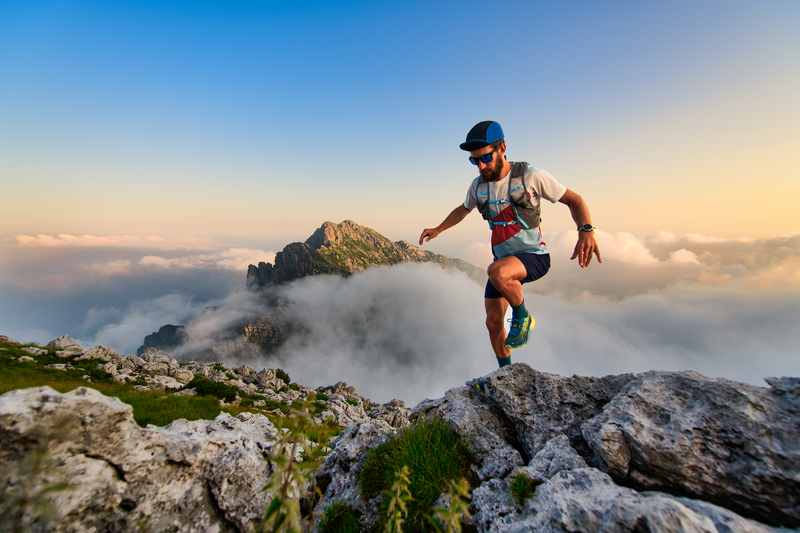
94% of researchers rate our articles as excellent or good
Learn more about the work of our research integrity team to safeguard the quality of each article we publish.
Find out more
REVIEW article
Front. Earth Sci. , 02 June 2020
Sec. Atmospheric Science
Volume 8 - 2020 | https://doi.org/10.3389/feart.2020.00133
This article is part of the Research Topic The Asian Monsoon View all 17 articles
Surface diabatic heating over the Tibetan Plateau (TP) is crucial for the onset and development of Asian summer monsoon (ASM), which is closely connected with the snow melting and freeze–thaw (SM-FT) processes in spring. This study reviews the recent processes about studies on the effects of SM-FT on the climate system over the ASM region. This review has shown that SM-FT in spring plays a dominant role in seasonal and inter-annual variations of surface diabatic heating over TP, which also have a significant relationship with the ASM activity. Moreover, the anomalies of SM-FT over TP significantly affect summer precipitation in Eastern China (EC). SM-FT in spring over TP would be a robust factor in the ASM system. The possible mechanism associated with the impacts of SM-FT on the summer general circulation in East Asia is also discussed. Under the climate change background, variations in regimes of frozen soil and snow have great potential effects on the climate in East Asia and around the globe. However, great uncertainties in the estimation of diabatic heating over TP (especially over the western TP) during spring still confine our understanding about the effects of TP thermal forcing on the ASM activity. It is suggested that improvement in the simulation of the SM-FT process in models is an effective approach for reducing the biases of climate projection in future.
Monsoon is a product of the distribution of ocean, land, and special topography; its onset and development are mainly attributed to the thermal contrast between ocean and land (Meehl, 1994; Webster, 1998). Asian summer monsoon (ASM) dominates the summer precipitation belt in South and East Asia. The thermal and terrain effects of the Tibetan Plateau (TP) are important factors in the ASM climate system (e.g., Ye et al., 1957; Ye and Gao, 1979; Wu and Zhang, 1998; Ye and Wu, 1998; Wang et al., 2003; Duan et al., 2005; Wu et al., 2012a). A distinct symbol of ASM onset is the easterly arriving on the southern sides of TP and South Asia High has a jump over TP in June, and Hadley cell is replaced by the monsoon cell (Ye and Gao, 1979). Since Ye et al. (1957) found that TP is a heat source for atmospheric dynamics, researches on the dynamic and thermal forcing of TP have received much attention in the past decades (e.g., Qian et al., 2003a; Wang et al., 2003; Ding et al., 2008, 2009; Wu et al., 2012a; Duan et al., 2013; Wang C. et al., 2017). Wu et al. (2012a) demonstrated that surface sensible heating over TP forms and maintains the atmospheric circulation in the ASM regions in summer; surface sensible heating over TP acts as a pump in middle troposphere to change the atmospheric circulation around TP (Wu, 1984; Wu and Liu, 2000).
Both statistical analyses and model simulations have shown that strong TP surface diabatic heating (i.e., surface sensible and latent heat fluxes) favors an early monsoon onset and a strong ASM circulation, corresponding to a northward extent of the precipitation belt in East China (e.g., Zhao and Chen, 2001; Wang B. et al., 2008; Liu et al., 2012; Duan et al., 2013). Surface diabatic heating depends on the land surface processes (Wang C. et al., 2008, 2017; Wang et al., 2009b). An evident characteristic of TP land surface is the existence of a large amount of frozen ground and snow. The snow melting and freeze–thaw (SM-FT) process in spring strongly influences the surface sensible and latent heat fluxes in surface energy balance (Chow et al., 2008; Yang and Wang, 2019b). Although there are many available different land surface datasets over TP (Table 1), because of the model uncertainties and the lack of observation data, especially on the shortage of high spatiotemporal soil moisture and land surface heat flux data, some debates still exist on how the thermal effects of TP influence the ASM (Wu et al., 2012b, 2014).
Table 1. Summary of current land surface datasets used in studies about the impacts of soil freeze–thaw and snow-melting.
There is no doubt that the land–atmosphere interaction over TP acts a crucial role in the ASM climate system. However, some issues that still remain should be further investigated. For instance, whether ASM onset is prior to or lags behind the increase of diabatic heating caused by surface status changes (e.g., SM-FT) over TP. In other words, is the changing of surface diabatic heating over TP a result of the establishment of ASM or do the changes of surface diabatic heating promote the development of ASM and its northward advance? How and why do surface diabatic heating anomalies over TP affect the ASM system anomalies? Answering these issues can be beneficial to deeply understand the characteristics of the ASM activity and its mechanism. In this study, we aim to review recent progresses about the relation between surface diabatic heating anomalies over TP as induced by the SM-FT process and the activities of ASM and attempt to answer some issues.
The structure of this study is organized as follows: section “Characteristics of Surface Diabatic Heating and Land Surface Process Over TP” reviews the variations of surface diabatic heating over TP and their relations with the land surface processes (i.e., SM-FT) during spring. Section “Connections Between SM-FT Over TP and ASM Activity” discusses the relationship between the ASM activity (e.g., summer precipitation anomalies in EC) and surface diabatic heating of TP. Section “Possible Mechanisms Associated With the Climatic Effects of SM-FT Process” discusses the possible mechanisms by which SM-FT anomalies over TP influence the summer atmospheric circulation in East Asia. The summary and possible prospects are given in section “Summary and Prospects.”
The TP, which is an immense topography crossing the east–west direction at the middle and the lower latitude in the Northern Hemisphere, not only blocks meridional airflows and the northward transportation of water vapor but also remarkably influences energy and water vapor exchange between the land surface over TP and the surrounding atmosphere. The TP thermal forcing is suggested to dominate the ASM systems, whereas large-scale orographically mechanical forcing is not essential (Wu et al., 2012a).
