- 1Jiangxi Key Laboratory for Mass Spectrometry and Instrumentation, East China University of Technology, Nanchang, China
- 2International Center of Future Science, and Dinosaur Evolution Research Center, Jilin University, Changchun, China
- 3Department of Life Sciences, National Chung Hsing University, Taichung, Taiwan
- 4Faculty of Land Resource Engineering, Kunming University of Science and Technology, Kunming, China
- 5ELKH, Research Centre for Natural Sciences, Budapest, Hungary
- 6Department of Biology, University of Toronto Mississauga, Mississauga, ON, Canada
There is broad consensus that many bones of extinct vertebrates contain Rare Earth Elements (REE) and other trace minerals that have been incorporated and enriched into the fossil during diagenesis. During fossilization, apatite minerals in vertebrate bones recrystallize from metastable biogenic forms to thermodynamically more stable inorganic apatite minerals while incorporating REEs from their environment. More than ∼95% of the REE in fossil bones are diagenetically incorporated post-mortem, and bones in different environments have different and distinct REE signatures, generally viewed to be controlled by sedimentological and taphonomic processes. The REE pattern in fossils is generally stable, and the normalized concentration patterns provide unique “fingerprints” or signatures that have been used for various geological and paleontological investigations. The discovery of embryos and their adults at the same site, a previously unrecorded occurrence in the fossil record, allowed us to compare for the first time the relationship between REE concentrations in the same taxon at widely differing ontogenetic stages. Here we compare REE patterns in bones from two distinct sites in Yunnan, China, both preserving embryonic and adult bones of Early Jurassic Sauropodomorpha dinosaurs. These dinosaurs are closely related to each other and close in geological age, and their bones show very similar REE patterns. However, the embryonic bones have significantly lower levels of total REEs when compared to their adult counterparts. We attribute the 10-fold La_REE difference and U concentration 5/1 ratio to the level of ossification of the bones and the dramatically lower concentration of apatites in the embryonic than in the adult bones. We conclude that the ontogenetic stage of fossil materials can have a significant impact on REE concentrations, and discuss the impact of these results on future work.
Introduction
Rare Earth Element (REE) concentration in fossilized bone is generally considered to be a post-mortem phenomenon (e.g., Bertram et al., 1992; Trueman and Benton, 1997; Williams, 1998; Trueman, 1999, 2013; Patrick et al., 2002; Trueman et al., 2003; Herwartz et al., 2013; McCormack et al., 2015). The total REE concentrations (ΣREE, in ppm) in extant bones are usually very low, while in fossilized bones, the REE can range from less than 1,000 ppm up to more than 20,000 ppm (Reynard et al., 1999; Lećuyer et al., 2003), often much higher than in sediments in mining operations, as indicated by the People’s Republic of China National Geology and Mining Standard, DZ/T 0204–2002. This high concentration of REE in fossil bone has been used in various geochemical studies for resolving problems in paleontology, including determinations of depositional environments, and testing whether fossil assemblages or parts of assemblages are autochthonous or allochthonous (Reynard and Balter, 2014; Hassler et al., 2018). Adsorption and substitution are dominant processes in the REE concentration in biogenic apatites (Reynard et al., 1999). The partitioning associated with adsorption has been studied experimentally between phosphates (PO43–) and water and iron-manganese hydroxides and water. Adsorption is easily accomplished to reach equilibrium but is also easily reversible. Thus, the weakly physical molecular van der Waals forces of these REE3+ are easily desorbed. In contrast, the mechanism of chemical substitution is controlled by bulk crystal-chemical properties, in which certain chemical components were replaced. During substitution, cations Ca2+, Ba2+ in the apatites are replaced by REE3+ and apatite is recrystallized by strong chemical bonds in a stable pattern, while the anions PO3–4, CO2–3, F–, OH–, and Cl– were replaced by other anions in stoichiometric and or non-stoichiometric fashion. Thus, the substitution mechanism plays a dominant role in the process that results in REEs being captured from groundwater and concentrated in fossil bones. The same mechanism also explains the concentration of Uranium in adult and embryonic bones.
Early Jurassic exposures (Figure 1) in Yunnan Province have yielded the remains of both embryos and adults of the same taxon (Reisz et al., 2013), Lufengosaurus (Figure 2), at the same site. These are very rare occurrences in the fossil record of terrestrial vertebrates, with a combination of similar taxa of similar age, both represented by the adult and embryonic bones. The discovery of these two Early Jurassic sites, with both fossilized adult and embryonic bone pairs are the crucial foundational source for this study and form the basis of the important discoveries discussed below, and the discovery of these two localities has given us the unprecedented opportunity, likely the first such case, to study REE patterns in fossils under these kinds of controlled conditions. Of course, it is possible that both dinosaur adult and embryonic bones from the very same site were found in the past somewhere else, but none of them were subjected to the REE comparison as this study. This excluded potential REE variability associated with fossils coming from different taxa and reduced as much as possible effects of preservation in different formations and different depositional environments. The embryonic and adult bones from Dawa, Lufeng, Yunnan belong to the early Sauropodomorpha Lufengosaurus (Fang et al., 2000). Similarly, embryonic and adult bones from Jiaojiadian, Yimen, Yunnan have been found and assigned to the larger early Sauropodomorpha Yimenosaurus (Bai et al., 1990). Phylogenetic analyses have placed both taxa within the broader Sauropodomorpha clade but well outside Sauropoda. These materials were collected from the middle (Dawa) and lower (Jiaojiadian) parts of Dark Red Beds Zhangjia’ao Member of the Early Jurassic (Sinemurian, 190–197 Ma), Lower Lufeng Formation, roughly 3–5 m below the top of the formation. Thus, we used two relatively closely related Sauropodomorpha dinosaurs from two different localities within the early Jurassic of China, extremes in ontogeny comparing embryonic bones with those from adults to test the relationship between levels of ossification and REE concentration. There is no perceived difference between the lithologies of these two localities.
