- 1Dipartimento di Scienze della Terra, dell’Ambiente e delle Risorse, Università degli Studi di Napoli Federico II, Naples, Italy
- 2PalaeoHub, Department of Archaeology, University of York, Heslington, United Kingdom
- 3Function, Evolution and Anatomy Research Lab, Zoology Division, School of Environmental and Rural Science, University of New England, Armidale, NSW, Australia
- 4Research Centre in Evolutionary Anthropology and Palaeoecology, School of Biological and Environmental Sciences, Liverpool John Moores University, Liverpool, United Kingdom
- 5Department of Earth Sciences, University of Florence, Florence, Italy
- 6Dipartimento di Bioscienze e Territorio, University of Molise, Pesche, Italy
- 7Department of Environmental Biology, Sapienza University of Rome, Rome, Italy
Modern humans have larger and more globular brains when compared to other primates. Such anatomical features are further reflected in the possession of a moderately asymmetrical brain with the two hemispheres apparently rotated counterclockwise and slid anteroposteriorly on one another, in what is traditionally described as the Yakovlevian torque. Developmental disturbance in human brain asymmetry, or lack thereof, has been linked to several cognitive disorders including schizophrenia and depression. More importantly, the presence of the Yakovlevian torque is often advocated as the exterior manifestation of our unparalleled cognitive abilities. Consequently, studies of brain size and asymmetry in our own lineage indirectly address the question of what, and when, made us humans, trying to trace the emergence of brain asymmetry and expansion of cortical areas back in our Homo antecedents. Here, we tackle this same issue by studying the evolution of human brain size, shape, and asymmetry on a phylogenetic tree including 19 apes and Homo species, inclusive of our fellow ancestors. We found that a significant positive shift in the rate of brain shape evolution pertains to the clade including modern humans, Neanderthals, and Homo heidelbergensis. Although the Yakovlevian torque is well evident in these species and levels of brain asymmetry are correlated to changes in brain shape, further early Homo species possess the torque. Even though a strong allometric component is present in hominoid brain shape variability, this component seems unrelated to asymmetry and to the rate shift we recorded. These results suggest that changes in brain size and asymmetry were not the sole factors behind the fast evolution of brain shape in the most recent Homo species. The emergence of handedness and early manifestations of cultural modernity in the archeological record nicely coincide with the same three species sharing the largest and most rapidly evolving brains among all hominoids.
Introduction
The evolution of the human brain is one of the most intensely investigated topics in anthropology. Most studies on the subject matter focus on the achievement of our outstanding brain size (Rilling, 2006); fewer more focus on brain shape, which is intrinsically hard to study given it takes producing skull endocasts of our ancestors that come short in numbers and are not always easily accessible (Holloway, 2018). Recent developments in virtual anthropology (Weber, 2014) are now making fossil human endocasts a less rare commodity (Bruner et al., 2018), so that we are gaining scientific knowledge on our brain evolution at an unprecedented rate (Falk et al., 2000; Zollikofer and De León, 2013). Paralleling such increasing availability of brain endocasts, phylogenetic comparative methods offer ever better opportunities to study the rate and direction of phenotypic evolution, allowing the inclusion of fossil forms in studies of trait evolution. This is a key addition as the inclusion of fossils to extant-species phylogenies provides better understanding of the tempo and mode of evolution (Slater et al., 2012; Puttick, 2016; Schnitzler et al., 2017).
Recent studies on endocranial volume (i.e., the best proxy for brain size) in hominins invariably point to the presence of phenotypic leaps coinciding with the appearance of Homo (Du et al., 2018), although several lines of evidence indicate that not all Homo species belong to this “unusually big-brained” class of species, the latter being restricted to Homo heidelbergensis, Homo neanderthalensis, and Homo sapiens (Ruff et al., 1997; Rightmire, 2004; Profico et al., 2017; Diniz-Filho et al., 2019). These studies point to a non-gradual process of brain increase along the hominin lineage, probably prompted by the causal association between speciation and brain size (Du et al., 2018; Melchionna et al., 2019; Rocatti and Perez, 2019; Sansalone et al., 2020). These recent findings are slowly superseding earlier reports describing a pattern of gradual brain size increase in hominins (e.g., Lee and Wolpoff, 2016).
Our understanding of the evolution of brain shape in the human lineage might be experiencing a reverse trend. Despite logical enthusiasm around early findings illustrating an exquisitely human brain shape and level of brain asymmetry (Holloway, 1981; Holloway and De La Coste-Lareymondie, 1982), it has been later noted that the typical brain shape in H. sapiens, which is characterized by a strong left-occipital right-frontal asymmetry known as the Yakovlevian torque (Toga and Thompson, 2003) or occipital bending (LeMay, 1976; Holloway and De La Coste-Lareymondie, 1982; Chance and Crow, 2007; Balzeau et al., 2013), is present to a degree in both fossil human species and great apes (Gannon et al., 1998; Balzeau and Gilissen, 2010; Frayer et al., 2016; Neubauer et al., 2020). This casts doubt on the link between brain asymmetry and properly human cognitive abilities and still suggests that the evolution of human brain shape is best viewed as a gradual process toward exaggerated asymmetry and large size (Balzeau and Gilissen, 2010; Corballis, 2010; Gomez-Robles et al., 2013; Neubauer et al., 2018). However, the observation by Xiang et al. (2019) that torque magnitude is independent of brain size variation within H. sapiens and repeated findings that brain asymmetry is more variable in humans than in apes (Balzeau et al., 2012a; Neubauer et al., 2020) challenge this view and point to either a punctuational evolutionary event (Crow, 1993; Hou et al., 2019; Xiang et al., 2019) or a shift in the rate of evolution adding variability in humans (Balzeau et al., 2012a; Neubauer et al., 2020).
Here, we used 3D geometric morphometrics to study brain size and shape evolution in Hominoidea using 123 cranial endocasts belonging to 19 different extant and fossil species, including Australopithecus africanus, Homo ergaster, Homo erectus, H. heidelbergensis, and H. neanderthalensis. We built a phylogenetic tree for the species in the study sample and applied a phylogenetic comparative method especially thought to work with phylogenetic hypotheses including paleontological data. We computed the rate of brain shape evolution and searched for possible rate shifts, and how they correlate to levels of brain asymmetry. We eventually tested whether there is a significant allometric component in brain shape variation.
Materials and Methods
Endocasts, Volumes, and Landmark Configuration
We included 123 skull digital models of both extinct and extant species in the analysis. The fossil specimens are Sts 5 (A. africanus); KNM-ER 3733 and KNM-ER 3883 (H. ergaster); Ngandong 7, Ngandong 12, Sambungmacan 3, Zhoukoudian DI, and Zhoukoudian LIII (H. erectus); Kabwe 1, Petralona, and Atapuerca 4 (H. heidelbergensis); Saccopastore 1, La Chapelle-aux-Saints, and La Quina 5 (H. neanderthalensis); and Abri Pataud, Chancelade 1, Cro-Magnon 1, Mladeè 1, and Skhul V (anatomically modern humans). We also included endocasts of 10 modern H. sapiens, 10 Pan troglodytes, 10 Gorilla beringei, 10 Gorilla gorilla, six Pongo abelii, eight Hylobates agilis, nine Hydrophis klossi, 10 Hylobates lar, 10 Hylobates muelleri, four Hoolock hoolock, five Hylobates pileatus, eight Symphalangus syndactylus, two Nomascus concolor, and two Nomascus leucogenys. To produce the digital endocasts, we used the CT scans of crania belonging to living species from the Smithsonian Institute and the Digital Morphology Museum, Kupri1, repositories. We first reconstructed the cranial surfaces by using the software Amira® (version 5.4.5, Visualization Sciences Group, ©20132). Endocasts were obtained within the R software environment by using the endomaker function (Profico et al., 2020). The function works by reproducing the inner surface of the cranial cavity. It is based on an automatic reconstruction procedure named AST-3D (Profico et al., 2018). The procedure consists in reproducing the inner surface starting from multiple points of view (POVs) manually placed inside the cavity. The advantage of endomaker is that it performs the AST-3D algorithm automatically on brain case, without placing POVs. The function further computes the endocranial volume (i.e., the actual volume of the digital endocast) calling the volendo function of the Arothron R package. volendo calculates the 3D space occupied by the digital endocast discretized into voxels, identifying the voxels inside the mesh as those included within a concave-hull containing the mesh of the endocast and then computing the sum of inner voxels.
We extracted the cranial endocasts of Sts 5, Petralona, Saccopastore 1, Ngandong 7, Ngandong 12, KNM-ER-3733, and KNM-ER-3883 by using the Arothron R package (Figure 1). The other hominin fossil specimens are either manually segmented 3D models or laser-scanned physical casts (details are reported in Supplementary Table S1).
To register endocast shapes, we manually collected from each specimen 16 landmarks by using Amira (Supplementary Table S2). Then, 1000 bilateral semilandmarks (500 on each side) were automatically placed and slid in the R software environment by using the Morpho R package (Schlager, 2017) (Figure 2).
