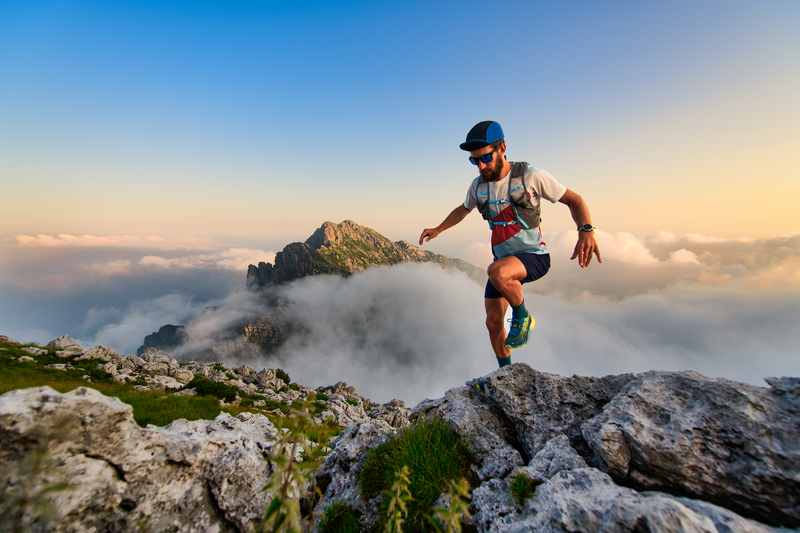
95% of researchers rate our articles as excellent or good
Learn more about the work of our research integrity team to safeguard the quality of each article we publish.
Find out more
ORIGINAL RESEARCH article
Front. Earth Sci. , 25 February 2020
Sec. Volcanology
Volume 8 - 2020 | https://doi.org/10.3389/feart.2020.00036
This article is part of the Research Topic The Impact of Open Science for Evaluation of Volcanic Hazards View all 8 articles
Among the hazards posed by volcanoes are the emissions of gases and particles that can affect air quality and damage agriculture and infrastructure. A recent intense episode of volcanic degassing associated with severe impacts on air quality accompanied the 2018 lower East Rift Zone (LERZ) eruption of Kīlauea volcano, Hawai'i. This resulted in a major increase in gas emission rates with respect to usual emission values for this volcano, along with a shift in the source of the dominant plume to a populated area on the lower flank of the volcano. This led to reduced air quality in downwind communities. We analyse open-access data from the permanent air quality monitoring networks operated by the Hawai'i Department of Health (HDOH) and National Park Service (NPS), and report on measurements of atmospheric sulfur dioxide (SO2) between 2007 and 2018 and PM2.5 (aerosol particulate matter with diameter <2.5 μm) between 2010 and 2018. Additional air quality data were collected through a community-operated network of low-cost PM2.5 sensors during the 2018 LERZ eruption. From 2007 to 2018 the two most significant escalations in Kīlauea's volcanic emissions were: the summit eruption that began in 2008 (Kīlauea emissions averaged 5–6 kt/day SO2 from 2008 until summit activity decreased in May 2018) and the LERZ eruption in 2018 when SO2 emission rates reached a monthly average of 200 kt/day during June. In this paper we focus on characterizing the airborne pollutants arising from the 2018 LERZ eruption and the spatial distribution and severity of volcanic air pollution events across the Island of Hawai'i. The LERZ eruption caused the most frequent and severe exceedances of the Environmental Protection Agency (EPA) PM2.5 air quality threshold (35 μg/m3 as a daily average) in Hawai'i in the period 2010–2018. In Kona, for example, the maximum 24-h-mean mass concentration of PM2.5 was recorded as 59 μg/m3 on the twenty-ninth of May 2018, which was one of eight recorded exceedances of the EPA air quality threshold during the 2018 LERZ eruption, where there had been no exceedances in the previous 8 years as measured by the HDOH and NPS networks. SO2 air pollution during the LERZ eruption was most severe in communities in the south and west of the island, as measured by selected HDOH and NPS stations in this study, with a maximum 24-h-mean mass concentration of 728 μg/m3 recorded in Ocean View (100 km west of the LERZ emission source) in May 2018. Data from the low-cost sensor network correlated well with data from the HDOH PM2.5 instruments, confirming that these low-cost sensors provide a robust means to augment reference-grade instrument networks.
Volcanic clouds are complex, evolving mixtures of volcanic and atmospheric gases, primary and secondary aerosol particles, ash and dust (Oppenheimer and McGonigle, 2004; Pfeffer et al., 2006b; von Glasow et al., 2009; Oppenheimer et al., 2010; Langmann, 2014). As well as the potential for global climatic consequences from explosive or large-scale volcanic emissions, low altitude volcanic clouds can have important impacts on air quality, human and animal health, and the environment on the local to regional scale (Hansell and Oppenheimer, 2004; Barsotti et al., 2010; Mather, 2015; Schmidt et al., 2015; Andronico and Del Carlo, 2016; Tam et al., 2016; Ilyinskaya et al., 2017).
Gaseous sulfur dioxide (SO2) is usually highly concentrated in volcanic emissions compared to the background atmosphere and is often the focus of gas emission monitoring due to the relative ease of its measurement and its important environmental and air quality consequences (Cadle et al., 1971; Lambert et al., 1988; Loughlin et al., 2012; Schmidt et al., 2015). Population sub-groups including children, asthmatics and cardiac- or respiratory-compromised individuals are particularly vulnerable to exposure to SO2 (ATSDR, 1998; CRI, 2004). For example, exposure to mass concentrations of 1,310 μg/m3 SO2 for 3 min can induce respiratory attacks in asthmatic individuals (Balmes et al., 1987; ATSDR, 1998). In 2010 the U.S. Environmental Protection Agency (EPA) set the National Ambient Air Quality Standard (NAAQS) for SO2 mass concentration exposure limits at 195 μg/m3 as an hourly average (EPA, 2010). Persistent volcanic SO2 emissions on the Island of Hawai'i led the state of Hawai'i being designated as unclassifiable for the EPA 2010 NAAQS, and as such Hawai'i uses the pre-2010 EPA SO2 exposure limit of 366 μg/m3 as 24-h average (EPA, 2013). The European Commission (EC) air quality standards recommend a SO2 mass concentration threshold of 350 μg/m3 for a 3-h average, and 125 μg/m3 as a daily average (EC, 2018).
Particulate matter (PM) in volcanic clouds is also significant in the context of environment and health. The chemical composition of volcanogenic PM2.5 (PM with diameter <2.5 μm) is highly heterogeneous. Typical chemical species include sulfates (primary emissions or formed via oxidation of sulfur gases) (Cadle et al., 1971; Stockwell and Calvert, 1983; Allen et al., 2002; Mather et al., 2003; Langmann, 2014) and halides, with an array of metals and metalloids including environmentally-harmful species such as lead and cadmium (Longo, 2013; Langmann, 2014). PM2.5 is a well-established indicator for air quality, since it commonly includes particulates derived from transport and industrial sources, fine wind-blown mineral dust, ambient matter, and volcanic material (Lim et al., 2012; Tam et al., 2016; Holgate, 2017; Butwin et al., 2019). It has been estimated that the health burden due to exposure to ambient PM2.5 globally amounts to more than three million premature deaths each year (Lim et al., 2012), and is especially linked to increases in death from cardiovascular and respiratory diseases in vulnerable individuals (Holgate, 2017). EPA NAAQS thresholds recommend a PM2.5 mass concentration exposure limit of 35 μg/m3 as a daily average (EPA, 2013). This is higher than the 24-h mean exposure guideline of 25 μg/m3 established by the World Health Organization (WHO, 2005).
Kīlauea volcano on the Island of Hawai'i consists of a summit caldera at 1,200 m a.s.l. and rift zones to the south-west and east. From 1983 until 2008, activity at Kīlauea was concentrated on the middle East Rift Zone (ERZ), primarily near the Pu‘u ‘Ō‘ō vent (Elias and Sutton, 2007; Poland et al., 2008) (Figure 1A). Between 2002 and 2006, average SO2 flux from the ERZ was 1.7 ± 0.7 kt/day, while emissions from the summit were low at 0.1 kt/day (Elias and Sutton, 2007). From November 2007 to March 2008, SO2 emissions at the summit increased to levels 10 times the long-term background (Wooten et al., 2009) (Figure 2A). On the twelfth of March 2008, a new vent opened within the Halema‘uma‘u summit crater, leading to sporadic explosive eruptions and increased degassing of SO2.
Figure 1. Island of Hawai'i. (A) Ground-based sampling networks; HDOH and NPS stations indicated with black stars. Kīlauea summit, Pu‘u ‘Ō‘ō vent and 2018 lower East Rift Zone eruption site indicated with red triangles. Selection area of community-operated PM2.5 instruments indicated with blue highlight. Wind directions at Kona International Airport (KIA, empty black cross) and Hilo International Airport (HIA, empty black square) displayed as seasonal wind roses for period 2007–2018 (NOAA, 2019). Note "Volcano Ob." stands for Volcano Observatory. (B) Population density per square kilometer on the Island of Hawai'i, based on 2010 Census data. Highest population density is located in the east-coast city of Hilo (population ~45,700) and the west-coast city of Kailua-Kona (population ~28,500) (Hawai'i Department of Business Economic Development and Tourism, 2011).