The estimation of TP diabatic heating is a basic problem. However, the magnitudes of surface sensible heat (SH) flux and latent heat (LH) flux have great discrepancies in different datasets (Cui and Wang, 2009; Shi and Liang, 2014; Xie et al., 2018; Yang Y. et al., 2019). The early works by Ye et al. (1957) and Flohn (1957) suggested that TP is a heat source for the surrounding atmosphere. The results of Flohn (1960) further indicated that SH is dominant prior to the monsoon onset, but LH becomes more important when the rainy season over TP arrives. Ye and Gao (1979) examined the diabatic heating over TP based on surface temperature from in situ observations. They concluded that TP is a heat source during summer and a heat sink in winter. Later studies further confirmed this finding (e.g., Luo and Yanai, 1983; Wu and Zhang, 1998; Duan and Wu, 2005). According to the results of Duan and Wu (2008), the SH reaches its maximum in May and June in the eastern and the western TP, respectively, and the mean SH in the western TP is larger than that in the eastern TP. The latent heat released to the atmosphere by precipitation reaches its maximum in summer (i.e., July) and is dominant in the eastern TP (Figure 1A). The SH presents a decreasing trend over TP (Figure 1B).
Figure 1. Characteristics of diabatic heating over Tibetan Plateau (TP). (A) Climatological mean (1984–2003) of surface sensible heat (SH), latent heat (LH) released to the atmosphere by precipitation, net radiation flux (RC) of the air column, and atmospheric heat source/sink (E) over the central and eastern TP and western TP. (B) Trend of atmospheric heat source/sink and its components. This figure is based on the data from Duan and Wu (2008).
Changes of surface diabatic heating are closely related to the land surface processes (Yang et al., 2002; Wang C. et al., 2008; Wang et al., 2009b), such as the SM-FT process. A study showed that the magnitude of SH trend increases with elevation (Zhu et al., 2018), and the elevation dependency of the SH trend is related to the declining trend of snow depth in the recent decades, which is contributed by the strengthening of the difference between surface and air temperature (Ts-Ta). The soil moisture content over eastern TP shows a decreasing trend (Gao et al., 2015; Meng et al., 2018); this might be related to the freeze–thaw process, lead to the decline of land evapotranspiration (Jung et al., 2010), and affect surface diabatic heating consequently. The vegetation activity anomalies (e.g., NDVI) over TP caused by frozen ground changes can also affect the surface energy balance and result in surface diabatic heating anomalies (Zhang, 2005; Cuo et al., 2013).
Due to the distinct characteristics of the land surface over TP, there being a large amount of frozen soil and extensive snow over TP (Qin et al., 2006; Che et al., 2008; Yang M. et al., 2019), their anomalies and changes must strongly influence the land surface processes (Wang et al., 2009b; Bao et al., 2017; Wang C. et al., 2017). For example, the runoff over TP increased due to snow melting and glacier retreating (Yao et al., 2007; Wang Z. et al., 2017; Gao et al., 2018). The SM-FT has significant impacts on the difference between surface and air temperature (Ts-Ta) before the onset of the East Asian summer monsoon (EASM) (Wang and Cui, 2011), which further leads to the surface diabatic heating anomalies. Studies showed that the energy and water exchanges between atmosphere and land are different between soil frozen and unfrozen periods (Wang and Shang, 2007; Wang et al., 2009b; Wang and Cui, 2011; Ge et al., 2016; Li et al., 2018). For example, the simulation conducted by Wang C. et al. (2008) over the western TP showed that soil water content increases with soil thawing (the LH increases), which has a considerable magnitude with SH in spring. The results from observations further confirmed this feature (Ge et al., 2016).
A distinct feature of the soil freeze–thaw (FT) process is the soil water phase change, which is distinguished from the unfrozen soil. As the soils freeze and their liquid water content decreases, a strong gradient in the matric potential develops, which drives the water toward the freezing front (Zhang et al., 2007; Swenson et al., 2012); while the soil is completely frozen, a small amount of soil liquid water, called “supercooled water,” exists (Niu and Yang, 2007), with the soil thawing rapidly in spring as the soil moisture increases. A recent study from observations and model simulations over TP suggested that the soil FT process has a water storage effect. The storage index (SI), which is defined as the ratio between soil moisture after soil thawing and soil moisture before soil freezing, is used to quantify the water storage capability of soil during the FT process. It can reach up to 0.95 over TP (Yang and Wang, 2019b). Missing the soil FT process could result in drier soil (the loss of soil moisture is about 10%) because of the enhanced evaporation in the after-thaw (AT) period (i.e., spring). The land surface processes (i.e., land–atmosphere energy exchange) in cases of frozen soil and unfrozen soil are remarkably different. Variations of soil temperature and soil moisture further influence the surface diabatic heating status. A model simulation showed that, without the FT process, the mean surface latent heat flux and surface sensible heat flux over the whole TP can decrease by −1.07 W m–2 and increased by 4.72 W m–2, respectively (Figure 2). Overall, the influence of the FT process on the soil moisture in spring is achieved through the water storage effect of FT.
Figure 2. Schematic diagram summarizing the impacts of soil freeze–thaw (FT) process on surface diabatic heating over Tibetan Plateau (TP). This diagram illustrates that soil moisture varies before freeze, FT, and after thaw (AT) periods, soil FT process has a water storage effect, and the storage index (SI) can reach 0.95 ± 0.06 at surface soil layer. Without the soil frozen process, the loss of soil moisture during AT period would be 10%, which further leads to anomalies of surface sensible heat by 4.73 ± 3.81 W/m2 and anomalies of surface latent heat by –1.07 ± 3.82 W/m2 through persistent soil moisture anomalies (Yang and Wang, 2019b). The map of the frozen soil over TP, including permafrost and seasonally frozen ground, comes from Li and Cheng (1996).
Along with the soil FT process, the soil moisture, the soil temperature, and the surface diabatic heating vary dramatically. Figure 3A shows the evolution of soil moisture, precipitation, and surface SH flux and surface LH flux based on the daily in situ observation data in Maqu, with the thawing of frozen ground, soil moisture content, and LH increase. In particular, the increase of LH has a considerable magnitude with SH in spring; about a month later, the LH continues to increase and becomes dominant, while the SH decreases, which is related to the coming of the rainy season over the eastern TP. Studies (e.g., Yang et al., 2002; Wang and Shang, 2007) suggested that the increase of soil moisture caused by the thawing of frozen ground about 20 days ago results in the onset of the subsequent rainy season of TP (Figure 3B) due to the supplement of abundant water vapor from surface evaporation. This implies that the thawing of frozen ground has an important role in the transition from dry season to wet season as well as the seasonal variations of surface diabatic heating over TP. The impacts of SM-FT on the coming of the wet season over TP can also be seen from the features of the clouds and the convection during the pre-onset periods of ASM. For instance, the results of Wang and Wang (2016) suggested that the frequency of a deep convective cloud increases rapidly after onset of the South Asian summer monsoon (SASM), which is attributed to the SM-FT process and the increase of surface diabatic heating (Wang et al., 2009a).