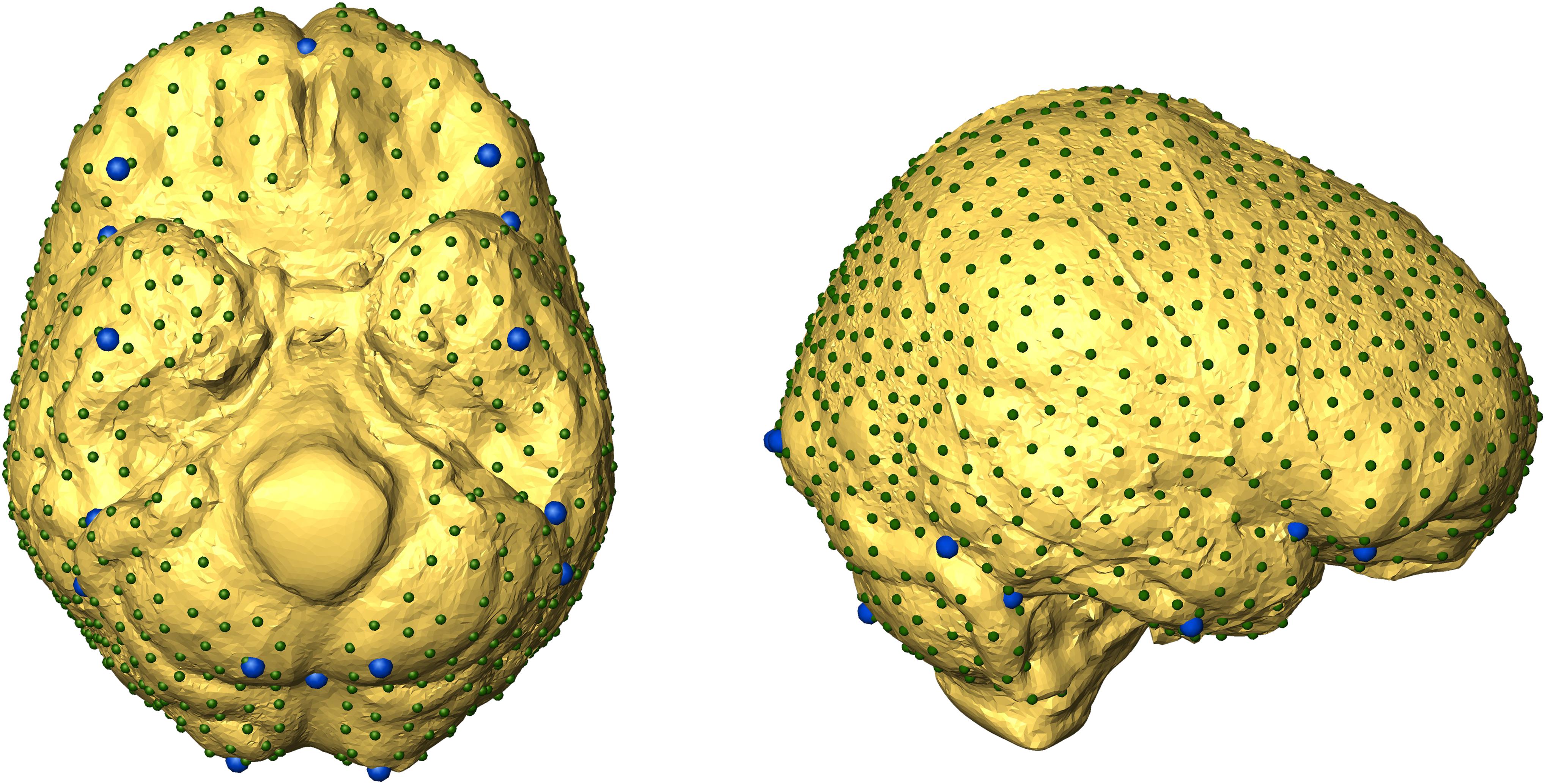
Figure 2. Landmark configurations used in the present work. Fixed landmarks (in blue) were manually defined. Semilandmarks are shown smaller and in dark green.
Some fossil specimens may suffer distortion by the taphonomic process, including missing portions. We did not include deformed specimens as they miss the relevant phenotypic information. However, in order to maximize sample size, we applied shape mirroring some specimens but nonetheless excluded them from the analysis of brain asymmetry as the mirroring erases such information. In particular, Kabwe I, La Quina 5, and KNM-ER 3883 are incomplete. In Kabwe I, small portions of the right occipital and parietal lobe are missing. A portion of the left cerebellum is missing in KNM-ER 3883. La Quina 5 lacks the entire base and a portion of the left frontoparietal lobe. Whenever we were not able to sample specific landmarks due to missing parts, the configurations were fixed by using the Morpho function fixLMmirror, estimating missing landmarks from their bilateral counterparts. This procedure is based on the reflecting and relabeling technique (Gunz et al., 2009). More specifically, the landmark configuration is mirrored, left and right landmarks are relabeled, and the missing data are imputed by deforming the mirrored version to the original one (Schlager, 2017). The same procedure was applied on the semilandmark sets after the sliding step. As this technique involves mirroring, the hominin specimens Kabwe I, La Quina 5, and KNM-ER 3883 were excluded from the asymmetry analysis.
Procrustes Analysis and RRphylo
We computed the mean landmark configuration per species. To this aim, we first performed a preliminary alignment of the specimens via Generalized Procrustes Analysis (GPA) (Gower, 1975) using the function procGPA in Morpho (Schlager, 2017). Subsequently, we computed the mean shape configuration per species.
To analyze the differences in brain shape among different species, we performed a Procrustes superimposition and a principal component analysis (PCA) on the vectorized landmark sets through the Morpho function procSym. This function returns both aligned coordinates and scores from the PCA.
In order to analyze the rate of brain shape evolution, we computed the phylogenetic tree for the species under analysis by using the phylogenetic hypotheses implemented in Meloro et al. (2015) and Melchionna et al. (2019). To search for evolutionary shifts in brain shape, we applied the RRphylo function in the R package RRphylo (Raia et al., 2020). The function relies on ridge regression to compute phenotypic evolutionary rates for each branch of the phylogeny and to estimate ancestral phenotypes with either univariate or multivariate data (Castiglione et al., 2018). In the present framework, we used all the principal component (PC) scores retrieved from the PCA pooled by species as phenotypic data, along with the Hominidae tree including the 19 analyzed species as the phylogeny (see Supplementary Material). Individual clades within the phylogeny were tested for the presence of rate shifts in brain shape evolution by applying the function search.shift. This function locates nodes subtending to clades possessing significantly higher/lower absolute phenotypic rate values by using a two-tailed permutation test (Castiglione et al., 2018). Since brain shape variations might possess a strong allometric component (Aristide et al., 2016), and brain asymmetry (see below) might represent an important source of brain shape variation, we performed the analysis by using once the endocranial volume and once the mean asymmetry per species as predictors. This is currently implemented in the multiple regression version of RRphylo (Melchionna et al., 2019; Serio et al., 2019). The analysis without endocranial volume taken as a prediction (pure shape) is presented as part of the Supplementary Material.
We analyzed the evolution of brain size using the mean endocranial volume per species as the phenotype and scanned the phylogeny and data seeking after significant shifts in the endocranial volume. The same procedure was applied to brain asymmetry. Eventually, to assess the presence of allometry in the brain shape data, we regressed brain shape against the mean endocranial volumes by means of generalized phylogenetic least squares regression (multivariate PGLS). We applied two different PGLS models. The first implements classic PGLS by using the procD.gls function in geomorph (Adams et al., 2019). The second rescales the tree branch lengths according to the multivariate rate variation across the tree branches as calculated by RRphylo before running procD.gls. These implementations are available in the function PGLS_fossil in the RRphylo package.
Measuring Asymmetry in Cranial Endocasts
Bilateral endocast asymmetry was assessed by comparing the brain morphology on the right and left sides. We used the same landmark and semilandmark configurations shown in Section “Endocasts, Volumes, and Landmarks Configuration.” In order to record differences in shape between the two halves of the endocast, we defined a set of 1000 semilandmarks, sampling 500 homologous points on each side. We built a symmetric reference for semilandmark placing and sliding procedures. In this way, the slid semilandmarks can be used as a proxy to calculate the difference in shape between right and left sides in all the specimens composing the sample.