Figure 2. SO2 mass concentrations relative to health guidelines in selected populated areas of Island of Hawai'i. (A) SO2 emissions from Kīlauea summit indicated with black stars; from ERZ indicated with red triangles; from 2018 LERZ eruption indicated with blue square. Note logarithmic scale. (B–D) SO2 air quality (24-h mean) in communities from 2007 to the end of 2018. Solid red line: Hawai'i Standard air quality threshold (24-h mean of 366 μg/m3); dashed orange line: European Commission (EC) air quality threshold (24-h mean of 125 μg/m3). Dashed black line: 2008 initiation of summit activity; blue highlight: 2018 LERZ eruption duration. (A) SO2 emissions data sourced from Elias and Sutton (2012), Beirle et al. (2014), Elias et al. (2018), and Elias et al. (in preparation). Summit emissions for 2008–2012 are from Beirle et al. (2014). LERZ data-point represents the average SO2 emission from the LERZ eruption for June 2018, taken from Kern et al. (2019). (B) Note that “Volcano Ob.” stands for Volcano Observatory; (D) seasonal average for Hilo between 2007 and 2017 indicated by orange bars.
Kīlauea's SO2 emissions peaked in the summer of 2008, when a total emission rate of up to 20 kt/day was measured by satellite sensors (Beirle et al., 2014). At this time, emissions from both the ERZ and the summit were significant, with the two sources contributing variable amounts to the total degassing rate (Elias and Sutton, 2012). For the period 2009–2017, the dynamic activity at Kīlauea was reflected in variable emissions, with a long-term average of 5–6 kt/day based on satellite and ground-based measurements (Eguchi et al., 2011; Elias and Sutton, 2012; Carn et al., 2016; Elias et al., 2018) (Figure 2A). Lava was first observed in the Halema‘uma‘u summit crater in September 2008, with a permanent lava lake visible from February 2010 until May 2018 (Patrick et al., 2013; Neal et al., 2019).
The 2018 Kīlauea eruption in the lower East Rift Zone (LERZ) began following the collapse of the Pu‘u ‘Ō‘ō vent on the thirtieth of April (Neal et al. 2019; HVO, 2018). Twenty-four fissures opened over a distance of 6.8 km in the vicinity of Leilani Estates (Figure 1A). During the first week of the LERZ eruption, spattering activity at individual fissures was typically short-lived (minutes to hours in duration) and lava was viscous with spatter deposition within tens of meters of individual fissures. On the eighteenth of May, the eruptive style evolved to less viscous lava and resulted in fast-moving lava flows which reached the ocean 2 days later (HVO, 2018). By the end of May 2018, activity had become focused at Fissure 8, and this remained the dominant fissure for the remainder of the LERZ eruption (Neal et al., 2019). Lava fountains from Fissure 8 reached heights of 80 m, and lava effusion rates ranged from 50 to 200 m3/s (Neal et al., 2019). Lava from Fissure 8 flowed in a semi-stable channel to the ocean and eventually covered an area of land 35.5 km2 in size (Neal et al. 2019; HVO, 2018). This eruption was the largest along Kīlauea's LERZ in the last two centuries and had far-reaching impacts around the Island of Hawai'i. With the collapse of the Pu‘u ‘Ō‘ō vent and draining of Kīlauea's summit magma reservoir, the dominant source of volcanic SO2 became the LERZ eruptive vents. SO2 emissions reached an average of 200 kt/day in June 2018 (Kern et al. 2019), severely impacting island-wide air quality. The eruption declined rapidly at the end of July and lava effusion ceased on the fourth of August 2018 (Neal et al., 2019).
Since initiation of intermittent fountaining activity at Kīlauea in 1983, SO2 emissions have been a health concern among downwind communities on the Island of Hawai'i. As emissions from Kīlauea are dispersed downwind, communities are exposed to volcanic smog, locally known as vog, predominantly composed of SO2 and fine particles of sulfuric acid aerosol (Longo, 2009; Longo et al., 2010; Halliday et al., 2015; Tam et al., 2016; Elias and Sutton, 2017). Prevailing trade winds from the north-east, particularly during the period from April to October, carry Kīlauea's emissions over the communities to the south and west (Longo et al., 2005, 2008; Michaud et al., 2007; Tam et al., 2016; Elias and Sutton, 2017) (Figure 1A). The Island of Hawai'i has relatively low population density in the south (Figure 1B), with ~4,400 residents in Ocean View and ~1,300 residents in Pāhala. Trade winds from the north-east are influenced by the high topography of Mauna Loa and Mauna Kea, generating more localized air movement in the lee of the island on the west coast (Michaud et al., 2007) (Figure 1A). This wind shadow allows a potentially longer residence time for air pollutants (volcanogenic or otherwise) along the densely-populated western coastline (Figure 1B). During the winter months (November to March), the trade winds weaken and southerly and westerly winds may distribute vog toward the densely-populated eastern coastline of the island (Wyrtki and Meyers, 1976; Mannino et al., 1996; Michaud et al., 2004) (Figure 1B).
Numerous studies have investigated the impact of Kīlauea's SO2 emissions on the health of island residents, even at the relatively low levels of degassing prior to the emergence of the lava lake in 2008. Mannino et al. (1996) reviewed the frequency of visits to emergency departments and hospitalizations for respiratory issues during periods of continuous and discontinuous SO2 emissions throughout the 1980s. Communities on the western side of the island frequently exposed to vog were found to have higher rates of hospitalizations for chronic obstructive pulmonary disease than the east-coast city of Hilo, which is rarely exposed to vog. Periods of weakened north-easterly trade winds coincided with a 15 % increase in emergency department visits for asthma in Hilo (Mannino et al., 1996). In 2004, the health of Hawai'i residents in vog-exposed and -unexposed communities was surveyed (Longo et al., 2008; Longo, 2009). Those in exposed communities were found to have a significantly increased prevalence of cough, phlegm, sinus congestion, rhinorrhoea, wheezing, eye irritation, and bronchitis than those in unexposed communities. Following the increase in SO2 flux from Kīlauea's summit in 2008, Longo (2013) reassessed the vog-related health impacts on the residents of the Island of Hawai'i. The magnitude of cardio-respiratory issues in vog-exposed communities was found to have increased as compared to 2004 (Longo et al., 2008), with the risk factor of acute cardiac events in persons aged >50 years increased by 12 % (Longo, 2013). A study by Tam et al. (2016) investigated the effects of vog on the respiratory health of school children across Hawai'i, finding that chronic exposure to vog was associated with increased prevalence of cough and potential decrease in lung function, but not with the prevalence of asthma or bronchitis. The unprecedented emission rates of the 2018 LERZ eruption has presented a continued motivation to further characterize the severity and distribution of volcanic air pollution during elevated volcanic activity.
The 2018 Kīlauea LERZ eruption provided a unique opportunity to study the impacts arising from a large low-altitude cloud rich in SO2 in a populated and well-instrumented part of the world. Here we used open-access data from a network of reference-grade instruments in populated areas around the Island of Hawai'i to determine the severity of SO2 and PM2.5 impact on air quality from the LERZ eruption. We compare air quality during the LERZ eruption to that from a lower emission period, defined to be January 2007 to December 2017 for SO2 and January 2010 to December 2017 for PM2.5. We examine a network of community-operated PM2.5 instruments and compare their measurements to those from reference-grade instruments. From these data, we demonstrate that SO2 and PM2.5 mass concentrations during the 2018 LERZ eruption in selected communities around the island were of a higher magnitude than during volcanic activity from Kīlauea during 2007 to 2017.
Hawai'i Department of Health (HDOH) ambient air quality stations continuously monitor SO2 and PM2.5 mass concentrations around the Island of Hawai'i (Figure 1A). Automated SO2 monitoring stations have been operational since 1997 in Hilo, 2005 in Kona, 2007 in Pāhala and 2010 in Ocean View. A National Park Service (NPS) ambient air quality station monitors SO2 inside of Hawai'i Volcanoes National Park at the Volcano Observatory. PM2.5 has been autonomously monitored since 2005 in Mountain View and Kona, 2008 in Hilo and Pāhala and since 2010 in Ocean View.
SO2 is measured by a pulsed fluorescence spectroscopy analyzer (model 43i manufactured by Thermo Scientific) that is designated by the EPA for measurements in the range of 0–1,000 ppb, with a lower detectable SO2 limit of 0.5 ppb and a precision of 1 ppb (Thermo Scientific 2010; EPA, 2016). FEM-designated instruments (Forum for Environmental Measurements), such as this SO2 analyzer, promote consistency in measurements and laboratory conditions ensuring that the instruments are of reference-grade quality (EPA, 2016). Following EPA regulations, the analyzers undergo in-situ calibration checks weekly, with a multi-point calibration run every 6 months. PM2.5 mass concentrations are measured by a Beta Attenuation Monitor (BAM) with a 60-min sampling rate. The BAM instrument (model BAM-1020, manufactured by Met One Instruments) is FEM-designated for measurements of particles in the size range of 0–1,000 μm (with PM2.5 being a small subset of the measured particle size range), with a resolution of 1 μm particle diameter and a lower detection limit of 4 μg/m3 (MetOne 2008; EPA, 2016). The BAM instruments undergo calibration and auditing every 6 months. Permanent HDOH ambient air quality stations are kept in air-conditioned enclosures to maintain long-term stability. Data from the air quality stations are streamed in near-real time to the HDOH website, which is open-access and publicly-available (HDOH, 2019).
Data from the HDOH and NPS station networks used in this study have been categorized into regions for the purpose of data analysis. The western region includes Kona HDOH station on the west coast of the Island of Hawai'i. The southern region includes Volcano Observatory NPS station and Pāhala and Ocean View HDOH stations. The eastern region includes the Hilo and Mountain View HDOH stations. We compare the HDOH and NPS SO2 timeseries data against the European Commission 24-h air quality threshold (125 μg/m3) and the Hawai'i 24-h ambient air quality standard (366 μg/m3). The HDOH PM2.5 timeseries data is compared to the World Health Organization 24-h exposure limit (25 μg/m3) and the Environmental Protection Agency NAAQS 24-h limit (35 μg/m3).