Figure 3. (A) Evolution of the in situ observation of soil moisture (SM), precipitation (Pr), surface sensible heat (SH), and surface latent heat (LH) in Maqu; the gray dashed line indicates the date of soil complete thaw (days = 82). (B) Lagging correlations (r) between soil moisture and precipitation in TTH during spring in year 1998 (black solid line) and 1999 (gray dash line); the red dashed line represents the lagging days (=20) by which r in both 1998 and 1999 passes the Student t significant test (p < 0.05). This figure is based on the data in Wang and Shang (2007).
Snow over TP is a dominant factor that influences the surface diabatic heating through its albedo effect and hydrological effect (Barnett et al., 1989). The snow cover over TP has evident spatial and temporal variations (Wang et al., 2009c; Wang Z. et al., 2017; Bo et al., 2015). Studies suggested that variations in the spatial distribution of snow cover over TP induce the change of energy and water exchanges between land and atmosphere, thereby affecting surface diabatic heating and changing thermal forcing of TP to the atmosphere (Zhang and Tao, 2001; Zhu and Ding, 2007). Wang and Cui (2011) suggested that due to the cooling effect of snow cover over TP by albedo, the difference between surface and air temperature (Ts-Ta) presents a decreasing trend in April. The stronger winter–spring snow cover over TP would lead to the colder anomalies of surface temperature due to the increase of albedo; both the SH and the LH decrease before the snow is completely melted. Studies suggested that the hydrological effects are dominant and far more important than the direct thermal or albedo effect (Barnett et al., 2005; Xu and Dirmeyer, 2012). The snow melting favors the increase of soil moisture and results in the increase of latent heat flux, the decrease of sensible heat flux, and cooler surface; the surface diabatic heating anomalies persistently affect the atmospheric circulation until the summer (Barnett et al., 1989; Yasunari et al., 1991; Qian et al., 2003a; Wang C. et al., 2017).
Many studies have emphasized on the contribution of TP thermal forcing to ASM (e.g., Ye et al., 1957; Ye and Gao, 1979; Wu and Zhang, 1998; Ye and Wu, 1998; Duan et al., 2005; Wu et al., 2012b; Cui et al., 2017). The changes of TP thermal forcing depend on the complex land surface process (e.g., SM-FT), which could influence the energy and water exchanges between land and atmosphere. There should be a connection between the SM-FT process over TP and the ASM activity.
An earlier study (Wang et al., 2003) suggested that the FT process over TP has significant impacts on the surrounding atmospheric circulations and is closely related with the summer precipitation in EC. As shown in Figure 4, the variation of the South China Sea summer monsoon is negatively correlated with the anomalies of soil frozen periods over TP (Shang and Wang, 2006). For instance, when the soil frozen period persists longer, the South China Sea Summer Monsoon would be weaker. Recent studies (Yang and Wang, 2019b, a) also indicated that the soil moisture memory calculated by the backward lagged correlation can reach up to 240 days (Figure 5A). This means that the soil moisture over TP during the preceding autumn (PSON) can persist up to spring (MAM), which is related to the soil FT process; the model simulation can also reproduce this persistence (Figure 5B). Similar as the spring soil moisture, the wetter soil moisture anomalies during PSON can lead to more precipitation over the Yangtze River Basin (YRB) and northeast China and less precipitation in south China and the Yellow River basin (Figure 5B); this corresponds to weak EASM. According to the water storage effect of the FT process, the mechanism associated with the impacts of the FT process over TP on EASM can be explained as follows: when the soil frozen periods are longer, more soil moisture anomalies can persist up to spring and favor the wetter anomalies of spring soil moisture; this would lead to weaker EASM. These results are consistent with the patterns of summer precipitation anomalies corresponding to the wetter spring soil moisture over TP (Wang et al., 2000, 2003; Chow et al., 2008; Li and Wang, 2016; Yang and Wang, 2019a).
Figure 4. (A) Changes in the anomalies of soil freezing days (blue solid line) and South China Sea summer monsoon index (red dashed line). (B) Correlation between the anomalies of soil freezing days and the anomalies of South China Sea summer monsoon index (Shang and Wang, 2006).
Figure 5. Seasonal persistence of soil moisture anomalies and its impacts on summer precipitation in Eastern China (EC) (Yang and Wang, 2019a). (A) Soil moisture memory, which is defined as the lagged days when the autocorrelation of soil moisture does not pass significant test at 0.01. (B) Patterns of soil moisture anomalies during preceding autumn (PSON), preceding winter (PDJF), and spring (MAM), when soil moisture is increased over the eastern region of the Tibetan Plateau (rectangular box). (C) Corresponding pattern of summer precipitation anomalies (mm/day) in EC to the soil moisture anomalies in panel (B).
The relationship between the anomalies of snow cover over TP and the ASM activity has been demonstrated by many studies (e.g., Chen, 1997, 1998, 2001; Chen and Wu, 2000; Qian et al., 2003a; Wu and Qian, 2003; Zhang et al., 2004; Wang et al., 2015). Many studies have demonstrated that there is a close relationship between snow cover over TP and EASM activity (Luo, 1995; Chen and Wu, 2000; Zhang and Tao, 2001; Xiao and Duan, 2016; Duan et al., 2018; You et al., 2020). For example, a heavier snow cover over TP can lead to a late onset of EASM and weaken the intensity of EASM. As a result, summer precipitation is less in south China and summer precipitation is more in the YRB (e.g., the flood occurs in YRB in 1998). The SASM is also a significant part of the ASM system, which has long been recognized to be influenced by snow cover over TP (Blanford, 1884; Chen and Wu, 2000; Fasullo, 2004; Brown and Mote, 2009; Dugam et al., 2009; Duan et al., 2014, 2018).
To explore the spatiotemporal variations of snow over TP and its relationship with ASM, Figure 6 shows the distribution of standard deviation and climatological mean of snow depth in the cold season and the evaluations of a standardized series of snow depth averaged over southern TP (STP) and northern TP (NTP) based on a dataset derived from SMMR, SSM/I, and AMSR-E passive microwave remote sensing data (Che et al., 2008). The snow over TP distributes mainly over southeastern TP, Himalaya, Kunlun, and Qilian Mountains. The standard deviations (interannual variations) of snow depth over the whole TP are considerable (Figure 6A). The seasonal variations of winter–spring snow cover over TP contain the snow melting to some extent. Soil moisture anomalies may contain the information of snow anomalies in the preceding winter and spring through the snow melting process (Barnett et al., 1989; Yasunari et al., 1991; Qian et al., 2003a). Due to the complex underlying land surface (e.g., topography and vegetation type), there should be a great difference in spatial variations of snow cover. As shown in Figure 6B, the interannual and interdecadal variations of snow depth over STP and NTP have distinct differences (Figure 6B). The difference of snow depth between NTP and STP has a significant correlation with the East Asian Summer Monsoon Index. This indicates that the impacts of the spatiotemporal anomalies of snow over TP on the ASM system are significant.
