- Department of Geosciences, The University of Arizona, Tucson, AZ, United States
Alluvial fans are a significant part of the sediment routing system, forming distinctive steep, fan-shaped deposits of coarse-grained detritus where rivers lose flow velocity after exiting confined mountain drainages. Processes on the fan are influenced by both internal (autogenic) feedback cycles like channel avulsion and by external (allogenic) conditions such as climate and tectonics. These conditions in turn influence the stratigraphic architecture (i.e., the pattern of channel stacking and sizes) within the fan. Studying stratigraphic architecture of alluvial fans can, therefore, provide insight into controls on fan deposition. We employ UAV-based photogrammetric models to analyze the stratigraphic architecture of two well-exposed ancient alluvial fans in the western US – the Eocene Richards Mountain Conglomerate and the Cretaceous Echo Canyon Conglomerate. Both fans were deposited under relatively warm, wet climates and compressional tectonic regimes. We use a seven-fold hierarchy of bounding surfaces and associated lithosomes to describe alluvial fan architecture. First- through fourth-order surfaces and lithosomes represent bedform to channel-scale features influenced primarily by autogenic processes on the fan. Controls on fifth-order surfaces/lithosomes have historically been poorly understood, but probably represent fanhead trench migration and lobe construction. Sixth-order surfaces bound individual alluvial fans and seventh-order surfaces correspond to formation boundaries. These are controlled primarily by tectonics. The fifth-order architectural style of the deposits in our two study areas is significantly different and we use this difference to try to isolate a primary control on fifth-order alluvial architecture. Average width:height ratios of fifth-order lithosomes are nearly twice as high for Echo Canyon (112:1) than for Richards Mountain (64:1). This indicates that active channels on the Echo Canyon fan were more mobile than those on the Richards Mountain fan. We attribute this to a more seasonal climate and less vegetation during the deposition of the Echo Canyon Conglomerate (relative to Richards Mountain). This would have increased lateral migration by destabilizing channels through increased sediment flux and flood events. Our results imply that fifth-order stratigraphic architecture of ancient alluvial fans may provide insight into allogenic processes related to paleoclimate. They also indicate risk of increased geologic hazards on alluvial fans where anthropogenic climate change increases future climate variability.
Introduction
Stratigraphic Architecture of Alluvial Fans
Alluvial fans are a significant part of the sediment routing system in areas of high relief. They form as rivers lose flow velocity after exiting confined mountain drainages (Bull, 1964). This promotes deposition of coarse-grained sediments which form a characteristic fan shape as the location of active deposition migrates back and forth over time (Bull, 1977; Leeder et al., 1998; Harvey et al., 2005; Blair and McPherson, 2009). Because alluvial fans necessarily occur in tectonically active regions they have the potential to preserve stratigraphic records of local tectonic events as well as climate (e.g., Dade and Verdeyen, 2007). Processes operating on the fan influence the distribution of various facies and the stratigraphic architecture (i.e., the pattern of channel stacking and sizes) within the fan. Studying stratigraphic architecture can therefore help reveal the tectonic and climatic conditions under which a fan was deposited and a wealth of studies exist which attempt to do just this (e.g., Gunster and Skowronek, 2001; Bettis, 2003; Harvey et al., 2003, 2005; Klinger et al., 2003). In practice, it is difficult to obtain detailed information on high-frequency climate and tectonic events from fan sedimentology due to difficulties with high-resolution dating of alluvial fan deposits, high temporal variability in fan sedimentation rates and lack of control in field-based studies (Dorn, 2009). However, general predictions of tectonic and climatic conditions over larger time scales of thousands to millions of years from stratigraphic architecture (as we attempt in this study) are more feasible.
Alluvial fan architecture may be controlled by different processes (Crews, 1985; DeCelles et al., 1991; Heller and Paola, 1996; Whipple et al., 1998; Allen et al., 2013) including allogenic processes such as tectonics (e.g., gross basin geometry, rates of subsidence, control of sediment supply via uplift and erosion of source terrain), climate (e.g., discharge controlled by precipitation levels, arid vs. humid settings, vegetation cover), or base level changes (which may be related to changes in climate or tectonic activity). Autogenic processes can also act as an important control on alluvial architecture (e.g., Bull, 1991; Parker et al., 1998; Clarke et al., 2010; Hofmann et al., 2011; Hajek et al., 2012; Miller et al., 2014; Ventra and Clarke, 2018; Bowman, 2019). Common autogenic processes on alluvial fans include failure of oversteepened slopes (resulting in debris flows), channel avulsion and lobe switching in response to sediment accumulation, incision and backfilling of fanhead trenches, sheet flow, and migration of channels, bars, and bedforms.
Stratigraphic architecture has been extensively studied in fluvial systems (e.g., Miall, 1985; Heller and Paola, 1996; Nichols and Fisher, 2007; Straub et al., 2009) but has been historically understudied in alluvial fans. Previous work has concluded that small-scale architecture (channel-scale and smaller) is controlled largely by autogenic processes, whereas large-scale architecture (fan-scale and above) is predominantly a result of long-term tectonic activity (DeCelles et al., 1991). Our study seeks to improve understanding of which processes are most important in controlling the style of intermediate-scale (channel complex) alluvial fan architecture by examining the intermediate-scale architecture of two ancient alluvial fan deposits in the western US that were deposited under similar tectonic and climatic settings. We use the architectural hierarchy established by DeCelles et al. (1991) as a starting point for this comparative study. DeCelles et al. (1991) created a seven-fold hierarchy of lithosomes (genetically related bodies of rock) to describe the internal organization of the Beartooth Conglomerate (Table 1 and Figure 1). The Beartooth Conglomerate is an informally named unit of upper Paleocene conglomerates exposed along the eastern flank of the Beartooth Range on the Montana/Wyoming border (Figure 2). The tectonic and climatic conditions (e.g., a compressional tectonic setting and relatively warm, humid climate) under which the Beartooth Conglomerate was deposited were similar to the conditions under which the Echo Canyon and Richards Mountain Conglomerates were deposited (DeCelles et al., 1991; Table 2).
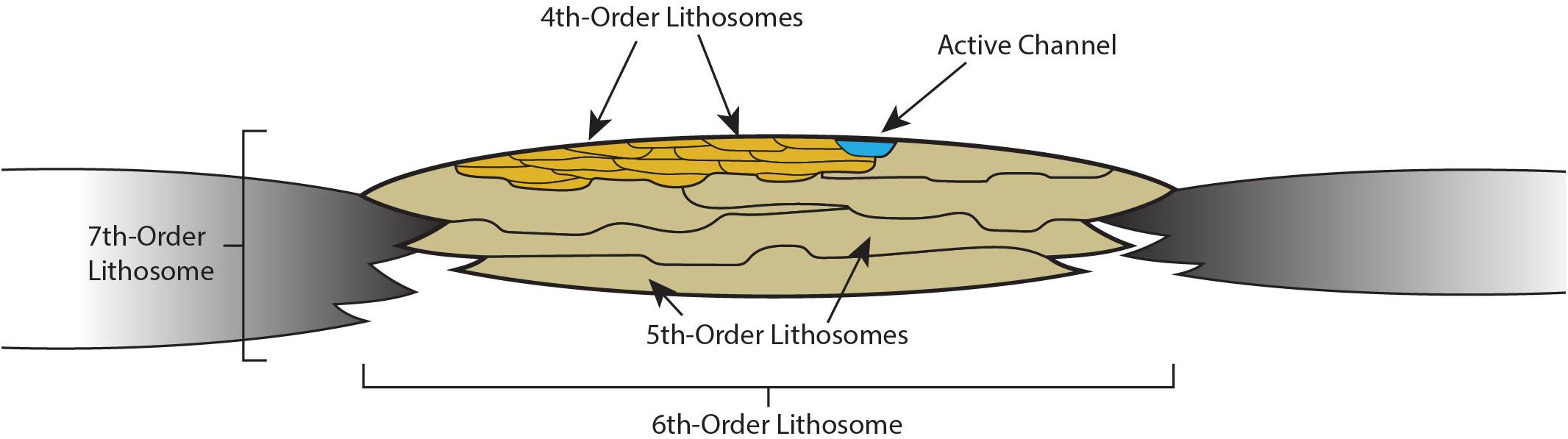
Figure 1. Simplified representation of the lithosomal hierarchy described in DeCelles et al. (1991). First- through third-order lithosomes and bounding surfaces represent bedforms, scour surfaces, and large-scale cross-strata (generally at sub-meter scale) and combine to form channel-filling fourth-order lithosomes (up to several meters thick and tens to hundreds of meters wide). As channels migrate across the fan, they leave fifth-order lithosomes composed of amalgamated fourth order lithosomes (tens of meters thick and hundreds to thousands of meters wide). Sixth- and seventh-order lithosomes correspond to individual alluvial fans and laterally amalgamated fans respectively (several hundred meters thick and several thousand meters wide). See Table 2 for details of the lithosomal hierarchy.
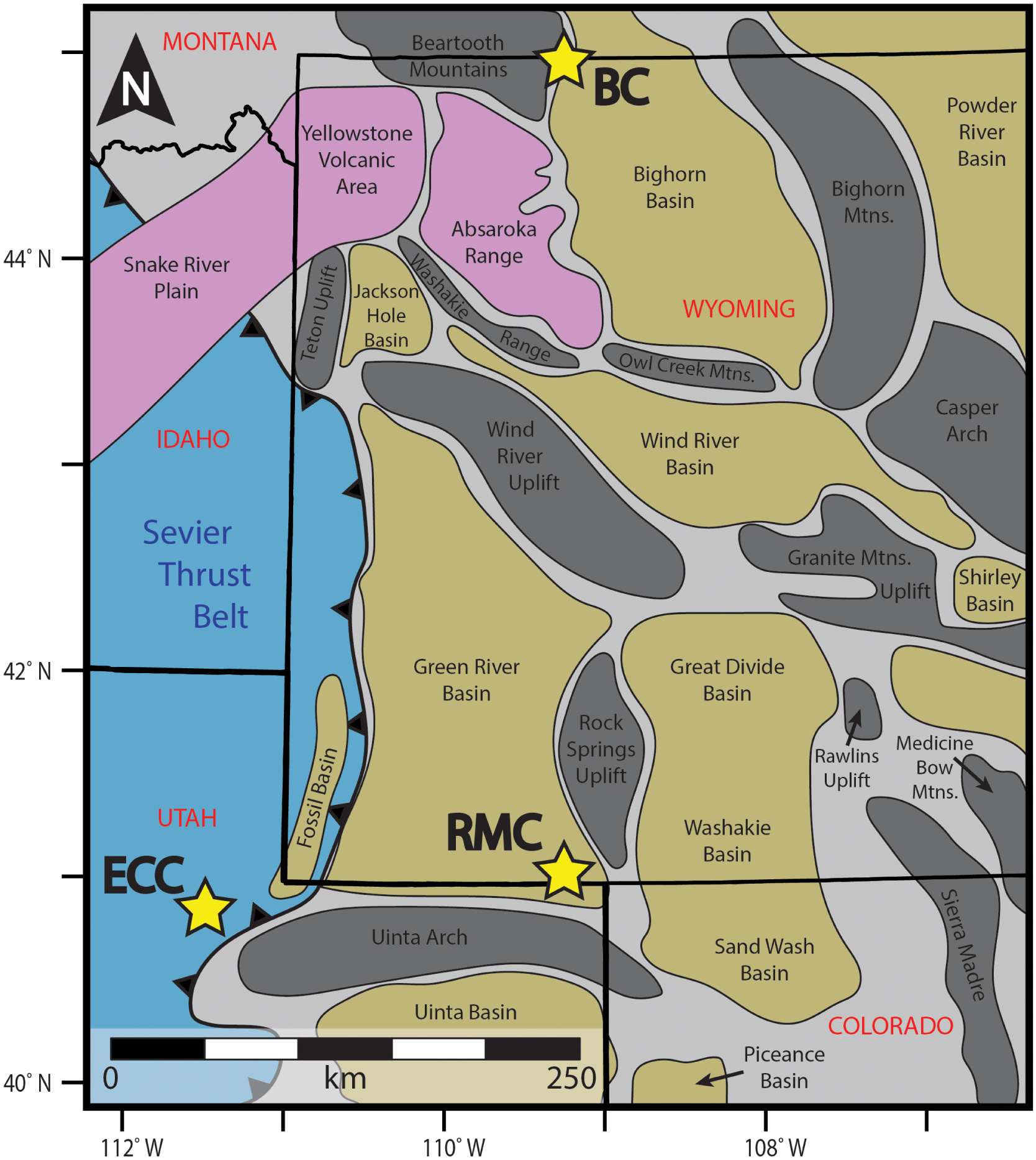
Figure 2. Surface locations of major structural features in the region surrounding the study areas. Locations of the Echo Canyon Conglomerate (ECC), Richards Mountain Conglomerate (RMC), and Beartooth Conglomerate (BC) are marked by stars. The Echo Canyon Conglomerate was shed from the Sevier Thrust Belt while the Richards Mountain Conglomerate and Beartooth Conglomerates were sourced from the Uinta and Beartooth Ranges respectively (both Laramide structures). Modified from Lamerson (1982), Roehler (1992), Smith et al. (2008), and Törö et al. (2015).
The real importance of better understanding alluvial architecture is the potential to link depositional conditions to fan dynamics. Alluvial fans are complex systems that have historically been poorly understood (e.g., Bull, 1977; Lecce, 1990) and alluvial architecture can be a powerful tool for interpreting the rock record and the path sediment follows from source to sink (Armitage et al., 2011; Michael et al., 2014; Romans et al., 2016; D’Arcy et al., 2017). Alluvial deposits commonly form important aquifers (Neton et al., 1994; Zhu et al., 2017) and occasionally serve as hydrocarbon reservoirs (Shepherd, 2009) where their internal structure could affect fluid flow paths. They also commonly occur in highly developed areas, and because they are associated with mountainous terrain and debris flows (Larsen et al., 2001), understanding how they function is important for geologic hazard mitigation. This study is particularly relevant in addressing the question of how processes operating on alluvial fans will change in response to climatic variation, considering the rapid climate change occurring at present across the globe.
Study Areas
Two field sites were examined for this study: Echo Canyon, UT and Richards Mountain, WY (Figures 2, 3). Both locations have well-exposed ancient alluvial fan deposits, known as the Echo Canyon Conglomerate and Richards Mountain Conglomerate, respectively (Figure 3). These fans were deposited under similar tectonic and climatic settings (e.g., Crawford, 1979; Crews and Ethridge, 1993; DeCelles, 1994) which are summarized in Table 2 (along with the depositional conditions for the Beartooth Conglomerate). In theory, we would expect this to result in similar architectural styles. Differences in architectural style would indicate that at least one of a number of controlling factors differed between the two sites. We compare the two study areas by quantitatively describing the alluvial architecture (specifically, the width:height ratios of fifth-order lithosomes, which represent channel complexes) at both sites.