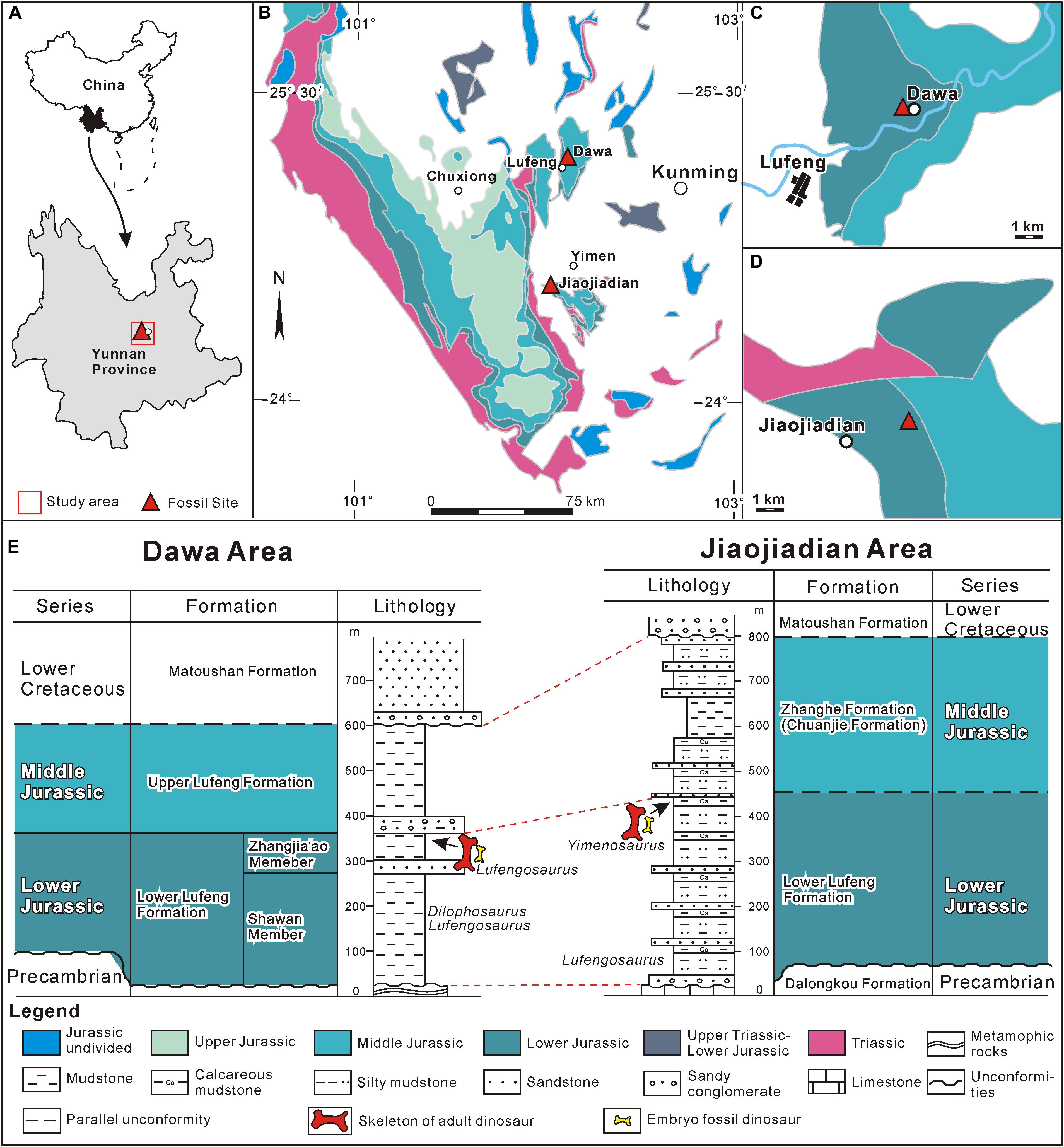
Figure 1. Location and stratigraphy of Dawa Lufengosaurus and Jiaojiadian Yimenosaurus fossil sites. (A) Geographic location of the study area. (B) Distribution of the Jurassic strata in central Yunnan Province and the location of the two dinosaur sites (70 Km apart). (C) Sketched geologic map of Dawa area. (D) sketched geologic map of Jiaojiadian area. (E) Stratigraphic columns of Dawa and Jiaojiadian fossil sites and their correlation.