Figure 6. (A) Distribution of stand deviation (color) and climatological mean (1981–2010; contour, interval is 4 cm) of snow depth over the Tibetan Plateau (TP) in the cold season (defined as the duration from November to April in the next year), regions surrounded by the blue dashed lines and red solid lines indicate the southern TP (STP) and northern TP (NTP), respectively. (B) Evaluations of standardized series of snow depth averaged over STP and NTP. (C) Evaluations of standardized series of snow depth differences between STP and NTP (N-STP) and East Asian summer monsoon index (EASMI) [defined by Li and Zeng (2001)]. The snow depth dataset is derived from SMMR, SSM/I, and AMSR-E passive microwave remote sensing data (Che et al., 2008).
Observations and numerical simulations have suggested that there is a strong positive correlation between winter snow cover and subsequent summer precipitation over the middle and the lower reaches of the Yangtze River valley (Chen, 1998, 2001; Wu and Qian, 2003). Recent studies (Wang C. et al., 2017; Yang et al., 2017) showed that the heavier snow cover during spring–winter over STP and NTP can lead to different patterns of summer precipitation in EC. Figure 7 shows the percentage distributions of precipitation anomalies based on the daily precipitation data collected from 756 observation stations of the Chinese Meteorological Administration in a typical year of TP winter–spring heavier snow cover in STP and NST and the corresponding model simulations of summer precipitation anomalies by increasing surface albedo in STP and NTP during the period from November to March of the next year (Wang C. et al., 2017), with heavier snow cover in the southern TP corresponding to the weakened ASM and the stronger summer precipitation in the Yangtze River basin, but less summer precipitation in south China (Figure 7A); when snow is heavier in the northern TP, the ASM is enhanced. The summer precipitations in the YRB and north China become less and more, respectively, along with the northward shift of the rain belt (Figure 7C). Numerical simulations further confirm these patterns of summer precipitation anomalies in EC (Figures 7B,D). Results about the relation between winter–spring snow cover over TP and summer precipitation in EC have been applied in the operational summer climate prediction in China in the past years (Wang and Geng, 2012) and have achieved much success.
Figure 7. The percentage distributions of precipitation anomalies (%; compared to 1981–2010 climatology) based on the daily precipitation data collected from 756 observation stations of the Chinese Meteorological Administration in a typical year of the Tibetan Plateau (TP) heavier snow cover in (A) southern TP (1987, which corresponds to weak Asian summer monsoon) and (C) northern TP (1990, which corresponds to strong Asian summer monsoon). The patterns of summer precipitation anomalies (sensitive experiments minus control experiment; mm/day) in Eastern China (EC) from a (B) sensitive experiment with heavier snow in southern TP and a (D) sensitive experiment with heavier snow in northern TP. The anomalies over the region with black crosses are statistically significant at 90% level under Student’s t test (Wang C. et al., 2017).
Many studies have explored the physical mechanism by which TP thermal forcing affects the atmospheric circulation in East Asia (e.g., Wu and Qian, 2003; Wang B. et al., 2008; Wu et al., 2012a; Duan et al., 2014). For example, rising TP temperatures can trigger two distinct Rossby wave trains along the upper-level westerly jet and the low-level monsoon westerly, respectively, which propagate toward the downstream regions and enhance the low-level anticyclonic ridge (Wang B. et al., 2008). Wang et al. (2009a) indicated that surface diabatic heating over TP can enhance the low-frequency waves and force these to move northward and southward from TP. Li and Mao (2018) showed that the intra-seasonal Rossby wave train along the Asian westerly jet presents as the anomalous anticyclone over TP, which is located between anomalous cyclones in the upstream and the downstream areas, tending to trigger another reversed vertical–meridional cell with updraft over the south of the middle and the lower reaches of the Yangtze River valley (SMLY) and downdraft to the north through vorticity advection.
The thermal forcing anomalies of TP have direct impacts on the atmospheric circulation over TP and its surrounding regions, for instance, the vertical monsoon cell around TP and the westerly winds at the northern TP, which are the important systems of ASM (e.g., Ye and Wu, 1998; Schiemann et al., 2009). Recent studies (Wang C. et al., 2017; Yang et al., 2017) suggested that the negative anomalies of surface diabatic heating over TP induced by a heavier snow cover lead to the weakening of the ascent of the monsoon cell, the meridional temperature gradient over the northern TP and the mid-latitude westerlies. In addition, the position of the westerly jet at the downstream exit region also shifts southward because of the negative anomalies of surface diabatic heating over TP, which corresponds to low-level anomalous anticyclone over South China Sea and changes the water vapor transport in EC. When soil moisture anomalies induced by the FT process are wetter over TP, the physical mechanism explained by the anomalies of mid-latitude westerlies is also consistent (Yang and Wang, 2019a). By reviewing the previous studies (e.g., Yanai et al., 1992; Zhang and Tao, 2001; Qian et al., 2003a,b; Wang et al., 2003; Wu and Qian, 2003; Zhang et al., 2004; Shang and Wang, 2006; Wang and Shang, 2007; Zhu and Ding, 2007; Zhang and Zuo, 2011; Xiao and Duan, 2016; Wang C. et al., 2017; Yang and Wang, 2019b,a; You et al., 2020), the schematic diagram of the physical mechanism associated with the impacts of surface diabatic heating anomalies induced by the SM-FT process anomalies on EASM is depicted in Figure 8.
Figure 8. Schematic diagram of the physical mechanism associated with the impacts of surface diabatic heating anomalies induced by snow melting and freeze–thaw anomalies on East Asian summer monsoon. This is a summary graphic derived from previous studies (i.e., ①: Wang and Shang, 2007; Yang and Wang, 2019a; ②, ③: Seneviratne et al., 2010; Zhang and Zuo, 2011; Yang and Wang, 2019b; ④, ⑤, ⑥, ⑦: Zhang and Tao, 2001; Qian et al., 2003a, b; Xiao and Duan, 2016; Wang C. et al., 2017; Duan et al., 2018; ⑧, ⑨: Yanai et al., 1992; Zhang et al., 2004; Zhu and Ding, 2007; Wang C. et al., 2017; ⑩, ⑪, ⑫: Qian et al., 2003a; Wang et al., 2003; Wu and Qian, 2003; Zhang et al., 2004; Shang and Wang, 2006; Zhu and Ding, 2007; You et al., 2020.
In this review, the robust roles of surface diabatic heating anomalies induced by the SM-FT process over TP in the activities of the ASM system are summarized. The variations of surface diabatic heating over TP are closely related to the SM-FT processes and play prior role in the formation of TP heat source of the atmosphere in spring and summer. The soil freeze–thaw process affects surface diabatic heating through water storage effect, which could result in about 10% change of the soil moisture content and up to 10 W m–2 anomalies of surface SH and LH. Winter–spring snow cover anomalies over TP lead to the surface diabatic heating anomalies through its albedo cooling effect and hydrological effects. The surface SH over TP shows a decreasing trend, which might be related to the changes of the SM-FT process under the warming background.