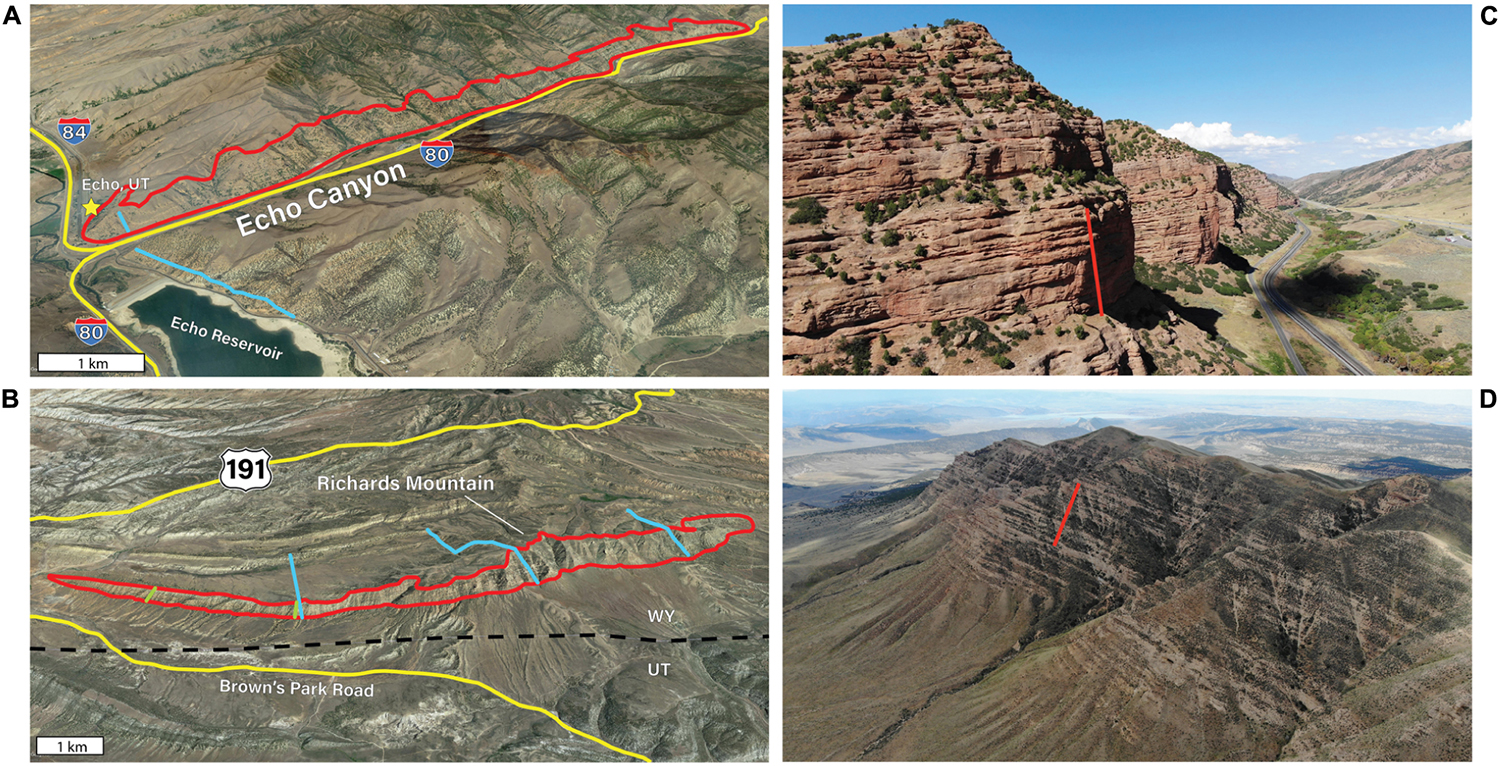
Figure 3. Overview of study areas. For (A) and (B), areas outlined in red indicate approximate extent of digital outcrop models. Blue lines indicate locations of sedimentary logs from previous workers. Green lines indicate locations of sedimentary logs measured by the authors. Roads are shown in yellow. Satellite imagery modified from Google Earth (2019). (A) Oblique view (looking north) of Echo Canyon study area. Outcrops are exposed along the north side of Echo Canyon, immediately northeast of the town of Echo, UT. Area outlined in red is approximately 11 km long. Sedimentary log from DeCelles (1994). (B) Oblique view (looking north) of the Richards Mountain study area. Richards Mountain is located in southwestern Wyoming, just north of the UT-WY border and east of the intersection of US 191 and Brown’s Park Road. Sedimentary logs in blue are from Crews (1985). Logs in green measured by S. Meek. Area outlined in red is approximately 13 km long. (C) The Echo Canyon Conglomerate crops out in sheer red cliffs over the first 11 km of Echo Canyon in northeastern Utah. View is to the northeast. Red line is approximately 60 m long. Red cliffs are predominantly clast-supported massive conglomerate with interbedded sandstone. Slope covered sections generally correspond to paleosols and/or mudstones. (D) The Richards Mountain Conglomerate is exposed in the 13 km hogback that forms Richards Mountain in southern Wyoming, just north of the Uinta Mountains. View is to the west. Red line is approximately 200 m long. Light colored bands are thick conglomerate beds. Vegetation-covered areas largely correspond with paleosols.
Eocene Richards Mountain Conglomerate (Wyoming)
The Richards Mountain Conglomerate is a widely used informal name for a coarse-grained alluvial fan deposited between ca. 56 and 53 Ma within the Eocene Wasatch Formation (Culbertson, 1969; Crews, 1985; Crews and Ethridge, 1993). The Richards Mountain Conglomerate holds up an approximately 13 km hogback that forms Richards Mountain in southern Wyoming (Figures 2, 3). The southern face of the mountain exposes a strike-parallel cross-section through a single alluvial fan shed into a foreland basin on the northern flank of the Laramide Uinta Arch (from which the fan sediments were sourced). Average paleoflow direction was to the north (Crews, 1985). The Richards Mountain fan conformably overlies fluvial rocks of the main body of the Wasatch Formation. The top of the conglomerate is a fan-delta and grades upward into lacustrine facies of the Wasatch and Green River formations. Basinward (north) the fan rapidly fines and interfingers with lacustrine and fluvial facies of the main body of the Wasatch Formation (Crews, 1985; Crews and Ethridge, 1993). The fan itself is notable for its abundant paleosols and pervasive pedogenic alteration (Figures 4, 5), as well as a distinct lack of the debris-flow facies (Crews and Ethridge, 1993) common to alluvial fans (Shultz, 1984; Larsen et al., 2001; Blair and McPherson, 2009). Climate at the time of deposition (shortly after the PETM) was warm and humid (Brooks, 1949; Frakes, 1979; Wolfe, 1980; Thrasher and Sloan, 2009). Evidence for a humid climate (Table 2) comes from the abundant paleosol development and the stream-flow dominated nature of the fan as well as from fossil plants characteristic of a warm, rainy forest or jungle (Crews and Ethridge, 1993).
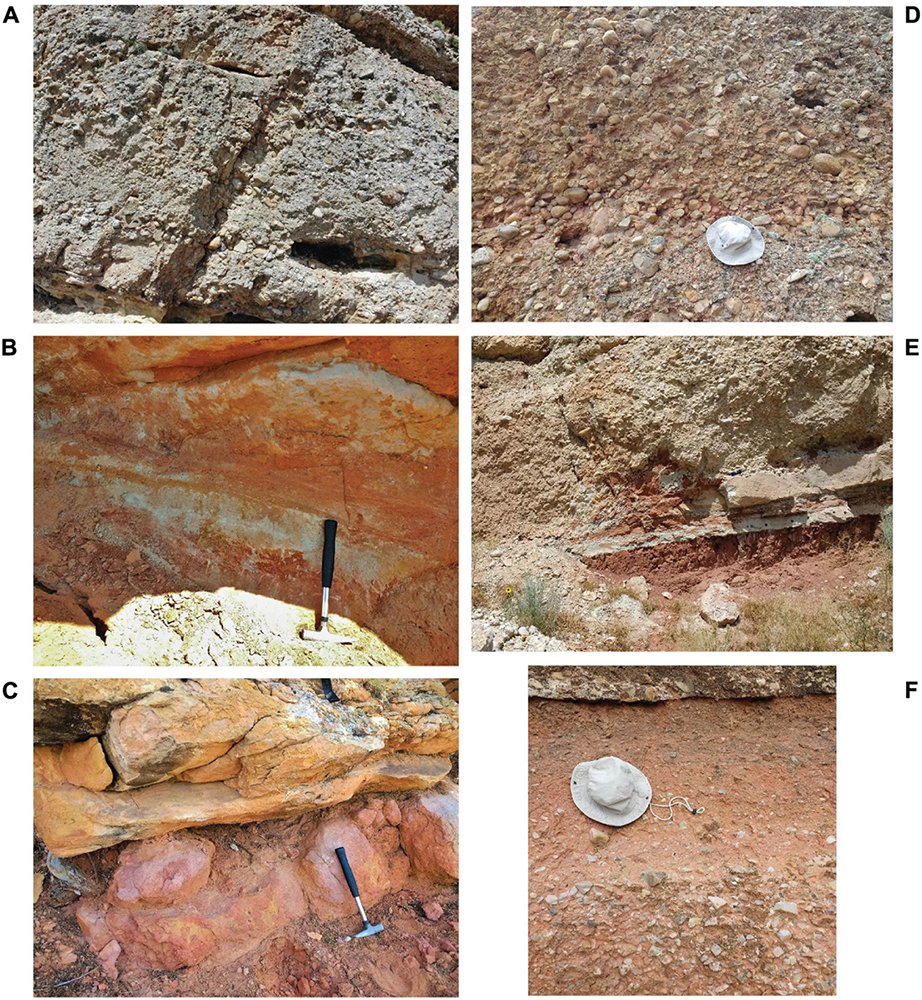
Figure 4. Key facies found in the Richards Mountain Conglomerate (A–C) and Echo Canyon Conglomerate (D–F). (A) Clast-supported structureless to poorly stratified cobble and boulder conglomerates. Interpreted as channel deposits on a streamflow-dominated alluvial fan. (B) Fine-grained sandstone with mottled red and yellow colors and ripple/trough-cross-stratification or structureless texture. Rhizoliths are abundant (light-colored, linear features near handle of hammer). Interpreted as sandy channel deposits that have undergone early pedogenesis. (C) Fine-grained, structureless mudstones (lower pink bed) with abundant rhizoliths and red and yellow mottling. Interpreted as mature paleosols which frequently cap the conglomerate and sandstone channel complexes that make up the bulk of fifth-order lithosomes. (D) Clast-supported, structureless cobble conglomerates. Interpreted as channel deposits probably emplaced in high-intensity, short-duration flows or possibly as debris flows. (E) Largely, structureless red mudstones with rhizoliths and some mottling of color at bottom of image. Interpreted as paleosols formed on inactive fan segments; frequently scoured away by overlying channel conglomerates (as also seen in this image). (F) Clast-supported, angular pebble to cobble conglomerate grading upward into matrix-supported angular pebble conglomerate. Interpreted as deposits from debris-flows or highly concentrated flows due to matrix-support and highly angular clasts.
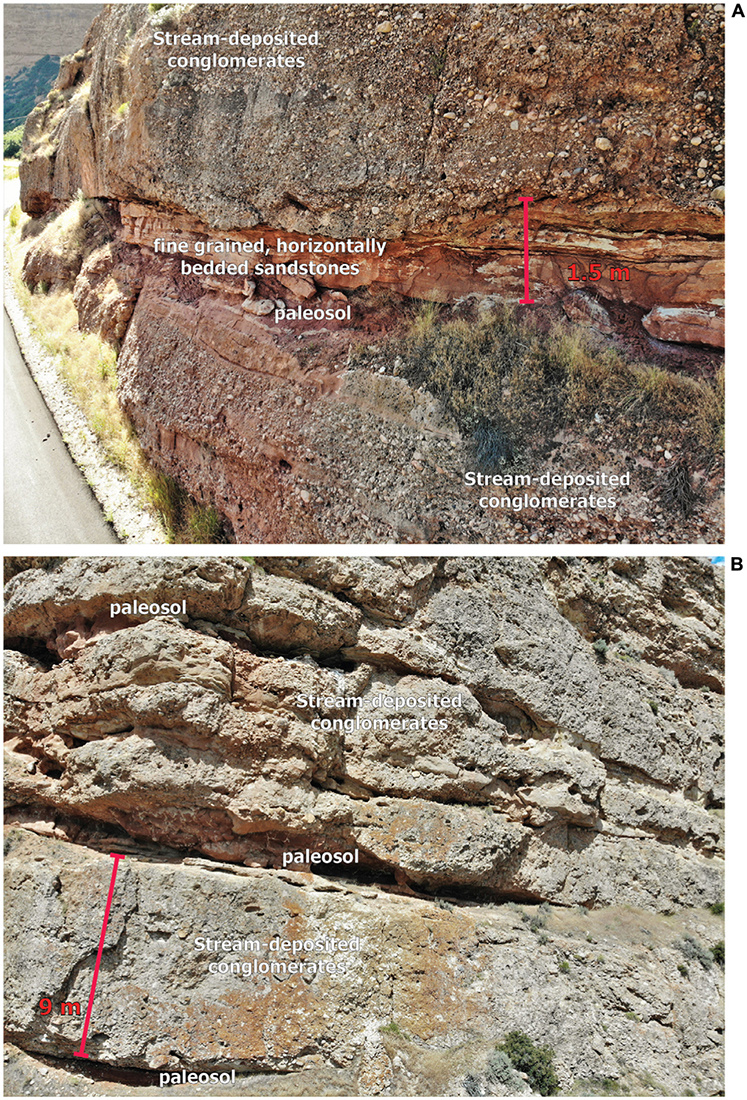
Figure 5. Representative outcrops showing common facies associations in study areas. Coarse-grained conglomeratic units representing channel deposits are usually capped by paleosol horizons, which are in turn scoured into by overlying conglomerates. (A) The Echo Canyon Conglomerate. Shown in this image are clast-supported, cobble conglomerates above and below a relatively fine-grained interval containing paleosols and thin-bedded sandstones. While these conglomerates are interpreted as having been deposited by streamflow due to imbrication and trough-cross-stratification, structureless clast-supported conglomerates are quite common in the Echo Canyon Conglomerate and are interpreted as debris-flow deposits. Clast- to matrix- supported very angular conglomerates are rare (but present) and are also interpreted as deposits from debris-flows or highly concentrated flows. (B) The Richards Mountain Conglomerate. Common facies shown here are clast-supported, cobble to boulder conglomerates (interpreted as streamflow deposits) and fine-grained paleosol intervals. Notice the mottled, reddish coloration of paleosol intervals. Rhizoliths are visible in the central paleosol as light-colored lines in the red unit.