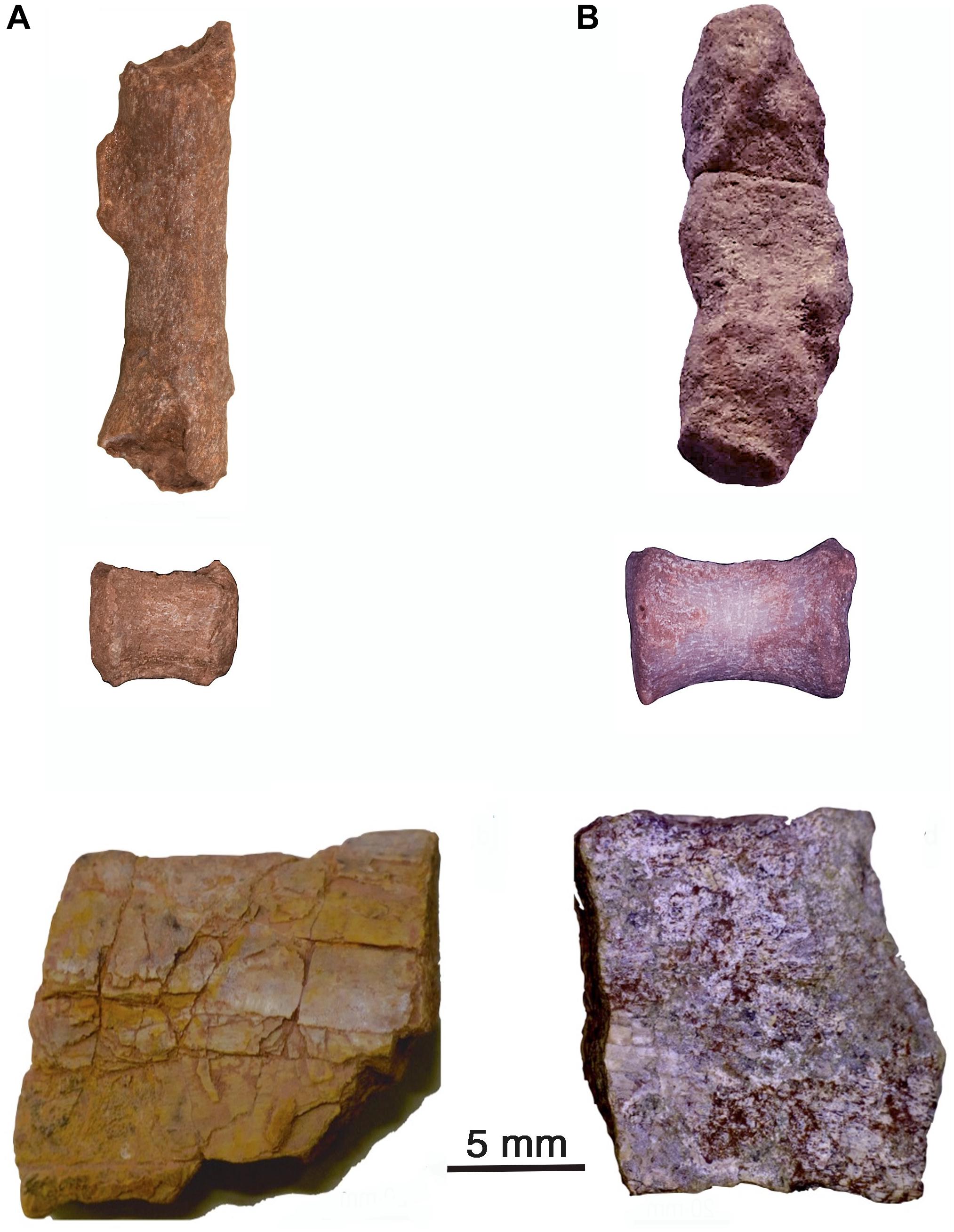
Figure 2. Fossil materials that were available for this study. (A) Embryonic femur and a caudal centrum (not used for chemical analyses), and adult femur fragment of Lufengosaurus. (B) Embryonic limb bone inside a concretion and a dorsal centrum (not used for chemical analyses), and adult femur fragment of Yimenosaurus.
It is often taken for granted that both fossilized and extant bone mineral is identified as calcium hydroxyapatite (HA, HO-Apatite), Ca10(PO4)6(OH)2 (de Jong, 1926), a single chemical compound. However, the hydroxyapatite crystal lattice (hexagonal space group P63/m) can accommodate various other ions, and carbonate ions CO32– can readily replace the hydroxyl ions OH– or orthophosphate ions PO43–, leading to carbonated apatite type A and type B, respectively, as confirmed by SR-FTIR (Reisz et al., 2013; Lee et al., 2017) and neutron scattering. In this respect, carbonatoapatite-B is closest to the actual bone mineral (Loong et al., 2000). During fossilization, these thermodynamically unstable, reactive, poorly crystalline bioapatites recrystallize to form more stable, less reactive states, in which trace elements in the pore-water were incorporated into the crystal lattice of the apatites in the fossil, and REE are trapped and concentrated (e.g., Trueman, 2013; Hassler et al., 2018). Several previous studies suggest that the REE composition of fossils reflects the environment of early diagenesis in both terrestrial and marine settings (e.g., Henderson et al., 1983; Staron et al., 2001; Metzger et al., 2004; Patrick et al., 2004; Martin et al., 2005; Kaflak et al., 2006).
Materials and Methods
Multiple adults and embryonic bones of the dinosaur Lufengosaurus (Lower Jurassic strata near Dawa, Lufeng County, Chuxiong Prefectural Museum, catalog no. C2019 2A233) and Yimenosaurus (Lower Jurassic strata near Jiaojiadian, Yimen County, Jilin University Museum, catalog no. JLUM TY-501 and JLUM TY-502) were collected and analyzed by coupled plasma optical emission spectrometer (ICP-OES) and inductively coupled plasma mass spectrometry (ICP-MS) (Rogers et al., 2010; Fassett et al., 2012). We used the same type of bone in all cases, focusing on the femur of embryos and femur fragments in the adults. Adult and embryonic bone cortex were dissolved and used in ICP-MS analyses in order to get a good average REE as well as P concentration for each bone. Since fossil bone can be very heterogeneous in nature, REE concentration could vary significantly in various parts of the individual fossil bone. It is, therefore, preferable to get an average measure of a particular region of the bone in both the adult and embryo, as done here, rather than through spot measurements, as used in LA-ICP-MS analysis, even if statistically sound numbers of spots were tested (Herwartz et al., 2011, 2013).
Lufengosaurus embryonic and adult material were collected from the same site near the village of Dawa, Lufeng County, in 2010. Yimenosaurus embryonic and adult bones were collected in January of 2018, from near the Jianjiadian village, including a big femur fragment together with fragmentary embryonic bones. We are confident of the fetal status of the embryonic bones, based on their level of ossification and ontogenetic stage, rather than being perinatal or neonatal (Reisz et al., 2012, 2013). The materials used for this study were within the mid-range of the embryonic ontogenetic series. Secondly, the embryonic bone of Yimenosaurus that we identified and used in this study was also at roughly the same ontogenetic stage as the embryonic bones of Lufengosaurus that we used, all consistent with embryonic stages of these Sauropodomorpha dinosaurs and those of the in-ovo embryonic materials of a similar Sauropodomorpha the South African Massospondylus (Reisz et al., 2012).