After the sliding procedure, we applied the following procedure to each bilateral semilandmark configuration:
i. Calculation of the rotation matrix to mirror, scale, and align (ordinary Procrustes analysis step) the left side into the right side and vice versa following Klingenberg et al. (2002) (Figure 3);
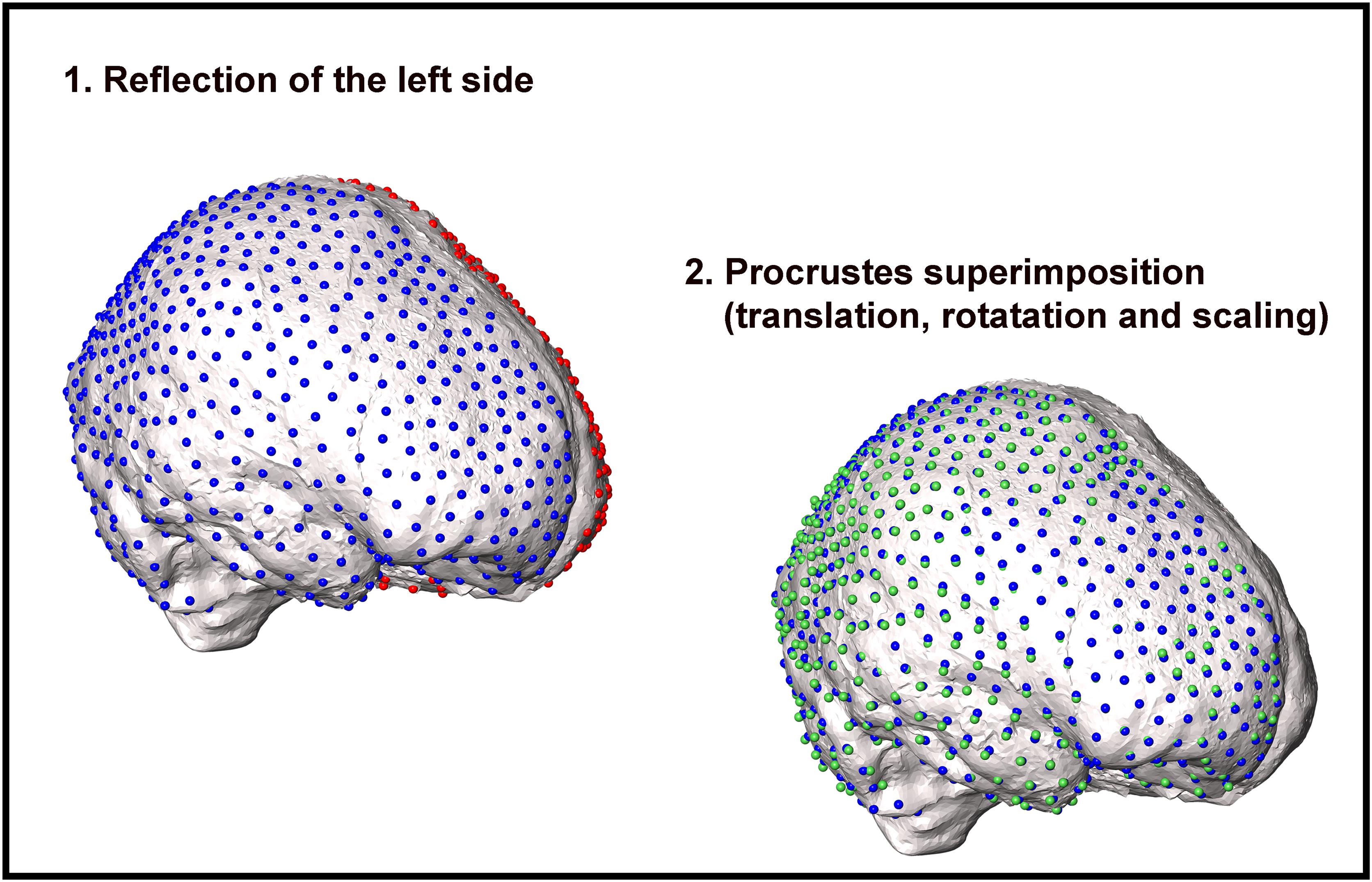
Figure 3. Workflow illustrating the procedure to compute bilateral asymmetry in cranial endocasts. First, the semilandmark configuration of one side (red spheres on the left side) is reflected on the other hemisphere (green spheres). Second, the mirrored semilandmark configuration is scaled to the centroid size of the right side (blue spheres). Third, the configurations are superimposed and rotated around the common centroid to achieve the best fit between the corresponding semilandmarks. For graphical purposes, the procedure is shown on one side only.
ii. Definition of a matrix of differences between the symmetric semilandmark configuration and the original one;
iii. Definition of a matrix of difference between right and mirrored left sides and vice versa.
At the end of this process, we obtained two suitable matrices to analyze the asymmetry of the cranial endocasts. We explored the relation between asymmetry and shape by applying a partial least squares (PLS). We defined as first block the endocast shape and as second block the matrix of asymmetry calculated on each specimen. The endocast shape consists in the semilandmark configurations after the GPA analysis (scaling included). We perform also a PLS on a less dense semilandmark configuration (50 semilandmarks on each side).
Results
The PCA results are shown in Figure 4 (PC1 vs PC2). On the PC1 (which explains 35.93% of the total variance), the overall shape goes from flattened endocasts (PC1 positive values) to more globular shapes with a rounded and expanded frontoparietal region. The occipital lobes become markedly asymmetric at PC1 negative values. Along the PC1, there is a separation between the most recent species of Homo (H. heidelbergensis, H. neanderthalensis, and H. sapiens) and the other hominids. Positive values of PC2 correspond to a brain shape that narrows in the temporal area (which is typical of the Australopithecus brain morphology), whereas at negative values of the PC2, the brain endocast flattens dorsoventrally.
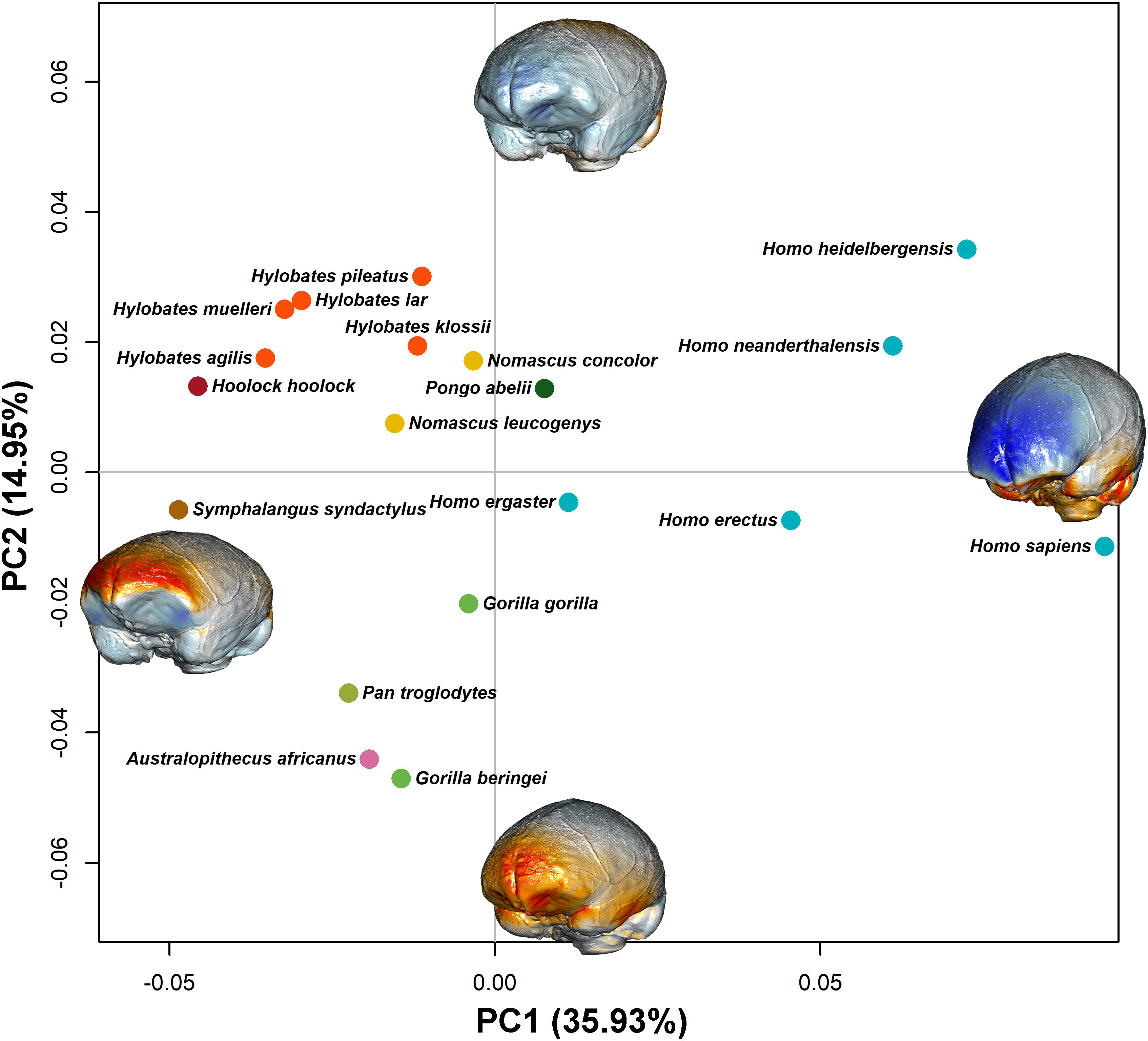
Figure 4. PC1 and PC2 scores are shown. Species represents the mean shape configuration between samples after alignment. They are colored by genus. The endocasts represent the shape at the extreme of the PC axis (endocasts are not in scale). Blue tones represent expansion from the consensus shape, and orange/red tones represent contraction. The two PCs explain, respectively, 35.93 and 14.95% of the total variation.
Brain volume (per unit mass) shows a negative rate shift coinciding with the genus Hylobates (ptwo–tailed < 0.001; Supplementary Figure S1). Interestingly, brain shape asymmetry shows a positive rate shift regarding the species of the genus Homo (ptwo–tailed = 0.979; Figure 5, right). When the average brain volume is considered as a predictor in RRphylo, there is a positive and significant rate shift in brain shape evolution for the clade including H. heidelbergensis, H. neanderthalensis, and H. sapiens (ptwo–tailed = 0.999; Figure 5, left) and a negative and significant rate shift for the Hylobatidae clade (ptwo–tailed < 0.001; Figure 5, left). When brain asymmetry is considered as a predictor in RRphylo, the results do not change; there is a positive and significant rate shift in brain shape evolution for the clade including H. heidelbergensis, H. neanderthalensis, and H. sapiens (ptwo–tailed = 0.999) and a negative and significant rate shift for the Hylobatidae clade (ptwo–tailed < 0.001). Eventually, the same two rate shifts still apply when shape is analyzed on its own, ignoring the allometric component.