Cretaceous Echo Canyon Conglomerate (Utah)
The Echo Canyon Conglomerate has been palynologically dated as Coniacian-Santonian in age (Jacobson and Nichols, 1982) and was deposited between ca. 87 and 85 Ma (Jacobson and Nichols, 1982; DeCelles, 1994; Painter et al., 2014). The unit crops out along Interstate Highway 80 over about 11 km of Echo Canyon in northeastern Utah (Figures 2, 3), where it is spectacularly exposed (particularly on the northwest side of the canyon). The outcrop cuts obliquely through the fan relative to paleoflow direction, which was predominantly eastward (DeCelles, 1994). It was deposited by multiple, coalesced alluvial fans in the proximal foreland basin or wedgetop of the Utah-Idaho-Wyoming salient of the Sevier Thrust Belt and sourced mainly from the Willard Thrust Sheet (DeCelles, 1988). The Echo Canyon Conglomerate conformably overlays fluvial to shallow marine deposits of the Henefer Formation (interpreted as a progradational fan-delta-front sequences) and is overlain by an angular unconformity with alluvial deposits of the Evanston Formation (DeCelles, 1994; Figure 6). Lateral facies changes in the Echo Canyon Conglomerate are largely unknown, as the margins of the fan have been lost to synorogenic erosion. The Echo Canyon Conglomerate has been interpreted to have been deposited during warm, subhumid-humid climate typical of western North America during the Late Cretaceous Epoch (Crawford, 1979; Crawford and Dott, 1979; Franczyk et al., 1992; Burgener et al., 2019). This is further supported by abundant plant fossils, paleosol development, and mudstones that may have been deposited in standing bodies of water (Crawford, 1979). It is important to note however, that, unlike the Richards Mountain Conglomerate, debris-flow facies are present at Echo Canyon (Crawford, 1979; DeCelles, 1994), although they are a relatively minor component of the section (Figures 4, 5).
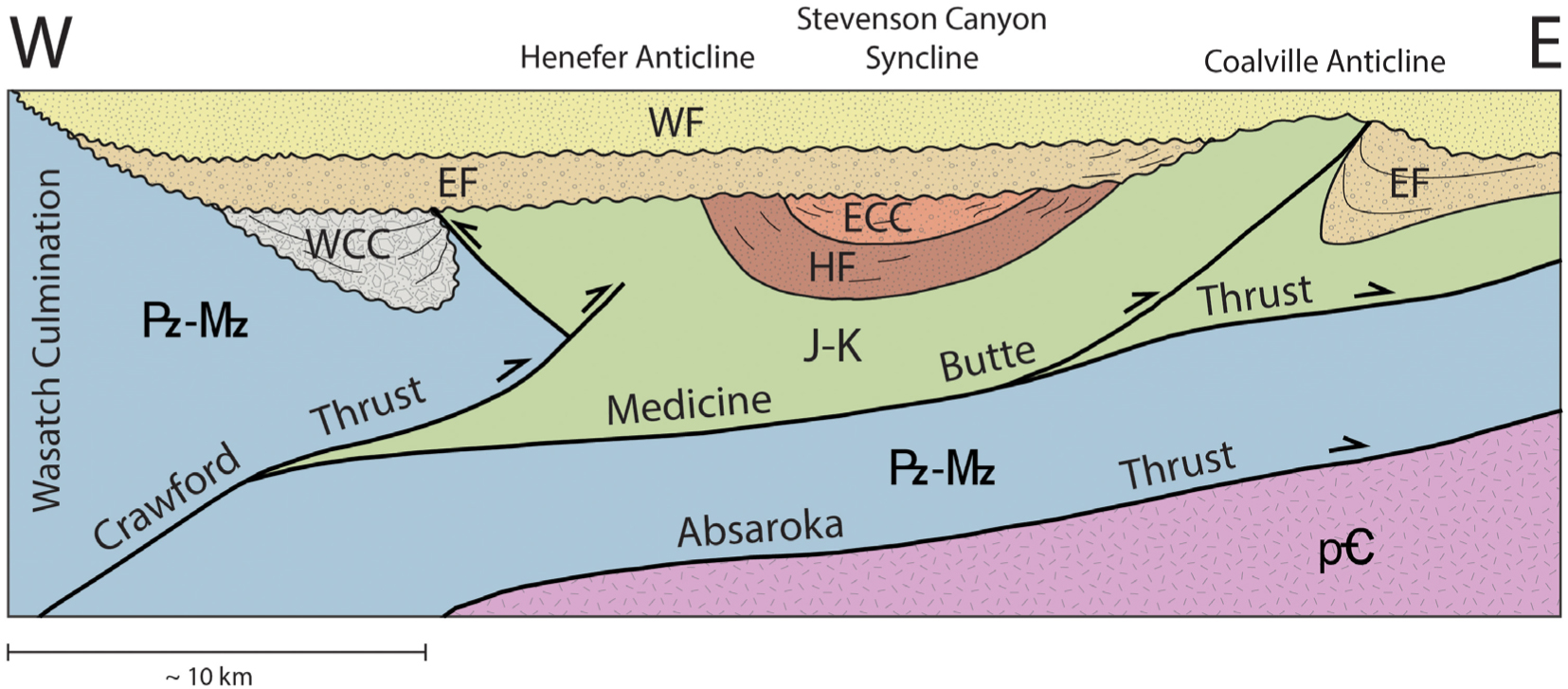
Figure 6. Schematic cross-section showing key structural elements and stratigraphic relationships of the synorogenic sediments near the Echo Canyon study area. There is no vertical exaggeration; however, relative formation thicknesses are only roughly to scale. The Echo Canyon Conglomerate (ECC) is confined to the Stevenson Canyon Syncline where conformably overlies the fluvial Henefer Formation (HF). The ECC is truncated above by an angular unconformity with conglomerates of the Evanston Formation (EF). Also represented here are conglomerates of the Wasatch Formation (WF) and the Weber Canyon Conglomerate (WCC), the Paleozoic to Mesozoic (|-}) rocks of the Crawford and Absaroka thrust sheets, the Jurassic to Cretaceous (J-K) rocks of the Medicine Butte Thrust sheet, and the underlying Precambrian crystalline basement rocks (=). Modified from DeCelles (1994).
Materials and Methods
Data on alluvial architecture was obtained from digital outcrop models generated using photogrammetry. These models are available in the data repository. Photogrammetric modeling is a process by which photos of an object taken from multiple vantage points can be combined into a spatially accurate three-dimensional model of that object. Though a relatively new technique, the use of digital outcrop models built using photogrammetric techniques has been well established as an effective tool for the geosciences (e.g., Fabuel-Perez et al., 2010; Bemis et al., 2014; García-Sellés et al., 2014; Smith et al., 2015; Carrivick et al., 2016). Key outcrops were photographed using a GPS-enabled quadcopter drone (DJI Mavic Air) which enables quick, cost-effective data collection over large areas, including inaccessible cliff faces. Photos were taken from distances of around 100–300 m from the outcrop. With a 12-megapixel camera on the drone this gives pixel sizes (and thus model resolution) of <10 cm. Images of each portion of the outcrop were taken from multiple angles and with significant overlap in order to provide the modeling software with sufficient data to match key points in the imagery and stitch together a model with full outcrop coverage. This required roughly 1,000–2,000 images at each study site. Digital outcrop models were built using the standard workflow in the Agisoft Metashape software package. Models were built in several individual sections, referred to in the Metashape software as “chunks.” While not required, building the model in these pieces is helpful when working with a large number of images, as each chunk uses only around 50–300 images. This allows for faster processing and avoids some issues with correlating images that were, for example, taken on different days under slightly different lighting conditions. The chunks can later be merged to produce a complete model of the outcrop. Building the model involves first uploading images to the individual chunks, then cropping images to remove unwanted portions (like the sky or distant mountains). 40,000 key points are then automatically identified on each image. 4,000 of these are used on each image to align it with overlapping images. Once tie points are in place, they are used to create a sparse point cloud which can be manually or automatically edited to remove misplaced points. A dense point cloud can then be generated at the desired resolution. High quality point clouds are generally better for detailed interpretation; however, lower quality point clouds are easier to manipulate for large areas. We created both low- and high-resolution point clouds for these purposes. Our high-resolution models contain around 70 million points per thousand images used. The final steps in model creation involve automatically generating a triangular mesh based on the dense point cloud and overlaying imagery onto this mesh as a “textured surface.” Again, both of these steps can be performed at either high- or low-resolution.
The final result of this process is a 3D, georeferenced digital model of the outcrop. These digital outcrop models provide high-resolution (features <10 cm are clearly visible) 2D and 3D outcrop data that would be difficult to accurately represent using sedimentary logs alone (models are available in the data repository). This level of coverage allows comprehensive analysis of alluvial architecture over the 10 + km exposures in this study, rather than just isolated outcrops. It should be noted that while exposure is excellent perpendicular to depositional dip in both study areas, there is essentially no downdip exposure of the Richards Mountain Conglomerate, and only limited downdip exposure of the Echo Canyon Conglomerate. We will therefore focus on architectural patterns and lithesome measurements only in a strike-parallel direction. Lithosome dimensions (summary in Table 3) were measured directly from the photogrammetric models by using the measure tool in Metashape. These measurements have sub-meter accuracy. Alluvial architecture of the study areas was described by qualitatively describing stacking patterns and quantifying dimensions (width and height, i.e., thickness) of lithosomes. We emphasize width:height ratios of fifth-order lithosomes as the forcing mechanisms behind these intermediate-scale architectural elements are poorly understood. Not all fifth-order lithosomes were measured due to poor exposure and/or incompleteness due to erosion of outcrops. Attempts were made, however, to measure as many of the most complete lithosomes as possible and to draw these measurements from all exposed portions of the fans in order to have a truly representative sample. A total of 41 fifth-order lithosomes were measured – 18 in Echo Canyon and 23 at Richards Mountain (all measurements available in Supplementary Table S1).
Interpretations of the digital outcrop models were informed by detailed sedimentary logs at each field site. Sections measured by the authors (Figure 7) were used in combination with sections measured by Crews (1985) and DeCelles (1994). These detailed sections were compared to the photogrammetric models to ensure accurate characterization of the facies within each formation (Figure 8), which is critical to correctly interpreting physical processes. Bounding surfaces of fifth-order lithosomes in outcrop are marked by the presence of major erosional surfaces at the base of thick packages of fourth-order lithosomes (Table 1). These frequently consist of conglomerates scouring into fine-grained paleosol caps on the underlying fifth-order lithosome (Figure 9). On a large scale (e.g., on the photogrammetric models) these surfaces can generally be recognized by the presence of a laterally extensive, thin and recessive boundary between packages of fourth order lithosomes. This recessive boundary corresponds to the paleosol caps on fifth-order lithsomes. These surfaces were identified and interpreted in Metashape (examples in Figure 10). Thickness can vary significantly across the fifth-order lithosomes due to scouring, pinching out of lithosomes and/or a lensoidal geometries. Heights for fifth-order lithosomes were therefore measured at the point of maximum thickness in order to provide a minimum estimate for the true maximum thickness of the lithosome (Table 3 and Supplementary Table S1).
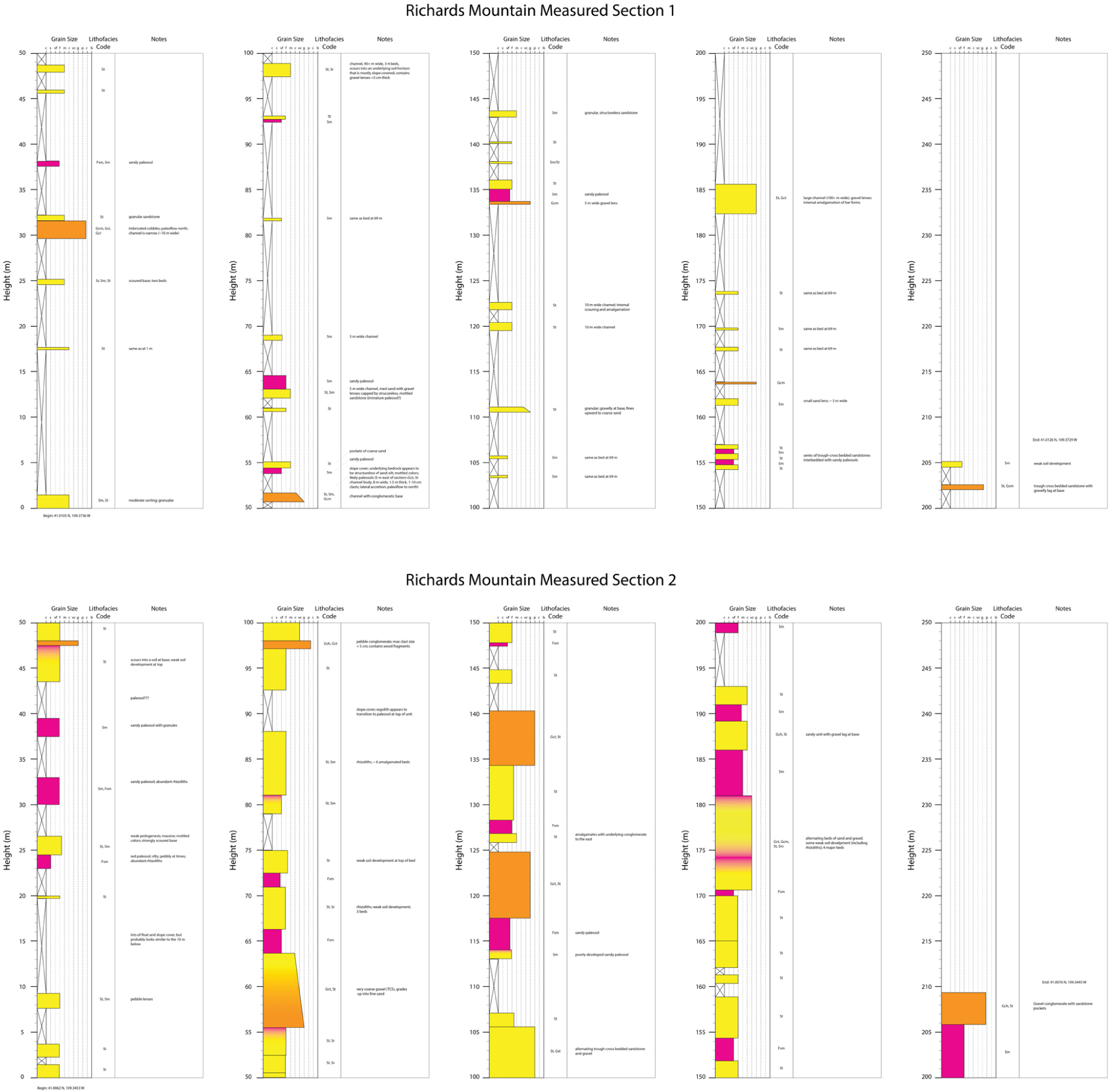
Figure 7. Details of sedimentary logs measured at Richards Mountain by the authors. Additional sedimentary logs used for interpreting the deposits at Richards Mountain can be found in Crews (1985). Facies codes represent the following: Fsm = Fine-grained, massive/structureless. Usually paleosols. Sm = massive/structureless sandstone. Usually indicates bioturbation and early pedogenesis in these logs. St = trough-cross-stratified sandstone. Sr = ripple-cross-stratified sandstone. Gcm = clast-supported massive/structureless conglomerates. Gct = clast-supported trough-cross-stratified conglomerates. Gci = clast-supported, imbricated conglomerates, Gch = clast-supported, horizontally bedded conglomerates.