Both adult and embryonic bone pairs were collected from the same site, respectively, avoiding stratigraphic and taphonomic variations. In the case of the Lufengosaurus embryonic material, the adult bone was located in the same site, a few feet from the accumulation as the embronic bones. In the case of the Yimen locality, the embryonic bones attributed to Yimenosaurus were found in the discarded sediments at the excavation site of the adult skeletal materials.
Coupled plasma optical emission spectrometer and ICP-MS analyses of the Dawa Lufengosaurus bone cortex were done at the National Kaohsiung University of Science and Technology, Kaohsiung, Taiwan, per NIEA M104.01C(2003) on Perkin-Elmer Optima 2100DV (Huang et al., 2009). Similar tests were also carried out at Academia Sinica, Taipei, Taiwan (ICP-MS). Additional ICP-MS (by using Agilent 7500ce) was carried out at National Tsing Hua University, Taiwan, arranged through the National Chung Hsing University. The Yimen bone material was analyzed at the East China University of Technology, China. The Yimen dinosaur embryonic and adult bones, the cortical region, and without the medullary cavity material were ground to powder separately. Yimen dinosaur bone powders were digested following the procedure of the People’s Republic of China National Standard GB/T 14506.19. Then the concentration of P, Ca, and Ti was measured by ICP-OES (PerkinElmer Optima 5300 DV), and the concentration of REE was measured by ICP-MS (Perkin Elmer ICP-MS element 2). The ICP-OES/MS provides both REE and P concentrations. Adult and embryonic bones were also analyzed by ICP-MS and solid-state NMR at the Hungarian Academy of Sciences, Hungary. SR-FTIR images were acquired (Lee et al., 2017) using SR-IMS at BL14A1 of NSRRC, Taiwan. The SR-FTIR spectra were collected in the mid-infrared range of 4000–650 cm–1. All the chemical analyses follow the strict analytical lab procedures of repetitive runs for valid resulting data. The Chinese Background Average (CBA) REE values were used in this study for normalization (Wang and Wei, 2011) rather than the NASC (Gromet et al., 1984) or PAAS (Nance and Taylor, 1976), since we are working on the Chinese fossils, and it is preferable to use the available local soil REE background values.
In a separate set of ICP-MS analyses, various growth stage adult and embryonic bones of Lufengosaurus were also tested for the relationship between total REE and phosphorus concentration (Supplementary Table S3) inside the fossil bones.
Results
The analyses that produced the average spidergrams of total La-REE of the two pairs of adult/embryonic bones from Dawa and Yimen were completed in several labs, as described in the section “Materials and Methods.” Figure 3 shows the averaged CBA-normalized La_REE concentrations (logarithmic Y-axis), La_REE (X-axis ordered relative to atomic number) (Raw and normalized data in Supplementary Tables S1a,b, S2).
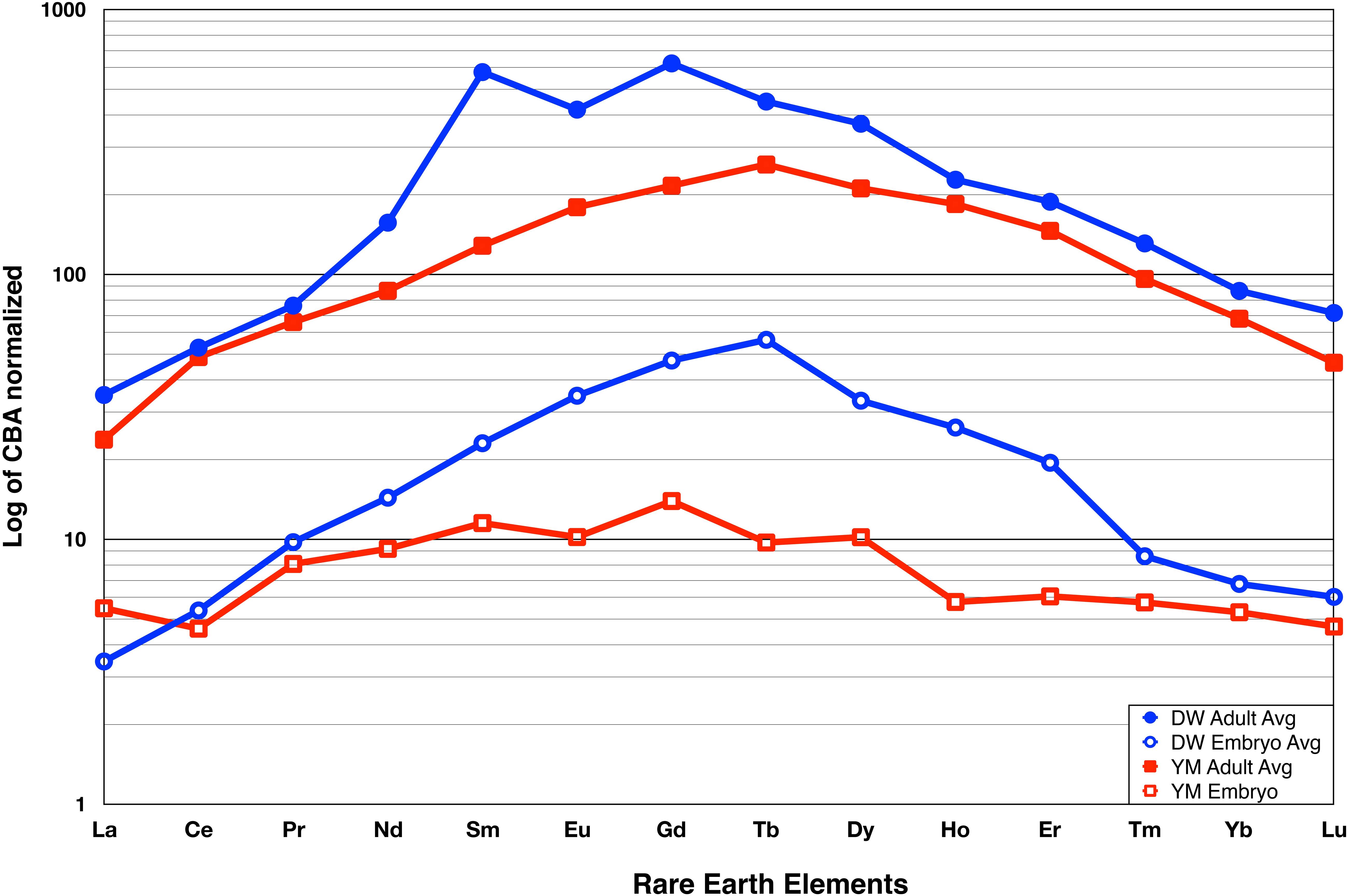
Figure 3. Lanthanide Rare Earth Element (La_REE) Spidergrams of Dawa (Lufengosaurus) and Jiaojiadian (Yimenosaurus) adult and embryo femur bones. Dawa average data are shown in blue while the Jiaojiadian average data are shown in red. The La_REE concentration data is presented at ppm normalized to CBA (y-axis) relative to the atomic number labeled as elemental symbol (x-axis). Both dinosaur adult and embryo pairs show that the total La_REE of the adult bone is an order of magnitude higher than that of the embryo. Complete individual spidergram can be found in Supplementary Figure S3.
