- 1School of Geography and Sustainable Development, University of St Andrews, St Andrews, United Kingdom
- 2Scottish Association for Marine Science, Scottish Marine Institute, Oban, United Kingdom
Fjords are recognized as globally significant hotspots for the burial and long-term storage of marine and terrestrially derived organic carbon (OC). By trapping and locking away OC over geological timescales, fjord sediments provide a potentially important yet largely overlooked climate regulation service. Currently, our understanding of the spatial distribution of OC within the surficial sediments of fjords is limited and this potentially implies an overestimation in the global estimates of OC buried in fjords as current calculation methods assume a homogenous seabed. Using the mid-latitude fjords of Scotland and Ireland as a natural laboratory, we have developed a multi-tiered methodological approach utilizing a spectrum of data ranging from freely available chart data to the latest multibeam geophysics to determine and map the seabed sediment type. Targeted sampling of fjord sediments was undertaken to establish a calibration of sediment type against OC content. The results show that fjord sediments are highly heterogeneous both in sediment type and OC content. Utilizing the tiered mapping outputs, first order estimates of the surficial (top 10 cm) sediment OC stock within Scottish fjords (4.16 ± 0.5 Mt OC) and Irish systems (2.09 ± 0.26 Mt OC), when normalized for area the surficial sediments of Scottish and Irish fjords hold 2027 ± 367 and 1844 ± 94 tonnes OC km-2 respectively far exceed estimates for the continental shelf, again highlighting fjord sediments as hotspots for the capture of OC. This tiered approach to mapping sediment type is ideally suited to areas of the marine environment where data availability and quality is a limiting issue. Further understanding of the spatial heterogeneity of these sediments provides a foundation to reevaluate global fjord OC burial rates and to better understand the role of fjord sediments in regulating the global climate.
Introduction
Sediments at the land-ocean interface have been identified as key components in the global carbon (C) cycle (Berner, 1982; Bauer et al., 2013), with fjord sediments being highlighted as hotspots for high organic carbon (OC) burial (Smith et al., 2015; Cui et al., 2016) and storage (Smeaton et al., 2016, 2017). Globally, fjords are estimated to bury 21–31 Tg OC yr–1 (Smith et al., 2015; Cui et al., 2016), which equates to approximately 11% of annual marine OC burial. Further it is estimated that 55–62% of the OC buried in fjords is terrestrial in origin (Cui et al., 2016). The trapping and storage of both marine and terrestrial OC in these systems may provide an important and largely overlooked climate regulation service.
While the importance of fjords as sedimentary OC stores is becoming clearer, our limited understanding of the spatial distribution and heterogeneity of OC on the seabed may result in the over-estimation of the quantity of OC buried and in turn the amount of OC stored annually. Current OC burial estimates (Smith et al., 2015; Cui et al., 2016) assume that the seabed sediments within fjords are homogenous and that the burial rates calculated from a sediment core is applicable across a fjord or even an entire geographical region. However, it is well known that the seabed of both fjords and other coastal sedimentary systems are spatially highly variable and that this heterogeneity impacts the sedimentary OC content of the sediments. This issue can be further confounded by the choice of coring sites; the best coring sites for paleo- and geochemical studies tend to be the OC rich muddy sediments. The coarser and OC poor sediments are often under-sampled as they are poor sites for coring. As well as the uncertainty arising in net OC burial rates, there is also uncertainty as to where the OC is buried and stored, which hinders potential policy or management actions aimed to protect these globally significant C stores.
The first steps toward reducing uncertainty in the global OC burial estimates within fjords are to improve our understanding of the sediment heterogeneity at the seabed. Fjords, and coastal systems in general, are less well studied than the continental shelf where there is a generally good understanding of the spatial distribution of sediment types (Lark et al., 2012; Bockelmann et al., 2018; Wilson et al., 2018; Kaskela et al., 2019). In turn, such data provide insights into the potential spatial heterogeneity of OC on the continental shelf (Diesing et al., 2017). The approaches to mapping sediment type on the shelf are largely statistically based (Lark et al., 2012; Bockelmann et al., 2018; Wilson et al., 2018) and require large datasets (sediment descriptions, grain size etc.) to allow reliable predictions. Scottish and Irish fjords largely lack these data, a pattern observed in coastal systems globally. Other approaches to map the seabed and sediment type utilize multibeam and backscatter geophysical techniques (Serpetti et al., 2012; Lark et al., 2015; Audsley et al., 2016; Brown et al., 2019) where changes in acoustic responses are used to characterize seabed type. These methods provide an understanding of the spatial distribution of sediment types but have largely not been applied to fjord systems. The lack of data alongside the complex geomorphological and oceanographic conditions within fjords hinders the application of the statistical approaches which are currently available. High resolution sampling and geophysical techniques require significant financial and time investments to collect and process the data required, commonly restricting their use to single fjords or small areas of the coast.