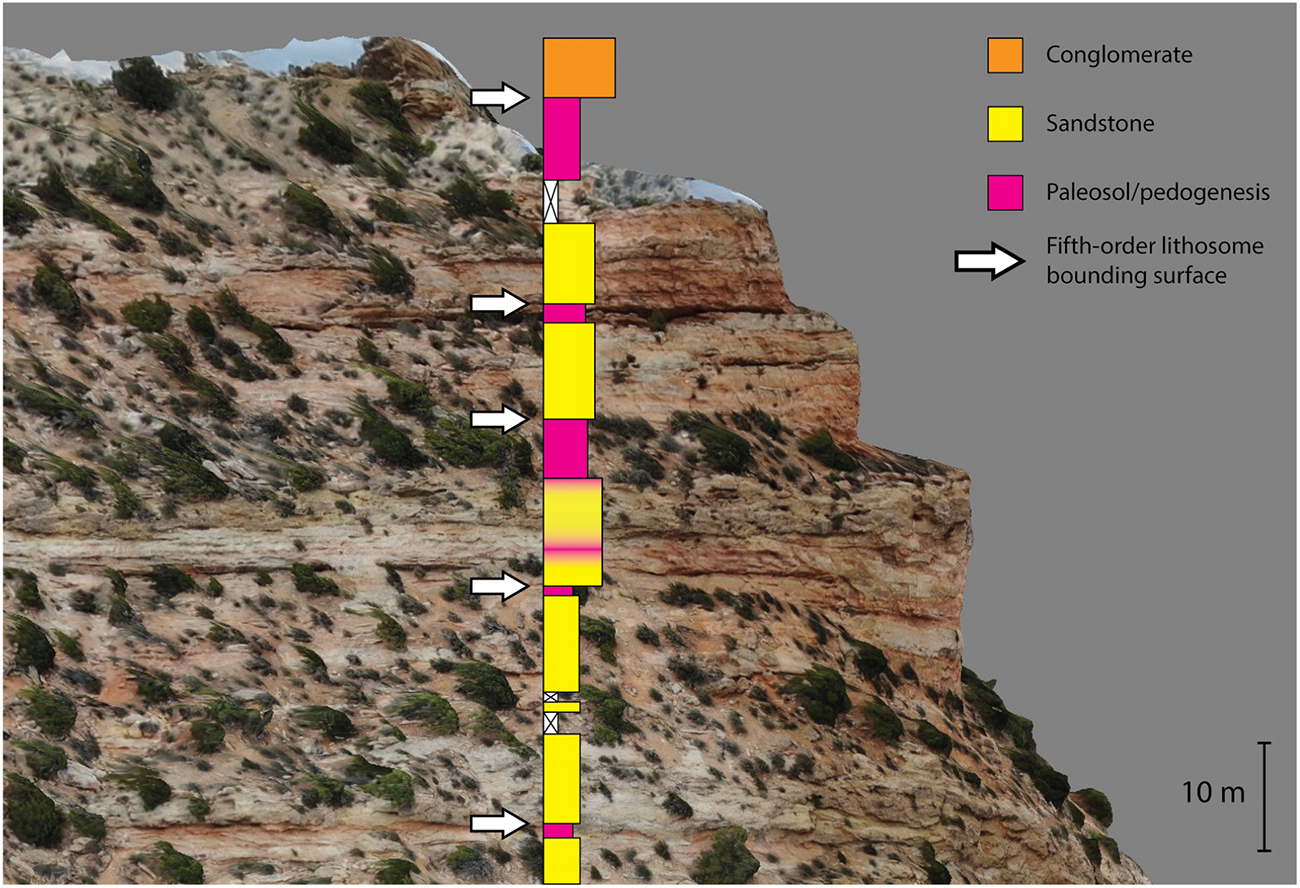
Figure 8. Small portion of the digital outcrop model from the western end of Richards Mountain with a simplified portion of the Richards Mountain Measured Section 2 (Figure 7B) overlain for facies correlation. Fifth-order bounding surfaces are marked by white arrows. These bounding surfaces can generally be recognized by their lateral continuity and the frequent presence of a recessive paleosol cap on the underlying lithosome. The boundary between lithosomes is placed at the top of this paleosol cap, where channel-filling sandstones or conglomerates of the overlying lithosome scour into the paleosols. Note that each fifth-order bounding surface corresponds with the top of a paleosol on the sedimentary log.
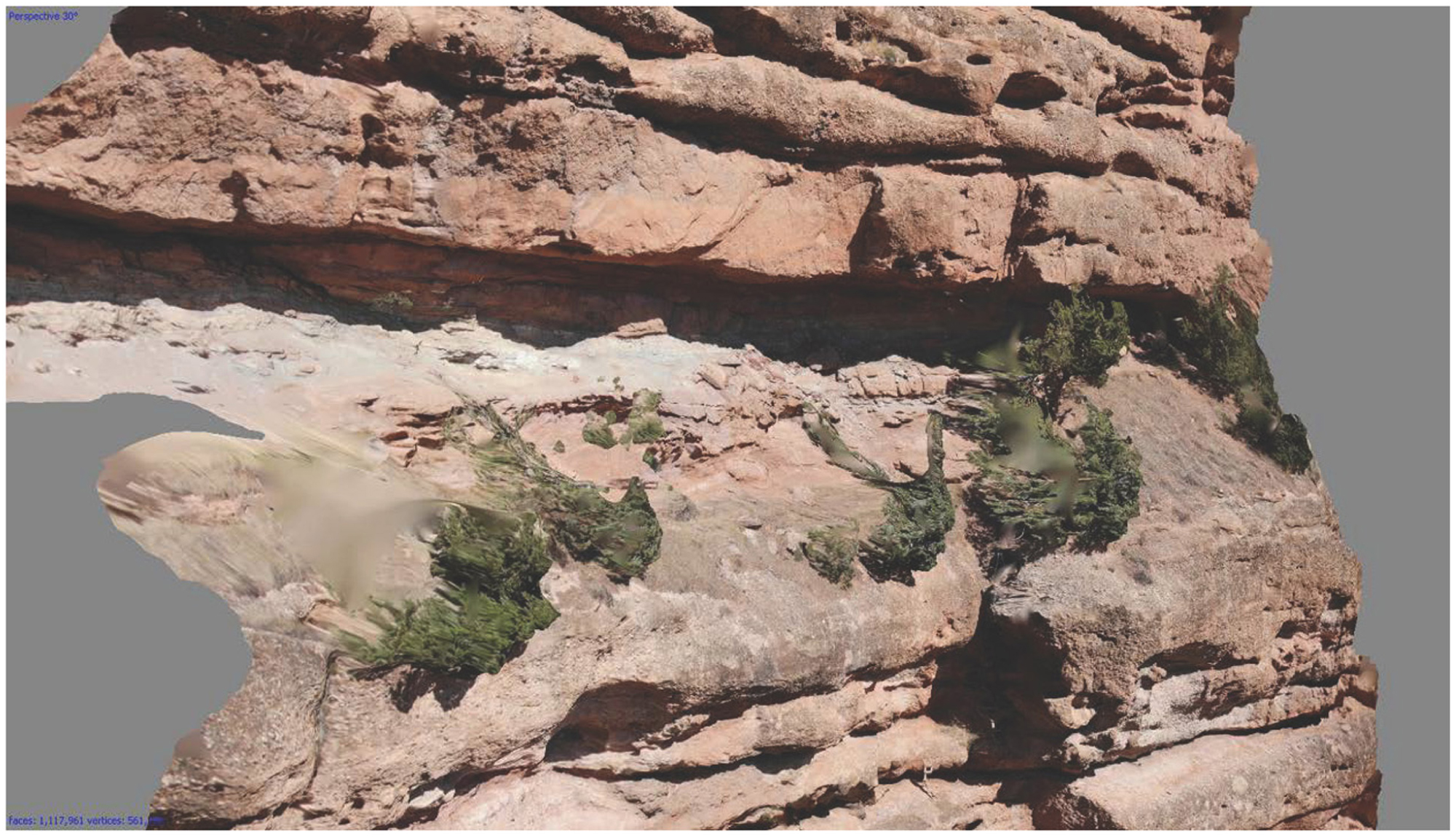
Figure 9. Close-up view the “Detailed Section” digital outcrop model from Echo Canyon (available in the data repository). Fourth-order lithosomes can be clearly seen as individual beds in the conglomerates at the top and bottom of the image. In the middle of the image is a light-colored, recessive unit that consists of green and red fine-grained, thinly bedded sandstones as well as structureless mudstones interpreted as paleosols. The top of this recessive unit corresponds to a fifth-order bounding surface. See juniper trees for scale.
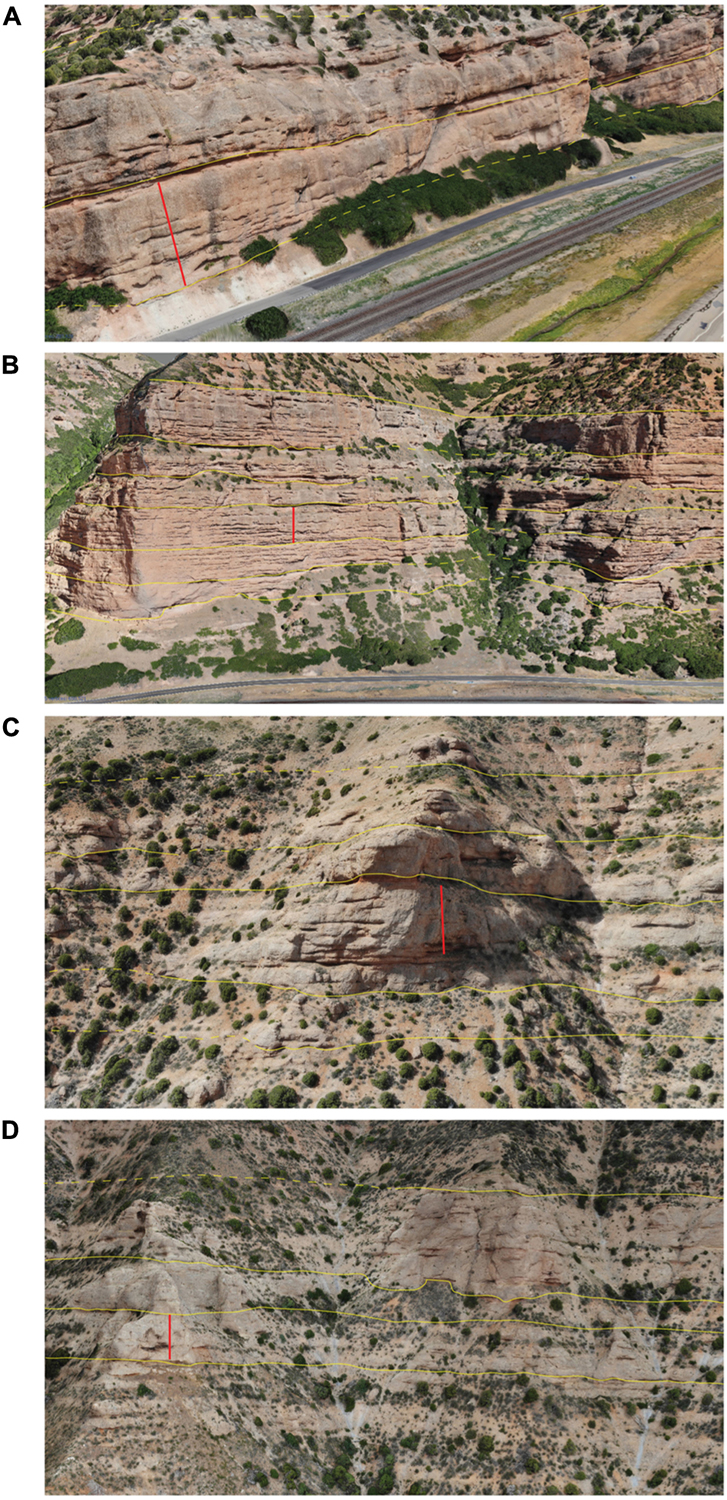
Figure 10. Interpreted selections from digital outcrop models. Fifth-order bounding surfaces are marked by yellow lines (dashed where uncertain or covered). These bounding surfaces can generally be recognized by their lateral continuity and the frequent presence of a paleosol cap on the underlying lithosome (though this is often removed by scouring from the overlying lithosome). Red lines on each images are approximately 25 m long. (A) and (B) are from the Echo Canyon model. (C) and (D) are from the Richards Mountain model.
It should be noted that the measured widths of fifth-order lithosomes are in all cases minimum estimates. At Richards Mountain this was due largely to outcrop quality – in many cases the edges of the channel complexes were poorly exposed as the conglomeratic and sandy units thinned. In Echo Canyon the reported widths are probably significantly more underestimated; nearly all of the measured lithosomes were truncated on one or both ends by normal faulting (which makes it difficult to correlate the strata within about 1 km of the canyon mouth) or by the angular unconformity with the overlying Evanston Formation (which has removed an unknown amount of the original depositional width of the channel complexes). Due to oblique exposure angles, apparent lithosome width was often far greater than the actual width; these widths were corrected for oblique exposure where necessary using the method outlined in Fabuel-Perez (2009), with the assumption of an average paleoflow direction to the east for Echo Canyon (DeCelles, 1994) and to the north for Richards Mountain (Crews and Ethridge, 1993). Average paleoflow direction was used in place of specific paleocurrent measurements from individual lithosomes due to the relative inaccessibility of many outcrops and the significant time savings involved. In smaller study areas, study areas where outcrops are readily accessible, and/or where ample time is available, individual paleocurrents would certainly be preferable to increase accuracy of width corrections. These paleocurrents would probably best be collected after identification of lithosomes from digital outcrop models when possible.