The La_REEs are classified by atomic numbers; low atomic numbers are light rare-earth elements (LREE), high atomic numbers are the heavy rare-earth elements (HREE), and those in-between are as the middle rare-earth elements (MREE). These bell-shaped curves show more enrichment of MREE than LREE and HREE (Figure 3), which can be assumed to represent the result of extensive recrystallization of the apatites in the presence of REE-bearing fluids.
It is important to note that both adult and embryonic bones are from the exact same sites of Lower Lufeng Formation with no stratigraphic difference. The two sites are apart only 70 km from each other (Bai et al., 1990; Fang et al., 2000). It is therefore not surprising that the spidergrams (Figure 3) show strong similarity to each other, with both adult spidergram curves (top pair) having similar shapes that are closely matched; similarly, the spidergrams of the embryonic bones (bottom pair) are also similar to each other. This finding is consistent with current knowledge of the two localities, their similar depositional environment, and their stratigraphic proximity. The similarity in the shape of the curves, their almost identical “bell shape” suggests that the diagenetic processes that produced these fossils were similar, and it can also be inferred to have occurred at relatively low temperatures under crystal-chemical control involving a substitution mechanism. This process caused extensive recrystallization of the apatites in the presence of REE-bearing fluids. The most striking feature of the spidergrams is the vast separation between the adult and embryonic bones at each locality; they are slightly more than one order of magnitude apart. This separation indicates that the total La_REE concentration of the adult bones is about ten times greater than, and U about five times than those in the embryonic bones. We were careful to avoid the central medullary cavity of the femur, where apatites are usually absent. Diagenetic processes normally result in the deposition of various chemicals other than REEs in the medullary cavity, affecting the overall REEs results, apatites are normally present in the circumferential bone-building portion of the bone, in the cortex. Therefore, the 10-fold difference in REE total concentration and five times of U is a reflection of the amount of apatites that is present in these comparable regions of the embryonic and adult bone (Herwartz et al., 2011, 2013).
Embryonic bones are known to have much lower levels of ossification than the adult bones of the same species (Reisz et al., 2005). This difference is particularly striking in the case of Sauropodomorpha dinosaurs, in which the embryonic bones are characterized by very rapid growth (Reisz et al., 2013), and by the presence of Primary Vascular Cavities (PVC) that occupy more than 50% of the cortex of the femur. In contrast, the adult bones of these two Sauropodomorphas have a very small percentage of Vascular Canals (VC), usually located at the center of each osteon. Overall, the level of ossification corresponds to the amount of apatites that the developing organism produces, with the rapidly developing embryos of Sauropodomorphas having much less apatites in the femur. It is, therefore, possible to correlate the amount of apatites present in the bone with the level of REE concentration that has occurred during diagenesis, with the embryonic bone having a significantly smaller concentration of apatites and hence REE than their adult counterparts.
In order to test the apatites/La_REEs relationship further, we undertook a separate set of ICP-MS analyses, with bones of Lufengosaurus being tested for phosphorus concentration (Supplementary Table S3) vs. total La_REE. This P element is a crucial component of apatites, and the amount of P is indicative of the apatites concentration in the bone. The results (Figure 4) show a strong correlation between the total La_REE and P inside the dinosaur bones at different developmental (ontogenetic) stages, (y = 0.0498x + 181.79, R2 = 0.9338). The higher the P concentration, the more La_REE having been incorporated inside the fossil bones. This linear correlation provides additional evidence of the close relationship between Pconc and the total amount of La_REE that has been incorporated into the bone.