PurpleAir (Utah, USA) instruments are low-cost (approximately $250 per unit) particulate sensors that are purchased and operated by individuals and provide open access data online (PurpleAir, 2019). PurpleAir instruments contain Plantower PMS5003 nephelometer sensors, which use a small fan to draw air through a laser-induced light, and a photo-diode detector converts 90°-scattered light into a voltage pulse (Kelly et al., 2017). PMS5003 sensors have a 10 s response time and detect particles between 0.3 and 10 μm in diameter (Kelly et al., 2017; Sayahi et al., 2019). The maximum consistency error of the sensors is stated by the manufacturer to be ± 10 μg/m3 between 0 and 100 μg/m3 (Plantower, 2016). The instruments are factory calibrated prior to sale (PurpleAir, 2019). PM mass concentration measurements are calculated using an atmospheric calibration factor, details of which are not provided by the manufacturer (Kelly et al., 2017; Zheng et al., 2018; Sayahi et al., 2019). PurpleAir instruments contain two Plantower PMS5003 sensors mounted in one housing, allowing self-consistency checks to alert when significant differences are reported between the internal sensors. An ESP8266 wireless chip is included in PurpleAir instruments to upload data via WiFi to an online cloud database, which is open-access (Sayahi et al., 2019).
Prior to the 2018 LERZ eruption, six community-operated PurpleAir instruments were located on the Island of Hawai'i, three of which were in the Kona area in the western region. Installation dates ranged from August 2017 to February 2018. Following the onset of the LERZ eruption and island-wide increase in atmospheric pollutants, the number of PurpleAir instruments increased, with a further twenty instruments installed across the western region of the island over the course of May to July 2018.
We carried out fieldwork during the 2018 LERZ eruption and co-located PurpleAir instruments with HDOH ambient air quality stations at Kona and Ocean View (Figure 1A). Two PurpleAir instruments were installed at the Kona station, one from the fifth of June to September 2018 and a second from the nineteenth of July to the third of August, and one PurpleAir instrument was installed at the Ocean View station from the nineteenth of July to the third of August 2018. The PurpleAir instruments were installed close to the inlet for the BAM instruments, on the roofs of the air quality shelters and away from obstructions. Other than the coordinates of the instrument, no environmental information is provided in association with the PurpleAir instruments. For this reason, measurement uncertainty arising from factors such as installation in proximity to potential contamination sources in community-operated PurpleAir instruments should be considered significantly higher than those placed in optimal conditions near HDOH stations. Community-operated PurpleAir instruments were selected for analysis along the region highlighted in blue in Figure 1A.
During 2007–2017, the west-coast city of Kailua-Kona was commonly exposed to low mass concentrations of SO2, with a maximum recorded 24-h-mean mass concentration of 79 μg/m3 recorded at the Kona HDOH station (Table 1). SO2 air pollution in Kona increased with the onset of summit activity in 2008 (2008–2017 average mass concentrations were 7.7 μg/m3 compared to 3 μg/m3 in 2007) (Figure 2B). During 2007–2017 there were no 24-h periods where SO2 mean mass concentrations at the HDOH Kona station exceeded Hawai'i or EC recommended thresholds (Figure 2B).
Kona experienced elevated SO2 mass concentrations during the 2018 LERZ eruption, with a peak 24-h-mean mass concentration of 136 μg/m3 measured at the Kona station. During the 2018 LERZ, SO2 mass concentrations in Kona did not exceed the Hawai'i SO2 threshold of 366 μg/m3 (Figure 2B), but did exceed the 125 μg/m3 EC threshold on one occasion (Table 1).
Following initiation of Kīlauea's summit activity in 2008, the HDOH-operated Pāhala station and the NPS-operated Volcano Observatory station routinely recorded high concentrations of SO2 (Figure 2C), with SO2 mass concentrations exceeding the Hawai'i 366 μg/m3 24-h-mean threshold 0.7 % of the time at both Volcano Observatory (twenty-eight exceedance events) and Pāhala (thirty exceedance events) (Table 1). The maximum 24-h-mean mass concentration recorded by the NPS station at Volcano Observatory during 2007–2017 was 1,068 μg/m3, and by the HDOH station in Pāhala was 776 μg/m3. The Ocean View HDOH station is located farther to the south-west than Pāhala and Volcano Observatory, at a greater distance from Kīlauea's summit and the ERZ (Figure 1A). During the period 2010–2017, SO2 mass concentrations recorded at Ocean View exceeded the Hawai'i 24-h-mean threshold 0.1 % of the time (three exceedance events). The maximum 24-h-mean mass concentration recorded at Ocean View was 403 μg/m3, significantly lower than measured at Volcano Observatory and Pāhala (Table 1).
In comparison, during the 3-months of the 2018 LERZ eruption, SO2 mass concentrations exceeded the Hawai'i 366 μg/m3 threshold 2.1 % of the time at Volcano Observatory (two exceedance events), 5.3 % of the time at Pāhala (five exceedance events) and 4.2 % at Ocean View (four exceedance events). Maximum 24-h-mean mass concentrations at Volcano Observatory and Pāhala were lower than those measured during 2008–2017 (450 and 555 μg/m3, respectively), but the relative frequency of exceedance events increased (Table 1). During the 2018 LERZ eruption, the Ocean View station recorded a peak 24-h-mean mass concentration of 728 μg/m3, almost double the previous peak measurement of 403 μg/m3 recorded at that station in January 2016.
During the period 2007–2017, SO2 mass concentrations in Hilo followed a distinct seasonality (Figure 2D). Peak SO2 mass concentrations were commonly observed in Hilo in November to March (average monthly concentration of 11 μg/m3) with low mass concentrations in the intervening months of April to October (average monthly mass concentration of 3.6 μg/m3, as calculated from 2007 to 2017) (Figure 2D). Exceedances of the EC 24-h-mean threshold (125 μg/m3) rarely occurred outside this peak season. In the period 2007–2017 there were twenty-one exceedance events during November to March, compared with just three between April to October. The seasonal variations in SO2 mass concentrations observed in Hilo can be explained by the strong prevalence of northeasterly trade winds during April to October (Wyrtki and Meyers, 1976) (Figure 1A). During these months, emissions from Kīlauea's summit and the ERZ were dispersed predominantly to the south-west of the Island. The trade winds weaken between November to March, allowing SO2 to be dispersed to the east of the island (Mannino et al., 1996; Michaud et al., 2004; Elias and Sutton, 2017).
During the third of May to fourth of August LERZ eruption, the HDOH station in Hilo recorded a maximum 24-h-mean mass concentration of 144 μg/m3, which was recorded on the twenty-first of June (Table 1), and was the only exceedance of the EC 24-h-mean threshold during the 3-month eruption. The SO2 mass concentrations measured during the LERZ eruption were lower than the average measurements during the 2007–2017 period. SO2 mass concentrations in Hilo are usually low during the months when the LERZ eruption occurred. Nevertheless, during the 2018 LERZ eruption, SO2 mass concentrations in Hilo rose significantly above the average for the season (average 24-h-mean SO2 mass concentration during 2018 LERZ eruption was 6.9 μg/m3, in comparison to the usual seasonal average of 3.6 μg/m3).
In the period 2010–2017, PM2.5 recorded by HDOH Kona station never exceeded the EPA 24-h-mean threshold of 35 μg/m3 (Figure 3A). The WHO 24-h-mean guideline of 25 μg/m3 was exceeded 1.2 % of the time at the Kona site (33 exceedance events). The maximum PM2.5 24-h-mean mass concentrations was 33 μg/m3, recorded in April 2016 (Table 1).
Figure 3. PM2.5 mass concentrations relative to health guidelines in select populated areas of Island of Hawai'i. (A–C) Concentrations of PM2.5 (24-h mean) in populated areas from 2010 to the end of 2018. Solid red line: EPA Federal Standard air quality threshold (24-h mean of 35 μg/m3); dashed orange line: World Health Organization (WHO) air quality guideline (24-h mean of 25 μg/m3); blue highlight: 2018 LERZ eruption duration.
During the 2018 LERZ eruption, PM2.5 exceeded the 24-h-mean 35 μg/m3 EPA threshold 8.4 % of the time at the Kona station (eight exceedance events). The lower guideline of 25 μg/m3 established by the WHO was exceeded 34.7 % of the time at the Kona site (33 exceedance events). The maximum PM2.5 24-h-mean mass concentration at the site was recorded as 59 μg/m3 on the twenty-ninth of May 2018.
During the period 2010–2017, the southern region of the Island of Hawai'i experienced variable levels of PM2.5 (Figure 3B). The maximum PM2.5 24-h-mean mass concentration recorded in Ocean View was 42 μg/m3, recorded in March 2016. In Pāhala the maximum recorded PM2.5 24-h-mean mass concentration was 97 μg/m3, recorded on the eighteenth of June 2012, and coincident with two brush fires in the vicinity of Pāhala which burned approximately 5,600 acres (Hawai'i Emergency Management Agency, 2018). The EPA 35 μg/m3 PM2.5 threshold was exceeded 0.1 % of the time at both Ocean View and Pāhala (four exceedance events and two exceedance events, respectively). The lower PM2.5 guideline of 25 μg/m3 established by the WHO was exceeded 0.9 % of the time at Ocean View (25 exceedance events) and 0.1 % of the time at Pāhala (three exceedance events).
