bras. Ciênc. Solo 35, 877–888. doi: 10.1590/S0100-06832011000300022
Nziguheba, G., Palm, C. A., Buresh, R. J., and Smithson, P. C. (1998). Soil phosphorus fractions and adsorption as affected by organic and inorganic sources. Plant Soil 198, 159–168. doi: 10.1023/A:1004389704235
Ohno, T., and Erich, M. S. (1997). Inhibitory effects of crop residue-derived organic ligands on phosphate adsorption kinetics. J. Environ. Qual. 26, 889–895. doi: 10.2134/jeq1997.00472425002600030041x
Olsen, S. R., and Sommers, L. E. (1982). “Phosphorus,” in Methods of Soil Analysis, Part 2. Chemical and Microbiological Properties, 2 Edn, eds A. L. Page, R. H. Miller, and Q. R. Keeney (Madison: ASA, SSSA), 403–430.
Pavinato, P. S., Rodrigues, M., Soltangheisi, A., Sartor, L. R., and Withers, P. J. A. (2017). Effects of cover crops and phosphorus sources on maize yield, phosphorus uptake, and phosphorus use efficiency. Agron. J. 109, 1039–1047. doi: 10.2134/agronj2016.06.0323
Pavinato, S. P., Merlin, A., and Rosolem, C. A. (2009). Phosphorus fractions in Brazilian Cerrado soils as affected by tillage. Soil Till. Res. 105, 149–155. doi: 10.1016/j.still.2009.07.001
R Core Team (2017). R: A Language and Environment for Statistical Computing. Vienna: R Foundation for Statistical Computing.
Redel, Y. D., Rubio, R., Rouanet, J. L., and Borie, F. (2007). Phosphorus bioavailability affected by tillage and crop rotation on a Chilean volcanic derived Ultisol. Geoderma 139, 388–396. doi: 10.1016/j.geoderma.2007.02.018
Rheinheimer, D., dos, S., Fornari, M. R., Bastos, M. C., Fernandes, G., Santanna, M. A., et al. (2019). Phosphorus distribution after three decades of different soil management and cover crops in subtropical region. Soil Tillage Res. 192, 33–41. doi: 10.1016/j.still.2019.04.018
Rheinheimer, D. S., and Anghinoni, I. (2001). Distribuição do fósforo inorgânico em sistemas de manejo de solo. Pesq. Agropec. Bras. 36, 151–160. doi: 10.1590/S0100-204X2001000100019
Rheinheimer, D. S., and Anghinoni, I. (2003). Accumulation of soil organic phosphorus by soil tillage and cropping systems in subtropical soils. Commun. Soil Sci. Plant Anal. 34, 2339–2354. doi: 10.1081/CSS-120024068
Santos, H. P., and Tomm, G. O. (2003). Disponibilidade de nutrientes e teor de matéria orgânica em função de sistemas de cultivo e de manejo de solo. Ciênc. Rural 33, 477–486. doi: 10.1590/S0103-84782003000300013
Santos, J. Z. L., Furtini Neto, A. E., Resende, A. V., Curi, N., Carneiro, L. F., and Costa, S. E. V. G. A. (2008). Frações de fósforo em solo adubado com fosfatos em diferentes modos de aplicação e cultivado com milho. Rev. Bras. Ciênc. Solo 32, 705–714. doi: 10.1590/s0100-06832008000200025
Selles, F., Kochhann, R. A., Denardin, J. E., Zentner, R. P., and Faganello, A. (1997). Distribution of phosphorus fractions in a Brazilian oxisol under different tillage systems. Soil Till. Res. 44, 23–34. doi: 10.1016/S0167-1987(97)00026-3
Sharpley, A. N. (1985). Phosphorus cycling in unfertilized and fertilized agricultural soils. Soil Sci. Soc. Am. J. 49, 905–911. doi: 10.2136/sssaj1985.03615995004900040023x
Sibanda, H. M., and Young, S. D. (1986). Competitive adsorption of humic acids and phosphate on goethite, gibbsite and two tropical soils. J. Soil Sci. 37, 197–204. doi: 10.1111/j.1365-2389.1986.tb00020.x
Soltangheisi, A., Rodrigues, M., Coelho, M. J. A., Gasperini, A. M., Sartor, L. R., and Pavinato, P. S. (2018). Changes in soil phosphorus lability promoted by phosphate sources and cover crops. Soil Tillage Res. 179, 20–28. doi: 10.1016/j.still.2018.01.006
Sousa, D. M. G., and Lobato, E. (2004). Cerrado: Correção Do Solo e Adubação, 2 Edn. Planaltina: Embrapa Informação Tecnológica.