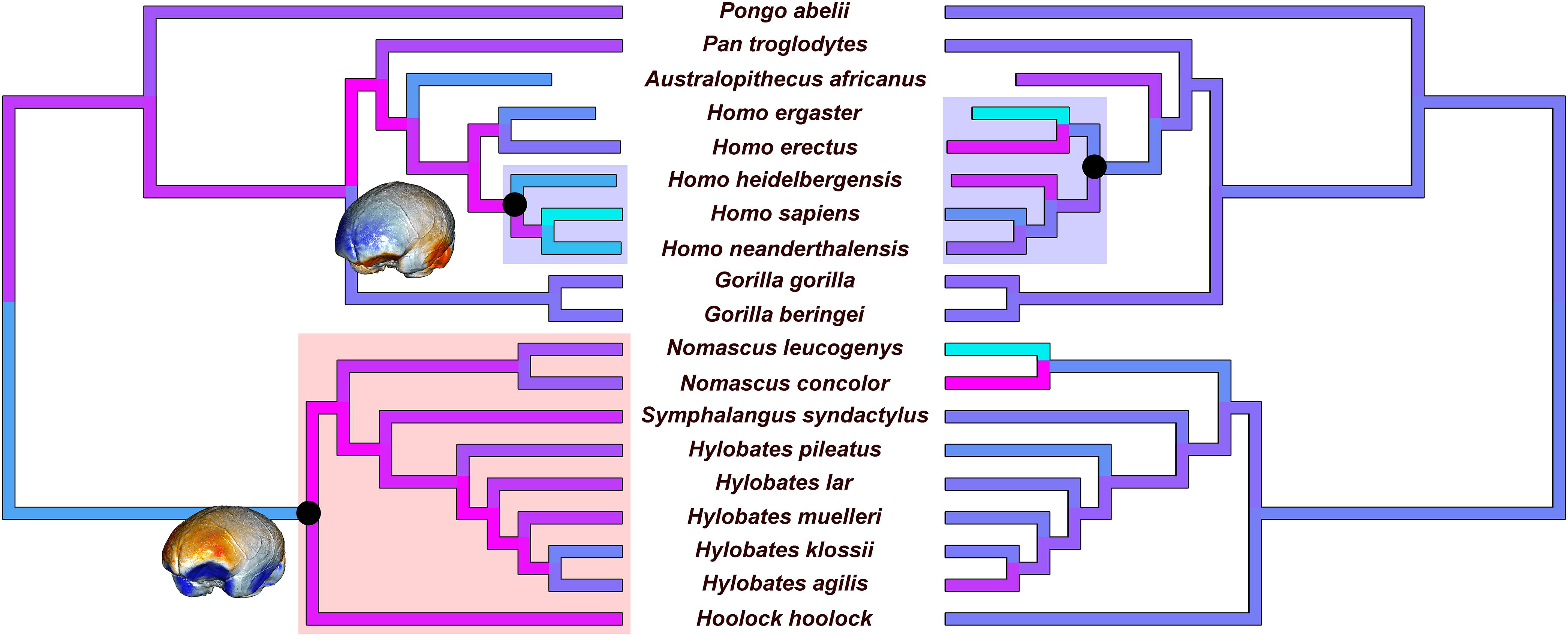
Figure 5. Results of the evolutionary rate analysis of brain shape when mean volume per species is used as a predictor (left). The reconstruction of the endocast shape of the common ancestors is provided (endocasts are not in scale) for the tree root and for the common ancestor of modern Homo species. Results of the rate analysis of the average asymmetry values are shown on the right side. Evolutionary rates are scaled for the branches of the tree from higher (cyan) to lower (magenta) values. Clades that show significantly higher and lower rates are highlighted with blue and red rectangles, respectively.
We found a positive allometric scaling between the brain shape and the endocast volume, either using the Brownian motion (p = 0.001) or allowing the evolutionary rates to change across the tree with RRphylo (p = 0.001).
The PLS performed between endocast shape and asymmetry highlights a significant correlation between the two blocks. The correlation coefficient related to the first axis is equal to 0.56, and the p-value is equal to 0.010 as assessed by a permutation test (N = 1000). The correlation coefficient of the second axis is equal to 0.45, and the p-value is equal to 0.001 (see Supplementary Figure S2). On the first PLS axis, Homo erectus and Middle to Late Pleistocene humans (i.e., H. neanderthalensis, H. heidelbergensis, and H. sapiens) are located at negative values of both axes (shape and asymmetry, Figure 6). The Hylobatidae are found at positive values of blocks 1 and 2. The first axis of covariation (PLS1, block 1) explains 14.23% of the total variance of the endocast shape. The shape variations related to the genus Homo show as in this group of species that the left hemisphere is larger (see Figure 6 and Supplementary Figure S3) with an expansion of the right parietal lobe.
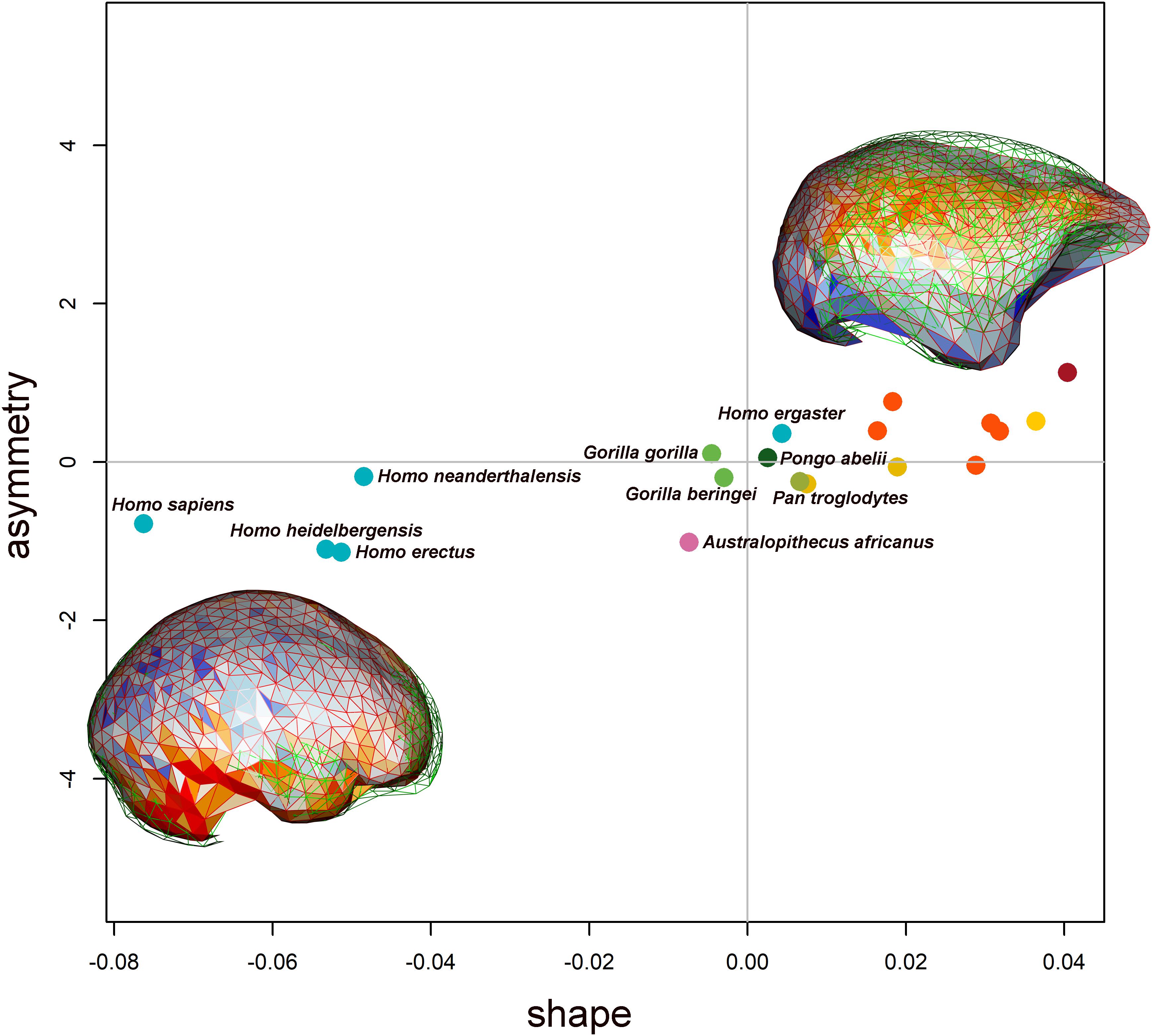
Figure 6. Biplot showing the first axis of the PLS. On the x-axis is the endocast shape, and on the y-axis is the endocast asymmetry. The species are reported with the same dot colors reported in Figure 4. The shape variations related to both shape and asymmetry are reported at the extreme of the x- and y-axes. The displacement between the right (wireframe in red) and left (wireframe in green) sides is shown overlapped to the colored mesh, indicating the local deformation. Warm and cold colors indicate, respectively, which regions are more contracted and expanded in the right side compared with the left one.
On the second axis of the PLS (see Supplementary Figure S2), the morphology associated at positive values highlights the right hemisphere extending anteriorly, according to the classical Yakovlevian torque pattern. At negative values of both axes, the endocast morphology shows a distortion along the midsagittal profile with a greater asymmetry in the superior parietal region and in the anterior portion of the frontal lobe. By reducing the number of semilandmarks on each hemisphere from 500 to 50, we observe the same pattern of shape variations in the PLS analysis (see Supplementary Figures S6, S7).