Mean PM2.5 mass concentrations in Pāhala during the 2018 LERZ eruption were higher than the 2010–2017 average (10 μg/m3 with respect to 6 μg/m3) (Table 1), however there were no 24-h periods which exceeded either the EPA or WHO 24-h-mean thresholds. During the 2018 LERZ eruption, PM2.5 recorded in Ocean View exceeded the 35 μg/m3 EPA threshold 10.5 % of the time (ten exceedance events) and exceeded the WHO 24-h-mean guideline 46.3 % of the time (44 exceedance events). In mid-June 2018, the Ocean View HDOH station recorded 3 consecutive days where 24-h-mean mass concentrations exceeded 35 μg/m3, unprecedented in the period 2010–2017. The maximum 24-h-mean mass concentration recorded in Ocean View during the 2018 LERZ eruption was 56 μg/m3, somewhat higher than the peak 24-h-mean mass concentration recorded at Ocean View in 2016 (42 μg/m3).
HDOH stations in the eastern region of the Island of Hawai'i recorded variable levels of PM2.5 during 2010–2017 (Figure 3C). The maximum PM2.5 24-h-mean mass concentration in Mountain View was 35 μg/m3, which was recorded in December 2015, and was the only exceedance of the 35 μg/m3 24-h-mean EPA threshold during the period 2010–2017 (Figure 3C). The lower PM2.5 24-h-mean guideline of 25 μg/m3 established by the WHO was exceeded 0.1 % of the time at Mountain View (three exceedance events).
During the 2018 LERZ eruption, PM2.5 mass concentrations in Mountain View were higher than the average mass concentrations for 2010–2017 (7 and 4 μg/m3, respectively) (Table 1). However, during the 2018 LERZ eruption, PM2.5 mass concentrations did not exceed either the EPA threshold of 35 μg/m3 or the WHO guideline of 25 μg/m3.
Time series of SO2 mass concentrations were analyzed in five populated areas on the Island of Hawai'i; Hilo, Volcano Observatory, Pāhala, Ocean View and Kona, for the period 2007–2018 (Figure 2). Time series of PM2.5 were analyzed in Hilo, Mountain View, Pāhala, Ocean View, and Kona for the duration 2010–2018 (Figure 3). Significant escalations in emissions from Kīlauea volcano can be identified (Figure 2A), which were registered by the air quality monitoring instruments around the Island of Hawai'i (Table 1, section 4.1 and 4.2).
High mass concentrations of SO2 and PM2.5 generally occurred in the southern and western parts of the Island of Hawai'i. Prevailing trade winds from the north-east dispersed SO2 emissions from Kīlauea volcano toward communities in the south and west of the island, as reported in previous studies (Longo et al., 2005, 2008; Michaud et al., 2007; Tam et al., 2016). During the 2018 LERZ eruption, the EPA 24-h-mean threshold for PM2.5 (35 μg/m3) and the Hawai'i threshold for SO2 (366 μg/m3) were exceeded in the south and west of the island (Figure 4). HDOH stations in the south of the island, 35–100 km away from Fissure 8, recorded 24-h events where SO2 exceeded Hawai'i thresholds (note that there were no HDOH or NPS permanent monitoring stations for SO2 in proximal location to the 2018 LERZ eruption site), but PM2.5 EPA exceedance events only occurred at HDOH stations 100 km or further away from Fissure 8 (Figure 4). This spatial variance between distribution of PM2.5 and SO2 is well-documented and thought to reflect the timescale of oxidation of sulfur dioxide gas into sulfate aerosol during dispersion (Cadle et al., 1971; Stockwell and Calvert, 1983; Porter et al., 2002; Ilyinskaya et al., 2017).
Figure 4. Exceedances of (A) 24-h Hawai'i Standard for SO2 during 2018 LERZ eruption and (B) EPA threshold for PM2.5 during 2018 LERZ eruption. Note: data were unavailable for PM2.5 at Volcano Observatory and SO2 at Mountain View.
Volcanic emissions at source commonly consist of a mixture of silicate ash particles, various gases and non-silicate aerosol (Oppenheimer and McGonigle, 2004; von Glasow et al., 2009; Langmann, 2014). The lifetime of SO2 in the lower troposphere is generally considered to be on the order of 1–3 days to a week (Allen et al., 2002; Rotstayn and Lohmann, 2002; Pfeffer et al., 2006a; Pattantyus et al., 2018), the rate of conversion depending on relative humidity and temperature, the availability of oxidants, and interaction with cloud or fog (Saxena and Seigneur, 1987; Oppenheimer et al., 1998). However, the SO2 oxidation pathways in a volcanic cloud are not necessarily the same as under background conditions (Galeazzo et al., 2018). Through a variety of reaction pathways (including oxidation with the hydroxyl radical, OH, and with hydrogen peroxide, H2O2, and O3), SO2 in volcanic clouds is gradually converted to sulfate aerosol (Stockwell and Calvert, 1983; Allen et al., 2002), which is a dominant component of volcanic PM2.5 (Tam et al., 2016; Pattantyus et al., 2018). The conversion rate of SO2 to sulfate aerosol is important for estimating the potential hazard of volcanic PM2.5 to human health and the downwind environments (Kroll et al., 2015).
HDOH stations measure the ambient air, which contains SO2 and PM2.5 derived from anthropogenic sources as well as natural non-volcanic and volcanic sources. In order to determine the influence of the volcanic eruption on the measured SO2 and aerosol abundances, it is first necessary to calculate the volcanic component of the HDOH measurements. During the 2018 LERZ eruption, Fissure 8 was the dominant source of volcanic SO2 emissions on the island, and following the decline of the eruption, the SO2 and PM2.5 mass concentrations decreased to below pre-LERZ eruption levels at all HDOH stations analyzed in this study (Figures 2, 3). The mass of pollutants recorded at HDOH stations during this post-LERZ eruption period (mid-August 2018 to the first of February 2019) are therefore used to define the background abundances arising from all other non-volcanic sources. During this time there was some SO2 emitted from Kīlauea's summit but at the lowest rate measured in decades at 0.1 kt/year (Nadeau et al., 2019). The volcanic component of the HDOH measurements was calculated by subtracting the average PM2.5 and SO2 mass concentration following the end of the 2018 LERZ eruption for each station from the mass concentrations measured during the LERZ eruption, to estimate the volcanogenic component. The sulfate aerosol component within the volcanic PM2.5 mass concentration was then estimated to be in the range 77–92 %, following the methods of Mather et al. (2012) and Kroll et al. (2015), of PM2.5 composition from Kīlauea.
Estimating the conversion rate of SO2 to sulfate from SO2 and SO42– datasets is not straightforward because several processes can occur simultaneously, including SO2 oxidation to sulfate, dispersion-dilution and deposition of SO2 and/or SO42– to the surface.
Here, a first-order decay constant for SO2 is estimated by the relationship between volcanic components of SO2 and SO42–, as follows;
where Sgas is the sulfur component of the volcanic SO2 mass concentration (μg/m3), Stotal is the sum of sulfur components of the volcanic SO42– and SO2 mass concentrations (μg/m3), t is the age of the volcanic cloud (seconds) and −k is a first-order decay constant.
The age of the volcanic cloud is here considered to be the time between emission of the cloud at the LERZ eruption source point and subsequent measurement at the HDOH station. From back-trajectory HYSPLIT simulations run between the HDOH ambient air quality stations and Fissure 8, the average age of the emissions and dispersal distance was calculated for dates between the eighteenth of July to the second of August 2018 (Ilyinskaya et al., in preparation). An estimate of the average first-order decay constant from our data-set is indicated in Figure 5. Although there is considerable scatter in the data, a broad trend of decreasing S fraction in the gas phase is apparent and a linear fit allows us to estimate a first-order rate constant of 3.8 × 10–6 s–1 with a 95 % confidence interval of ± 1.26 × 10–6 s–1. This first-order decay constant for SO2 relative to total sulfur can represent an estimate of the average rate of SO2 oxidation to sulfate only if negligible sulfur deposition has occurred. Nevertheless, our value is similar to the SO2 oxidation rate calculated by Kroll et al. (2015) from direct measurements of sulfur in gas and particle phase in Kīlauea's emission cloud from the summit to Pāhala. Kroll et al. (2015) identified a diurnal cycle in measured sulfate as a fraction of total sulfur, from which they calculated a noontime instantaneous SO2 oxidation rate of 2.4 × 10–6 s–1.
Figure 5. Relationship between the age of the volcanic cloud and the volcanic sulfur components measured at Pāhala, Ocean View and Kona HDOH ambient air quality stations. Sgas is the sulfur component of the volcanic SO2 mass concentration, Stotal is the sum of sulfur components of the volcanic SO42– and SO2 mass concentrations. Age is derived from HYSPLIT back-trajectory simulations (Ilyinskaya et al., in preparation). Y axis error bars indicate range of SO42– component in volcanic PM2.5 composition from Kīlauea. X axis error bars indicate one standard deviation of HYSPLIT back-trajectory results specific to Kona, Ocean View and Pāhala. Correlation trendline based on the data points, with a first-order decay constant of 3.8 x 10–6 s–1 with a 95 % confidence interval of ± 1.26 x 10–6 s–1.
A subset of the community-operated PurpleAir instruments on the Island of Hawai'i were selected for intercomparison with the established institutional data-sets across the western region of the island. The low-cost and portable nature of the PurpleAir instruments (Figure 6F) facilitated installation of a monitoring network across the western region (Figure 6A), with a high spatial resolution of measurements in comparison to the locations of HDOH ambient air quality sites (Figure 6A). This can be advantageous to capture the effects of local topographic and meteorological factors, which may influence dispersion of and deposition from volcanic plumes. Mass concentrations from PurpleAir instruments and HDOH PM2.5 instruments during the course of the 2018 LERZ eruption are presented in Figures 6B–E.