While there are several approaches to mapping sediment type, only a few studies consider how this relates to the distribution of surficial OC and none of these are from fjords (Serpetti et al., 2012; Diesing et al., 2017). A common thread through much of this research is the link between OC and the physical properties of the seabed, in particular the sediment type and particle size (Mayer, 1994; Keil et al., 1997; McBreen et al., 2008; Serpetti et al., 2012; Diesing et al., 2017; Bao et al., 2019). If a relationship between sediment type and OC can be established for fjords there is potential for wide scale mapping and quantification of OC within surficial fjord sediments as significant volumes of legacy and “non-traditional” sediment type data are available globally (Mitchell et al., 2019).
Using the mid-latitude fjords of Scotland and Ireland as an example, we developed a tiered approach to mapping which acknowledges data gaps and limitations yet provides a robust technique to map the sediment type and surficial (top 10 cm) OC content across fjords with differing levels of existing data coverage. This research will provide a new template for both fjordic and other marine systems globally, delivering a strong foundation to re-evaluate global sedimentary OC burial rates in fjords and a tool to allow for targeted management and eventually protection of these globally significant C stores from future disturbance.
Study Area
The west coast and islands of Scotland are dominated by sea lochs (fjords) (Figure 1). In total, there are 226 fjords which can be subdivided into 111 large fjords (over 2 km long, with fjord length twice fjord width) (Edwards and Sharples, 1986) and 115 smaller systems. This study focuses on 133 systems spanning both groups (Supplementary Table 1). Recently, the 111 large fjords have been shown to be significant long-term store of OC with an estimated 252.4 ± 62 Mt OC being held within their postglacial sediments (Smeaton et al., 2017). Further research showed that a significant proportion of the OC stored in Loch Sunart (42 ± 10%) and Loch Teacuis (64.8 ± 5.2%) was terrestrial in origin (Smeaton and Austin, 2017) which are comparable to other fjords systems globally (Cui et al., 2016). Smeaton et al. (2017) highlighted that the Scottish fjords can be subdivided into different types both geographically (Mainland, Inner Hebrides, Outer Hebrides and the Shetland islands) and based on physical characteristics; for example, the fjords of the mainland and Inner Hebrides are classic fjords characterized by heavily glaciated geomorphology (Syvitski et al., 1987; Syvitski and Shaw, 1995; Howe et al., 2002). By contrast, the systems that dominate the coastline of the Outer Hebrides and the Shetland Islands differ significantly and tend to be shallower with a more subdued submarine geomorphology and could be referred to a fjards. Fjards are long and narrow and a products of glacial processes but unlike classical fjords they are relatively shallow and flat-bottomed and lack the geomorphological characteristics observed within glacially over-deepened fjords (Syvitski et al., 1987; Syvitski and Shaw, 1995; Howe et al., 2010). The differing geomorphological characteristics of these systems has resulted in different OC contents within the postglacial sediments, with the mainland fjords storing the majority of the estimated 252.4 ± 62 Mt of OC (Smeaton et al., 2017).