Sousa, D. M. G., Rein, T. A., Goedert, W. J., Lobato, E., and Nunes, R. S. (2010). “Fósforo,” in Boas Práticas Para Uso Eficiente de Fertilizantes: Volume 2, Nutrientes, eds L. I. Prochnow, V. Casarin, and S. R. Stipp (Piracicaba: INPI - Brasil), 67–132.
Sousa, D. M. G., and Volkweiss, S. J. (1987a). Efeito residual do superfosfato triplo aplicado em pó e em grânulos no solo. Rev. Bras. Ciênc. Solo 11, 141–146.
Sousa, D. M. G., and Volkweiss, S. J. (1987b). Reações do superfosfato triplo em grânulos com solos. Rev. Bras. Ciênc. Solo 11, 133–140.
Tiecher, T., Gomes, M. V., Ambrosini, V. G., Amorim, M. B., and Bayer, C. (2018). Assessing linkage between soil phosphorus forms in contrasting tillage systems by path analysis. Soil Till. Res. 175, 276–280. doi: 10.1016/j.still.2017.09.015
Tiecher, T., Rheinheimer, D. S., and Calegari, A. (2012a). Soil organic phosphorus forms under different soil management systems and winter crops, in a long term experiment. Soil Till. Res. 124, 57–67. doi: 10.1016/j.still.2012.05.001
Tiecher, T., Rheinheimer, D. S., Kaminski, J., and Calegari, A. (2012b). Forms of inorganic phosphorus in soil under different long-term soil tillage systems and winter crops. Rev. Bras. Ciênc. Solo 36, 271–281. doi: 10.1590/S0100-06832012000100028
Zapata, F., and Roy, R. N. (2004). Use of phosphate rocks for sustainable agriculture. FAO Fert. Plant Nut. Bullet. 13, 1–148.
Zhang, T. Q., Mackenzie, A. F., Liang, B. C., and Drury, C. F. (2004). Soil test phosphorus and phosphorus fractions with long-term phosphorus addition and depletion. Soil Sci. Soc. Am. J. 68, 519–528. doi: 10.2136/sssaj2004.5190
Zheng, Z., Simard, R. R., Lafond, J., and Parent, L. E. (2001). Changes in phosphorus fractions of a Humic Gelysol as influenced by cropping systems and nutrient sources. Can. J. Soil Sci. 81, 175–183. doi: 10.4141/S00-666
Keywords: no-tillage, P fractionation, legacy P, P source, P distribution
Citation: Nunes RS, de Sousa DMG, Goedert WJ, de Oliveira LEZ, Pavinato PS and Pinheiro TD (2020) Distribution of Soil Phosphorus Fractions as a Function of Long-Term Soil Tillage and Phosphate Fertilization Management. Front. Earth Sci. 8:350. doi: 10.3389/feart.2020.00350
Received: 13 January 2020; Accepted: 28 July 2020;
Published: 02 September 2020.
Edited by:
Christophe Darnault, Clemson University, United StatesReviewed by:
Leon Etienne Parent, Laval University, CanadaCopyright © 2020 Nunes, de Sousa, Goedert, de Oliveira, Pavinato and Pinheiro. This is an open-access article distributed under the terms of the Creative Commons Attribution License (CC BY). The use, distribution or reproduction in other forums is permitted, provided the original author(s) and the copyright owner(s) are credited and that the original publication in this journal is cited, in accordance with accepted academic practice. No use, distribution or reproduction is permitted which does not comply with these terms.
*Correspondence: Rafael de Souza Nunes, cmFmYWVsLm51bmVzQGVtYnJhcGEuYnI=
Disclaimer: All claims expressed in this article are solely those of the authors and do not necessarily represent those of their affiliated organizations, or those of the publisher, the editors and the reviewers. Any product that may be evaluated in this article or claim that may be made by its manufacturer is not guaranteed or endorsed by the publisher.
Research integrity at Frontiers
Learn more about the work of our research integrity team to safeguard the quality of each article we publish.