Results
First- Through Fourth-Order Lithosomes
Architectural styles of first-order through fourth-order lithosomes (Table 1) are predominantly shaped by autogenic sedimentary processes (DeCelles et al., 1991). Detailed measurements of these small-scale lithosomes were not made for this study; however, it is worth noting that observations in the field did not reveal any obvious differences in the average dimensions of these lithosomes between the two study areas. Major differences between the small-scale lithosomes of the two fans were mostly confined to lithology – for example, in the presence of debris-flow facies in Echo Canyon and the extensive pedogenic alteration to much of the Richards Mountain section.
Fifth-Order Lithosomes
Fifth-order lithosomes represent the deposits of channel complexes that migrate across alluvial fans (Table 1). The proximal parts of these channel complexes are formed by fanhead trenches, while the distal portions consist of depositional lobes (DeCelles et al., 1991). A total of 41 fifth-order lithosomes were measured; 23 at Richards Mountain and 18 in Echo Canyon (see Table 3 and Figure 11). At Richards Mountain, these ranged from approximately 300–5,000 m in width (averaging 1,742 m) and 13–43 m in height (averaging 26 m). Width:height ratio ranged from 13:1 to 181:1 and averaged 64:1. At Echo Canyon, fifth-order lithosomes ranged from approximately 1,150–3,800 m wide (averaging 2,505 m) and 14–33 m high (averaging 23 m). Width:height ratio in Echo Canyon ranged from 57:1 to 179:1 and averaged 112:1 (nearly twice the average at Richards Mountain). A two-sample t-test (one-tailed) showed that these values were truly significantly larger at Echo Canyon (M = 112, SD = 37) than at Richards Mountain (M = 64, SD = 37), t(39) = 3.46, p = 0.0007. Neither study area showed any obvious vertical trends in width:height ratios of fifth-order lithosomes. Width:height ratios did tend to be larger in the axial portion of the Richards Mountain fan than on the fan margins. The Echo Canyon fan did not have any clear trends in lateral variation of width:height ratios.
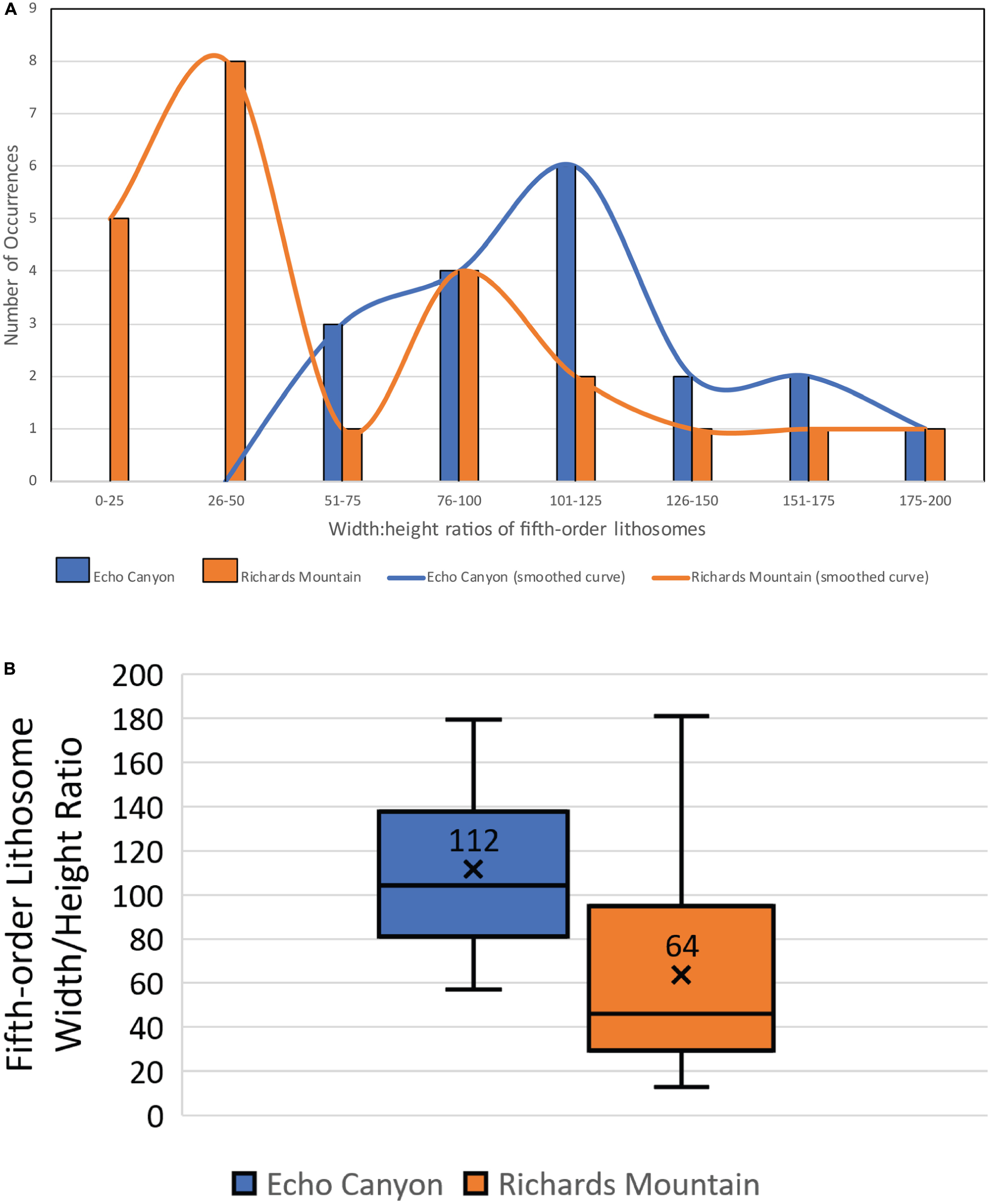
Figure 11. Statistical summary of width:height ratios of fifth-order lithosomes in the Echo Canyon Conglomerate and the Richards Mountain Conglomerate. Lithosome widths were corrected where necessary for oblique exposure angles. Height was measured at the point of maximum observed thickness. A two-sample t-test (one-tailed) showed that these ratios were significantly larger at Echo Canyon (M = 112, SD = 37) than at Richards Mountain (M = 64, SD = 37), t(39) = 3.46, p = 0.0007. (A) Distribution of fifth-order width:height ratios. Lines represent a smoothed curve of the distributions. Note that Echo Canyon width:height ratios tend to be larger than those at Richards Mountain. (B) Box-and-whisker plots showing range of width:height ratios for fifth-order lithosomes in the Echo Canyon and Richards Mountain Conglomerates. “X” indicates average. Note that average width:height ratios for fifth-order lithosomes in the Echo Canyon Conglomerate are nearly twice those observed in the Richards Mountain Conglomerate.
Stacking patterns and qualitative descriptions also varied between the two study areas. The fifth-order lithosomes in the Echo Canyon Conglomerate tend to have a tabular shape in cross-section, continue across the vast majority of the sixth-order lithosomes that contain them and have scoured away most of the paleosol caps on underlying channel complexes (although partial paleosol caps are common). In contrast, the fifth-order lithosomes of the Richards Mountain Conglomerate are more lenticular in shape, never traverse the entire fan and show a greater tendency toward compensational stacking patterns (i.e., axes of channel complexes are offset from one another). Figure 12 depicts a simplified version of the observed geometries and architectural styles. Additionally, the preservation of a thick and laterally continuous paleosol cap on fifth-order lithosomes is more common in the Richards Mountain fan relative to Echo Canyon.
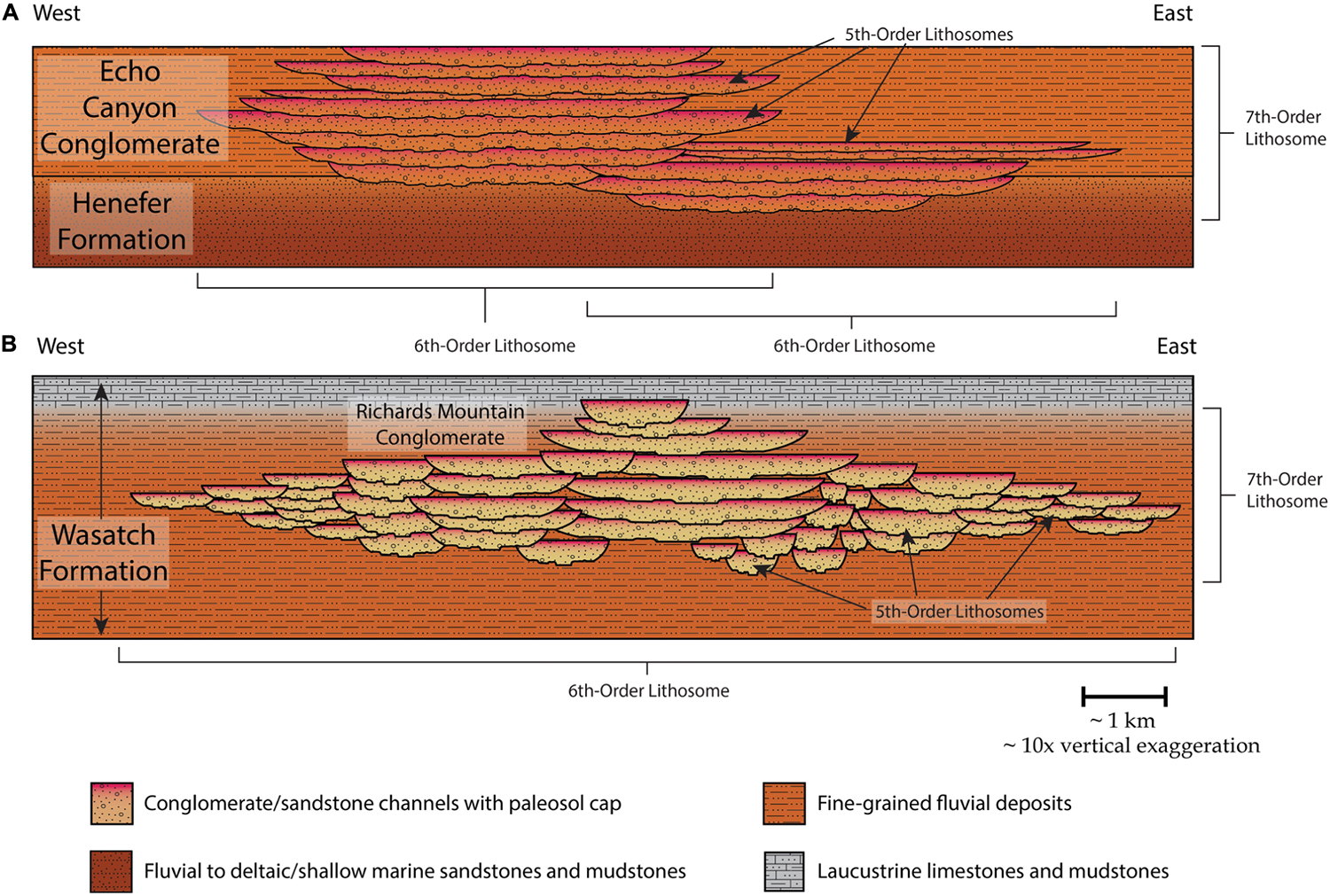
Figure 12. Simplified representation of the architectural styles of the Echo Canyon Conglomerate (A) and the Richards Mountain Conglomerate (B). Width:height ratios of fifth-order lithosomes are represented at their correct relative sizes between the two fans (with approximately10× vertical exaggeration). Stacking patterns of fifth-order lithosomes are represented schematically for both fans. While the dimensions, placement, and number of fifth-order lithosomes do not exactly match the actual deposits, general trends in the dimensions and placement of lithosomes do accurately represent the real study areas. Note that in the Echo Canyon Conglomerate, fifth-order lithosomes are organized into multiple sixth-order lithosomes (individual alluvial fans). Contrast this with the Richards Mountain Conglomerate, where the fifth-order lithosomes comprise only one, larger sixth-order lithosome. Note also that at Echo Canyon, the fifth-order lithosomes extend laterally across essentially the entire sixth-order lithosome that contains them, while at Richards Mountain the fifth-order lithosomes extend laterally across only a relatively small portion of their sixth-order lithosome.
Sixth- and Seventh-Order Lithosomes
Sixth- and seventh-order lithosomes (Table 1) were also not quantitatively analyzed for this study, but a few qualitative observations are informative, as they provide insights into the tectonic histories of the study areas. The Richards Mountain Conglomerate consists of a single sixth-order lithosome (an individual alluvial fan). The sixth-order surfaces bounding this lithosome consist of gradational contacts with fluvial and lacustrine facies of the Wasatch Formation above and below, respectively (Crews, 1985; Crews and Ethridge, 1993). These sixth-order surfaces coincide with the seventh-order surfaces that bound the entire Richards Mountain Conglomerate (since the entire section contains only one sixth-order lithosome). Other minor sixth-order lithosomes do exist along strike at the same stratigraphic interval within the Wasatch Formation (Crews, 1985; Crews and Ethridge, 1993); however, these do not overlap with the fan exposed on Richards Mountain.
The Echo Canyon Conglomerate displays quite a different large-scale architectural style. Here, the formation is composed of at least two overlapping fans (sixth-order lithosomes). Whether others existed along strike at the time of deposition is not known, although it seems likely. The sixth-order bounding surfaces are erosional contacts between the various fans. In Echo Canyon, the seventh-order bounding surfaces that encompass the formation consist of the transitional contact with the underlying fluvial Henefer Formation and an angular unconformity with the overlying Hams Fork Conglomerate of the Evanston Formation.
Discussion
Perhaps the most striking and intriguing of our results is the great difference in average width:height ratios of the fifth-order lithosomes in these two ancient alluvial fans. Fifth-order lithosomes of a given thickness in the Echo Canyon Conglomerate average nearly twice the width of their counterparts in the Richards Mountain Conglomerate (Figures 11, 12 and Table 3). In order to interpret the meaning of this disparity in width:height ratios, we must first understand what process controls the width of fifth-order lithosomes. We interpret this to be a function of the efficiency of the migration of channels across the fan. Essentially, the channels (and it follows, channel complexes) of the Echo Canyon Conglomerate were more effective at sweeping across those alluvial fans than were the channel complexes of the fan at Richards Mountain. Many conditions, both allogenic and autogenic, can influence the rate of channel migration in fluvial or alluvial systems. The number of factors that can play a role make determining the dominant processes and factors controlling a complex and challenging task. We examine below the likelihood of four different major processes/conditions having contributed to the observed difference in width:height ratios between the study areas: (1) the nature of the source material and resulting sediment on the fan, (2) the position (proximal vs. distal) on the fan at which dimensions are measured, (3) tectonic influences such as rates of uplift and subsidence, and (4) climatic controls on efficiency of channel migration.