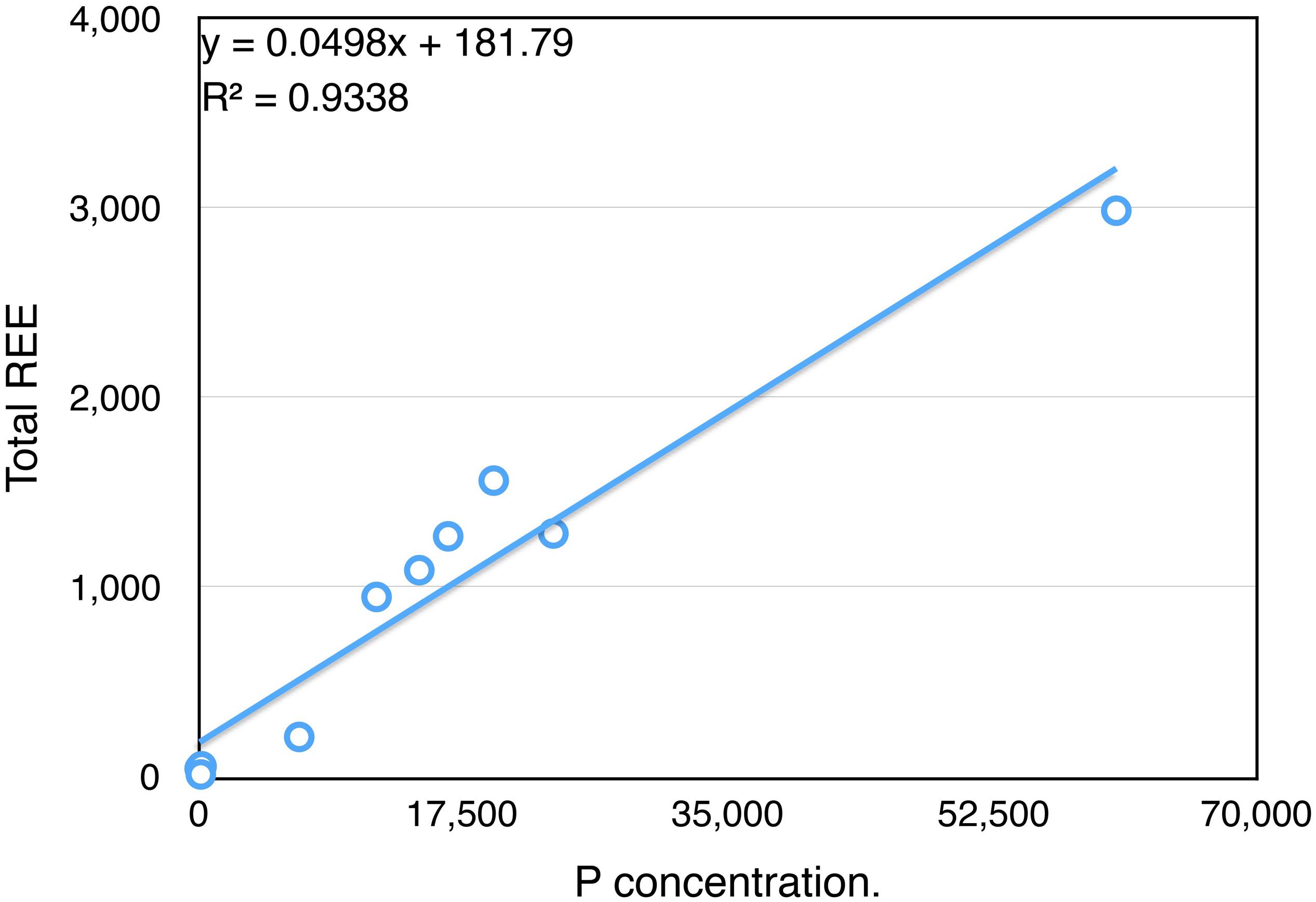
Figure 4. Excellent Correlation between P (phosphorus) and ΣLa_REE in Lufengosaurus skeletal elements. P and ΣLa_REE values inside the bones of the Early Jurassic Lufengosaurus measured at different ontogenetic stages show strong correlation (0.9338) regardless of the stage of growth. Complete data can be found in Supplementary Table S3.
Discussion
The results show that there are significant differences in the ΣLa_REE concentration (10/1 ratio) between the adults and embryos as well as in the Ac_REE U concentration (5/1 ratio). This difference is well correlated with the known differences between embryonic and adult bones. For example, previous studies have allowed for quantification of levels of PVC being present in the embryonic femora of Lufengosaurus, showing 55% PVC in the embryonic bone cortex, in sharp contrast to that presence of 5% or less VC in the adult bone (Reisz et al., 2013). Overall, there is a significant difference between the amount of ossification present in the form of apatites between the embryo and the adult. During ontogeny, the level of vascularity decreases, and the total amount of bone and apatite increases, which is the main ingredient to catch REE, reaching its maximum at the full adult condition. Embryonic and adult bones attributed to Yimenosaurus reveal similar patterns, as would be expected for two closely related taxa with similar developmental trajectories. The slight differences between the patterns and levels of La_REE concentration in the two adult bones, and between the two embryonic bones can be attributed to the geographic separation and slight age differences between the two very similar sedimentary sites, i.e., local variation.
Our results also indicate that there is a strong correlation between the ΣLa_REE and P concentrations in the bones, which confirms the close relationship between the ΣLa_REE and the amount of apatites present in the bone. Future studies may use ΣREE concentration as a proxy for P inside fossilized dinosaur bones and hence apatites concentration. Our results also point out two critical aspects of REE preservation in fossil bones. (1) The overall content of REE that has been incorporated into the bone depended on the amount of apatites present in the bone. Ontogenetically different stages of fossils will preserve different ΣREE levels, making it important to know the ontogenetic stage of the fossil being analyzed. (2) For stratigraphic confirmations, it is best to have the analyzed specimens at about the same ontogenetic stage, and the mixed-use of adult bones from one depositional environment/formation and juvenile bones from the others should be avoided. The fact that the ΣREE ratio between the adult and embryo from the same site has an order of magnitude difference underscores the likelihood that fossil materials of different ontogenetic stages may lead to results that would be difficult to interpret correctly. Given the result of this study, future work should include such as intermediate stages of ontogeny, permitting quantification of the amount of apatites being present in particular bones, and hence a more precise calculation of the level of ossification relative to the size that a particular animal achieved at the time of death. Finally, the REE values may be used to quantify the material strength of bones in extinct organisms, allowing for a dramatic increase in our ability to predict locomotory capabilities and behavior in animals that roamed the earth but have no living equivalents.
Data Availability Statement
All datasets generated for this study are included in the article/Supplementary Material.
Author Contributions
All authors contributed to the research. TH collected the specimens of the Dawa and Yimen dinosaur adult and embryo materials, initiated and conducted the research. SW, RY, and LK did the analyses and participated in preparation of the manuscript. HC guided the experiments. SZ and XL contributed to the geology. RR guided the project and wrote the manuscript together with TH.