The SM-FT process over TP has a significant relation with the ASM activity. There is a negative correlation between the anomalies of soil frozen periods and South China Sea summer monsoon. The impacts of soil FT process over TP on the surrounding atmospheric circulation are through its effects on soil moisture anomalies. The winter–spring snow cover over TP has a significant negative relationship with the onset of ASM; the spatiotemporal anomalies of snow cover over the northern TP and the southern TP have distinct different impacts on ASM system, which is an effective signal in the seasonal prediction of summer precipitation in EC.
The possible physical mechanism associated with the impacts of the SM-FT process on the atmospheric circulation in East Asia is summarized as follows: the positive (negative) anomalies of surface diabatic heating over TP induced by the anomalous SM-FT could weaken (enhance) the ascent of the TP monsoon cell and lead to the southward (northward) position of the westerly jet at the downstream exit region. These further influence the belt of summer precipitation in EC. The TP thermal forcing anomalies affect the atmospheric circulation over the downstream region through the anomalous Rossby wave propagation.
The impacts of the soil freeze–thaw process and snow melting over TP on the ASM system have achieved much progress in the past decades. However, the uncertainties of surface diabatic heating estimation over TP still restrict our understanding on the thermal forcing effects of TP, especially over the western TP where the in situ observations are sparse and satellite retrievals are unreliable. Currently, the parameterization of the SM-FT processes in a numerical model still has great uncertainties, which partly results in the disagreement in model simulations of climate over TP and East Asia. How to accurately estimate the surface diabatic heating of TP by reasonably depicting the SM-FT processes is still the challenge in a future study.
CW designed the study, contributed to the idea, and completed the manuscript writing. KY contributed to the data analysis and manuscript writing. FZ contributed to the manuscript review.
This work was supported by the project “Changes in the Land–Atmosphere Coupling System on the Tibetan Plateau and Its Global Climate Effects,” funded by the National Natural Science Foundation of China (91837205), and the National Natural Science Foundation of China (41661144017, 41801015, and 41805032).
The authors declare that the research was conducted in the absence of any commercial or financial relationships that could be construed as a potential conflict of interest.
Bao, H. Y., Yang, K., and Wang, C. (2017). Characteristics of GLDAS soil-moisture data on the Tibet Plateau. Sci. Cold Arid. Reg. 9, 127–141.
Barnett, T. P., Adam, J. C., and Lettenmaier, D. P. (2005). Potential impacts of a warming climate on water availability in snow-dominated regions. Nature 438, 303–309. doi: 10.1038/nature04141
Barnett, T. P., Dümenil, L., Schlese, U., Roeckneg, E., and Latif, M. (1989). The effect of Eurasian snow cover on regional and global climate variations. J. Atmos. Sci. 46, 661–686. doi: 10.1126/science.239.4839.504
Blanford, H. F. (1884). On the connexion of the Himalaya snowfall with dry winds and seasons of drought in India. Proc. Roy. Soc. Lond. 37, 1–23.
Bo, Y., Li, X., and Wang, C. (2015). Seasonal characteristics of the interannual variations centre of the Tibetan Plateau snow cover. J. Glaciol. Geocryol. 36, 1353–1362.
Brown, R. D., and Mote, P. W. (2009). The response of Northern hemisphere snow cover to a changing climate. J. Clim. 22, 2124–2145. doi: 10.1175/2008jcli2665.1
Che, T., Li, X., Jin, R., Armstrong, R., and Zhang, T. (2008). Snow depth derived from passive microwave remote-sensing data in China. Ann. Glaciol. 49, 145–154. doi: 10.3189/172756408787814690
Chen, L. (1997). “The influence ofwinter–spring snowcover over Tibetan Plateau on atmospheric circulation and flood season precipitation in south China,” in Middle and Long-Term Water Temperature Forecast Corpus, Vol. 1, (Beijing: China Waterpower Press∗), 194–195.
Chen, L. (1998). Test and application of the relationship between anomalous snow cover in winter-spring over Qinghai-Xizang Plateau and the first summer precipitation in South China. Q. J. Appl. Meteor. 9, 1–32.
Chen, L. (2001). The role of the anomalous snow cover over the Qinghai-Xizang Plateau and ENSO in the great floods of 1998 in the Changjiang River valley. Chin. J. Atmos. Sci. 25, 184–192.
Chen, L., and Wu, R. (2000). Interannual and decadal variations of snow cover over Qinghai-Xizang Plateau and their relationships to summer monsoon rainfall in China. Adv. Atmos. Sci. 17, 18–30. doi: 10.1007/s00376-000-0040-7
Chow, K. C., Chan, J. C. L., Shi, X., Liu, Y., and Ding, Y. (2008). Time-lagged effects of spring Tibetan Plateau soil moisture on the monsoon over China in early summer. Int. J. Climatol. 28, 55–67. doi: 10.1002/joc.1511
Cui, Y., Chang, Z., Yu, L., and Wang, C. (2017). Effects of Qinghai-Xizang Plateau’s spring surface diabatic heating anomalies on the East Asian summer monsoon intensity. J. Arid Meteor. 35, 1–11.
Cui, Y., and Wang, C. (2009). Comparison of sensible and latent heat fluxes from reanalysis datasets during the transition season over the western Tibetan Plateau. Prog. Nat. Sci. 19, 719–726. doi: 10.1016/j.pnsc.2008.11.001
Cuo, L., Zhang, Y., Wang, Q., Zhang, L., Zhou, B., Hao, Z., et al. (2013). Climate change on the northern Tibetan Plateau during 1957–2009: Spatial patterns and possible mechanisms. J. Clim. 26, 85–109. doi: 10.1175/jcli-d-11-00738.1
Ding, Y., Sun, Y., Wang, Z., Zhu, Y., and Song, Y. (2009). Inter-decadal variation of the summer precipitation in China and its association with decreasing Asian summer monsoon Part II: possible causes. Int. J. Climatol. 29, 1926–1944. doi: 10.1002/joc.1759
Ding, Y., Wang, Z., and Sun, Y. (2008). Inter-decadal variation of the summer precipitation in East China and its association with decreasing Asian summer monsoon. Part I: observed evidences. Int. J. Climatol. 28, 1139–1161. doi: 10.1002/joc.1615
Duan, A., Liu, Y., and Wu, G. (2005). Heating status of the Tibetan Plateau from April to June and rainfall and atmospheric circulation anomaly over East Asia in midsummer. Sci. China Ser. D-Earth Sci. 48, 250–257.
Duan, A., Wang, M., Lei, Y., and Cui, Y. (2013). Trends in summer rainfall over China associated with the Tibetan Plateau sensible heat source during 1980–2008. J. Clim. 26, 261–275. doi: 10.1175/jcli-d-11-00669.1
Duan, A., and Wu, G. (2005). Role of the Tibetan Plateau thermal forcing in the summer climate patterns over subtropical Asia. Clim. Dyn. 24, 793–807. doi: 10.1007/s00382-004-0488-8
Duan, A., and Wu, G. (2008). Weakening trend in the atmospheric heat source over the Tibetan Plateau during recent decades. Part I: observations. J. Clim. 21, 3149–3164. doi: 10.1175/2007jcli1912.1
Duan, A., Xiao, Z., and Wang, Z. (2018). Impacts of the Tibetan Plateau winter/spring snow depth and surface heat source on Asian summer monsoon: a review. Chin. J. Atmos. Sci. 42, 755–766.