Figure 6. Hawai'i Department of Health ambient air quality stations and community-operated PurpleAir instruments along the western region of the Island of Hawai'i selected for analysis in this study. (A) Distribution of publicly-owned PurpleAir instruments, indicated by blue stars with reference numbers, distribution of Kona and Ocean View HDOH stations indicated by green circles. PurpleAir instruments within 10 km radius of Kona HDOH station indicated by dashed yellow circle. (B–D) 24-h average mass concentration measurements from 20 PurpleAir instruments during the 2018 LERZ eruption; (E) 24-h average PM2.5 from BAM instruments at Kona and Ocean View HDOH ambient air quality stations during the 2018 LERZ eruption. (F) Size and morphology of the PurpleAir instruments, seen from the front (left image) and side (right image). Photos by kind permission of PurpleAir LLC.
Small differences were found between individual PurpleAir instruments in the same location. Two PurpleAir instruments (Figure 6A, PurpleAir references 10 and 11) were co-located at the Kona HDOH station, and the PurpleAir instruments ran together for 16 days. During this time, the maximum absolute differences in 24-h average measurements between the PurpleAir instruments was 2.3 μg/m3; which was 6 % of the total measured concentration. Correlation between the two PurpleAir instruments was very strong (Pearson's r = 0.99). Similar results were found by Malings et al. (2019) with co-location of nine PurpleAir instruments at a site in Pennsylvania for a period of 66 days (Pearson's r > 0.9). The high correlation between co-located individual PurpleAir instruments indicates high standardization. With this being the case, relative PM2.5 mass concentrations measured by PurpleAir instruments over a wider geographical area should be comparably reliable.
Three PurpleAir instruments were co-located with HDOH PM2.5 instruments (BAM) to determine the accuracy of PurpleAir instrument PM2.5 measurements in relation to reference-grade instruments (Figure 6A, PurpleAir references 10, 11, and 20). The co-located PurpleAir measurements correlated well with the BAM measurements, with Pearson's r values of 0.99, 0.97, and 0.91 (Figures 7A,B). However, PurpleAir instruments did record higher mass concentrations of PM2.5 in comparison to the BAM analyzers (Figures 7A,B). PurpleAir reference 10 (co-located with Kona HDOH station) (Figure 6A) recorded the greatest measurement off-set, with up to 40 % higher mass concentrations of PM2.5 relative to the Kona BAM analyzer measurements (Figure 7A). This trend was also found when the community-operated PurpleAir instruments across the western region of the island were compared to the BAM at Kona HDOH station (Figure 7C), with strong correlation between PurpleAir instruments and BAM (Pearson's r = 0.92) but an average 30 % higher mass concentrations measured by PurpleAir instruments within 10 km of the Kona BAM instrument. Previous testing of PurpleAir instruments in Pennsylvania and California has yielded similar findings (AQ-SPEC, 2017; Malings et al., 2019), with over-estimation of PM2.5 mass concentrations measured by PurpleAir instruments relative to BAM reference-grade analyzers.
Figure 7. PurpleAir and HDOH PM2.5 comparison. (A) Two PurpleAir instruments co-located with Kona HDOH station. (B) One PurpleAir instrument co-located with Ocean View HDOH station. (C) Comparison of all PurpleAir instruments along the western region of the Island of Hawai'i compared with Kona HDOH measurements. PurpleAir instruments within 10 km of Kona HDOH indicated by dark gray stars, PurpleAir instruments at greater distances are indicated by light gray circles. Linear regression and 95 % confidence interval fit to instruments <10 km from Kona HDOH ambient air quality station. Measurement periods vary with earliest PurpleAir data from August 2017 and most recent from January 2019. (A,B) PurpleAir error bars show measurement variations between the two sensors within a single PurpleAir instrument, and therefore indicate the minimum error.
Some discrepancy between PurpleAir and BAM measurements may be expected, as it is well-known that low-cost instruments measuring PM2.5 with light scattering methods have not historically agreed with measurements obtained from reference grade instruments with different operating principles (Watson et al., 1998; Wilson et al., 2002; Chow et al., 2008; Burkart et al., 2010). In comparison with sensors of other operating principles, light-scattering optical particle sensors have been shown to suffer effects of relative humidity (Wang et al., 2015; Crilley et al., 2018), since the operating principle relies on indirect measurement of particle size and shape based on scattered light, and an assumed particle shape and refractive index. Conversely, BAM instruments measure direct changes in aerosol mass concentrations based on the loss of electrons on a filter which the aerosol has been deposited on (Watson et al., 1998; Manikonda et al., 2016), and so are not influenced by the hygroscopic growth of individual particles. Zheng et al. (2018) analyzed the performance of Plantower PMS3003 (an earlier version of the Plantower PMS5003 housed in PurpleAir instruments used in this study) against a reference grade scattered light spectrometer (with good correlation of R2 = 0.8) and a BAM instrument (with lower correlation of R2 = 0.5). They concluded that a likely explanation contributing to the discrepancy is the potential for hygroscopic growth of aerosol particles due to ambient humidity, which alters the light-scattering properties of the aerosol and therefore the measurement made by the optical sensor (Watson et al., 1998; Cabada et al., 2004; Spinetti and Buongiorno, 2007; Jayaratne et al., 2018). In this instance, Zheng et al. (2018) found that the low-cost light-scattering sensor correlated best with the reference-grade instrument of the same operating principle, finding a lower correlation against the reference-grade instrument operating on principles other than light-scattering. The PurpleAir instrument over-estimation of PM2.5 relative to the BAM reference-grade instrument may therefore be due to influences of humidity acting on the light scattering (Zheng et al., 2018).
An additional consideration in explaining the overestimation of PM2.5 by the PurpleAir instruments relative to the reference-grade BAM is the particle density of the measured particulates. The Plantower PMS5003 sensors contained within the PurpleAir instruments provide mass concentration measurements which are calculated by an unreported atmospheric calibration factor (Zheng et al., 2018). We assume that this calibration factor uses an average particle density, likely similar to that for an urban environment, such as 1.65 g cm–3 (Pitz et al., 2003; Liu et al., 2015; Crilley et al., 2018). If the Plantower PMS5003 sensor measures ambient air with a particle density dissimilar to the average particle density used in the atmospheric calibration factor, the resulting sensor output would be biased. For example, introduction of volcanic aerosol, primarily sulfate with an average particle density of 1.77 g cm–3 (Sarangi et al., 2016), into the air measured by the sensor could result in sensor output bias as a result of dissimilarity between the real and assumed particle density.
Despite the PM2.5 over-estimation, the strong correlation between the PurpleAir and BAM instruments indicates that the PurpleAir instruments provided qualitatively valuable measurements of the atmospheric conditions. The high degree of intra-instrument performance, similar to findings by Malings et al. (2019), indicates that the PurpleAir instruments are reliable for determining relative variations in PM2.5. The dense network of instruments with a high spatial resolution along the western region of Hawai'i during the 2018 LERZ eruption gives a good indication of the relative amounts of PM2.5 across the region, at a finer spatial resolution than available from the sparsely-located BAM instruments (Figure 6A). Low-cost community-operated networks, such as the PurpleAir instruments across the western region of Hawai'i during the 2018 LERZ eruption, can therefore be invaluable in providing insights into smaller-scale heterogeneities in air quality across a regional area at a scale inaccessible by the usually more disperse reference-grade instruments (Figures 6B–E). Additionally, as far as the authors are aware, this is the first validation of PurpleAir instruments in a volcanic environment. Their strong correlation to the BAM instruments along the west coast of the Island of Hawai'i during the 2018 LERZ eruption indicates that they are suitable for augmenting reference-grade instrument networks in periods of volcanic unrest. Considering that these instruments also provide a source of open-access data to the public, they present an opportunity to improve community awareness and inclusion of the general public in hazard assessment of downwind volcanic PM2.5 air pollution.
Kīlauea's 2018 eruption was the largest LERZ eruption in the last two centuries. SO2 emissions reached a monthly average of 200 kt/day during June (Kern et al. 2019), significantly exceeding emissions from Kīlauea during 2008–2017, which averaged 5–6 kt/day (Eguchi et al., 2011; Beirle et al., 2014; Carn et al., 2016; Elias et al., 2018). During the 2018 LERZ eruption, SO2 mass concentrations exceeding the Hawai'i 24-h-mean threshold (366 μg/m3) primarily occurred in the south of the island, at Volcano Observatory and in Pāhala and Ocean View (2.1, 5.3, and 4.2 % of the time during the 3-month long eruption, respectively). SO2 mass concentrations were elevated at the HDOH Kona station (average 24-h-mean mass concentration of 39 μg/m3 during the LERZ eruption, relative to 7 μg/m3 during 2007–2017), but mass concentrations were highest in Ocean View, Pāhala and at Volcano Observatory (average 24-h-mean mass concentrations of 117, 129, and 56 μg/m3, respectively). The Hawai'i 24-h-mean threshold was exceeded five times in Pāhala and twice at Volcano Observatory, but peak mass concentrations did not exceed those from the period 2007–2017. In Ocean View, the Hawai'i 24-h-mean threshold was exceeded four times and 24-h-mean mass concentrations peaked at 728 μg/m3, almost double the previous peak measurement of 403 μg/m3 recorded at that station in January 2016.
PM2.5 mass concentrations recorded at HDOH stations around the island from 2010 to 2017 rarely exceeded the EPA 24-h-mean threshold of 35 μg/m3 (0.1 % of the time at both Ocean View and Pāhala). The lower 24-h-mean PM2.5 limit (25 μg/m3) set by the World Health Organization was exceeded with greater frequency, particularly in Kona and Ocean View (1.2 and 0.9 % of the time, respectively). During the 2018 LERZ eruption, PM2.5 air pollution was significantly higher than 2010–2017 levels in Kona and Ocean View, exceeding WHO guidelines 34.7 and 46.3 % of the time, respectively. Peak 24-h-mean mass concentrations in Ocean View were recorded at 56 μg/m3, and 3 consecutive days in June were recorded with mean 24-h mass concentrations exceeding 35 μg/m3. The Kona HDOH stations recorded eight 24-h periods which exceeded EPA thresholds, unprecedented in the 2010–2017 period.