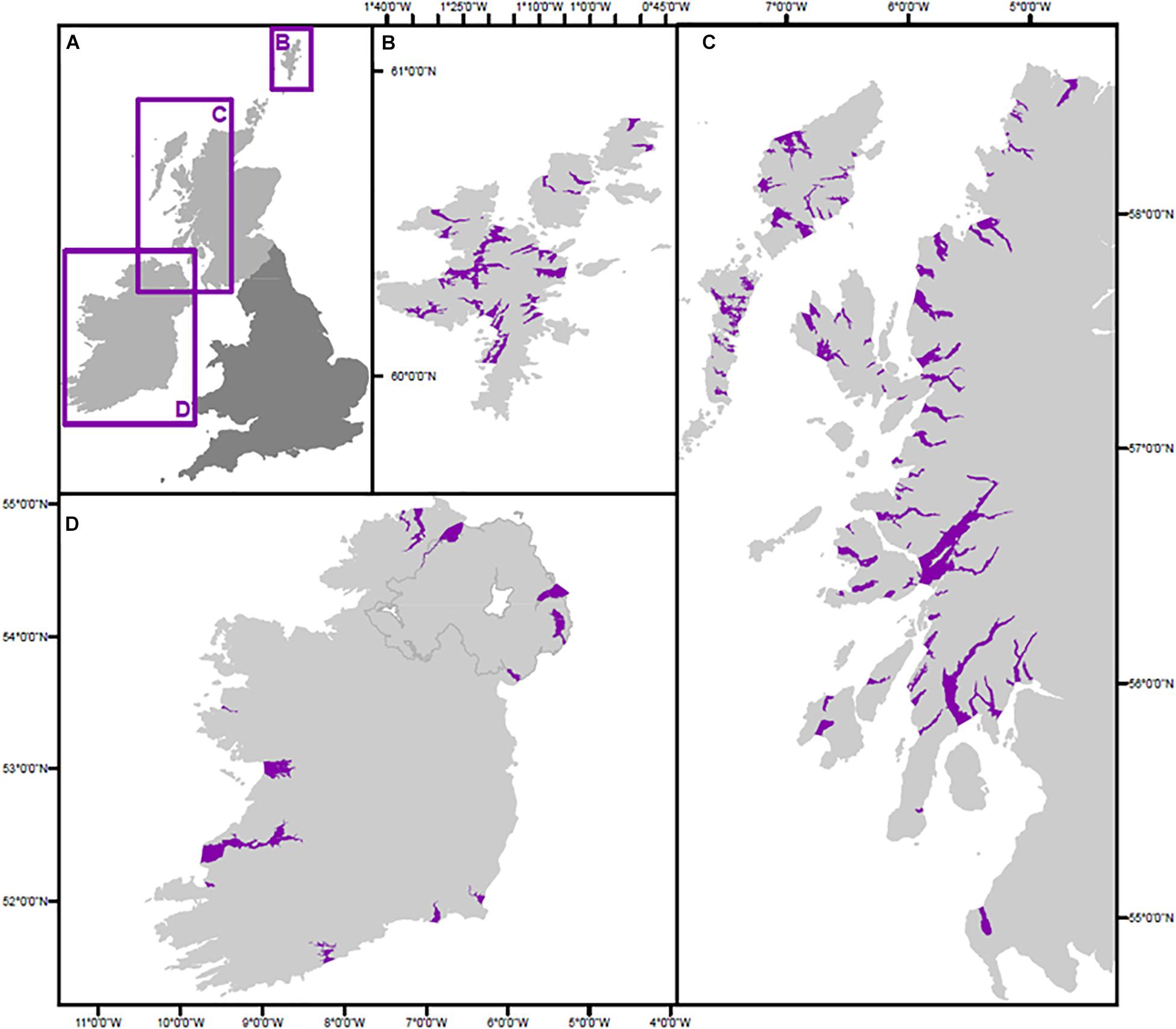
Figure 1. (A) Overview map illustrating the different regions focused on in this study. Maps highlighting the individual fjords of the (B) Shetland Islands, (C) Scottish Mainland and Inner and Outer Hebrides and (D) Northern and Republic of Ireland.
Ireland’s glacial history (Ehlers et al., 1991; Peters et al., 2015; O’Cofaigh et al., 2019) shares similarities with Scotland and this has resulted in a coastline with comparable geomorphology (Sinnot and Devoy, 1992) to that of Western Scotland. The Irish coast is dominated by bays and sea loughs (Figure 1); of these numerous systems, only Carlingford Lough, Killary Harbour and Lough Swilly are considered fjords (Nairn, 2005). The other sea loughs can be classified as fjards similar to the systems found on the islands of Scotland. These sea loughs are similar to the sea lochs of Shetland and the Outer Hebrides of Scotland. This study includes the three Irish fjords, in addition to 12 sea loughs (Figure 1D).
Materials and Methods
Primary Data Collection
Sampling
Surficial sediment samples were collected from 20 fjords around Scotland (Supplementary Figure 1). Between 2004 and 2017, sampling was conducted in Lochs Sunart, Etive, Creran, Ewe and Melfort from the RV Seòl Mara, RV Calanus, MV Walrus and RV Morwena. In 2015, samples were collected from the Shetland Islands from six fjords onboard the MV Moder Dy (Lo Giudice Cappelli et al., 2019 in review). The final phase of sampling took place in July 2017 aboard the MRV Alba na Mara, where 74 samples were collected from across eight Northern Scottish fjords (Lochs Eriboll, a Chairn Bhain, Glencoul, Glendhu, Gairloch, Torridon, Carron, Kishorn). A number of sampling techniques were employed during these cruises. The majority of the samples were collected using grab samplers (both Van Veen and Day) with corers (Gilson, Craib, Sholkovitch and Mega) also being employed at various times and locations. Cores were subsampled at 1 cm intervals. Full details of these samples and sampling techniques can be found in the Supplementary Data.