Discussion of Key Facies
Correctly interpreting environment of deposition and identifying lithosomal bounding surfaces in alluvial fan deposits requires recognition of key facies and the spatial relationships between facies. Key facies in each study area (Figure 4) are similar, but important differences exist. Key facies of the Richards Mountain Conglomerate include clast-supported, structureless to poorly stratified or imbricated cobble and boulder conglomerates and fine-grained sandstones with ripple and/or trough-cross-stratification interpreted as channel deposits on a streamflow-dominated alluvial fan. Debris flow deposits are conspicuously absent. This raises questions about whether the Richards Mountain Conglomerate should be classified as an alluvial fan at all rather than just a coarse braid plain deposit (Crews and Ethridge, 1993). However, given the large clast size (up to 1.5 m), the plano-convex cross-sectional shape of the deposit, and the rapid disappearance of coarse material basinward (pinching out from a maximum thickness of 750 m over just a few km) we feel it is still appropriate to characterize this deposit as an alluvial fan in agreement with previous interpretations (Crews and Ethridge, 1993). The sandstones frequently exhibit mottled red and yellow colors, rhizoliths, and a structureless texture. These are interpreted as sandy channel deposits that have undergone early pedogenesis. Structureless, mottled mudstones are also common and are interpreted as mature paleosols.
In the Echo Canyon Conglomerate clast-supported cobble conglomerates are the most common facies. Some of the conglomerate beds in Echo Canyon show imbrication and/or stratification and likely represent stream-flow deposits. However, most are structureless and are interpreted as channel deposits probably emplaced in high-intensity, short-duration flows or possibly as debris flows. Rare beds of clast-supported, angular pebble to cobble conglomerate grading upward into matrix-supported angular pebble conglomerate also exist and are interpreted as deposits from debris-flows or highly concentrated flows due to their matrix-support and highly angular clasts. Structureless red or mottled mudstones with rhizoliths are common (though not as common as at Richards Mountain) and are interpreted as paleosols. Facies in both study areas follow a consistent pattern of spatial organization (Figures 5, 8, 9). One or more beds of channel-fill conglomerates are overlain by finer-grained intervals of thin-bedded or structureless sandstones and mudstones that represent sheet-flow deposits and pedogenesis on inactive fan segments. Overlying conglomerates representing renewed channel deposition scour into the fine-grained paleosol caps (which are frequently completely removed by the channel scour). The erosional surface between the fine-grained caps and the overlying conglomerates represents the bounding surface between fifth-order lithosomes.
Significance of Small- and Large-Scale Alluvial Architecture
Small-scale alluvial architecture (first- to fourth-order lithosomes; Figure 1 and Table 1) in these ancient alluvial fans is probably most important in helping to reveal how autogenic processes were operating during deposition. While we did not examine the architecture of these smaller features in detail for this study, in-depth examination could yield further insight into paleoclimate (specifically precipitation) through examination of flow conditions. Large-scale (sixth- and seventh-order) lithosomes were also not analyzed in detail, but they do provide some important insight into the general tectonic histories of these synorogenic alluvial fans. For example, the synclinal structure of the Echo Canyon Conglomerate along with its erosional truncation in an angular unconformity with the overlying Hams Fork Conglomerate of the Evanston Formation are a clear record of its incorporation into the wedge-top of the Sevier thrust belt. By comparison, the Richards Mountain Conglomerate lacks any of this post-depositional deformation and truncation, indicating it existed only within the foredeep of the Uinta Mountain uplift.
Possible Causes of Variability in Width:Height Ratios of Fifth-Order Lithosomes
Position on Fan, Grain Size, and Source Area Lithology
Alluvial architecture will change downstream based on the interactions between basin geometry, sediment supply, subsidence rate and avulsion type/frequency (Heller and Paola, 1996; Allen et al., 2013). The downstream position on an alluvial fan at which dimensions of channel complexes are exposed (Table 2) will therefore likely affect what the observed width:height ratios of those channel complexes are. Heller and Paola (1996) used numerical models to demonstrate the wide range of possible changes in downstream alluvial architecture based on the interaction of these factors. Based on their work and assuming typical foreland basin geometries (greatest accommodation toward the hinterland) we would expect to see fifth-order lithosomes thinning and stacking more densely in our study areas as accommodation decreases. Furthermore, assuming channels remain relatively straight as is common on alluvial fans, the rate of channel migration should increase downfan, since a small adjustment in channel trajectory at the fan apex would translate to a much larger adjustment in position several kilometers downstream. Both effects should combine to produce a larger width:height ratio of fifth-order lithosomes in the more distal portions of these alluvial fans.
Additionally, the decrease in grain-size that occurs between proximal and distal portions of alluvial fans could impact channel mobility and alluvial architecture. Several studies (e.g., Nanson and Hickin, 1986; Constantine et al., 2008) have demonstrated that grain-size of channel bank material is tied to bank erodibility and, thus, channel migration rates. In general, these studies have found that erodibility and migration rates increase with decreasing grain size down to silt-sized material after which cohesion in clay-sized particles decreases erodibility and channel migration. It does seem plausible that downstream position of the outcrop exposures in the study areas could account for at least part of the increase in width:height ratios observed in the Echo Canyon Conglomerate relative to the Richards Mountain Conglomerate as a result of changes in either accommodation or grain size. Maximum clast sizes at Richards Mountain (over 100 cm) are considerably greater than those in Echo Canyon (about 40 cm; Supplementary Figure S1). This may suggest that the Richards Mountain outcrops represent more proximal deposits than Echo Canyon, though there are many other factors that could be affecting grain size, such as lithology of the sediment source area or length of sediment routing system (e.g., Blair, 1999; Michael et al., 2014; D’Arcy et al., 2017). For example, a source area dominated by poorly resistant mudstones should tend toward finer-grained sediment than a source area dominated by resistant limestones and quartzites. In the case of these two study areas, source lithology is unlikely to be a strong control on the observed differences in maximum grain size. Detailed clast composition data is available for both the Echo Canyon Conglomerate (Crawford, 1979; DeCelles, 1988, 1994) and the Richards Mountain Conglomerate (Crews, 1985; Crews and Ethridge, 1993). Both alluvial fans were sourced from equivalent stratigraphic sections and contain resistant Mesozoic and Paleozoic sandstones and limestones and Proterozoic quartzites. It seems more likely then that differences in grain size between the two fans are due to other factors, including downfan position, size and gradient of the fan, or distance of the fan apex from the source area. Regardless of the exact control on clast size, finer-grained conglomerates could have allowed for more erodible channel banks on the Echo Canyon Fan and, therefore, more mobile channels and higher width:height ratios for fifth-order lithosomes.
There are problems, however, with using fan position alone to explain the observed differences in alluvial architecture. If the major differences in architecture between Echo Canyon and Richards Mountain are due primarily to fan position, we would expect to see comparable differences between proximal and distal sediments within each individual fan. These relationships are not observed, however. The largest width:height ratios observed at Richards Mountain actually occurred in the axial, most proximal portion of the fan where clast sizes are at a maximum. Sandy channel complexes on the margins of the fan had smaller width:height ratios than some of these more proximal channel complexes, opposite of what would have been expected. At Echo Canyon, no clear differences in architectural style were evident in a downstream direction. Due to the lack of a clear relationship between fan position and architectural style, even within the individual fans, we also believe that fan position is not an adequate explanation for the significant differences observed between width:height ratios of fifth-order lithosomes in the two study areas, though it cannot be ruled out entirely.
Tectonics
Alluvial fans require high relief source terrains in order to form and so by necessity exist in intimate association with areas of active tectonism. Tectonic conditions in turn have the potential to exert a strong control on alluvial architectural style. Changes in architecture may result from different basin geometries, variable rates of uplift and/or changes in the balance between sediment supply and rate of creation of accommodation via basin subsidence (Heller and Paola, 1996; Allen et al., 2013). Both the Uinta Uplift and the Sevier Thrust Belt are the result of compressional tectonics. The Uinta Uplift was produced by “thick-skinned” Laramide-style deformation (basement-cored uplifts) while the Sevier Thrust Belt resulted from “thin-skinned” deformation mostly involving sedimentary cover rocks. Despite these differences in structural style, both source terrains were tectonically active during fan deposition and basin geometries were not significantly different (i.e., both were deposited in flexural foredeeps), removing these major factors in the different architectural styles of the two study areas.
The balance between sediment supply and subsidence has been recognized by many workers (e.g., Leeder, 1978; Knox, 1983; Heller and Paola, 1996; Allen et al., 2013) as a controlling factor on alluvial and fluvial architecture. Assuming sediment supply is constant, increased subsidence rates should allow for more effective trapping of sediment and lead to greater rates of aggradation. Increased aggradation will in turn increase the preservation potential of fine-grained overbank deposits and lead to more isolated channel bodies (Leeder, 1978; Knox, 1983). Alternatively, reduced rates of subsidence and aggradation provide relatively more time for lateral migration of channels while also allowing for increased winnowing and bypass of fine-grained sediments. So, for a given thickness of sediment (all else being held equal), the active channel on an alluvial fan should be able to migrate across a greater portion of the fan and remove more of the fine material (including paleosol caps on fifth-order lithosomes) from the underlying fan surface in a slowly subsiding basin than it could in a rapidly subsiding and aggrading basin.
With this concept in mind, tectonically controlled differences in subsidence rates seem like an attractive way to explain the differences in architectural style between the Echo Canyon and Richards Mountain Conglomerates. If rates of subsidence and sediment accumulation were greater during deposition of the Richards Mountain Conglomerate than at Echo Canyon, it could nicely explain the greater preservation of paleosols and other fine-grained material at Richards Mountain and the increased effectiveness of lateral channel migration at Echo Canyon. However, when subsidence rates at both study areas are calculated from existing subsidence curves (Palmer, 1983; Crews and Ethridge, 1993; DeCelles, 1994), it appears that the Richards Mountain Conglomerate was not accumulating significantly (if at all) faster than the Echo Canyon Conglomerate (approximately 0.1–0.25 mm/year at Richards Mountain vs. 0.18–0.23 mm/year at Echo Canyon). Admittedly, these are very rough estimates given the lack of high-resolution age data in the study areas. The minimal difference in estimated subsidence rates between the study areas does suggest, however, that tectonic controls alone are unlikely to explain the difference in architectural styles we observe. Better resolution for depositional ages on the two fans would aid significantly in resolving to exactly what degree tectonics may have played a role in shaping architectural style.
Climate
Variation in climatic factors, including mean annual precipitation and seasonality of precipitation, can function as strong controls on stratigraphic architecture and lateral channel migration. Review of previous work on the paleoclimate of our study areas (e.g., Crawford, 1979; Crews, 1985; Fricke et al., 2009; Foreman et al., 2012) indicates that the most significant difference in the two climates was probably their amount and style of precipitation. Precipitation strongly influences vegetation cover and sediment supply and directly controls discharge, all of which may affect architectural style. Greater mean annual precipitation (i.e., a wetter climate) supports greater vegetation cover. Vegetation exerts a primary control on channel migration by increasing the cohesiveness of channel margins, which decreases erosion and lateral migration. Increased vegetation also secondarily stabilizes channels by limiting erosion in the catchment area and thereby decreasing sediment flux (Smith, 1994; Gran and Paola, 2001; Giardino and Lee, 2011; Wickert et al., 2013). High sediment flux destabilizes channels by overwhelming the transport capacity of the stream and causing frequent, small avulsions and increased rates of lateral migration (Salcher et al., 2010; Wickert et al., 2013; Legg and Olson, 2014), a phenomenon that is readily observable in sediment-choked braided rivers (Ashworth et al., 2004).
Interestingly, sediment flux does not increase linearly with increasing precipitation. Instead, sediment flux peaks in semiarid-subhumid climates at around 30 cm mean annual precipitation. Sediment supply is limited by vegetation cover in wetter climates and by lack of runoff in dryer climates (Langbein and Schtjmm, 1958; Smith, 1994). Increased variability in precipitation also increases sediment flux; both field studies and numerical models have shown spikes in sediment flux where precipitation and discharge are highly variable and/or seasonal (e.g., Smith, 1994; Cecil and Terence Edgar, 2003; Pelletier et al., unpublished). Strong seasonality and variability in precipitation, such as in monsoonal climates, is therefore another contributor to channel mobility because it destabilizes channels through increased sediment flux as well through large-scale flood events (Starkel, 1979). This may be observed occurring on fluvial megafans in monsoonal climates (Leier et al., 2005), which exhibit some of the most mobile channels on the planet. The opposite has also been documented after the construction of reservoirs; the river channels downstream of new reservoirs usually experience decreased lateral migration due to regulation of flow and trapping of sediment upstream (Shields et al., 2000; Larsen et al., 2006).
Several lines of evidence support the interpretation that the Echo Canyon Conglomerate was deposited in a more seasonal climate than was the Richards Mountain Conglomerate and thus may have had greater rates of lateral channel migration due to increased sediment flux, more variable discharge, and decreased vegetation. While both fans were streamflow dominated (Crawford, 1979; Crews and Ethridge, 1993; DeCelles, 1994), debris flows are present only at Echo Canyon (Crawford, 1979; DeCelles, 1994) and are conspicuously lacking at Richards Mountain (Crews, 1985; Crews and Ethridge, 1993). High-intensity or long-duration precipitation is generally required to form a debris flow (Caine, 1980), and the rainfall threshold for debris flow initiation is generally higher in areas with year-round wet climates (Wilson, 2000; Giannecchini, 2006). Debris flows are therefore favored in areas with highly variable precipitation and commonly occur after infrequent but strong and brief storms where only minimal infiltration occurs (Starkel, 1979; Pierson, 1980; Radbruch-Hall et al., 1982). Debris flows at Echo Canyon therefore suggest that these conditions were at least occurring on occasion. Crawford (1979) also interpreted the common occurrence of soft sediment deformation features and extremely poorly sorted and somewhat chaotic conglomerates in Echo Canyon as an indicator that much of that fan was deposited by short, intense flows. Furthermore, climate models, stable isotope data, and the fossil record strongly suggest that a North American monsoon, fueled by moisture from the Western Interior Seaway, was active on the eastern flank of the Sevier Orogenic belt (i.e., at the location of the Echo Canyon fan) during the late Cretaceous (Fricke et al., 2009). No such monsoonal conditions are known to have existed in western North America during the time that the Richards Mountain Conglomerate was being deposited. Most evidence suggests that the Richards Mountain fan was active under year-round wet conditions. Again, debris flows have not been observed on this fan, signs of pedogenic alteration are pervasive throughout the fan (even in sandy and gravelly channel deposits), and signs of vegetation (including rhizoliths, woody material, and leaves that probably came from a jungle) are abundant (Crews, 1985; Crews and Ethridge, 1993; Figures 4, 5). The interfingering of the Richards Mountain Conglomerate downdip with the lacustrine Green River Formation and its transition to a fan delta at the top of the section also show that conditions were wet enough (at least at times) to support large lakes. All these characteristics suggest a relatively stable and wet climate that would have contributed to channel stability through steady flow, dense vegetation, and reduced sediment flux. Monsoonal conditions and increased sediment fluxes are speculated to have occurred during the PETM and the PETM appears to have influenced stratigraphic architecture of fluvial deposits (Foreman et al., 2012) to produce a similar architectural style to that observed in Echo Canyon. It is possible that the Richards Mountain Conglomerate avoided these effects because it was deposited several million years after the peak of the PETM.