Following the decline of the 2018 LERZ eruption, mass concentrations of both SO2 and PM2.5 measured at the HDOH stations decreased to below pre-LERZ eruption levels, indicating that a large proportion of the air quality anomalies measured during the eruption were volcanogenic. The post-LERZ HDOH measurements are here assumed to be representative of the background (non-volcanically-perturbed) atmosphere, and subtracting these abundances from those recorded during the LERZ eruption provides an estimate of the purely volcanogenic PM2.5 and SO2. The sulfate aerosol component within the volcanic PM2.5 is calculated as between 77 and 92 %, following Mather et al. (2012). HYSPLIT back-trajectory simulations provide an estimate of emission age following dispersion from source to measurement point (Ilyinskaya et al., in preparation), and a first-order SO2 decay constant is estimated at 3.8 × 10–6 s–1.
Community-operated PurpleAir instruments provided a high spatial resolution network across the western region of the island, informing the public regarding PM2.5 mass concentrations in their locality. Low measurement variability (Pearson's r = 0.99) was found between co-located individual PurpleAir instruments, indicating a high level of intra-instrument performance. Observations recorded by co-located PurpleAir and BAM instruments correlated well (Pearson's r = 0.99, 0.97, and 0.94), but PurpleAir instruments were found to overestimate the PM2.5 mass concentration by up to 40 %, relative to the BAM instrument. This likely reflects inherent differences in instrument operating principles and may be associated with changes in optical-properties of aerosol arising from hygroscopic growth in ambient humidity. Nevertheless, the PurpleAir instruments are suitable for providing a low-cost network to augment reference-grade instruments, and contribute an open-access source of readily-available information to the public leading to development of community awareness toward air quality.
This study has assessed the impacts to air quality in downwind communities around the Island of Hawai'i from 2007 to 2018. Spatial variability of air quality around the island during the 2018 LERZ eruption was comparable to patterns identified over the previous decade, but PM2.5 and SO2 pollution levels resulting from the 2018 LERZ eruption were significantly higher in western and southern regions of the island. A study of the potential health burden of these significant impacts on air quality might further illuminate aspects of the dose-response to volcanogenic emissions in addition to the impacts of public health protection measures put in place during the eruption.
The datasets analyzed for this study can be found in the Hawai'i Department of Health Ambient Air Quality Data repository [http://health.hawaii.gov/cab/hawaii-ambient-air-quality-data/], and in the PurpleAir sensor list repository [https://www.purpleair.com/sensorlist].
RW performed the data analysis and wrote the original draft. EI, AS, TR, MP, and TM contributed to data interpretation. All co-authors contributed to draft review and editing.
RW is funded by the Leeds-York Natural Environment Research Council (NERC) Doctoral Training Partnership (DTP) NE/L002574/1, in CASE partnership with the Icelandic Meteorological Office. RW, EI, EM, PW, and EL were funded for campaign-fieldwork by the Centre for the Observation and Modeling of Earthquakes, Volcanoes and Tectonics (COMET) grant COME3001. The authors also acknowledge support to EI from the NERC urgency grant NE/M021130/1 Source and longevity of sulfur in Icelandic flood basalt eruption plumes and GCRF NE/P015271/1 and NE/R009465/1 Unseen but not unfelt: resilience to persistent volcanic emissions (UNRESP). We acknowledge support from the NERC ESS DTP to PW, EPSRC CASE funding to EM, Alfred P. Sloan Foundation through support of the Deep Carbon Observatory to ME and EL and Leverhulme Early Career Fellowship to EL, and ANR Projet de Recherche Collaborative VOLC-HAL-CLIM ANR-18-CE01-0018 and Orléans Labex Voltaire ANR-10-LABX-100-0 to TR. CO acknowledges support from NERC grant NE/N009312/1, and EI, TM, ME, and CO further acknowledge support from NERC grant NE/S00436X/1: Volcanic plume understanding and forecasting: Integrating remote-sensing, in-situ observations and models (V-PLUS).
AD was employed by company PurpleAir LLC.
The remaining authors declare that the research was conducted in the absence of any commercial or financial relationships that could be construed as a potential conflict of interest.
We thank James Ciszewski at the Hawai'i Department of Health for assistance with fieldwork and data-gathering; Lacey Holland and Steven Businger at the University of Hawai'i at Manoa for volcanic emissions trajectory and HYSPLIT discussions; and the National Park Service and Hawai'i Volcanoes National Park for their contributions.
Any use of trade, firm, or product names is for descriptive purposes only and does not imply endorsement by the U.S. Government.
Allen, A., Oppenheimer, C., Ferm, M., Baxter, P., Horrocks, L., Galle, B., et al. (2002). Primary sulfate aerosol and associated emissions from Masaya Volcano, Nicaragua. J. Geophys. Res. Atmos. 107, ACH5–ACH8. doi: 10.1029/2002JD002120
Andronico, D., and Del Carlo, P. (2016). PM10 measurements in urban settlements after lava fountain episodes at Mt. Etna, Italy: pilot test to assess volcanic ash hazard to human health. Nat. Hazards Earth Syst. Sci. 16, 29–40. doi: 10.5194/nhess-16-29-2016
AQ-SPEC (2017). PurpleAir PA-I Sensor Evaluation Report. Available online at: http://www.aqmd.gov/aq-spec/product/purpleair-pa-ii (accessed Feburary 13, 2019).
ATSDR (1998). Public Health Statement Sulfur Dioxide. 7446-09-5. Agency for Toxic Substances and Disease Registry.
Balmes, J. R., Fine, J. M., and Sheppard, D. (1987). Symptomatic bronchoconstriction after short-term inhalation of sulfur dioxide. Am. Rev. Respir. Dis. 136, 1117–1121. doi: 10.1164/ajrccm/136.5.1117
Barsotti, S., Andronico, D., Neri, A., Del Carlo, P., Baxter, P., Aspinall, W., et al. (2010). Quantitative assessment of volcanic ash hazards for health and infrastructure at Mt. Etna (Italy) by numerical simulation. J. Volcanol. Geotherm. Res. 192, 85–96. doi: 10.1016/j.jvolgeores.2010.02.011
Beirle, S., Hörmann, C., Penning de Vries, M., Dörner, S., Kern, C., and Wagner, T. (2014). Estimating the volcanic emission rate and atmospheric lifetime of SO2 from space: a case study for Kīlauea volcano, Hawai'i. Atmos. Chem. Phys. 14, 8309–8322. doi: 10.5194/acp-14-8309-2014
Burkart, J., Steiner, G., Reischl, G., Moshammer, H., Neuberger, M., and Hitzenberger, R. (2010). Characterizing the performance of two optical particle counters (Grimm OPC1. 108 and OPC1. 109) under urban aerosol conditions. J. Aerosol Sci. 41, 953–962. doi: 10.1016/j.jaerosci.2010.07.007
Butwin, M. K., von Löwis, S., Pfeffer, M. A., and Thorsteinsson, T. (2019). The effects of volcanic eruptions on the frequency of particulate matter suspension events in Iceland. J. Aerosol Sci. 128, 99–113. doi: 10.1016/j.jaerosci.2018.12.004
Cabada, J. C., Khlystov, A., Wittig, A. E., Pilinis, C., and Pandis, S. N. (2004). Light scattering by fine particles during the Pittsburgh Air Quality Study: measurements and modeling. J. Geophys. Res. Atmos. 109. doi: 10.1029/2003JD004155
Cadle, R., Wartburg, A., and Grahek, P. (1971). The proportion of sulfate to sulfur dioxide in Kīlauea Volcano fume. Geochim. Cosmochim. Acta 35, 503–507. doi: 10.1016/0016-7037(71)90046-9
Carn, S., Clarisse, L., and Prata, A. J. (2016). Multi-decadal satellite measurements of global volcanic degassing. J. Volcanol. Geothermal Res. 311, 99–134. doi: 10.1016/j.jvolgeores.2016.01.002
Chow, J. C., Doraiswamy, P., Watson, J. G., Chen, L.-W., Ho, S. S., and Sodeman, D. A. (2008). Advances in integrated and continuous measurements for particle mass and chemical composition. J. Air Waste Manage. Assoc. 58, 141–163. doi: 10.3155/1047-3289.58.2.141
CRI (2004). Health Effects of Project Shad Chemical Agent: Sulfur Dioxide [cas 7446-09-5]. National Academies. The Centre for Research Information.
Crilley, L. R., Shaw, M., Pound, R., Kramer, L. J., Price, R., Young, S., et al. (2018). Evaluation of a low-cost optical particle counter (Alphasense OPC-N2) for ambient air monitoring. Atmos. Meas. Tech. 11, 709–720. doi: 10.5194/amt-11-709-2018
Eguchi, K., Uno, I., Yumimoto, K., Takemura, T., Nakajima, T. Y., Uematsu, M., et al. (2011). Modulation of cloud droplets and radiation over the North Pacific by sulfate aerosol erupted from Mount Kīlauea. SOLA 7, 77–80. doi: 10.2151/sola.2011-020
Elias, T., Kern, C., Horton, K., Sutton, A. J., and Garbeil, H. (2018). Measuring SO2 emission rates at Kīlauea Volcano, Hawai'i USA, 2014-2017. Front. Earth Sci. 6:214. doi: 10.3389/feart.2018.00214
Elias, T., and Sutton, A. J. (2007). Sulfur Dioxide Emission Rates from Kīlauea Volcano, Hawai'i, an Update: 2002-2006. US Geological Survey.
Elias, T., and Sutton, A. J. (2012). Sulfur Dioxide Emission Rates From Kīlauea Volcano, Hawai'i, 2007–2010. Technical report, US Geological Survey.