In addition, further samples were collected from the British Geological Survey (BGS) and British Ocean Sediment Core Research Facility (BOSCROF) sample repository to augment the sample collection. The repository provided sediments from across 15 fjords, bringing the number of samples for this study to 356 covering 35 fjords.
Physical Properties
On collection, the surficial samples were described using the modified Folk classification (Folk, 1954) scheme using five standard classes: gravel, sand, coarse sediment, mixed sediment and mud and muddy sand (Lark et al., 2012; Kaskela et al., 2019). The scheme was further supplemented with rock and biogenic/carbonate classes. Wet (WBD) and dry bulk density (DBD) were calculated from the sediment cores following the methodology set out by Dadey et al. (1992). These data were further supplemented by DBD data from Loch Sunart, Creran, Broom and Little Loch Broom and Etive (Smeaton et al., 2016, 2017). Additionally, DBD values for the coarser sediments (gravel, sand, etc.) were derived from the literature (Flemming and Delafontaine, 2000; Diesing et al., 2017).
Geochemical Analysis
The 356 surface sediment samples were analyzed to determine bulk elemental composition (OC and N). Each sample was freeze dried, homogenized, and approximately 10 mg of processed sediment was weighed out into silver capsules. The encapsulated samples underwent acid fumigation (Harris et al., 2001) to remove the carbonate, this entailed placing the samples in a desiccator with a beaker of 37% HCl acid for 48 h. Upon removal from the desiccator the samples were dried for 24 h at 40°C, OC was measured using an Elementar Vario EL elemental analyzer. The standard deviation of triplicate measurements (n = 30) were OC: 0.18% and N: 0.06%, respectively. Further quality control was assured by the repeat analysis of standard reference material B2178 (Medium Organic content standard from Elemental Microanalysis, United Kingdom) these samples deviated from the reference value by: OC = 0.07% and N = 0.02% (n = 20).
Secondary Data Collection
Sediment Type (Point Observations)
Point observations on sediment type (i.e., descriptions) for Scottish and Irish fjords were compiled from numerous sources (Supplementary Table 2). The main sources of these data were from habitat surveys which require substrate type to be recorded for each individual habitat; these data were accessed through the Joint Nature Conservation Committee (JNCC) Marine Recorder database. These data is largely collected by scuba divers and/or camera drops, and as a consequence tends to be from the shallower, marginal regions (i.e., edges, sill) of the fjords and therefore underrepresent a significant portion of the seabed.
Nautical charts also provide an important resource of bottom type data. The United Kingdom Hydrographic Office (UKHO) have been compiling a database of seabed bottom type for over 100 years to help characterize potential anchoring locations. This record of sediment type data is readily available but has been largely overlooked as a resource for seabed mapping. While this data may be considered “non-traditional,” it is quality controlled by UKHO under its statutory responsibilities to provide accurate information on charts for anchoring. The chart data has excellent spatial coverage across both Scottish and Irish fjords (Supplementary Figure 2), although the data does not extend to the shallower areas of the fjords. The habitat and chart datasets complement one another and together they provide observations from across the shallow and deeper regions of the fjords.
The dataset was further augmented with seabed descriptions from the BGS and Infomar. In total 60,405 point observations describing seabed sediment type were compiled for fjords across both Scotland and Ireland (Supplementary Figure 2). Supplementary Table 1 provides information on the location of the original data used within this study.
Unifying the Data
The secondary datasets differ in the classification schemes used to describe the seabed sediment types. The UKHO, BGS, Infomar and the data extracted from the nautical charts use the classical Folk scheme (Folk, 1954) which consists of 12 different classes. Furthermore, the sediment type data associated with the habitat descriptions accessed through the JNCC marine recorder do not utilize a standardized classification scheme and descriptions differ between surveys. To unify the data to the modified Folk classification scheme outlined in Section “Physical Properties,” a hierarchical classification tree similar to that used in Kaskela et al. (2019) was developed (Supplementary Figure 3).
Organic Carbon and Grain Size Data
Grain size and OC data for the fjords of Scotland and Ireland is limited (Supplementary Figures 4, 5) and is mainly associated with monitoring activities, particularly for the detection of polycyclic aromatic hydrocarbons (Webster et al., 2000, 2001, 2003, 2004; Guinan et al., 2001; Russell et al., 2008, 2011; McIntosh et al., 2012). The data from these studies was extracted and further supplemented by data from the International Council for the Exploration of the Sea (ICES) which stores all marine monitoring data for North Atlantic nations required under the OSPAR convention (Oslo Paris Convention). The grain size data included in these datasets are largely reported in the % < 63 μm (i.e., clay and silt content) which has been shown to correlate to OC content in some environments (Serpetti et al., 2012).