Discussion
Humans are often noted for their exceptional brain size and extremely developed cognitive abilities. It has long been noted that our brain is strongly asymmetric, with evident differences between the left and right sides (Leuret and Gratiolet, 1839). The left cerebral hemisphere often extends posteriorly beyond the right, and the latter extends beyond the left frontally, originating the so-called petalia (Galaburda et al., 1978; Toga and Thompson, 2003; Balzeau and Gilissen, 2010). This asymmetric structure is often described as the Yakovlevian torque (Yakovlev and Rakic, 1966). The torque is more than a mere distortion of the bilateral symmetry of the brain. Several studies have indicated that a significant relationship exists between brain asymmetry and the lateralization of cognitive functions such as handedness and linguistic processing. In modern humans, the right frontal (RF) protrusion and left occipital (LO) protrusion are statistically correlated with right-handedness in 85–90% of individuals (Cashmore et al., 2008; Häberling and Corballis, 2016), whereas the reverse pattern is associated to some degree with left-handedness and ambidexterity (Cashmore et al., 2008; Grimaud-Herve and Lordkipanidze, 2010). Lateralization is further observed in hemispheric language dominance. Approximately 95% of right-handed individuals have left-hemispheric dominance for language (Rasmussen and Milner, 1977; Pujol et al., 1999; Szaflarski et al., 2002). A strong variation in the degree of Yakovlevian torque is associated with several functional disorders like depression and schizophrenia (Mackay et al., 2010; Maller et al., 2015; Liu et al., 2016). In schizophrenia, in particular, cerebral asymmetry is reduced, and language processing is less lateralized than in non-schizophrenic subjects (Sommer et al., 2001). This evidence testifies to the intimate connection between the pattern of structural brain asymmetry and lateralization in the development of higher cognitive abilities (Walker, 1980; Gevins et al., 1983; Lefebvre et al., 2004).
Brains of non-human anthropoids are also functionally lateralized to a degree (Holloway, 1981; Cantalupo and Hopkins, 2001; Wu et al., 2006; Falk, 2007; Hofman and Falk, 2012; Gomez-Robles et al., 2013; Hopkins et al., 2015; Poza-Rey et al., 2017). Hylobatids possibly show higher frequency of left- than right-handers (Morino et al., 2017). The great apes present specific patterns of hemispheric asymmetry, which is less frequent than in humans and rarely involves both the frontal and the occipital lobes. Some scholars (Prieur et al., 2016, 2019; Marie et al., 2018) noted the presence of a low-frequency right-handedness bias in bimanual tasks in chimpanzees and gorillas (Hopkins et al., 2019). Although several studies (McGrew and Marchant, 2001; Mosquera et al., 2007; Harrison and Nystrom, 2008) indicate that great apes do not display any hand preference at the population level (Fitch and Braccini, 2013; Uomini, 2015), some groups can have a majority of left-handers (Cashmore et al., 2008; Uomini, 2009; Chapelain et al., 2011), chimpanzee groups were reported to be predominantly right-handed (Hopkins et al., 2004), and even in monkeys, handedness is related to hemispheric specialization (Margiotoudi et al., 2019).
With so much emphasis on brain asymmetry and its relationship to cognition, it is unsurprising that profound effort has been devoted to studying fossil endocasts so as to reveal the evolution of brain asymmetry in hominids and non-human apes. Different studies now point in the direction that a clearly asymmetrical brain pertains to modern human species, whereas the status of australopiths and early Homo is much less obvious (Tobias, 1987; Rightmire, 2004; Wu et al., 2006). Brain asymmetry is documented in Paleolithic H. sapiens, in H. neanderthalensis (albeit the endocast of La Chapelle-aux-Saints has a reversed RO/LF petalia pattern), and in Middle Pleistocene H. heidelbergensis (Holloway et al., 2004). A reversed RO/LF petalia pattern has been described in Homo floresiensis (Falk et al., 2005) as well in KNM-ER 1813, in some specimens of H. erectus from Asia (Sangiran 2, Sangiran 10, Sangiran 17, and Ckn.L.2) and in D2280 from Dmanisi, while D2282 is described as possessing an RF/LO pattern (Grimaud-Herve and Lordkipanidze, 2010) like four of the five endocasts of H. erectus from Ngandong (Poza-Rey et al., 2017). A human-like pattern is also described in Homo rudolfensis KNM-ER 1470 (Holloway, 1983) and H. ergaster KNM-ER 3733, KNM-ER 3883, and KNM-WT 15000 (Holloway et al., 2004). Small RF/LO petalias have been described for A. africanus, Paranthropus boisei, and Paranthropus aethiopicus (Holloway et al., 2004), while the Homo habilis specimens from Olduvai usually lack RF/LO petalias (Tobias, 1987; Holloway et al., 2004, but see Frayer et al., 2016). Despite this wealth of data apparently suggesting a continuous development of brain asymmetry throughout human evolution, the precise quantification of the magnitude of the asymmetry and its evolutionary significance in terms of cognitive abilities remain elusive (Balzeau et al., 2020). Furthermore, given the 3D geometric complexity of the torque, its recognition on endocranial casts by using traditional linear measurements as in these studies is often prone to a consistent degree of uncertainty. Our analysis based on 3D geometric morphometrics overcomes this limitation and offers the first integrated glimpse on the rates of evolutionary change of brain shape in the different branches of the Hominoidea. Although the allometric component of brain shape change is strong, the pattern is not linear. Rates of the shape change are distinctly lower for Hylobatidae and distinctly higher for the clade including H. heidelbergensis, H. neanderthalensis, and H. sapiens. The early Homo (H. erectus + H. ergaster) and Australopithecus, conversely, are in line with the apes (Figures 4, 6), although early Homo show the same level of asymmetry as later species in the genus, resulting in a significant rate shift in the asymmetry level coinciding with the genus (Figure 5, right). This means that the relative expansion of brain size in Middle to Late Pleistocene Homo is accompanied by a major reorganization in the brain morphology that goes beyond sheer allometric effects and asymmetry and possibly keeps changing in H. sapiens (Bastir et al., 2008; Neubauer et al., 2018; Weaver and Gunz, 2018; Gunz et al., 2019) and H. neanderthalensis (Balzeau et al., 2012b; Mounier et al., 2016).
The pattern of low evolutionary rates in hylobatids is consistent with their evolutionary history and ecologies. The origin of Hylobatidae is placed at 21.8 Ma, and the radiation of the clade occurred between 6.4 and 8.0 Ma (Israfil et al., 2011). Israfil et al. (2011) suggest that such rapid radiation could be paired with biogeographic factors as the population dispersal and variations in the density of the forestal habitat (vicariant speciation). The low degree of body size differentiation and relatively minor ecological diversity when compared to great apes might be responsible for the apparently slow rate of brain shape evolution in this group.
Along the human lineage, the shifts in evolutionary rate in brain size evolution (Du et al., 2018; Diniz-Filho et al., 2019) and increased rates of brain shape evolution (this study) coincide, thus suggesting that brain evolution followed a distinctive path joining H. heidelbergensis, H. neanderthalensis, and H. sapiens. This is entirely consistent with the archeological record of human behavior. Numerous studies on the anterior dentition in H. heidelbergensis, Neanderthals, and Paleolithic modern humans show a predominant right-hand frequency (> 90%), similar to the pattern for living humans attributed to right-handers (de Castro et al., 1988; Lozano et al., 2009; Frayer et al., 2012; Poza-Rey et al., 2017). Studies of experimental production of lithic stone tools demonstrate that brain lateralization is involved in the production of late Acheulean tools or when participants watch Acheulean tool production, but not when participants make or watch others making Oldowan tools (Stout, 2011; Stout and Khreisheh, 2015). Uomini and Meyer (2013) suggested that the same areas for stone tool manufacture, of the Acheulean in particular, are lateralized and located on the left side in brain areas also involved in language processing and social learning.
It is intriguing that the birth of symbolic culture, marked by the use of pigments and personal ornamentation, traces back to the Middle Stone Age with Acheulean tradition at some 300 ka and is itself consistent with human fossil specimens belonging to the species H. heidelbergensis (Henshilwood et al., 2002; d’Errico, 2003; d’Errico and Henshilwood, 2007; Manzi, 2016) and H. neanderthalensis (Zilhão et al., 2010). It must be noted, though, that the indication for symbolic culture in species other than H. sapiens is controversial to say the least (Mcbrearty and Brooks, 2000; White et al., 2019).
Here, we demonstrate that the pace of brain shape evolution shifted since the appearance of the common ancestor to H. heidelbergensis, H. neanderthalensis, and H. sapiens. Although these species share a large brain, levels of brain asymmetry were comparatively large in earlier Homo species, and allometric effects cannot entirely account for the shift toward the faster rate of brain shape evolution in the Middle to Late human species. Remarkably, the rate shift coincides with archeological evidence for the emergence of symbolic culture.
Data Availability Statement
All datasets generated for this study are included in the article/Supplementary Material.
Author Contributions
PR, MM, and AP conceived the study. MM, AP, SC, and CS performed the analyses. All authors contributed to the article and approved the submitted version.
Conflict of Interest
The authors declare that the research was conducted in the absence of any commercial or financial relationships that could be construed as a potential conflict of interest.
Acknowledgments
We are grateful to Costantino Buzi, Chiara Iacuzzo, and Maria Modafferi for sharing some of their own work on fossil endocasts with us.
Supplementary Material
The Supplementary Material for this article can be found online at: https://www.frontiersin.org/articles/10.3389/feart.2020.00273/full#supplementary-material
This file includes:
The phylogenetic tree used in the analyses in Newick format.
FIGURE S1 | Results of the evolutionary rate analysis on the average volumes.
FIGURE S2 | Biplot showing the second axis of the PLS.
FIGURE S3 | Variation in centroid size between right and left hemisphere within the genus Homo.
FIGURE S4 | Variation in centroid size between right and left hemisphere in great apes (Homo excluded) plus Australopithecus africanus.
FIGURE S5 | Variation in centroid size between right and left hemisphere in lesser apes.
FIGURE S6 | Biplot showing the first axis of the PLS using a subset of 100 semilandmarks (50 on each side).
FIGURE S7 | Biplot showing the second axis of the PLS using a subset of 100 semilandmarks (50 on each side).