Elias, T., and Sutton, A. J. (2017). Volcanic Air Pollution Hazards in Hawai'i. Technical report, US Geological Survey. Available online at: https://pubs.usgs.gov/fs/2017/3017/fs20173017.pdf
Environmental Protection Agency (2010). Primary National Ambient Air Quality Standard for Sulfur Dioxide; Final Rule. Available online at: https://www.govinfo.gov/content/pkg/FR-2010-06-22/pdf/2010-13947.pdf, 40 CFR Parts 50, 53, and 58 (accessed January 15, 2019)
Environmental Protection Agency (2013). Federal and State Ambient Air Quality Standards. Available online at: http://health.hawaii.gov/cab/files/2013/05/naaqs_jan_2013.pdf (accessed January 15, 2019)
Environmental Protection Agency (2016). List of Designated Reference and Equivalent Methods. National Exposure Research Laboratory.
European Commission (2018). Environment Air Quality Standards. Available online at: http://ec.europa.eu/environment/air/quality/standards.htm (accessed January 21, 2019)
Galeazzo, T., Bekki, S., Martin, E., Savarino, J., and Arnold, S. R. (2018). Photochemical box modelling of volcanic SO2 oxidation: isotopic constraints. Atmos. Chem. Phys. 18, 17909–17931. doi: 10.5194/acp-18-17909-2018
Halliday, T., Lynham, J., and de Paula Neto, A. (2015). Vog: Using Volcanic Eruptions to Estimate the Health Costs of Particulates and SO2. College of Social Sciences; Department of Economics.
Hansell, A., and Oppenheimer, C. (2004). Health hazards from volcanic gases: a systematic literature review. Arch. Environ. Health Int. J. 59, 628–639. doi: 10.1080/00039890409602947
Hawai'i Department of Business Economic Development and Tourism (2011). Research and Economic Analysis Division. Statistics and Data Support Branch. Hawai'i State Data Center. 2010 Census Tract Names and Their Population, Housing and Land Area for the State of Hawai'i Honolulu. Hawai'i State Data Center Report Number 2010-2.
Hawai'i Emergency Management Agency (2018). 2018 State of Hawai'i Hazard Mitigation Plan. Section 4.15; Wildfire Available online at: https://dod.hawaii.gov/hiema/files/2018/06/Draft-Section-4.15-Wildfire-Placeholder.pdf (accessed August 9, 2019)
HDOH (2019). Hawai'i Department of Health Clean Air Branch, Hawai'i Ambient Air Quality Data. Available online at: http://health.hawaii.gov/cab/hawaii-ambient-air-quality-data/ (accessed August 13, 2019)
Holgate, S. (2017). "Every breath we take: the lifelong impact of air pollution" - a call for action. Clin. Med. 17, 8–12. doi: 10.7861/clinmedicine.17-1-8
Ilyinskaya, E., Schmidt, A., Mather, T. A., Pope, F. D., Witham, C., Baxter, P., et al. (2017). Understanding the environmental impacts of large fissure eruptions: aerosol and gas emissions from the 2014–2015 Holuhraun eruption (Iceland). Earth Planet. Sci. Lett. 472, 309–322. doi: 10.1016/j.epsl.2017.05.025
Jayaratne, R., Liu, X., Thai, P., Dunbabin, M., and Morawska, L. (2018). The influence of humidity on the performance of a low-cost air particle mass sensor and the effect of atmospheric fog. Atmos. Meas. Tech. 11, 4883–4890. doi: 10.5194/amt-11-4883-2018
Kelly, K. E., Whitaker, J., Petty, A., Widmer, C., Dybwad, A., Sleeth, D., et al. (2017). Ambient and laboratory evaluation of a low-cost particulate matter sensor. Environ. Pollut. 221, 491–500. doi: 10.1016/j.envpol.2016.12.039
Kern, C., Elias, T., Nadeau, P., Lerner, A., Werner, C., Cappos, M., et al. (2019). “Sulfur dioxide emissions associated with Kīlauea Volcano's 2018 fissure eruption; V43C-0209,” in American Geophysical Union Fall Meeting.
Kroll, J. H., Cross, E. S., Hunter, J. F., Pai, S., et al. (2015). Atmospheric evolution of sulfur emissions from Kīlauea: real-time measurements of oxidation, dilution, and neutralization within a volcanic plume. Environ. Sci. Technol. 49, 4129–4137. doi: 10.1021/es506119x
Lambert, G., Le Cloarec, M., and Pennisi, M. (1988). Volcanic output of SO2 and trace metals: a new approach. Geochim. Cosmochim. Acta 52, 39–42. doi: 10.1016/0016-7037(88)90054-3
Langmann, B. (2014). On the role of climate forcing by volcanic sulphate and volcanic ash. Adv. Meteorol. 2014, 1–17. doi: 10.1155/2014/340123
Lim, S. S., Vos, T., Flaxman, A. D., Danaei, G., Shibuya, K., Adair-Rohani, H., et al. (2012). A comparative risk assessment of burden of disease and injury attributable to 67 risk factors and risk factor clusters in 21 regions, 1990–2010: a systematic analysis for the Global Burden of Disease Study 2010. Lancet 380, 2224–2260.
Liu, Z., Hu, B., Ji, D., Wang, Y., Wang, M., and Wang, Y. (2015). Diurnal and seasonal variation of the pm2.5 apparent particle density in Beijing, China. Atmos. Environ. 120, 328–338. doi: 10.1016/j.atmosenv.2015.09.005
Longo, B. M. (2009). The Kīlauea Volcano adult health study. Nurs. Res. 58, 23–31. doi: 10.1097/NNR.0b013e3181900cc5
Longo, B. M. (2013). Adverse health effects associated with increased activity at Kīlauea Volcano: a repeated population-based survey. ISRN Public Health 2013:475962. doi: 10.1155/2013/475962
Longo, B. M., Grunder, A., Chuan, R., and Rossignol, A. (2005). SO2 and fine aerosol dispersion from the Kīlauea plume, Kau district, Hawai'i, USA. Geology 33, 217–220. doi: 10.1130/G21167.1
Longo, B. M., Rossignol, A., and Green, J. B. (2008). Cardiorespiratory health effects associated with sulphurous volcanic air pollution. Public Health 122, 809–820. doi: 10.1016/j.puhe.2007.09.017
Longo, B. M., Yang, W., Green, J. B., Crosby, F. L., and Crosby, V. L. (2010). Acute health effects associated with exposure to volcanic air pollution (vog) from increased activity at Kīlauea Volcano in 2008. J. Toxicol. Environ. Health A 73, 1370–1381. doi: 10.1080/15287394.2010.497440
Loughlin, S., Aspinall, W., Vye-Brown, C., Baxter, P., Braban, C., Hort, M., et al. (2012). Large-Magnitude Fissure Eruptions in Iceland: Source Characterisation. BGS Open File Report, OR/12/098.
Malings, C., Tanzer, R., Hauryliuk, A., Saha, P. K., Robinson, A. L., Presto, A. A., et al. (2019). Fine particle mass monitoring with low-cost sensors: corrections and long-term performance evaluation. Aerosol Sci. Technol. 54, 1–40. doi: 10.1002/essoar.10500022.5
Manikonda, A., Zíková, N., Hopke, P. K., and Ferro, A. R. (2016). Laboratory assessment of low-cost PM monitors. J. Aerosol Sci. 102, 29–40. doi: 10.1016/j.jaerosci.2016.08.010
Mannino, D. M., Ruben, S., Holschuh, F. C., Holschuh, T. C., Wilson, M. D., and Holschuh, T. (1996). Emergency department visits and hospitalizations for respiratory disease on the Island of Hawai'i, 1981 to 1991. Hawai'i Med. J. 55.
Mather, T., Allen, A., Oppenheimer, C., Pyle, D., and McGonigle, A. (2003). Size-resolved characterisation of soluble ions in the particles in the tropospheric plume of Masaya volcano, Nicaragua: origins and plume processing. J. Atmos. Chem. 46, 207–237. doi: 10.1023/A:1026327502060
Mather, T., Witt, M., Pyle, D., Quayle, B., Aiuppa, A., Bagnato, E., et al. (2012). Halogens and trace metal emissions from the ongoing 2008 summit eruption of Kīlauea volcano, Hawai'i. Geochim. Cosmochim. Acta 83, 292–323. doi: 10.1016/j.gca.2011.11.029
Mather, T. A. (2015). Volcanoes and the environment: lessons for understanding Earth's past and future from studies of present-day volcanic emissions. J. Volcanol. Geothermal Res. 304, 160–179. doi: 10.1016/j.jvolgeores.2015.08.016
Michaud, J.-P., Grove, J. S., and Krupitsky, D. (2004). Emergency department visits and 'vog'-related air quality in Hilo, Hawai'i. Environ. Res. 95, 11–19. doi: 10.1016/S0013-9351(03)00122-1
Michaud, J. D., Michaud, J.-P., and Krupitsky, D. (2007). Temporal variability in SO2 exposures at Hawai'i Volcanoes National Park, USA. Environ. Geol. 52, 81–92. doi: 10.1007/s00254-006-0459-y
Nadeau, P., Elias, T., Kern, C., Lerner, A., et al. (2019). The 2018 Eruption and Beyond: A New Era of Degassing at Kīlauea Volcano. IUGG19-1696.