Funding
The research support fund from Jilin University to RR and TH. East China University of Technology Support fund for SW, RY, and HC.
Conflict of Interest
The authors declare that the research was conducted in the absence of any commercial or financial relationships that could be construed as a potential conflict of interest.
Acknowledgments
We thank L. Y. Jin and J. Chen of the Dinosaur Evolution Research Center of the Jilin University Museum for the logistic supports. We also thank the researchers, C. C. Chiang, Y. C. Lee, and technicians at NSRRC, Academia Sinica, NCHU, NTHU, and NKUST, C. L. Huang and C. T. Lin, for their generous help in analyzing the materials discussed in this manuscript. Field works were helped by C. W. Yang, S. M. Zhong, and graduate students of S. T. Zhang.
Supplementary Material
The Supplementary Material for this article can be found online at: https://www.frontiersin.org/articles/10.3389/feart.2020.00230/full#supplementary-material
References
Bai, Z., Yang, J., and Wang, G. (1990). A new genus of prosauropod from the Yuxi (region) of Yunnan Province [Yunnan Yuxi yuanxijiao lei yi xin shu]: Yuxi Cultural Organization. Yuxi Wen Tuan 1, 14–23.
Bertram, C. J., Elderfield, H., Aldridge, R. J., and Morris, S. C. (1992). 87Sr/86Sr, 143Nd/144Nd and REEs in Silurian phosphatic fossils. Earth Planet. Sci. Lett. 113, 239–249. doi: 10.1016/0012-821x(92)90222-h
de Jong, W. F. (1926). La substance minérale dans les os. Recueil Travaux Chimiques Pays-Bas. 45, 445–449.
Fang, X. X., Long, Q. Q., Lu, L. W., Zhang, Z. X., Pan, S. G., Wang, Y. M., et al. (2000). “Lower, middle, and upper jurassic subdivision in the Lufeng region, Yunnan Province,” in Proceedings of the Third National Stratigraphical Congress of China, Beijing, 208–214.
Fassett, J. E., Heaman, L. M., and Simonetti, A. (2012). Direct U-Pb dating of Cretaceous and Paleocene dinosaur bones, San Juan Basin, New Mexico. Geology 40, 263–264. doi: 10.1130/G32758Y.12012
Gromet, L. P., Dymek, R. F., Haskin, L. A., and Korotev, R. L. (1984). The “North American shale composite”: its compilation, major and trace element characteristics. Geochim. Comochim. Acta 48, 2469–2482. doi: 10.1016/0016-7037(84)90298-9
Hassler, A., Martin, J. E., Amiot, R., Tacail, T., Godet, A. F., Allain, R., et al. (2018). Calcium isotopes offer clues on resource partitioning among Cretaceous predatory dinosaurs. Proc. R. Soc. Lond. Ser. B 285:20180197. doi: 10.1098/rspb.2018.0197
Henderson, P., Marlow, C. A., Molleson, T. I., and Williams, C. T. (1983). Patterns of chemical change during bone fossilization. Nature 306, 358–360. doi: 10.1038/306358a0
Herwartz, D., Tutken, T., Jochum, K. P., and Sander, P. M. (2013). Rare earth element systematics of fossil bone revealed by LA-ICPMS analysis. Geochim. Cosmochim. Acta 103, 161–183. doi: 10.1016/j.gca.2010.09.036
Herwartz, D., Tütken, T., Münker, C., Jochum, K. P., Stoll, B., and Sander, P. M. (2011). Timescales and mechanisms of REE and Hf uptake in fossil bones. Geochim. Cosmochim. Acta 75, 82–105.
Huang, T. D., Huang, C. L., Lin, C. T., Knell, M., and Yang, W. J. (2009). Exploring Dinosaur Strata of Yunnan via Rare Earth Element Analyses. Western Pacific Earth Sci. 9, 15–36.
Kaflak, A., Chmielewski, D., Górecki, A., Slosarczyk, A., and Kolodziejski, W. (2006). Efficiency of 1H-31P NMR cross-polarization in bone apatite and its mineral standards. Solid State Nuclear Magn. Reson. 29, 345–348. doi: 10.1016/j.ssnmr.2005.11.005
Lećuyer, C., Bogey, C., Garcia, J. P., Grandjean, P., Barrat, J. A., Floquet, M., et al. (2003). Stable isotope composition and rare earth element content of vertebrate remains from the Late Cretaceous of northern Spain (Lan<o): did the environmental record survive? Palaeogeogr. Palaeoclimatol. Palaeoecol. 193, 457–472. doi: 10.1016/S0031-0182(03)00261-X
Lee, Y. C., Chiang, C. C., Huang, P. Y., Chung, C. Y., Huang, T. D., Wang, C. C., et al. (2017). Evidence of preserved collagen in an Early Jurassic sauropodomorph dinosaur revealed by synchrotron FTIR microspectroscopy. Nat. Commun. 8:14220. doi: 10.1038/ncomms14220
Loong, C. K., Rey, C., Kuhn, L. T., Combes, C., Wu, Y., Chen, S. H., et al. (2000). Evidence of hydroxyl-ion deficiency in bone apatites: an inelastic neutron-scattering study. Bone 26, 599–602. doi: 10.1016/s8756-3282(00)00273-8
Martin, J. E., Patrick, D., Kihm, A. J., Foit, F. F. Jr., and Grandstaff, D. E. (2005). Lithostratigraphy, Tephrochronology, and Rare Earth Element Geochemistry of Fossils at the Classical Pleistocene Fossil Lake Area, South Central Oregon. J. Geol. 113, 139–155. doi: 10.1086/427665
McCormack, M. J., Bahr, A., Gerdes, A., Tütken, T., and Prinz-Grimm, P. (2015). Preservation of successive diagenetic stages in Middle Triassic bonebeds: evidence from in situ trace element and strontium isotope analysis of vertebrate fossils. Chem. Geol. 410, 108–123. doi: 10.1016/j.chemgeo.2015.06.003
Metzger, C. A., Terry, D. O. Jr., and Grandstaff, D. E. (2004). Effect of paleosol formation on rare earth element signatures in fossil bone. Geology 32, 497–500.