Unlike the other secondary data, the OC data collected from these sources will not be used to map the surficial OC content. Rather it will be used as a ground-truthing and validation dataset to test the accuracy of the outputs by directly comparing to the OC values from the predictive mapping to that of known OC values. Quality control measures were taken to assure these data are comparable to the primary OC data collect as part of this research. This involves only using OC data collected from bulk samples, and discarding OC data measured from specific size fractions (i.e., <63 and 20 μm).
Backscatter Data
Backscatter data was accessed through the BGS offshore index and Infomar’s data portal from 24 Scottish fjords and 5 Irish systems (Supplementary Figure 2). The data was assessed to determine the areal coverage and the quality of the backscatter signal (Supplementary Table 3). Geophysical techniques such as multibeam and backscatter often struggles to map the full extent of the seabed in fjords because of the geomorphological nature of these systems. The shallower areas (i.e., edges, sills) within fjords and coastal regions are difficult to survey because the water depth does not allow access to the vessels carrying the geophysical instruments.
Mapping the Seabed
Tier 1 Point Observations
Each of the modified Folk classes and the point observations within each class (Supplementary Figure 6) were assigned a numerical value from 0 for biogenic/carbonate to 7 for mud and muddy sand. The compiled data were statistically tested to determine the gridding technique best suited to the interpolation of the data. Twelve gridding techniques were subjected to cross validation (Chiles and Delfiner, 2009), where the residual Z mean value and standard deviation were examined. The technique with the lowest residual Z mean and standard deviation was chosen as the gridding technique best suited for this study. Kriging, with linear interpolation (Cressie, 1990), performed best with the interpolation because of the irregularly spaced nature of the data used in this study. This technique was twinned with a 1000 by 10000 node structure to create computationally efficient mapping of the different sediment types. Finally, the areal coverage of each sediment type was calculated for the 133 Scottish and 15 Irish systems (Supplementary Data). In addition to mapping the sediment type, this approach allows for the kriging standard error (KSE) to be calculated by taking the square root of the kriging variance. This allows the KSE to be mapped and used as a measure of uncertainty related to the predicted values (i.e., as a proxy for confidence in our predictions).
Tier 2 Backscatter
Backscatter classification was undertaken utilizing an unsupervised classification technique which has been widely used for benthic habitat mapping (Brown et al., 2011; Calvert et al., 2014). Unsupervised classification was performed using the ISO Cluster algorithm in ArcGIS (Brown and Collier, 2008; Ierodiaconou et al., 2011). This method organizes the backscatter raster into a number of groups using the maximum likelihood supervised classification approach. We undertook 250 iterations of the clustering procedure and found increasing the number of iterations beyond 250 had negligible impact on the outputs but increased computational strain similar to that observed by Calvert et al. (2014). The ISO Cluster outputs were ground-truthed using the independent sediment type dataset produced from the samples collected from the research cruises (see Section Physical Properties) and those reported in the ICES database (see Section Organic Carbon and Grain Size Data).
Chi-squared (χ2) tests for independence where carried out on contingency tables created from ISO Cluster analysis. As χ2 is sensitive to the sample size n, with large n producing significant χ2, we were able to calculate Cramer’s V statistics (Cramér, 1946). Cramer’s V values range from 0 (no relationship) to 1 (relationship) and are not sensitive to sample size; results from these statistical analyses are reported in the Supplementary Data.
Tier 3 Composite Mapping
Composite sediment type maps (Tier 3) were created through the merger of the Tier 1 and 2 maps. This approach was applied to the 29 fjords which have both Tier 1 and 2 maps available. As with the previous tiered approaches, the areal coverage of each sediment type was calculated (Supplementary Data).
Surficial Carbon Mapping
The spatial distribution of the surficial OC was mapped utilizing the OC data collected from the 356 samples collected from fjords around Scotland. The minimum and maximum values were calculated for each of the Folk classes and corrected to exclude outliers (i.e., lower and upper whiskers on a boxplot). These values were utilized to create a ramped scale increasing in 0.5% OC increments, the ramped scale was applied to the best available sediment type map (i.e., Tier 1 to 3) for each of the Scottish and Irish fjords. The surficial OC maps were ground-truthed using the OC data produced from the data reported in the literature and in the ICES database (see Section Organic Carbon and Grain Size Data).