National Oceanic Atmospheric Administration (2019). Climate Data Online Data Tools. Available online at: https://www.ncdc.noaa.gov/cdo-web/datatools (accessed May 1, 2019)
Neal, C. A., Brantley, S. R., Antolik, L., Babb, J. L., Burgess, M., Calles, K., et al. (2019). The 2018 rift eruption and summit collapse of Kīlauea Volcano. Science 363, 367–374. doi: 10.1126/science.aav7046
Oppenheimer, C., Francis, P., and Stix, J. (1998). Depletion rates of sulfur dioxide in tropospheric volcanic plumes. Geophys. Res. Lett. 25, 2671–2674. doi: 10.1029/98GL01988
Oppenheimer, C., Kyle, P., Eisele, F., Crawford, J., Huey, G., Tanner, D., et al. (2010). Atmospheric chemistry of an Antarctic volcanic plume. J. Geophys. Res. Atmos. 115. doi: 10.1029/2009JD011910
Oppenheimer, C., and McGonigle, J. (2004). Exploiting ground-based optical sensing technologies for volcanic gas surveillance. Ann. Geophys. 47.
Patrick, M. R., Orr, T., Sutton, A. J., Tamar, E., and Swanson, D. (2013). The First Five Years of Kīlauea's Summit Eruption in Halema'uma'u Crater, 2008–2013. U.S. Geological Survey Fact Sheet 2013–3116. Available online at: https://pubs.er.usgs.gov/publication/fs20133116
Pattantyus, A. K., Businger, S., and Howell, S. G. (2018). Review of sulfur dioxide to sulfate aerosol chemistry at Kīlauea Volcano, Hawai'i. Atmos. Environ. 185, 262–271. doi: 10.1016/j.atmosenv.2018.04.055
Pfeffer, M., Langmann, B., and Graf, H.-F. (2006a). Atmospheric transport and deposition of Indonesian volcanic emissions. Atmos. Chem. Phys. 6, 2525–2537. doi: 10.5194/acp-6-2525-2006
Pfeffer, M., Rietmeijer, F., Brearley, A., and Fischer, T. P. (2006b). Electron microbeam analyses of aerosol particles from the plume of Poás Volcano, Costa Rica and comparison with equilibrium plume chemistry modeling. J. Volcanol. Geothermal Res. 152, 174–188. doi: 10.1016/j.jvolgeores.2005.10.009
Pitz, M., Cyrys, J., Karg, E., Wiedensohler, A., Wichmann, H.-E., and Heinrich, J. (2003). Variability of apparent particle density of an urban aerosol. Environ. Sci. Technol. 37, 4336–4342. doi: 10.1021/es034322p
Plantower (2016). Digital Universal Particle Concentration Sensor PMS5003 Series Data Manual. Available online at: http://www.aqmd.gov/docs/default-source/aq-spec/resources-page/plantower-pms5003-manual_v2-3.pdf (accessed November 13, 2018)
Poland, M., Miklius, A., Orr, T., Sutton, J., Thornber, C., and Wilson, D. (2008). New episodes of volcanism at Kīlauea Volcano, Hawai'i. Eos Trans. Am. Geophys. Union 89, 37–38. doi: 10.1029/2008EO050001
Porter, J. N., Horton, K. A., Mouginis-Mark, P. J., Lienert, B., Sharma, S. K., Lau, E., et al. (2002). Sun photometer and lidar measurements of the plume from the Hawai'i Kīlauea Volcano, Hawai'i Volcano Pu'u 'Ō'ō vent: aerosol flux and SO2 lifetime. Geophys. Res. Lett. 29, 30–31. doi: 10.1029/2002GL014744
PurpleAir (2019). PurpleAir: Air Quality Monitoring. Available online at: https://www.purpleair.com/ (accessed October 26, 2018).
Rotstayn, L. D., and Lohmann, U. (2002). Simulation of the tropospheric sulfur cycle in a global model with a physically based cloud scheme. J. Geophys. Res. Atmos. 107. doi: 10.1029/2002JD002128
Sarangi, B., Aggarwal, S. G., Sinha, D., and Gupta, P. K. (2016). Aerosol effective density measurement using scanning mobility particle sizer and quartz crystal microbalance with the estimation of involved uncertainty. Atmos. Meas. Tech. 9, 859–875. doi: 10.5194/amt-9-859-2016
Saxena, P., and Seigneur, C. (1987). On the oxidation of SO2 to sulfate in atmospheric aerosols. Atmos. Environ. 21, 807–812. doi: 10.1016/0004-6981(87)90077-1
Sayahi, T., Butterfield, A., and Kelly, K. (2019). Long-term field evaluation of the Plantower PMS low-cost particulate matter sensors. Environ. Pollut. 245, 932–940. doi: 10.1016/j.envpol.2018.11.065
Schmidt, A., Leadbetter, S., Theys, N., Carboni, E., Witham, C., Stevenson, J., et al. (2015). Satellite detection, long-range transport, and air quality impacts of volcanic sulfur dioxide from the 2014–2015 flood lava eruption at Bárðarbunga (Iceland). J. Geophys. Res. Atmos. 120, 9739–9757. doi: 10.1002/2015JD023638
Spinetti, C., and Buongiorno, M. (2007). Volcanic aerosol optical characteristics of Mt. Etna tropospheric plume retrieved by means of airborne multispectral images. J. Atmos. Solar Terrest. Phys. 69, 981–994. doi: 10.1016/j.jastp.2007.03.014
Stockwell, W. R., and Calvert, J. G. (1983). The mechanism of the HO-SO2 reaction. Atmos. Environ. 17, 2231–2235. doi: 10.1016/0004-6981(83)90220-2
Tam, E., Miike, R., Labrenz, S., Sutton, A. J., Elias, T., Davis, J., et al. (2016). Volcanic air pollution over the Island of Hawai'i: emissions, dispersal, and composition. association with respiratory symptoms and lung function in Hawai'i Island school children. Environ. Int. 92, 543–552. doi: 10.1016/j.envint.2016.03.025
US Geological Survey Hawai'ian Volcano Observatory (2018). Cooperator Report to Hawai'i County Civil Deference; Preliminary Analysis of the Ongoing Lower East Rift Zone (LERZ) Eruption of Kīlauea Volcano, Fissure 8 Prognosis and Ongoing Hazards. Available online at: https://volcanoes.usgs.gov/vsc/file_mngr/file-185/USGS%20Preliminary%20Analysis_LERZ_7-15-18_v1.1.pdf (accessed Feburary 27, 2019)
von Glasow, R., Bobrowski, N., and Kern, C. (2009). The effects of volcanic eruptions on atmospheric chemistry. Chem. Geol. 263, 131–142. doi: 10.1016/j.chemgeo.2008.08.020
Wang, Y., Li, J., Jing, H., Zhang, Q., Jiang, J., and Biswas, P. (2015). Laboratory evaluation and calibration of three low-cost particle sensors for particulate matter measurement. Aerosol Sci. Technol. 49, 1063–1077. doi: 10.1080/02786826.2015.1100710
Watson, J., Chow, J., Moosmüller, H., Green, M., Frank, N., and Pitchford, M. (1998). Guidance for Using Continuous Monitors in PM2.5 Monitoring Networks. EPA-454/R-98-012. Environmental Protection Agency, Office of Air Quality.
Wilson, W. E., Chow, J. C., Claiborn, C., Fusheng, W., Engelbrecht, J., and Watson, J. G. (2002). Monitoring of particulate matter outdoors. Chemosphere 49, 1009–1043. doi: 10.1016/s0045-6535(02)00270-9
Wooten, K. M., Thornber, C. R., Orr, T. R., Ellis, J. F., and Trusdell, F. A. (2009). Catalog of Tephra Samples From Kīlauea's Summit Eruption, March-December 2008. U.S. Geological Survey Open-File Report 2009-1134, 26.
World Health Organization (2005). WHO Air Quality Guidelines for Particulate Matter, Ozone, Nitrogen Dioxide and Sulfur Dioxide. Global update.
Wyrtki, K., and Meyers, G. (1976). The trade wind field over the Pacific Ocean. J. Appl. Meteorol. 15, 698–704. doi: 10.1175/1520-0450(1976)015<0698:TTWFOT>2.0.CO;2
Keywords: Kīlauea, volcano, PM2.5, SO2, emissions, air quality, Hawai'i
Citation: Whitty RCW, Ilyinskaya E, Mason E, Wieser PE, Liu EJ, Schmidt A, Roberts T, Pfeffer MA, Brooks B, Mather TA, Edmonds M, Elias T, Schneider DJ, Oppenheimer C, Dybwad A, Nadeau PA and Kern C (2020) Spatial and Temporal Variations in SO2 and PM2.5 Levels Around Kīlauea Volcano, Hawai'i During 2007–2018. Front. Earth Sci. 8:36. doi: 10.3389/feart.2020.00036
Received: 23 October 2019; Accepted: 03 February 2020;
Published: 25 February 2020.
Edited by:
Elisa Trasatti, Istituto Nazionale di Geofisica e Vulcanologia (INGV), ItalyReviewed by:
Claudia Spinetti, Istituto Nazionale di Geofisica e Vulcanologia (INGV), ItalyCopyright © 2020 Whitty, Ilyinskaya, Mason, Wieser, Liu, Schmidt, Roberts, Pfeffer, Brooks, Mather, Edmonds, Elias, Schneider, Oppenheimer, Dybwad, Nadeau and Kern. This is an open-access article distributed under the terms of the Creative Commons Attribution License (CC BY). The use, distribution or reproduction in other forums is permitted, provided the original author(s) and the copyright owner(s) are credited and that the original publication in this journal is cited, in accordance with accepted academic practice. No use, distribution or reproduction is permitted which does not comply with these terms.
*Correspondence: Rachel C. W. Whitty, ZWVyY3d3QGxlZWRzLmFjLnVr
Disclaimer: All claims expressed in this article are solely those of the authors and do not necessarily represent those of their affiliated organizations, or those of the publisher, the editors and the reviewers. Any product that may be evaluated in this article or claim that may be made by its manufacturer is not guaranteed or endorsed by the publisher.
Research integrity at Frontiers
Learn more about the work of our research integrity team to safeguard the quality of each article we publish.