In summary, lithologic evidence and indications of a late Cretaceous North American monsoon support the interpretation of the Echo Canyon Conglomerate as having been deposited under a strongly seasonal, subhumid climate, while the Richards Mountain Conglomerate appears to have been deposited in a relatively more stable and humid climate. This would have led to relatively higher rates of lateral channel migration on the Echo Canyon fan due to flashier discharge, higher sediment flux, and possibly sparser vegetation cover. It is also possible that the increased sediment supply at Echo Canyon could have overwhelmed creation of accommodation and allowed for increased winnowing of overbank deposits and paleosols and larger width:height ratios of fifth-order lithosomes as discussed previously.
Potential Sources of Error
Sources of error in this study were primarily minor flaws in the photogrammetric models including misalignments of up to several meters between model chunks (caused by changing lighting conditions between photos) or accidental incomplete coverage of some outcrops. Further error was likely introduced by using general paleoflow directions to correct for apparent lithosome widths (Table 3 and Supplementary Table S1). While using general paleoflow directions certainly saves an immense amount of time in the field (one of the main advantages of the method we employ in this study), it does introduce a potentially significant source of error depending on the variability of individual paleocurrent directions. These challenges, along with difficulty in correlation of some fifth-order lithosomes due to faulting, erosion, or slope cover meant that measurements of fifth-order lithosome widths in particular were subject to errors of several meters (due to lack of exposure of thin beds on the margins of lithosomes). This was compensated for by measuring as many lithosomes as possible from all parts of the study areas with adequate exposure. We should note again though, that width:height ratios of fifth-order lithosomes (particularly at Echo Canyon) are minimum estimates. Fortunately, because underestimation of width:height ratios are probably greater at Echo Canyon, this should not change our primary observation that fifth-order lithosomes there have significantly larger width:height ratios as compared to those observed on Richards Mountain.
Lack of data is, of course, always of concern and this study was no exception. Downfan position, subsidence rates and local climate all had to be roughly estimated due to limited outcrop extent and the lack of detailed age and geochemical paleoclimate data at our particular study sites. This introduces uncertainty into our discussion of possible contributing factors to variations in alluvial architecture, but again the evidence that does exist is overwhelmingly in support of climate as the main driver of architectural style.
Implications
Given the evidence to support increased channel mobility through a dryer and more seasonal climate at Echo Canyon than found at Richards Mountain (and the lack of strong evidence to explain increased lateral migration due to source material lithology, position on the fan, or subsidence rates) we propose that the differences observed in width:height ratios of fifth-order lithosomes between the study areas are primarily due to differences in climate. Other studies (e.g., Smith, 1994; Ritter et al., 1995; D’Arcy et al., 2017) have reached similar conclusions about climate being the dominant driver behind changes in alluvial fan development. Our interpretation has two major implications. First, providing other allogenic and autogenic factors can be reasonably eliminated as causal mechanisms, stratigraphic architecture of fifth-order lithosomes in alluvial fans may provide insight into paleoclimate, specifically seasonality. Secondly, as anthropogenic climate change continues to affect modern and future climates, we might expect to observe increased lateral migration of channel complexes on alluvial fans in regions where precipitation is becoming more variable. Climate studies predict that large portions of the globe (including areas such as California, northern Venezuela, etc. already at risk from debris flow events and other alluvial processes) will experience more extreme precipitation events in coming decades (e.g., Kunkel et al., 2013), meaning that this effect has the potential to be widespread. While this statement assumes that other factors are held constant (an admittedly questionable assumption given the great uncertainties associated with climate change) it may still hold true in a general sense. The time scale over which fan processes may respond to changing climate is somewhat uncertain due to lack of high-resolution temporal data from alluvial fan deposits; however, at the least this insight allows for increased awareness of the potential for future changes and increases in geological hazards associated with alluvial fan dynamics.
Conclusion
We find that the width:height ratios of fifth-order lithosomes in the alluvial fan deposits of the Cretaceous Echo Canyon Conglomerate are nearly twice those of their counterparts in the Eocene Richards Mountain Conglomerate. This indicates that some mechanism was allowing the channel complexes on the Echo Canyon fan to more effectively sweep across the alluvial fan surface. Most evidence points toward climate being the main driver behind this enhanced lateral migration, though other factors such as subsidence rates may have played minor roles. A more seasonal and warmer climate during the deposition of the Echo Canyon Conglomerate in the late Cretaceous allowed channel to migrate more effectively across the fan by destabilizing channels through increased sediment flux, flood events, and possibly decreased vegetation as compared to the wetter, more consistent climate during Richards Mountain deposition.
Our results imply that the architectural styles (specifically with/height ratios of fifth-order lithosomes) of ancient alluvial fans may be cautiously used to provide insight into allogenic controls related to paleoclimate where sufficient evidence exists to eliminate other causal mechanisms. They also suggest that in regions where anthropogenic climate change results in more variable patterns of precipitation, alluvial fans may show decreased channel stability and, hence, greater risk to human activity and development on alluvial fans.
Data Availability Statement
All datasets generated for this study are included in the article/Supplementary Material.
Author Contributions
BC and SM contributed to the conception and design of the study. BC and PD provided background information, an introduction to the study areas, and mentoring, reviewed and provided feedback and editing on the manuscript. SM collected field data, built and analyzed photogrammetric models, performed statistical analysis, drafted figures and tables, and wrote the manuscript. All authors contributed to the article and approved the submitted version.
Funding
Funding for this project was provided to SM by the 2018 AAPG Grants-in-Aid Program (Alexander and Geraldine Wanek Memorial Grant) and the University of Arizona Department of Geosciences.
Conflict of Interest
The authors declare that the research was conducted in the absence of any commercial or financial relationships that could be construed as a potential conflict of interest.
Acknowledgments
We are grateful to those at the AAPG and the University of Arizona who provided financial assistance for this project, to the faculty in the University of Arizona Department of Geosciences for their input and suggestions, and to Samantha Meek for her assistance with field work.
Supplementary Material
The Supplementary Material for this article can be found online at: https://www.frontiersin.org/articles/10.3389/feart.2020.00215/full#supplementary-material
References
Allen, P. A., Armitage, J. J., Carter, A., Duller, R. A., Michael, N. A., Sinclair, H. D., et al. (2013). The Qs problem: sediment volumetric balance of proximal foreland basin systems. Sedimentology 60, 102–130. doi: 10.1111/sed.12015
Armitage, J. J., Duller, R. A., Whittaker, A. C., and Allen, P. A. (2011). Transformation of tectonic and climatic signals from source to sedimentary archive. Nat. Geosci. 4, 231–235. doi: 10.1038/ngeo1087
Ashworth, P. J., Best, J. L., and Jones, M. (2004). Relationship between sediment supply and avulsion frequency in braided rivers. Geology 32, 21–24. doi: 10.1130/G19919.1
Bemis, S. P., Micklethwaite, S., Turner, D., James, M. R., Akciz, S., Thiele, T., et al. (2014). Ground-based and UAV-based photogrammetry: a multi-scale, high-resolution mapping tool for structural geology and paleoseismology. J. Struct. Geol. 69, 163–178. doi: 10.1016/j.jsg.2014.10.007
Bettis, E. A. III (2003). Patterns in holocene colluvium and alluvial fans across the prairie-forest transition in the midcontinent USA. Geoarcheol. An Int. J. 18, 779–797. doi: 10.1002/gea.10087
Blair, T. C. (1999). Cause of dominance by sheetflood vs. debris-flow processes on two adjoining alluvial fans, Death Valley, California. Sedimentology 46, 1015–1028. doi: 10.1046/j.1365-3091.1999.00261.x
Blair, T. C., and McPherson, J. G. (2009). “Processes and forms of alluvial fans,” in Geomorphology of Desert Environments, eds A. J. Parsons and A. D. Abrahams (Berlin: Springer Science+Business Media), 413–466.
Bull, W. B. (1964). Alluvial fans and near-surface subsidence in western Fresno County, California. Paper Presented at United States Geological Survey Professional Paper 437-A. Reston: United States Geological Survey.
Bull, W. B. (1977). The alluvial-fan environment. Prog. Phys. Geogr. Earth Environ. 1, 222–270. doi: 10.1177/030913337700100202
Burgener, L., Hyland, E., Huntington, K. W., Kelson, J. R., and Sewall, J. O. (2019). Revisiting the equable climate problem during the Late Cretaceous greenhouse using paleosol carbonate clumped isotope temperatures from the Campanian of the Western Interior Basin, USA. Palaeogeogr. Palaeoclimatol. Palaeoecol. 516, 244–267. doi: 10.1016/j.palaeo.2018.12.004
Caine, N. (1980). The rainfall intensity:duration control of shallow landslides and debris flows. Geogr. Ann. Ser. A, Phys. Geogr. 62, 23–27. doi: 10.1080/04353676.1980.11879996
Carrivick, J. L., Smith, M. W., and Quincey, D. J. (2016). Structure from Motion in the Geosciences. Chichester, UK: John Wiley & Sons, Ltd.
Cecil, C. B., and Terence Edgar, N. (2003). Climate Controls on Stratigraphy: SEPM Special Publication No.77. Available online at: http://www.sepm. org.downloadedfromhttps://pubs.geoscienceworld.org/books/chapter-pdf/379 8282/9781565762145_frontmatter.pdf (accessed February 4, 2019).
Clarke, L., Quine, T. A., and Nicholas, A. (2010). An experimental investigation of autogenic behaviour during alluvial fan evolution. Geomorphology 115, 278–285. doi: 10.1016/j.geomorph.2009.06.033
Constantine, C. R., Dunne, T., and Hanson, G. J. (2008). Examining the physical meaning of the bank erosion coefficient used in meander migration modeling. Geomorphology 106, 242–252. doi: 10.1016/j.geomorph.2008.11.002
Crawford, G. A., and Dott, R. H. (1979). Alluvial-Fan Deposition and Tectonic Significance of Two Late Cretaceous-Paleocene Conglomerates in North-Central Utah Thrust Belt. Available online at: http://archives.datapages.com.erl.lib.byu.edu/data/bulletns/1977-79/images/pg/00630003/0400/04370.pdf (accessed April 11, 2018).
Crawford, K. A. (1979). Sedimentology and Tectonic Significance of the Late Cretaceous-Paleocene Echo Canyon and Evanston Synorogenic Conglomerates of the North-Central Utah Thrust Belt. Madison, WI: University of Wisconsin.
Crews, S. G. (1985). Response of Basin-Margin Depositional Sytems to Laramide Uplift of the Northeastern Uinta Mountains, M.S. thesis, Colorado State University, Colorado.
Crews, S. G., and Ethridge, F. G. (1993). Laramide tectonics and humid alluvial fan sedimentation, NE Uinta Uplift, Utah and Wyoming. J. Sediment. Petrol. 63, 420–436.
Culbertson, W. C. (1969). Facies Changes in the Eocene Rocks in the Southeastern Part of the Green River Basin, Wyoming. Available online at: http://archives.datapages.com.erl.lib.byu.edu/data/uga/data/016/016001/pdfs/205.pdf (accessed April 11, 2018).
Dade, B. W., and Verdeyen, M. E. (2007). Tectonic and climatic controls of alluvial-fan size and source-catchment relief. J. Geol. Soc. Lond. 164, 353–358. doi: 10.1144/0016-76492006-039
D’Arcy, M., Whittaker, A. C., and Roda-Boluda, D. C. (2017). Measuring alluvial fan sensitivity to past climate changes using a self-similarity approach to grain-size fining, Death Valley, California. Sedimentology 64, 388–424. doi: 10.1007/978-1-4614-7495-1_23
DeCelles, P. G. (1988). Lithologic provenance modeling applied to the Late Cretaceous synorogenic Echo Canyon Conglomerate, Utah: a case of multiple source areas. Geology 16, 1039–1043.
DeCelles, P. G. (1994). Late cretaceous-paleocene synorogenic sedimentation and kinematic history of the sevier thrust belt, Northeast Utah and Southwest Wyoming. Geol. Soc. Am. Bull. 106, 32–56. doi: 10.1130/0016-7606(1994)106<0032:lcpssa>2.3.co;2
DeCelles, P. G., Gray, M. B., Ridgeway, K. D., Cole, R. B., Pivnik, D. A., Pequera, N., et al. (1991). Controls on synorogenic alluvial-fan architecture, beartooth conglomerate (Palaeocene), wyoming and montana. Sedimentology 38, 567–590. doi: 10.1111/j.1365-3091.1991.tb01009.x
Dorn, R. I. (2009). “The role of climatic change in alluvial fan development the persistence of climatic change in alluvial-fan studies,” in Geomorphology of Desert Environments, eds A. J. Parsons and A. D. Abrahams (Cham: Springer Science+Business Media), 723–742. doi: 10.1007/978-1-4020-5719-9_24
Fabuel-Perez (2009). A New Approach for Outcrop Characterization and Geostatistical Analysis of a Low-Sinuosity Fluvial -Dominated Succession Using Digital Outcrop Models. Available online at: https://watermark-silverchair-com.ezproxy1.library.arizona.edu/bltn08102.pdf (accessed February 11, 2019).
Fabuel-Perez, I., Hodgetts, D., and Redfern, J. (2010). Integration of digital outcrop models (DOMs) and high resolution sedimentology - workflow and implications for geological modelling: oukaimeden sandstone formation, High Atlas (Morocco). Pet. Geosci. 16, 133–154. doi: 10.1144/1354-079309-820
Foreman, B. Z., Heller, P. L., and Clementz, M. T. (2012). Fluvial response to abrupt global warming at the Palaeocene/Eocene boundary. Nature 491, 92–95. doi: 10.1038/nature11513
Franczyk, K. J., Fouch, T. D., Johnson, R. C., Molenaar, C. M., and Cobban, W. A. (1992). “Cretaceous and tertiary paleogeographic reconstructions for the uinta-piceance basin,” in Proceedings of the U.S. Geological Survey Bulletin 1787: Evolution of Sedimentary Basins - Uinta and Piceance Basins. Reston: United States Geological Survey.