The surficial OC stocks (top 10 cm) were calculated for each fjord to allow comparison to other surficial sediment stock estimates (Burrows et al., 2014, 2017; Diesing et al., 2017; Luisetti et al., 2019). The surficial OC stock was calculated using the sediment coverage (m2) combined with the sediment depth (0.1 m), bulk density data (kg m–3) and the available OC data (%). The bulk density and mean OC contents used in the calculations are reported in Supplementary Table 4.
Results and Interpretation
Exploring the Relationship Between Sediment Type and OC
Using the secondary data (Supplementary Figures 3, 4), the relationship between grain size (% < 63 μm and OC was explored to determine if it could be exploited as a tool to map surficial OC (Figure 2) across both Scotland and Ireland. The results indicate that the clay and silt component of the sediment and OC content in Scottish and Irish fjords are poorly correlated (especially as OC increases), although there is be a degree of covariance between the variables.
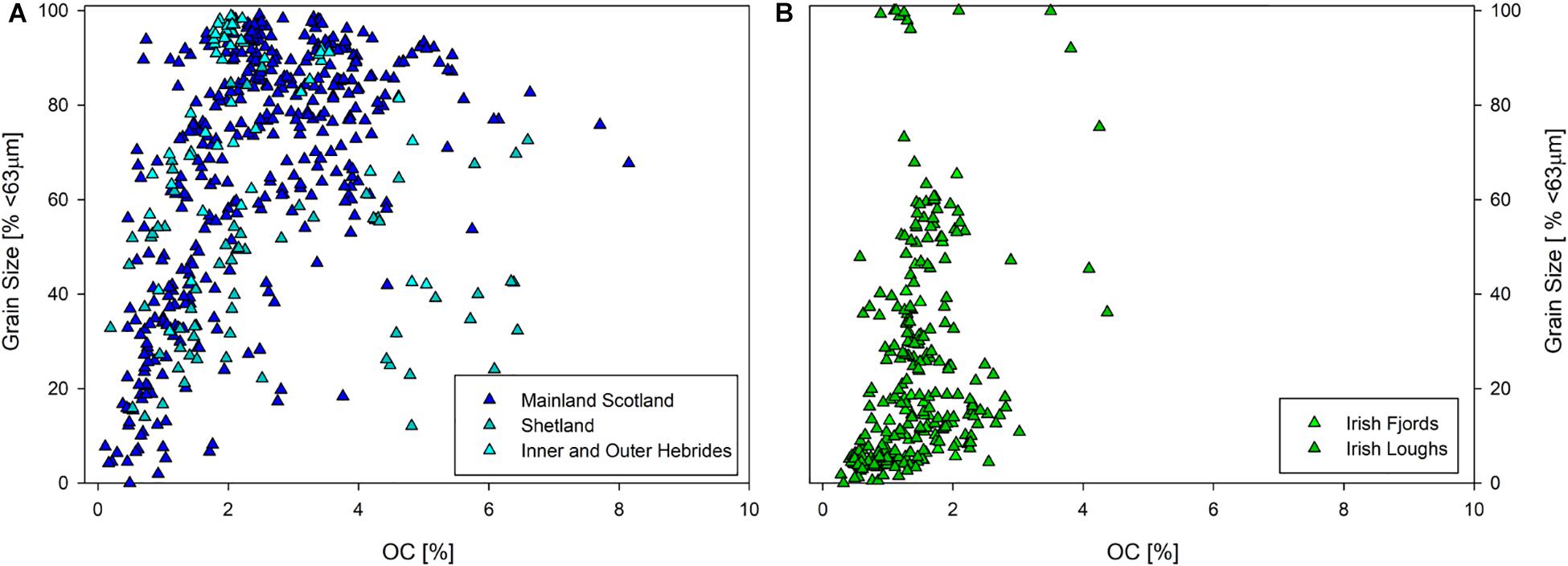
Figure 2. The relationship between grain size (% < 63 μm) and OC content explored using secondary data (A) Scotland (n = 670) and (B) Ireland (n = 274).
This is counter to the previous findings on continental shelves (McBreen et al., 2008; Serpetti et al., 2012; Diesing et al., 2017) where there is normally a strong correlation between mud content (i.e., % < 63 μm) and OC. Fjords, are significantly influenced by local factors (hydrology, run-off, bathymetry, etc.) this in conjunction with the greater heterogeneity in both bottom water temperature and dissolved oxygen concentrations, both of which are known to influence sediment OC (Woulds et al., 2007, 2016; Middelburg and Levin, 2009), may explain why the universal relationship between sediment grain size and OC content is not as strong as some studies from open-shelf regions suggest. However, when this relationship is explored within individual systems (Supplementary Figures 7–9) strong correlations are observed between grain size and OC but this relationship is not universal across all 33 fjords where data are available.