TABLE S1 | List of materials used in this study.
TABLE S2 | Definition of landmarks acquired on each endocast.
DATA.rda: an RData file containing the phylogenetic tree, the landmark and semilandmark sets used in the analyses of the endocast shape (123 specimens), the semilandmark sets used in the asymmetry analyses (120 specimens), the vector of the corresponding ECV values and a mesh containing the triangulated semilandmark configuration.
script.R: The annotated script used to replicate the analyses reported in this study. The supplementary material is available on Melchionna et al. (2020). From smart apes to human brain boxes. A uniquely derived brain shape in late hominins clade: supplementary material. Zenodo: doi: 10.5281/zenodo.3931032.
Footnotes
- ^ http://dmm.pri.kyoto-u.ac.jp/dmm/WebGallery/index.html
- ^ https://www.fei.com/software/amira-for-life-sciences/
References
Adams, D. C., Collyer, M., Kaliontzopoulou, A., and Sherratt, E. (2019). geomorph: Software for Geometric Morphometric Analyses. R Package Version 3.1.0. Available online at: https://cran.r-project.org/package=geomorph
Aristide, L., Dos Reis, S. F., Machado, A. C., Lima, I., Lopes, R. T., and Perez, S. I. (2016). Brain shape convergence in the adaptive radiation of new world monkeys. Proc. Natl. Acad. Sci. U.S.A. 113, 2158–2163. doi: 10.1073/pnas.1514473113
Balzeau, A., Ball-Albessard, L., and Kubicka, A. M. (2020). Variation and correlations in departures from symmetry of brain torque, humeral morphology and handedness in an archaeological sample of Homo sapiens. Symmetry 12:432. doi: 10.3390/sym12030432
Balzeau, A., and Gilissen, E. (2010). Endocranial shape asymmetries in Pan paniscus, Pan troglodytes and Gorilla gorilla assessed via skull based landmark analysis. J. Hum. Evol. 59, 54–69. doi: 10.1016/j.jhevol.2010.03.013
Balzeau, A., Gilissen, E., and Grimaud-Hervé, D. (2012a). Shared pattern of endocranial shape asymmetries among great apes, anatomically modern humans, and fossil hominins. PLoS One 7:e29581. doi: 10.1371/journal.pone.0029581
Balzeau, A., Holloway, R. L., and Grimaud-Hervé, D. (2012b). Variations and asymmetries in regional brain surface in the genus Homo. J. Hum. Evol. 62, 696–706. doi: 10.1016/j.jhevol.2012.03.007
Balzeau, A., Grimaud-Hervé, D., Détroit, F., Holloway, R. L., Combès, B., and Prima, S. (2013). First description of the Cro-Magnon 1 endocast and study of brain variation and evolution in anatomically modern Homo sapiens. Bull. Mem. Soc. Anthropol. Paris 25, 1–18. doi: 10.1007/s13219-012-0069-z
Bastir, M., Rosas, A., Lieberman, D. E., and O’Higgins, P. (2008). Middle cranial fossa anatomy and the origin of modern humans. Anat. Rec. 291, 130–140. doi: 10.1002/ar.20636
Cantalupo, C., and Hopkins, W. D. (2001). Asymmetric broca’s area in great apes. Nature 414, 505–505. doi: 10.1038/35107134
Cashmore, L., Uomini, N., and Chapelain, A. (2008). The evolution of handedness in humans and great apes: a review and current issues. J. Anthropol. Sci. 86, 1–30. doi: 10.1007/s10764-010-9484-5
Castiglione, S., Tesone, G., Piccolo, M., Melchionna, M., Mondanaro, A., Serio, C., et al. (2018). A new method for testing evolutionary rate variation and shifts in phenotypic evolution. Met. Ecol. Evol. 9, 974–983. doi: 10.1111/2041-210X.12954
Chance, S. A., and Crow, T. J. (2007). Distinctively human: cerebral lateralisation and language in Homo sapiens. J. Anthropol. Sci. 85, 83–100.
Chapelain, A. S., Hogervorst, E., Mbonzo, P., and Hopkins, W. D. (2011). Hand preferences for bimanual coordination in 77 Bonobos (Pan paniscus): replication and extension. Int. J. Primatol. 32, 491–510.
Corballis, M. C. (2010). “Handedness and cerebral asymmetry: an evolutionary perspective,” in Two Halves of the Brain: Information Processing in the Cerebral Hemispheres, eds K. Hugdahl and R. Westerhausen (Cambridge, MA: The MIT press), 65–88. doi: 10.7551/mitpress/9780262014137.003.0063
Crow, T. J. (1993). Sexual selection, machiavellian intelligence, and the origins of psychosis. Lancet 342, 594–598. doi: 10.1016/0140-6736(93)91415-i
de Castro, J. B., Bromage, T. G., and Jalvo, Y. F. (1988). Buccal striations on fossil human anterior teeth: evidence of handedness in the middle and early Upper Pleistocene. J. Hum. Evol. 17, 403–412. doi: 10.1016/0047-2484(88)90029-2
d’Errico, F. (2003). The invisible frontier. A multiple species model for the origin of behavioral modernity. Evol. Anthropol. 12, 188–202. doi: 10.1002/evan.10113
d’Errico, F., and Henshilwood, C. S. (2007). Additional evidence for bone technology in the southern African Middle Stone Age. J. Hum. Evol. 52, 142–163. doi: 10.1016/j.jhevol.2006.08.003
Diniz-Filho, J. A. F., Jardim, L., Mondanaro, A., and Raia, P. (2019). Multiple components of phylogenetic non-stationarity in the evolution of brain size in fossil hominins. Evol. Biol. 46, 47–59. doi: 10.1007/s11692-019-09471-z
Du, A., Zipkin, A. M., Hatala, K. G., Renner, E., Baker, J. L., Bianchi, S., et al. (2018). Pattern and process in hominin brain size evolution are scale-dependent. Proc. R. Soc. B 285:20172738. doi: 10.1098/rspb.2017.2738
Falk, D., Hildebolt, C., Smith, K., Morwood, M. J., Sutikna, T., Brown, P., et al. (2005). The brain of LB1, Homo floresiensis. Science 308, 242–245. doi: 10.1126/science.1109727
Falk, D., Redmond, J. C. Jr., Guyer, J., Conroy, C., Recheis, W., Weber, G. W., et al. (2000). Early hominid brain evolution: a new look at old endocasts. J. Hum. Evol. 38, 695–717. doi: 10.1006/jhev.1999.0378
Fitch, W. T., and Braccini, S. N. (2013). Primate laterality and the biology and evolution of human handedness: a review and synthesis. Ann. N. Y. Acad. Sci. 1288, 70–85. doi: 10.1111/nyas.12071
Frayer, D. W., Clarke, R. J., Fiore, I., Blumenschine, R. J., Pérez-Pérez, A., Martinez, L. M., et al. (2016). OH-65: the earliest evidence for right-handedness in the fossil record. J. Hum. Evol. 100, 65–72. doi: 10.1016/j.jhevol.2016.07.002
Frayer, D. W., Lozano, M., Bermúdez de Castro, J. M., Carbonell, E., Arsuaga, J. L., Radovčić, J., et al. (2012). More than 500,000 years of right-handedness in Europe. Laterality 17, 51–69. doi: 10.1080/1357650X.2010.529451
Galaburda, A. M., LeMay, M., Kemper, T. L., and Geschwind, N. (1978). Right-left asymmetrics in the brain. Science 199, 852–856. doi: 10.1126/science.341314
Gannon, P. J., Holloway, R. L., Broadfield, D. C., and Braun, A. R. (1998). Asymmetry of chimpanzee planum temporale: humanlike pattern of wernicke’s brain language area homolog. Science 279, 220–222. doi: 10.1126/science.279.5348.220
Gevins, A. S., Schaffer, R. E., Doyle, J. C., Cutillo, B. A., Tannehill, R. S., and Bressler, S. L. (1983). Shadows of thought: shifting lateralization of human brain electrical patterns during brief visuomotor task. Science 220, 97–99. doi: 10.1126/science.6828886
Gomez-Robles, A., Hopkins, W. D., and Sherwood, C. C. (2013). Increased morphological asymmetry, evolvability and plasticity in human brain evolution. Proc. R. Soc. B 280, 20130575–20130575. doi: 10.1098/rspb.2013.0575
Gower, J. C. (1975). Generalized procrustes analysis. Psychometrika 40, 33–51. doi: 10.1007/BF02291478
Grimaud-Herve, D., and Lordkipanidze, D. (2010). “The fossil hominid’s brain of dmanisi: D 2280 and D 2282,” in The Human Brain Evolving: Paleoneurological Studies in Honor of Ralph L. Holloway, eds K. Schick and N. Toth (Gosport, IN: Stone Age Institute Press), 59–82.
Gunz, P., Mitteroecker, P., Neubauer, S., Weber, G. W., and Bookstein, F. L. (2009). Principles for the virtual reconstruction of hominin crania. J. Hum. Evol. 57, 48–62. doi: 10.1016/j.jhevol.2009.04.004
Gunz, P., Tilot, A. K., Wittfeld, K., Teumer, A., Shapland, C. Y., van Erp, T. G. M., et al. (2019). Neandertal introgression sheds light on modern human endocranial globularity. Curr. Biol. 29, 120–127. doi: 10.1016/j.cub.2018.10.065
Häberling, I. S., and Corballis, M. C. (2016). Cerebellar asymmetry, cortical asymmetry and handedness: two independent networks. Laterality 21, 397–414. doi: 10.1080/1357650X.2015.1110161
Harrison, R. M., and Nystrom, P. (2008). Handedness in captive bonobos (Pan paniscus). Folia Primatol. 79, 253–268. doi: 10.1159/000113539
Henshilwood, C. S., d’Errico, F., Yates, R., Jacobs, Z., Tribolo, C., Duller, G. A. T., et al. (2002). Emergence of modern human behavior: middle stone age engravings from South Africa. Science 295, 1278–1280. doi: 10.1126/science.1067575
Hofman, A., and Falk, D. (2012). Evolution of the Primate Brain: From Neuron To Behavior, Vol. 195. Amsterdam: Elsevier.