Duan, A., Xiao, Z., Wu, G., and Wang, M. (2014). Study progress of the influence of the Tibetan Plateau winter and spring snow depth on Asian summer monsoon. Meteorol. Environ. Sci. 37, 94–101.
Dugam, S. S., Bansod, S. D., and Kakade, S. B. (2009). Pre-monsoon zonal wind Index over Tibetan Plateau and sub-seasonal Indian summer monsoon rainfall variability. Geophys. Res. Lett. 36:L11809.
Fasullo, J. (2004). A stratified diagnosis of the Indian Monsoon—Eurasian snow cover relationship. J. Clim. 17, 1110–1122. doi: 10.1175/1520-0442(2004)017<1110:asdoti>2.0.co;2
Flohn, H. (1957). Large-scale aspects of the “summer monsoon” in south and east Asia. J. Meteor. Soc. Jpn. 75, 180–186. doi: 10.2151/jmsj1923.35a.0_180
Flohn, H. (1960). “Recent investigations on the mechanism of the “summer monsoon” of southern and eastern Asia,” Paper Presented at Proceedings of the Symposium Monsoon of the World, New Delhi.
Gao, B., Yang, D., Qin, Y., Wang, Y., Li, H., Zhang, Y., et al. (2018). Change in frozen soils and its effect on regional hydrology, upper Heihe basin, northeastern Qinghai–Tibetan Plateau. Cryosphere 12, 657–673. doi: 10.5194/tc-12-657-2018
Gao, Y., Li, X., Leung, L. R., Chen, D., and Xu, J. (2015). Aridity changes in the Tibetan Plateau in a warming climate. Environ. Res. Lett. 10, 34013–34024. doi: 10.1007/s00248-017-1098-4
Ge, J., Yu, Y., Li, Z., Xie, J., Liu, C., and Zan, B. (2016). Impacts of freeze/thaw processes on land surface energy fluxes in the permafrost region of Qinghai-Xizang Plateau. Plateau Meteorol. 35, 608–620.
Helfrich, S. R., Mcnamara, D., Ramsay, B. H., Baldwin, T., and Kasheta, T. (2007). Enhancements to, and forthcoming developments in the Interactive Multisensor Snow and Ice Mapping System (IMS). Hydrol. Process. 21, 1576–1586. doi: 10.5194/amt-11-2983-2018
Jung, M., Reichstein, M., Ciais, P., Seneviratne, S., Sheffield, J., Goulden, M., et al. (2010). Recent decline in the global land evapotranspiration trend due to limited moisture supply. Nature 467, 951–954. doi: 10.1038/nature09396
Li, D., and Wang, C. (2016). The relation between soil moisture over the Tibetan Plateau in spring and summer precipitation in the eastern China. J. Glaciol. Geocryol. 38, 89–99.
Li, J., and Mao, J. (2018). The impact of interactions between tropical and midlatitude intraseasonal oscillations around the Tibetan Plateau on the 1998 Yangtze floods. Q. J. R. Meteorol. Soc. 144, 1123–1139. doi: 10.1002/qj.3279
Li, J., and Zeng, Q. (2001). A unified monsoon index. Geophys. Res. Lett. 29:1274. doi: 10.1029/2001GL013874
Li, S., and Cheng, G. (1996). Frozen ground map of the Qinghai-Tibet Plateau. Lanzhou: Gansu Culture Press.
Li, S., Yang, K., and Wang, C. (2018). Bias characteristics of land surface model (CLM4.5) over Tibetan Plateau during soil freezing-thawing period and its causes. J. Glaciol. Geocryol. 40, 322–334.
Li, X., and Koike, T. (2003). Frozen soil parameterization in SiB2 and its validation with GAME-Tibet observations. Cold Reg. Sci. Technol. 36, 165–182. doi: 10.1016/s0165-232x(03)00009-0
Liu, Y., Wu, G., Hong, J., Dong, B., Duan, A., Bao, Q., et al. (2012). Revisiting Asian monsoon formation and changes associated with Tibetan Plateau forcing: II. Change Clim. Dyn. 39, 1183–1195. doi: 10.1007/s00382-012-1335-y
Luo, H., and Yanai, M. (1983). The large-scale circulation and heat sources over the Tibetan Plateau and surrounding areas during the early summer of 1979. Part I: precipitation and kinematic analyses. Mon. Wea. Rev. 111, 922–944. doi: 10.1175/1520-0493(1983)111<0922:tlscah>2.0.co;2
Luo, Y. (1995). Studies on the effect of snow cover over the Qinghai-Xizang Plateau in winter and spring on general circulation over east Asia in summer. Plateau Meteorol. 14, 505–512.
Meehl, G. A. (1994). Coupled ocean-atmosphere-land processes and South Asian monsoon variability. Science 265, 263–267. doi: 10.1126/science.266.5183.263
Meng, X., Li, R., Luan, L., Lyu, S., Zhang, T., Ao, Y., et al. (2018). Detecting hydrological consistency between soil moisture and precipitation and changes of soil moisture in summer over the Tibetan Plateau. Clim. Dyn. 51, 4157–4168. doi: 10.1007/s00382-017-3646-5
Niu, G., and Yang, Z.-L. (2007). Effects of frozen soil on snowmelt runoff and soil water storage at a continental scale. J. Hydrometeorol. 7, 937–952. doi: 10.1175/jhm538.1
Njoku, E., Jackson, T., Lakshmi, V., Chan, T., and Nghiem, S. (2003). Soil moisture retrieval from AMSR-E. IEEE Trans. Geosci. Remote Sens. 41, 215–229. doi: 10.1109/TGRS.2002.808243
Orsolini, Y., Wegmann, M., Dutra, E., Liu, B., Balsamo, G., Yang, K., et al. (2019). Evaluation of snow depth and snow cover over the Tibetan Plateau in global reanalyses using in situ and satellite remote sensing observations. Cryosphere 13, 2221–2239. doi: 10.5194/tc-13-2221-2019
Pu, Z., Xu, L., and Salomonson, V. V. (2007). MODIS/Terra observed seasonal variations of snow cover over the Tibetan Plateau. Geophys. Res. Lett. 34:L06706.
Qian, Y., Zhang, Y., and Zheng, Y. Q. (2003a). Impacts of the Tibetan Plateau snow anomaly in winter and spring on precipitation in China in spring and summer. J. Arid Meteor. 21, 1–7.