In contrast, when the sediments are classified using the modified Folk scale there is a clear link between OC and sediment type (Figure 3). The OC content in these sediment types increase significantly as we transition from coarser grained to the muddier substrates. This pattern is consistent over all the sediment classes apart from gravel where the OC content is equivalent to that of the coarse sediment class. This is due to the small number of samples (n = 3) in comparison to the other classes; additionally, gravels can trap fine particles (and OC) within their coarse sedimentary matrix.
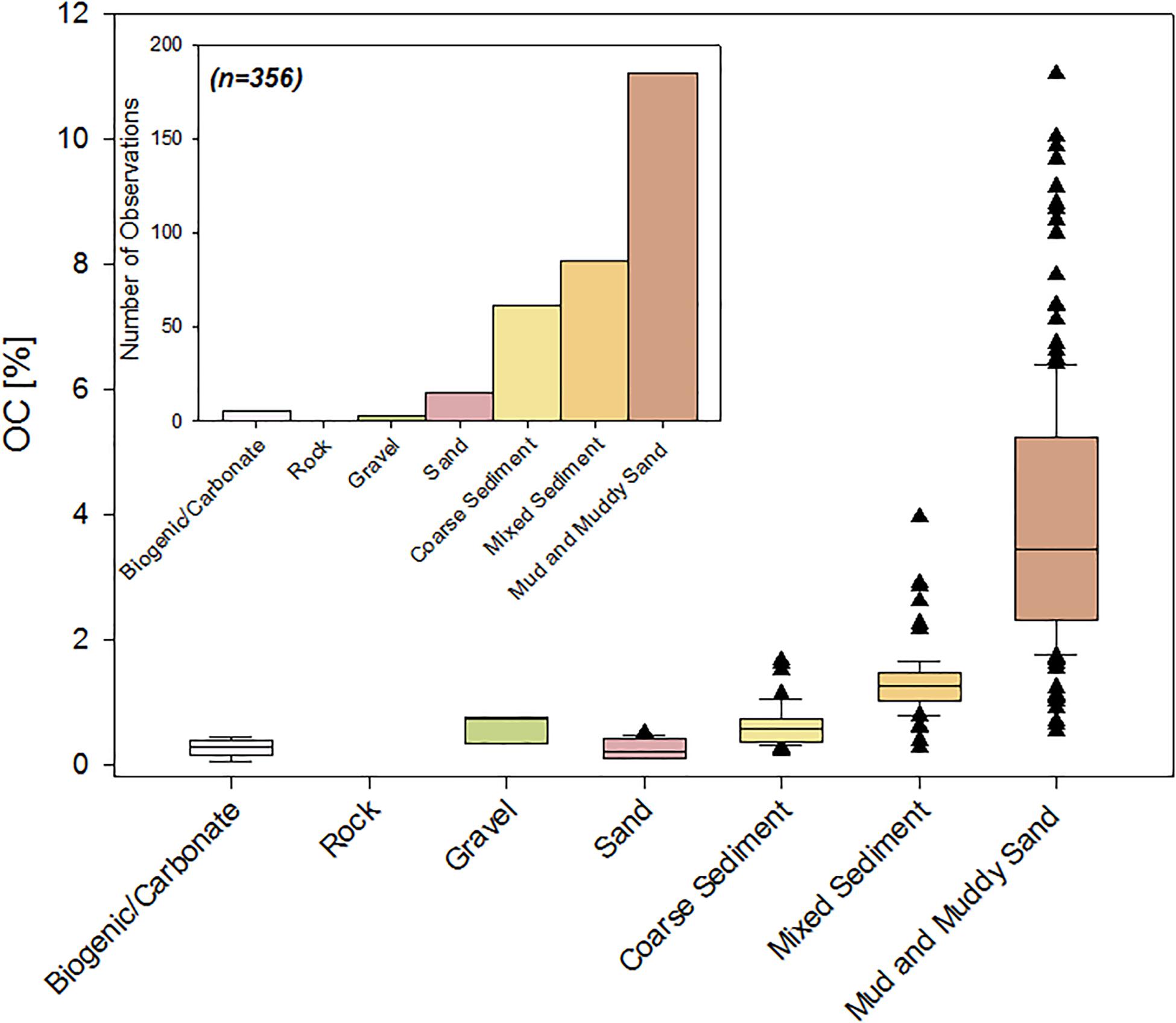
Figure 3. OC content of samples (n = 356) across the modified Folk classification scheme collected from 35 fjords around Scotland. Inset: Number of samples in each of the Folk classes.