Holloway, R. L. (1981). Volumetric and asymmetry determinations on recent hominid endocasts: spy I and II, Djebel Ihroud I, and the salè Homo erectus specimens, with some notes on neandertal brain size. Am. J. Phys. Anthropol. 55, 385–393. doi: 10.1002/ajpa.1330550312
Holloway, R. L. (1983). Human paleontological evidence relevant to language behavior. Hum. Neurobiol. 2, 105–114.
Holloway, R. L. (2018). “On the making of endocasts: the new and the old in paleoneurology,” in Digital Endocasts, eds E. Bruner, N. Ogihara, and H. C. Tanabe (Cham: Springer), 1–8. doi: 10.1007/978-4-431-56582-6_1
Holloway, R. L., and De La Coste-Lareymondie, M. C. (1982). Brain endocast asymmetry in pongids and hominids: some preliminary findings on the paleontology of cerebral dominance. Am. J. Phys. Anthropol. 58, 101–110. doi: 10.1002/ajpa.1330580111
Holloway, R. L., Yuan, M. S., and Broadfield, D. C. (2004). The Human Fossil Record: Brain Endocasts: the Paleoneurological Evidence. New York, NY: John Wiley & Sons Publishers.
Hopkins, W. D., Mareno, M. C., and Schapiro, S. J. (2019). Further evidence of a left hemisphere specialization and genetic basis for tool use skill in chimpanzees (Pan troglodytes): reproducibility in two genetically isolated populations of apes. J. Comp. Psychol. 133, 512–519. doi: 10.1037/com0000183
Hopkins, W. D., Misiura, M., Pope, S. M., and Latash, E. M. (2015). Behavioral and brain asymmetries in primates: a preliminary evaluation of two evolutionary hypotheses. Ann. N. Y. Acad. Sci. 1359, 65–83. doi: 10.1111/nyas.12936
Hopkins, W. D., Wesley, M. J., Izard, M. K., Hook, M., and Schapiro, S. J. (2004). Chimpanzees (Pan troglodytes) are predominantly right-handed: replication in three populations of apes. Behav. Neurosci. 118:659. doi: 10.1037/0735-7044.118.3.659
Hou, L., Xiang, L., Crow, T. J., Leroy, F., Rivière, D., Mangin, J.-F., et al. (2019). Measurement of sylvian fissure asymmetry and occipital bending in humans and Pan troglodytes. Neuroimage 184, 855–870. doi: 10.1016/j.neuroimage.2018.08.045
Israfil, H., Zehr, S. M., Mootnick, A. R., Ruvolo, M., and Steiper, M. E. (2011). Unresolved molecular phylogenies of gibbons and siamangs (family: hylobatidae) based on mitochondrial, Y-linked, and X-linked loci indicate a rapid Miocene radiation or sudden vicariance event. Mol. Phyl. Evol 58, 447–455. doi: 10.1016/j.ympev.2010.11.005
Klingenberg, C. P., Barluenga, M., and Meyer, A. (2002). Shape analysis of symmetric structures: quantifying variation among individuals and asymmetry. Evolution 56, 1909–1920. doi: 10.1111/j.0014-3820.2002.tb00117.x
Lee, S.-H., and Wolpoff, M. H. (2016). The pattern of evolution in pleistocene human brain size. Paleobiology 29, 186–196. doi: 10.1017/S0094837300018054
Lefebvre, L., Reader, S. M., and Sol, D. (2004). Brains, innovations and evolution in birds and primates. Brain Behav. Evol. 63, 233–246. doi: 10.1159/000076784
LeMay, M. (1976). Morphological cerebral asymmetries of modern man, fossil man, and nonhuman primate. Ann. N. Y. Acad. Sci. 280, 349–366. doi: 10.1111/j.1749-6632.1976.tb25499.x
Leuret, F., and Gratiolet, P.-L. (1839). Anatomie Comparée Du Système Nerveux: Considéré Dans Ses Rapports Avec L’intelligence. Paris: J.-B. Bailliere.
Liu, W., Mao, Y., Wei, D., Yang, J., Du, X., Xie, P., et al. (2016). Structural asymmetry of dorsolateral prefrontal cortex correlates with depressive symptoms: evidence from healthy individuals and patients with major depressive disorder. Neurosci. Bull. 32, 217–226. doi: 10.1007/s12264-016-0025-x
Lozano, M., Mosquera, M., De Castro, J. M. B., Arsuaga, J. L., and Carbonell, E. (2009). Right handedness of Homo heidelbergensis from sima de los huesos (Atapuerca, Spain) 500,000 years ago. Evol. Hum. Behav. 30, 369–376. doi: 10.1016/j.evolhumbehav.2009.03.001
Mackay, C. E., Roddick, E., Barrick, T. R., Lloyd, A. J., Roberts, N., Crow, T. J., et al. (2010). Sex dependence of brain size and shape in bipolar disorder: an exploratory study. Bipolar Disord. 12, 306–311. doi: 10.1111/j.1399-5618.2010.00804.x
Maller, J. J., Anderson, R., Thomson, R. H., Rosenfeld, J. V., Daskalakis, Z. J., and Fitzgerald, P. B. (2015). Occipital bending (Yakovlevian torque) in bipolar depression. Psychiatry Res. Neuroimag. 231, 8–14. doi: 10.1016/j.pscychresns.2014.11.008
Manzi, G. (2016). Humans of the middle pleistocene: the controversial calvarium from Ceprano (Italy) and its significance for the origin and variability of Homo heidelbergensis. Quat. Int. 411, 254–261. doi: 10.1016/j.quaint.2015.12.047
Margiotoudi, K., Marie, D., Claidière, N., Coulon, O., Roth, M., Nazarian, B., et al. (2019). Handedness in monkeys reflects hemispheric specialization within the central sulcus. An in vivo MRI study in right-and left-handed olive baboons. Cortex 118, 203–211. doi: 10.1016/j.cortex.2019.01.001
Marie, D., Roth, M., Lacoste, R., Nazarian, B., Bertello, A., Anton, J., et al. (2018). Left brain asymmetry of the planum temporale in a nonhominid primate: redefining the origin of brain specialization for language. Cereb. Cortex 28, 1808–1815. doi: 10.1093/cercor/bhx096
Mcbrearty, S., and Brooks, A. S. (2000). The revolution that wasn’t: a new interpretation of the origin of modern human behavior. J. Hum. Evol. 39, 453–563. doi: 10.1006/jhev.2000.0435
McGrew, W. C., and Marchant, L. F. (2001). Ethological study of manual laterality in the chimpanzees of the mahale mountains, Tanzania. Behaviour 138, 329–358. doi: 10.1163/15685390152032497
Melchionna, M., Mondanaro, A., Serio, C., Castiglione, S., Di Febbraro, M., Rook, L., et al. (2019). Macroevolutionary trends of brain mass in primates. Biol. J. Linn. Soc. 129, 14–25. doi: 10.1093/biolinnean/blz161
Melchionna, M., Profico, A., Serio, C., and Raia, P. (2020). From Smart Apes To Human Brain Boxes. A Uniquely Derived Brain Shape In Late Hominins Clade: Supplementary Material. London: Zenodo.