Qian, Y., Zheng, Y., Zhang, Y., and Miao, M. (2003b). Responses of China’s summer monsoon climate to snow anomaly over the Tibetan Plateau. Int. J. Climatol. 23, 593–613. doi: 10.1002/joc.901
Qin, D., Liu, S. Y., and Li, P. J. (2006). Snow cover distribution, variability, and response to climate change in western China. J. Clim. 19, 1820–1833. doi: 10.1175/jcli3694.1
Rodell, M., et al. (2004). The Global Land Data Assimilation System. Bull. Amer. Meteor. Soc. 85, 381–394.
Schiemann, R., Lüthi, D., and Schär, C. (2009). Seasonality and interannual variability of the westerly jet in the Tibetan Plateau region. J. Clim. 22, 2940–2957. doi: 10.1175/2008jcli2625.1
Seneviratne, S. I., Corti, T., Davin, E. L., Hirschi, M., Jaeger, E. B., Lehner, I., et al. (2010). Investigating soil moisture–climate interactions in a changing climate: a review. Earth. Sci. Rev. 99, 125–161. doi: 10.1016/j.earscirev.2010.02.004
Shang, D., and Wang, C. (2006). The effect of the frozen-thaw process in Tibetan Plateau on summer monsoon over Eastern Asia. J. Arid Meteor. 24, 19–22.
Shi, Q., and Liang, S. (2014). Surface-sensible and latent heat fluxes over the Tibetan Plateau from ground measurements, reanalysis, and satellite data. Atmos. Chem. Phys. 14, 5659–5677. doi: 10.5194/acp-14-5659-2014
Swenson, S. C., Lawrence, D. M., and Lee, H. (2012). Improved simulation of the terrestrial hydrological cycle in permafrost regions by the Community land model. J. Adv. Model. Earth Syst. 4:M08002. doi: 10.1029/2012MS000165
Wang, B., Bao, Q., Hoskins, B., Wu, G., and Liu, Y. (2008). Tibetan Plateau warming and precipitation changes in East Asia. Geophys. Res. Lett. 35:L14702. doi: 10.1029/2008GL034330
Wang, C., Shi, R., and Zuo, H. (2008). Analysis on simulation of characteristic of land surface in western Qinghai-Xizang Plateau during frozen and thawing. Plateau Meteorol. 27, 239–248.
Wang, C., and Cui, Y. (2011). Characteristics of the difference of temperature between surface and atmosphere over the Tibetan Plateau in the early stage of East Asian summer monsoon onset. Clim. Environ. Res. 16, 586–596.
Wang, C., Dong, A., Wang, S., and Yang, D. (2000). The correlation between precipitation in Northwest China during spring and snow depth in the Tibetan Plateau. J. Glaciol. Geocryol. 22, 340–346.
Wang, C., Dong, W., and Wei, Z. (2003). Study on relationship between the frozen-thaw process in Qinghai-Xizang Plateau and circulation in East-Asia. Chin. J. Geophys. 46, 309–316.
Wang, C., and Geng, L. (2012). Researching and application of the singular spectrum analysis combined with multi-regression in prediction of summer precipitation over China. Meteorol. Mon. 38, 41–46.
Wang, C., Li, Y., and Wang, Y. (2015). The impacts of interdecadal variability of circulation and Winter Monsoon on Winter Snowfall over the Tibetan Plateau. Clim. Environ. Res. 20, 421–432.
Wang, C., and Shang, D. (2007). Effect of the variation of the soil temperature and moisture in the transition from dry-season to wet-season over Northern Tibet Plateau. Plateau Meteorol. 26, 677–685.
Wang, C., Cui, Y., Jin, S., and Yan, Q. (2009a). Characteristics of Low Frequency Oscillation of spring atmospheric circulation over Qinghai-Xizang Plateau during strong and weak year of the South China Sea summer monsoon. Prog. Nat. Sci. 11, 1194–1202.
Wang, C., Shi, R., and Cui, Y. (2009b). Simulation analysis on characteristics of land surface over western Qinghai-Xizang Plateau during freezing–thawing period. Sci. Cold Arid Reg. 1, 329–340.
Wang, C., Wang, Z. L., and Cui, Y. (2009c). Spatial distributions and interannual variations of snow cover over China in the last 40 years. Sci. Clod Arid. Reg. 1, 509–518.
Wang, C., Yang, K., Li, Y., Wu, D., and Bo, Y. (2017). Impacts of Spatiotemporal Anomalies of Tibetan Plateau Snow Cover on Summer Precipitation in Eastern China. J. Clim. 30, 885–903. doi: 10.1175/jcli-d-16-0041.1
Wang, Z., Wu, R., and Huang, G. (2017). Low-frequency snow changes over the Tibetan Plateau. Int. J. Climatol. 38, 949–963. doi: 10.1002/joc.5221
Wang, Y., and Wang, C. (2016). Features of clouds and convection during the pre-and post-onset periods of the Asian summer monsoon. Theor. Appl. Climatol. 123, 551–564. doi: 10.1007/s00704-015-1372-7
Webster, P. J. (1998). Monsoons: processes, predictability and the prospects for prediction. J. Geophys. Res. 103, 14451–14510. doi: 10.1029/97jc02719
Wu, G. (1984). The nonlinear response of the atmosphere to largescale mechanical and thermal forcing. J. Atmos. Sci. 41, 2456–2476. doi: 10.1175/1520-0469(1984)041<2456:tnrota>2.0.co;2
Wu, G., Duan, A., Liu, Y., Mao, J., Ren, R., Bao, Q., et al. (2014). Tibetan Plateau climate dynamics: recent research progress and outlook. Natl. Sci. Rev. 2, 100–116. doi: 10.1093/nsr/nwu045
Wu, G., and Liu, Y. (2000). Thermal adaptation, overshooting, dispersion, and subtropical high. Part I: thermal adaptation and overshooting. Chin. J. Atmos. Sci. 24, 433–436.