Nance, W. B., and Taylor, S. R. (1976). Rare earth element patterns and crustal evolution–I. Australian post-Archean sedimentary rocks. Geochim. Cosmochim. Acta 40, 1539–1551. doi: 10.1016/0016-7037(76)90093-4
Patrick, D., Martin, J. E., Parris, D. C., and Grandstaff, D. E. (2002). Rare Earth Element Signatures of fossil vertebrates compared with lithostratigraphic subdivisions of the Upper Cretaceous Pierre Shale, central South Dakota. Proc. South Dakota Acad. Sci. 81, 161–179.
Patrick, D., Martin, J. E., Parris, D. C., and Grandstaff, D. E. (2004). Paleoenvironmental interpretations of rare earth element signatures in mosasaurs (Reptilia) from the upper Cretaceous Pierre Shale, central South Dakota. USA. Palaeogeogr. Palaeoclimatol. Palaeoecol. 212, 277–294. doi: 10.1016/s0031-0182(04)00315-3
Reisz, R. R., Evans, D. C., Roberts, E. M., Sues, H. D., and Yates, A. M. (2012). Oldest known dinosaurian nesting site and reproductive biology of the Early Jurassic sauropodomorph Massospondylus. Proc. Natl. Acad. Sci. 109, 2428–2433. doi: 10.1073/pnas.1109385109
Reisz, R. R., Huang, T. D., Roberts, E. M., Peng, S. R., Sullivan, C., Stein, K., et al. (2013). Embryology of Early Jurassic dinosaur from China with evidence of preserved organic remains. Nature 496, 210–214. doi: 10.1038/nature11978
Reisz, R. R., Scott, D., Sues, H.-D., Evans, D. C., and Raath, M. A. (2005). Embryos of an Early Jurassic prosauropod dinosaur and their evolutionary significance. Science 309, 761–764. doi: 10.1126/science.1114942
Reynard, B., and Balter, V. (2014). Trace elements and their isotopes in bones and teeth: diet, environments, diagenesis, and dating of archeological and paleontological samples. Palaeogeogr. Palaeoclimatol. Palaeoecol. 416, 4–16. doi: 10.1016/j.palaeo.2014.07.038
Reynard, B., Lećuyer, C., and Grandjean, P. (1999). Crystal-chemical controls on rare-earth element concentrations in fossil biogenic apatites and implications for paleoenvironmental reconstructions. Chem. Geol. 155, 233–241. doi: 10.1016/s0009-2541(98)00169-7
Rogers, R. R., Fricke, H. C., Addona, V., Canavan, R. R., Dwyer, C. N., Harwood, C. L., et al. (2010). Using Laser Ablation-Inductively Coupled Plasma-Mass Spectrometry (LA-ICP-MS) to Explore Geochemical Taphonomy of Vertebrate Fossils in the Upper Cretaceous Two Medicine and Judith River Formations of Montana. Palaios 25, 183–195. doi: 10.2110/palo.2009.p09-084r
Staron, R. M., Granstaff, B. S., Gallagher, W. B., and Grandstaff, D. E. (2001). REE Signatures in Vertebrate Fossils from Sewell, NJ: implications for Location of the K-T Boundary. Palaios 16, 255–265. doi: 10.1669/0883-1351(2001)016<0255:rsivff>2.0.co;2
Trueman, C. N. (1999). Rare earth element geochemistry and taphonomy of terrestrial vertebrate assemblages. PALAIOS 14, 555–568.
Trueman, C. N. (2013). Chemical taphonomy of biomineralized tissues. Palaeontology 2013, 1–12. doi: 10.1111/pala.12041
Trueman, C. N., and Benton, M. J. (1997). A geochemical method to trace the taphonomic history of reworked bones in sedimentary setting. Geology 25, 263–266.
Trueman, C. N., Benton, M. J., and Palmer, M. R. (2003). Geochemical taphonomy of shallow marine vertebrate assemblages. Palaeogeogr. Palaeoclimatol. Palaeoecol. 197, 151–169. doi: 10.1016/s0031-0182(03)00457-7
Wang, Y., and Wei, F. S. (2011). Chemistry of soil environmental elements, page 336, Table 18.9; REE Background basic statistic values, (Layer A) whole country soil∗. Chin. Environ. Publ.
Keywords: ICP-OES, ICP-MS, dinosaur bone structure and composition, rare earth elements in dinosaur bones, SR-FTIR microspectroscopy, paleontology, dinosaur bone growth
Citation: Wang S, Huang TD, Yan R, Chen H, Zhang S, Li X, Kótai L and Reisz RR (2020) Rare Earth Elements in Dinosaur Bones Across the Embryo-Adult Spectrum. Front. Earth Sci. 8:230. doi: 10.3389/feart.2020.00230
Received: 02 January 2020; Accepted: 28 May 2020;
Published: 19 June 2020.
Edited by:
Eric M. Roberts, James Cook University, AustraliaReviewed by:
Terry A. Gates, North Carolina State University, United StatesEspen Knutsen, James Cook University, Australia
Copyright © 2020 Wang, Huang, Yan, Chen, Zhang, Li, Kótai and Reisz. This is an open-access article distributed under the terms of the Creative Commons Attribution License (CC BY). The use, distribution or reproduction in other forums is permitted, provided the original author(s) and the copyright owner(s) are credited and that the original publication in this journal is cited, in accordance with accepted academic practice. No use, distribution or reproduction is permitted which does not comply with these terms.
*Correspondence: Timothy D. Huang, dGltZF9odWFuZ0B5YWhvby5jb20=; Robert R. Reisz, cm9iZXJ0LnJlaXN6QHV0b3JvbnRvLmNh
†These authors share first authorship