Meloro, C., Cáceres, N. C., Carotenuto, F., Sponchiado, J., Melo, G. L., Passaro, F., et al. (2015). Chewing on the trees: constraints and adaptation in the evolution of the primate mandible. Evolution 69, 1690–1700. doi: 10.1111/evo.12694
Morino, L., Uchikoshi, M., Bercovitch, F., Hopkins, W. D., and Matsuzawa, T. (2017). Tube task hand preference in captive hylobatids. Primates 58, 403–412. doi: 10.1007/s10329-017-0605-z
Mosquera, M., Llorente, M., Riba, D., Estebaranz, F., González-Brao, M., Lorenzo, C., et al. (2007). Ethological study of manual laterality in naturalistic housed chimpanzees (Pan troglodytes) from the mona foundation sanctuary (Girona, Spain). Laterality 12, 19–30. doi: 10.1080/13576500600886754
Mounier, A., Balzeau, A., Caparros, M., and Grimaud-Hervé, D. (2016). Brain, calvarium, cladistics: a new approach to an old question, who are modern humans and Neandertals? J. Hum. Evol. 92, 22–36. doi: 10.1016/j.jhevol.2015.12.006
Neubauer, S., Gunz, P., Scott, N. A., Hublin, J.-J., and Mitteroecker, P. (2020). Evolution of brain lateralization: a shared hominid pattern of endocranial asymmetry is much more variable in humans than in great apes. Sci. Adv. 6:eaax9935. doi: 10.1126/sciadv.aax9935
Neubauer, S., Hublin, J.-J., and Gunz, P. (2018). The evolution of modern human brain shape. Sci. Adv. 4:eaao5961. doi: 10.1126/sciadv.aao5961
Poza-Rey, E. M., Lozano, M., and Arsuaga, J.-L. (2017). Brain asymmetries and handedness in the specimens from the sima de los huesos site (Atapuerca, Spain). Quat. Int. 433, 32–44. doi: 10.1016/j.quaint.2015.10.004
Prieur, J., Lemasson, A., Barbu, S., and Blois-Heulin, C. (2019). History, development and current advances concerning the evolutionary roots of human right-handedness and language: brain lateralisation and manual laterality in non-human primates. Ethology 125, 1–28. doi: 10.1111/eth.12827
Prieur, J., Pika, S., Barbu, S., and Blois-Heulin, C. (2016). Gorillas are right-handed for their most frequent intraspecific gestures. Anim. Behav. 118, 165–170. doi: 10.1016/j.anbehav.2016.06.008
Profico, A., Buzi, C., Melchionna, M., Veneziano, A., and Raia, P. (2020). Endomaker, a new algorithm for fully automatic extraction of cranial endocasts and the calculation of their volumes. Am. J. Phys. Anthropol. 172:43. doi: 10.1002/ajpa.24043
Profico, A., Piras, P., Buzi, C., Di Vincenzo, F., Lattarini, F., Melchionna, M., et al. (2017). The evolution of cranial base and face in cercopithecoidea and hominoidea: modularity and morphological integration. Am. J. Primatol. 79:e22721. doi: 10.1002/ajp.22721
Profico, A., Schlager, S., Valoriani, V., Buzi, C., Melchionna, M., Veneziano, A., et al. (2018). Reproducing the internal and external anatomy of fossil bones: two new automatic digital tools. Am. J. Phys. Anthropol. 16:499. doi: 10.1002/ajpa.23493
Pujol, J., Deus, J., Losilla, J. M., and Capdevila, A. (1999). Cerebral lateralization of language in normal left-handed people studied by functional MRI. Neurology 52, 1038–1038. doi: 10.1212/WNL.52.5.1038
Puttick, M. N. (2016). Partially incorrect fossil data augment analyses of discrete trait evolution in living species. Biol. Lett. 12:20160392. doi: 10.1098/rsbl.2016.0392
Raia, P., Castiglione, S., Serio, C., Mondanaro, A., Melchionna, M., Di Febbraro, M., et al. (2020). RRphylo: Phylogenetic Ridge Regression Methods for Comparative Studies. R Package Version 2.3.0.
Rasmussen, T., and Milner, B. (1977). The role of early left-brain injury in determining lateralization of cerebral speech functions. Ann. N. Y. Acad. Sci. 299, 355–369. doi: 10.1111/j.1749-6632.1977.tb41921.x
Rightmire, G. P. (2004). Brain size and encephalization in early to mid-pleistocenehomo. Am. J. Phys. Anthropol. 124, 109–123. doi: 10.1002/ajpa.10346
Rilling, J. K. (2006). Human and nonhuman primate brains: are they allometrically scaled versions of the same design? Evol. Anthropol. 15, 65–77. doi: 10.1002/evan.20095
Rocatti, G., and Perez, S. I. (2019). The evolutionary radiation of hominids: a phylogenetic comparative study. Sci. Rep. 9:15267. doi: 10.1038/s41598-019-51685-w
Ruff, C. B., Trinkaus, E., and Holliday, T. W. (1997). Body mass and encephalization in pleistocene homo. Nature 387:173. doi: 10.1038/387173a0
Sansalone, G., Allen, K., Ledogar, J. A., Ledogar, S., Mitchell, D. R., Profico, A., et al. (2020). Variation in the strength of allometry drives rates of evolution in primate brain shape. Proc. R. Soc. B 20200807. doi: 10.1098/rspb.2020.0807
Schlager, S. (2017). “Morpho and Rvcg - shape analysis in R,” in Statistical Shape and Deformation Analysis, eds G. Zheng, S. Li, and G. Szekely (Cambridge, MA: Academic Press), 217–256. doi: 10.1016/b978-0-12-810493-4.00011-0
Schnitzler, J., Theis, C., Polly, P. D., and Eronen, J. T. (2017). Fossils matter - understanding modes and rates of trait evolution in musteloidea (Carnivora). Evol. Ecol. Res. 18, 187–200.
Serio, C., Castiglione, S., Tesone, G., Piccolo, M., Melchionna, M., Mondanaro, A., et al. (2019). macroevolution of toothed whales exceptional relative brain size. Evol. Biol. 46, 332–342. doi: 10.1007/s11692-019-09485-7
Slater, G. J., Harmon, L. J., and Alfaro, M. E. (2012). Integrating fossils with molecular phylogenies improves inference of trait evolution. Evolution 66, 3931–3944. doi: 10.1111/j.1558-5646.2012.01723.x
Sommer, I. E. C., Ramsey, N. F., and Kahn, R. S. (2001). Language lateralization in schizophrenia, an fMRI study. Schizophr. Res. 52, 57–67. doi: 10.1016/S0920-9964(00)00180-8
Stout, D. (2011). Stone toolmaking and the evolution of human culture and cognition. Philos. Trans. R. Soc. B 366, 1050–1059. doi: 10.1098/rstb.2010.0369
Stout, D., and Khreisheh, N. (2015). Skill learning and human brain evolution: an experimental approach. Camb. Archaeol. J. 25, 867–875. doi: 10.1017/S0959774315000359
Szaflarski, J. P., Binder, J. R., Possing, E. T., McKiernan, K. A., Ward, B. D., and Hammeke, T. A. (2002). Language lateralization in left-handed and ambidextrous people: fMRI data. Neurology 59, 238–244. doi: 10.1212/WNL.59.2.238
Tobias, P. V. (1987). The brain of Homo habilis: a new level of organization in cerebral evolution. J. Hum. Evol. 16, 741–761. doi: 10.1016/0047-2484(87)90022-4
Toga, A. W., and Thompson, P. M. (2003). Mapping brain asymmetry. Nat. Rev. Neurosci. 4, 37–48. doi: 10.1038/nrn1009
Uomini, N. T. (2009). The prehistory of handedness: archaeological data and comparative ethology. J. Hum. Evol. 57, 411–419. doi: 10.1016/j.jhevol.2009.02.012
Uomini, N. T. (2015). “Paleoneurology and behaviour,” in Human Paleoneurology, ed. E. Bruner (Cham: Springer), 121–143. doi: 10.1007/978-3-319-08500-5_6
Uomini, N. T., and Meyer, G. F. (2013). Shared brain lateralization patterns in language and acheulean stone tool production: a functional transcranial doppler ultrasound study. PLoS One 8:e72693. doi: 10.1371/journal.pone.0072693
Walker, S. F. (1980). Lateralization of functions in the vertebrate brain: a review. Br. J. Psychol. 71, 329–367. doi: 10.1111/j.2044-8295.1980.tb01750.x
Weaver, T. D., and Gunz, P. (2018). Using geometric morphometric visualizations of directional selection gradients to investigate morphological differentiation. Evolution 72, 838–850. doi: 10.1111/evo.13460
Weber, G. W. (2014). Virtual anthropology. Am. J. Phys. Anthropol. 156, 22–42. doi: 10.1002/ajpa.22658
White, R., Bosinski, G., Bourrillon, R., Clottes, J., Conkey, M. W., Rodriguez, S. C., et al. (2019). Still no archaeological evidence that Neanderthals created Iberian cave art. J. Hum. Evol. 17:102640. doi: 10.1016/j.jhevol.2019.102640
Wu, X., Schepartz, L. A., Falk, D., and Liu, W. (2006). Endocranial cast of Hexian Homo erectus from South China. Am. J. Phys. Anthropol. 130, 445–454. doi: 10.1002/ajpa.20378
Xiang, L., Crow, T., and Roberts, N. (2019). Cerebral torque is human specific and unrelated to brain size. Brain Struct. Funct. 224, 1141–1150. doi: 10.1007/s00429-018-01818-0
Yakovlev, P. I., and Rakic, P. (1966). Patterns of decussation of bulbar pyramids and distribution of pyramidal tracts on two sides of the spinal cord. Trans. Am. Neurol. Assoc. 91, 366–367.
Zilhão, J., Angelucci, D. E., Badal-García, E., d’Errico, F., Daniel, F., Dayet, L., et al. (2010). Symbolic use of marine shells and mineral pigments by Iberian Neandertals. Proc. Natl. Acad. Sci. U.S.A. 107, 1023–1028. doi: 10.1073/pnas.0914088107
Keywords: endocast, brain volume, asymmetry, primates, human evolution, Homo neanderthalensis, Homo heidelbergensis
Citation: Melchionna M, Profico A, Castiglione S, Sansalone G, Serio C, Mondanaro A, Di Febbraro M, Rook L, Pandolfi L, Di Vincenzo F, Manzi G and Raia P (2020) From Smart Apes to Human Brain Boxes. A Uniquely Derived Brain Shape in Late Hominins Clade. Front. Earth Sci. 8:273. doi: 10.3389/feart.2020.00273
Received: 20 December 2019; Accepted: 16 June 2020;
Published: 14 July 2020.
Edited by:
Mary Teresa Silcox, University of Toronto Scarborough, CanadaReviewed by:
Antoine Balzeau, Centre National de la Recherche Scientifique (CNRS), FranceEmmanuel Paul Gilissen, Royal Museum for Central Africa, Belgium
Copyright © 2020 Melchionna, Profico, Castiglione, Sansalone, Serio, Mondanaro, Di Febbraro, Rook, Pandolfi, Di Vincenzo, Manzi and Raia. This is an open-access article distributed under the terms of the Creative Commons Attribution License (CC BY). The use, distribution or reproduction in other forums is permitted, provided the original author(s) and the copyright owner(s) are credited and that the original publication in this journal is cited, in accordance with accepted academic practice. No use, distribution or reproduction is permitted which does not comply with these terms.
*Correspondence: Antonio Profico, YW50b25pby5wcm9maWNvQHlvcmsuYWMudWs=