Wu, G., Liu, Y., Dong, B., Liang, X., Duan, A., Bao, Q., et al. (2012a). Revisiting Asian monsoon formation and change associated with Tibetan Plateau forcing: I. Formation. Clim. Dyn. 39, 1169–1181. doi: 10.1007/s00382-012-1334-z
Wu, G., Liu, Y., He, B., Bao, Q., Duan, A., and Jin, F.-F. (2012b). Thermal controls on the Asian Summer Monsoon. Sci. Rep. 2:65. doi: 10.1038/srep00404
Wu, G., and Zhang, Y. (1998). Tibetan Plateau forcing and the timing of the monsoon onset over South Asia and the South China Sea. Mon. Wea. Rev. 126, 913–927. doi: 10.1175/1520-0493(1998)126<0913:tpfatt>2.0.co;2
Wu, T., and Qian, Z. (2003). The relation between the Tibetan winter snow and the Asian summer monsoon and rainfall: an observational investigation. J. Clim. 16, 2038–2051. doi: 10.1175/1520-0442(2003)016<2038:trbttw>2.0.co;2
Xiao, Z., and Duan, A. (2016). Impacts of Tibetan Plateau Snow Cover on the Interannual variability of the East Asian Summer Monsoon. J. Clim. 29, 8495–8514. doi: 10.1175/JCLI-D-16-0029.1
Xie, J., Yu, Y., Li, J., Ge, J., and Liu, C. (2018). Comparison of surface sensible and latent heat fluxes over the Tibetan Plateau from reanalysis and observations. Meteorol. Atmos. Phys. 131, 567–584. doi: 10.1007/s00703-018-0595-4
Xu, L., and Dirmeyer, P. (2012). Snow–atmosphere coupling strength. Part II: Albedo effect versus hydrological effect. J. Hydrometeorol. 14, 404–418. doi: 10.1175/jhm-d-11-0103.1
Yanai, M., Li, C., and Song, Z. (1992). Seasonal heating of the Tibetan Plateau and its effects on the evolution of the Asian summer monsoon. J. Meteor. Soc. Jpn. 70, 319–351. doi: 10.2151/jmsj1965.70.1b_319
Yang, K., Hu, T., and Wang, C. (2017). A numerical study on the relationship between the spring–winter snow cover anomalies over the northern and southern Tibetan Plateau and summer precipitation in East China. Chin. J. Atmos. Sci. 41, 345–356. doi: 10.3878/j.issn.1006-9895.1604.16119
Yang, K., and Wang, C. (2019a). Water storage effect of soil freeze-thaw process and its impacts on soil hydro-thermal regime variations. Agric. For. Meteorol. 265, 280–294. doi: 10.1016/j.agrformet.2018.11.011
Yang, K., and Wang, C. (2019b). Seasonal persistence of soil moisture anomalies related to freeze–thaw over the Tibetan Plateau and prediction signal of summer precipitation in eastern China. Clim. Dyn. 53, 2411–2424. doi: 10.1007/s00382-019-04867-1
Yang, K., Wang, C., and Bao, H. (2016). Contribution of soil moisture variability to summer precipitation in the Northern Hemisphere. J. Geophys. Res. Atmos. 121, 108–124.
Yang, M., Wang, X., Pang, G., Wan, G., and Liu, Z. (2019). The Tibetan Plateau cryosphere: observations and model simulations for current status and recent changes. Earth. Sci. Rev. 190, 353–369. doi: 10.1016/j.earscirev.2018.12.018
Yang, Y., Liu, Y., Li, M., Hu, Z., and Ding, Z. (2019). Assessment of reanalysis flux products based on eddy covariance observations over the Tibetan Plateau. Theor. Appl. Climatol. 138, 275–292. doi: 10.1007/s00704-019-02811-1
Yang, M., Yao, T., He, Y., Zhang, X., and Ma, Y. (2002). The water cycles between land surface and atmosphere in northern part of Tibetan Plateau. Sci. Geogr. Sin. 22, 29–33.
Yao, T., Pu, J., Lu, A., Wang, Y., and Yu, W. (2007). Recent glacial retreat and its impact on hydrological processes on the Tibetan Plateau. China, and surrounding regions. Arct. Antarct. Alp. Res. 39, 642–650. doi: 10.1657/1523-0430(07-510)[yao]2.0.co;2
Yasunari, T., Kitoh, A., and Tokioka, T. (1991). Local and remote responses to excessive snow mass over Eurasia appearing in the Northern spring and summer climate-a study with the MRI GCM. J. Meteorol. Soc. Jpn. 69, 473–487. doi: 10.2151/jmsj1965.69.4_473
Ye, D., and Gao, Y. (1979). Meteorology of the Qinghai-Xizang Plateau. Beijing: Chinese Science Press.
Ye, D., Luo, S., and Chu, P. (1957). The wind structure and heat balance in the lower troposphere over Tibetan Plateau and its surrounding. Acta Meteor. Sin. 28, 20–33.
Ye, D., and Wu, G. (1998). The role of the heat source of the Tibetan Plateau in the general circulation. Meteorol. Atmos. Phys. 67, 181–198. doi: 10.1007/bf01277509
You, Q., Wu, T., Shen, L., Pepin, N., Zhang, L., Jiang, Z., et al. (2020). Review of snow cover variation over the Tibetan Plateau and its influence on the broad climate system. Earth. Sci. Rev. 201:103043. doi: 10.1016/j.earscirev.2019.103043
Zhang, R., and Zuo, Z. (2011). Impact of spring soil moisture on surface energy balance and summer monsoon circulation over East Asia and precipitation in East China. J. Clim. 24, 3309–3322. doi: 10.1175/2011jcli4084.1
Zhang, S., and Tao, S. (2001). Influences of snow cover over the Tibetan Plateau on Asian summer monsoon. Chin. J. Atmos. Sci. 25, 372–390.
Zhang, T. (2005). Influence of the seasonal snow cover on the ground thermal regime: an overview. Rev. Geophys. 43:RG4002.
Zhang, X., Sun, S., and Xue, Y. (2007). Development and testing of a frozen soil parameterization for cold region studies. J. Hydrometeorol. 8, 852–861.
Zhang, Y., Li, T., and Wang, B. (2004). Decadal change of the spring snow depth over the Tibetan Plateau: the associated circulation and influence on the east Asian summer monsoon. J. Clim. 17, 2780–2793. doi: 10.1175/1520-0442(2004)017<2780:dcotss>2.0.co;2
Zhao, P., and Chen, L. (2001). Climatic features of atmospheric heat source/sink over the Qinghai-Xizang Plateau in 35 years and its relation to rainfall in China. Sci. China Ser. D-Earth Sci. 44, 858–864. doi: 10.1007/bf02907098
Zhu, L., Huang, G., Fan, G., Qü, X., Wang, Z., and Hua, W. (2018). Elevation-dependent sensible heat flux trend over the Tibetan Plateau and its possible causes. Clim. Dyn. 52, 3997–3400.
Keywords: Tibetan Plateau, Asian summer monsoon, diabatic heating, freeze–thaw process, snow melting
Citation: Wang C, Yang K and Zhang F (2020) Impacts of Soil Freeze–Thaw Process and Snow Melting Over Tibetan Plateau on Asian Summer Monsoon System: A Review and Perspective. Front. Earth Sci. 8:133. doi: 10.3389/feart.2020.00133
Received: 02 March 2020; Accepted: 07 April 2020;
Published: 02 June 2020.
Edited by:
Wen Chen, Institute of Atmospheric Physics (CAS), ChinaReviewed by:
Haishan Chen, Nanjing University of Information Science and Technology, ChinaCopyright © 2020 Wang, Yang and Zhang. This is an open-access article distributed under the terms of the Creative Commons Attribution License (CC BY). The use, distribution or reproduction in other forums is permitted, provided the original author(s) and the copyright owner(s) are credited and that the original publication in this journal is cited, in accordance with accepted academic practice. No use, distribution or reproduction is permitted which does not comply with these terms.
*Correspondence: Chenghai Wang, d2NoQGx6dS5lZHUuY24=
Disclaimer: All claims expressed in this article are solely those of the authors and do not necessarily represent those of their affiliated organizations, or those of the publisher, the editors and the reviewers. Any product that may be evaluated in this article or claim that may be made by its manufacturer is not guaranteed or endorsed by the publisher.
Research integrity at Frontiers
Learn more about the work of our research integrity team to safeguard the quality of each article we publish.