Fricke, H. C., Foreman, B. Z., and Sewall, J. O. (2009). Integrated climate model-oxygen isotope evidence for a North American monsoon during the Late Cretaceous. Earth Planet. Sci. Lett. 289, 11–21. doi: 10.1016/j.epsl.2009.10.018
García-Sellés, D., Granado, P., Gratacos, O., Carrera, N., and Arbues, P. (2014). “Capture and geological data extraction: tools for a better analysis and digital modelling,” in Proceedings of the Vertical Geology Conference. Lausanne: University of Lausanne.
Giannecchini, R. (2006). Natural Hazards and Earth System Sciences Relationship between Rainfall and Shallow Landslides in the Southern Apuan Alps (Italy). Available online at: http://www.nat-hazards-earth-syst-sci.net/6/357/2006/ (accessed June 29, 2019).
Giardino, J. R., and Lee, A. A. (2011). Rates of Channel Migration on the Brazos River. Available online at: http://www.twdb.texas.gov/publications/reports/contracted_reports/doc/0904830898_Brazos.pdf (accessed July 20, 2019).
Google Earth (2019). Satellite Imagery of Echo Canyon, Utah and Richards Mountain, Wyoming. Images Acquired by Landsat/Copernicus, Maxar Technologies, USDA Farm Service Agency, and State of Utah. Available online at: earth.google.com/web/ (accessed December 16, 2019).
Gran, K., and Paola, C. (2001). Riparian vegetation controls on braided stream dynamics. Water Resour. Res. 37, 3275–3283. doi: 10.1029/2000WR000203
Gunster, N., and Skowronek, A. (2001). Sediment-soil sequences in the Granada Basin as evidence for long-and short-term climatic changes during the Pliocene and Quaternary in the Western Mediterranean. Quat. Int. 78, 17–32. doi: 10.1016/s1040-6182(00)00112-9
Hajek, E. A., Heller, P. L., and Schur, E. L. (2012). Field test of autogenic control on alluvial stratrigraphy (Ferris Formation, Upper Cretaceous - Paleogene, Wyoming). Geol. Soc. Am. Bull. 124, 1898–1912. doi: 10.1130/B30526.1
Harvey, A. M., Foster, G., Hannam, J., and Mather, A. E. (2003). The Tabernas alluvial fan and lake system, southeast Spain: applications of mineral magnetic and pedogenic iron oxide analyses towards clarifying the Quaternary sediment sequences. Geomorphology 50, 151–171. doi: 10.1016/s0169-555x(02)00212-x
Harvey, A. M., Mather, A. E., and Stokes, M. (2005). Alluvial Fans: Geomorphology, Sedimentology, Dynamics (Geological Society Special Publication No. 251). Available online at: https://sudartomas.files.wordpress.com/2012/11/alluvialfans_geomorphologysedimentologydynamics.pdf (accessed April 12, 2018).
Heller, P. L., and Paola, C. (1996). Downstream changes in alluvial architecture: an exploration of controls on channel-stacking patterns. J. Sediment. Res. 66, 297–306.
Hofmann, M. H., Wroblewski, A., and Boyd, R. (2011). Mechanisms controlling the clustering of fluvial channels and the compensational stacking of cluster belts. J. Sediment. Res. 81, 670–685. doi: 10.2110/jsr.2011.54
Jacobson, S. R., and Nichols, D. J. (1982). Palynological dating of syntectonic units in the Utah-Wyoming Thrust belt: the evanston formation, echo canyon conglomerate, and little muddy creek conglomerate. Geol. Stud. Cordilleran Thrust Belt 2, 735–750.
Klinger, Y., Avouac, J. P., Bourles, D., and Tisnerat, N. (2003). Alluvial deposition and lake-level fluctuations forced by Late Quaternary climate change: the Dead Sea case example. Sediment. Geol. 162, 119–139. doi: 10.1016/j.sedgeo.2003.07.001
Knox, J. C. (1983). “Responses of river systems to Holocene climates,” in Late Quaternary Environments of the United States, Vol. 2, the Holocene, eds H. E. Wright and S. C. Porter (Minneapolis: University of Minnesota Press), 26–41. doi: 10.5749/j.ctttt09b.8
Kunkel, K. E., Karl, T. R., Easterling, D. R., Redmond, K., Young, J., Yin, X., et al. (2013). Probable maximum precipitation and climate change. Geophys. Res. Lett. 40, 1402–1408. doi: 10.1002/grl.50334
Lamerson, P. R. (1982). The Fossil Basin and Its Relationship to the Absaroka Thrust System, Wyoming and Utah. Denver: Rocky Mountain Association of Geologists, 279–340.
Langbein, W. B., and Schtjmm, S. A. (1958). Yield of sediment in relation to mean annual precipitation. Transactions 39, 1076–1084.
Larsen, E. W., Fremier, A. K., and Girvetz, E. H. (2006). Modeling the effeccts of variable annual flow on river channel meansder migration patterns, Sacramento River, California, USA. J. Am. Water Resour. Assoc. 42, 1063–1075. doi: 10.1111/j.1752-1688.2006.tb04514.x
Larsen, M. C., Wieczorek, G. F., Eaton, L. S., Morgan, B. A., and Torres- Sierra, H. (2001). Venezuelan debris flow and flash flood disaster of 1999 studied. EOS 82, 572–573.
Lecce, S. A. (1990). “The alluvial fan problem,” in Alluvial Fans: A Field Approach, eds A. H. Rachocki and M. Church (Hoboken, NJ: John Wiley & Sons Ltd), 3–24.
Leeder, M. R. (1978). “A quantitative stratigraphic model for alluvium, with special reference to channel deposit density and interconnectedness,” in Fluvial Sedimentology: Canadian Society of Petroluem Geologists Memoir, Vol. 5, ed. A. D. Miall (Calgary, AB: CSPG), 587–596.
Leeder, M. R., Harris, T., and Kirkby, M. J. (1998). Sediment supply and climate change: implications for basin stratigraphy. Basin Res. 10, 7–18. doi: 10.1046/j.1365-2117.1998.00054.x
Legg, N. T., and Olson, P. L. (2014). Channel Migration Processes and Patterns in Western Washington: A Synthesis for Floodplain Management and Restoration. Available online at: https://fortress.wa.gov/ecy/publications/SummaryPages/1406028.html (accessed July 9, 2019).
Leier, A. L., DeCelles, P. G., and Pelletier, J. D. (2005). Mountains, monsoons, and megafans. Geology 33, 289–292. doi: 10.1130/G21228.1
Miall, A. D. (1985). Architectural-element analysis: a new method of facies analysis applied to fluvial deposits. Earth Sci. Rev. 22, 261–308. doi: 10.1016/0012-8252(85)90001-7
Michael, N. A., Whittaker, A. C., Carter, A., and Allen, P. A. (2014). Volumetric budget and grain-size fractionation of a geological sediment routing system: eocene escanilla formation, south-central Pyrenees. Bull. Geol. Soc. Am. 126, 585–599. doi: 10.1130/B30954.1
Miller, K. L., Reitz, M. D., and Jerolmack, D. J. (2014). Generalized sorting profile of alluvial fans. Geophys. Res. Lett. 41, 7191–7199. doi: 10.1002/2014GL060991
Nanson, G. C., and Hickin, E. J. (1986). A statistical analysis of bank erosion and channel migration in western Canada. Geol. Soc. Am. Bull. 97, 497–504.
Neton, M. J., Dorsch, J., Olson, C. D., and Young, S. C. (1994). Architecture and directional scales of heterogeneity in alluvial-fan aquifers. J. Sediment. Res. Sect. B Stratigr. Glob. Stud. 64, 245–257. doi: 10.1306/D4267FA0-2B26-11D7-8648000102C1865D
Nichols, G. J., and Fisher, J. A. (2007). Processes, facies and architecture of fluvial distributary system deposits. Sediment. Geol. 195, 75–90. doi: 10.1016/j.sedgeo.2006.07.004
Painter, C. S., Carrapa, B., Decelles, P. G., Gehrels, G. E., and Thomson, S. N. (2014). Exhumation of the North American Cordillera revealed by multi-dating of Upper Jurassic-Upper Cretaceous foreland basin deposits. Geol. Soc. Am. Bull. 126, 1439–1464. doi: 10.1130/B30999.1
Palmer, A. R. (1983). The decade of north american geology 1983 geologic time scale. Geology 11, 503–504.
Parker, G., Paola, C., Whipple, K. X., and Mohrig, D. (1998). Alluvial fans formed by channelized fluvial and sheet flow. I: theory. J. Hydraul. Eng. 124, 985–995. doi: 10.1061/(asce)0733-9429(1998)124:10(985)
Pierson, T. C. (1980). Erosion and deposition by debris flows at Mt Thomas, North Canterbury, New Zealand. Earth Surf. Process. 5, 227–247. doi: 10.1002/esp.3760050302
Radbruch-Hall, D. H., Colton, R. B., Davies, W. E., Lucchitta, I., Skipp, B. A., and Varnes, D. J. (1982). Landslide overview map of the conterminous United States. Paper Presented at Geological Survey Professional Paper 1183 (Washington, D.C: Geological Survey).
Ritter, J. B., Miller, J. R., Enzel, Y., and Wells, S. G. (1995). Reconciling the roles of tectonism and climate in Quaternary alluvial fan evolution. Geology 23, 245–248.
Roehler, H. W. (1992). Introduction to Greater Green River Basin Geology, Physiography, and History of Investigations. Washington, D.C: Geological Survey, A1–A14.
Romans, B. W., Castelltort, S., Covault, J. A., Fildani, A., and Walsh, J. P. (2016). Environmental signal propagation in sedimentary systems across timescales. Earth Sci. Rev. 153, 7–29. doi: 10.1016/j.earscirev.2015.07.012
Salcher, B. C., Faber, R., and Wagreich, M. (2010). Climate as main factor controlling the sequence development of two Pleistocene alluvial fans in the Vienna Basin (eastern Austria) - A numerical modelling approach. Geomorphology 115, 215–227. doi: 10.1016/j.geomorph.2009.06.030
Shepherd, M. (2009). “Less common reservoir types,” in Oil field production geology: AAPG Memoir 91, ed. M. Shepherd (Tulsa: American Association of Petroleum Geologists), 311–312. doi: 10.1306/13161227m913372
Shields, F. D. Jr., Simon, A., and Steffen, L. J. (2000). Reservoir Effects on Downstream River Channel Migration. Available online at: https://pubag.nal.usda.gov/download/4055/PDF (accessed July 13, 2019).
Shultz, A. W. (1984). Subaerial debris-flow deposition in the Upper Paleozoic Cutler Formation, Western Colorado. J. Sediment. Petrol. 54, 759–772.
Smith, G. A. (1994). Climatic influences on continental deposition during late-stage filling of an extensional basin, southeastern Arizona. Geol. Soc. Am. Bull. 106, 1212–1228. doi: 10.1130/0016-7606(1994)106<1212:ciocdd>2.3.co;2
Smith, M. E., Carroll, A. R., and Singer, B. S. (2008). Synoptic reconstruction of a major ancient lake system: eocene green river formation, Western United States. Bull. Geol. Soc. Am. 120, 54–84. doi: 10.1130/B26073.1
Smith, M. W., Carrivick, J. L., and Quincey, D. J. (2015). Structure from motion photogrammetry in physical geography. Prog. Phys. Geogr. 40, 247–275. doi: 10.1177/0309133315615805
Starkel, L. (1979). The role of extreme meteorological events in the shaping of mountain relief. Geogr. Pol. 41, 13–20.
Straub, K. M., Paola, C., Mohrig, D., Wolinsky, M. A., and George, T. (2009). Compensational stacking of channelized sedimentary deposits. J. Sediment. Res. 79, 673–688. doi: 10.2110/jsr.2009.070
Thrasher, B. L., and Sloan, L. C. (2009). Carbon dioxide and the early Eocene climate of western North America. Geology 37, 807–810. doi: 10.1130/G30090A.1
Törö, B., Pratt, B. R., and Renaut, R. W. (2015). Tectonically Induced Change in Lake Evolution Recorded by Seismites in the Eocene Green River Formation, Wyoming. Terra 27, 218–224. doi: 10.1111/ter.12150
Ventra, D., and Clarke, L. E. (2018). “Geology and geomorphology of alluvial and fluvial fans: current progress and research perspectives,” in Geology and Geomorphology ofAlluvial and Fluvial Fans: Terrestrial and Planetary Perspectives, eds D. Ventra and L. E. Clarke (London: Geology and Geomorphology ofAlluvial and Fluvial Fans: Terrestrial and Planetary Perspectives), 1–21. doi: 10.1144/sp440.16
Whipple, K. X., Parker, G., Paola, C., and Mohrig, D. (1998). Channel dynamics, sediment transport, and the slope of alluvial fans: experimental study. J. Geol. 106, 677–693.
Wickert, A. D., Martin, J. M., Tal, M., Kim, W., Sheets, B., and Paola, C. (2013). River channel lateral mobility: metrics, time scales, and controls. J. Geophys. Res. Earth Surf. 118, 396–412. doi: 10.1029/2012JF002386
Wilson, R. C. (2000). “Climatic variations in rainfall thresholds for debris-flow activity,” in Proceedings of the 1st Plinius Conf. on Mediterranean Storms, eds P. Claps and F. Siccardi (Maratea: United States Geological Survey), 415–424.
Wolfe, J. A. (1980). Tertiary climates and floristic relationships at high latitudes in the northern hemisphere. Palaeogeogr. Palaeoclimatol. Palaeoecol. 30, 313–323. doi: 10.1016/0031-0182(80)90063-2
Keywords: stratigraphic architecture, alluvial fan, allogenic, autogenic, paleoclimate, fan dynamics, Western US
Citation: Meek SR, Carrapa B and DeCelles PG (2020) Recognizing Allogenic Controls on the Stratigraphic Architecture of Ancient Alluvial Fans in the Western US. Front. Earth Sci. 8:215. doi: 10.3389/feart.2020.00215
Received: 16 August 2019; Accepted: 22 May 2020;
Published: 24 June 2020.
Edited by:
Miquel Poyatos Moré, University of Oslo, NorwayReviewed by:
Stuart Clarke, Keele University, United KingdomNicholas Perez, Texas A&M University, United States
Copyright © 2020 Meek, Carrapa and DeCelles. This is an open-access article distributed under the terms of the Creative Commons Attribution License (CC BY). The use, distribution or reproduction in other forums is permitted, provided the original author(s) and the copyright owner(s) are credited and that the original publication in this journal is cited, in accordance with accepted academic practice. No use, distribution or reproduction is permitted which does not comply with these terms.
*Correspondence: Scott R. Meek, c2NvdHRybWVla0BnbWFpbC5jb20=