Mapping Sediment Type
The sediment type of the 133 Scottish fjords and 15 Irish systems were successfully mapped using the three-tiered approach described above (high resolution maps included in the Supplementary Material) which also allowed the aerial coverage of each sediment type to be calculated (Supplementary Data). To determine the differences between the different mapping techniques and their relative performance, we initially used the Loch Linnhe complex of fjords which consist of six fjords with differing data availability (Figure 4).
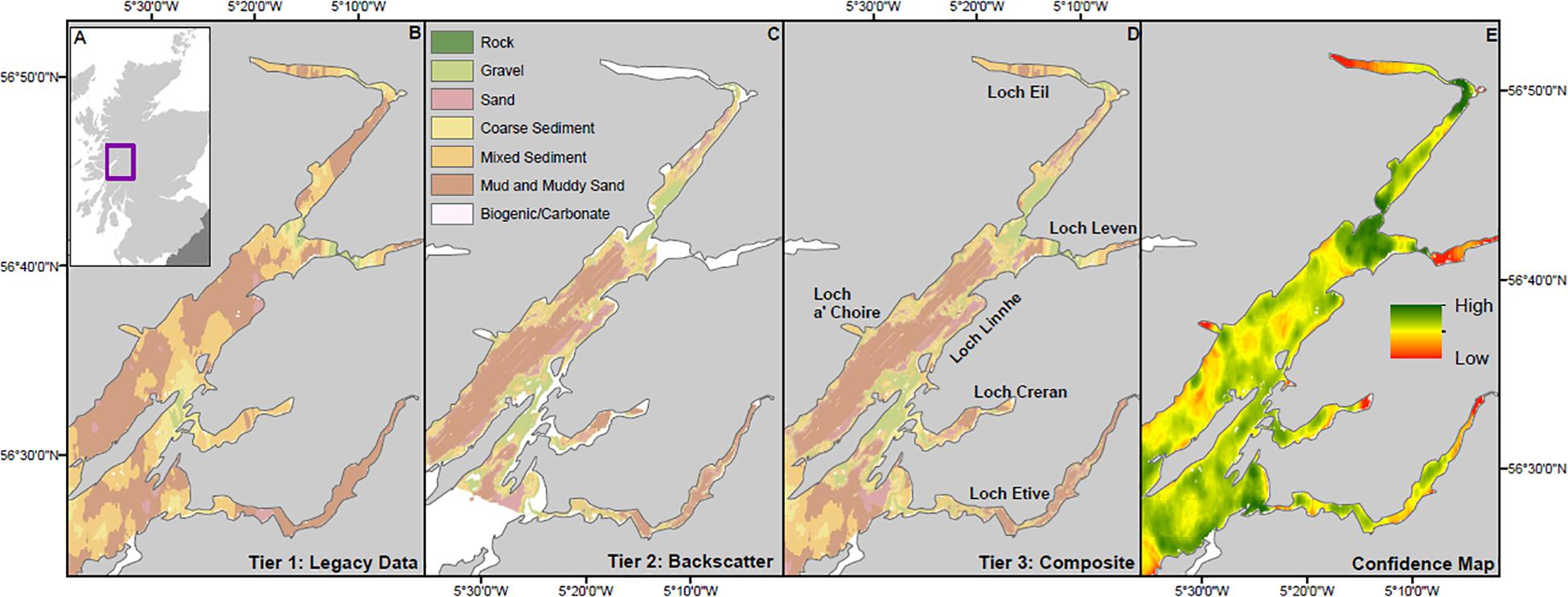
Figure 4. Sediment type mapped across the Loch Linnhe fjord complex. (A) Loch Linnhe in context of the United Kingdom, (B) Tier 1, point observations, (C) Tier 2, backscatter, (D) Tier 3 Composite approaches, and (E) Confidence map produced from the Kriging Standard Error.
When we compare the outputs from Tier 1 and Tier 3 mapping for the Loch Linnhe complex, Tier 1 underestimates the areal coverage of all the sediment classes when compared to the Tier 3 map, with the exception of the coarse sediment (Table 1). Most of the classes show an underestimation of between 0.78 and 2.75%, while the coarse sediment was overestimated by 5.46%. The relatively small difference between the Tier 1 and 3 mapping approaches provides confidence that if high quality backscatter is not available, robust maps of sediment type can be produced from available point observations.

Table 1. Difference in seabed coverage (%) for each of modified Folk classes (Kaskela et al., 2019) across the Loch Linnhe fjord complex calculated using the different mapping techniques, Green indicates that the Tier 1 map underestimates seabed coverage while Red is indicative of an overestimation in comparison to the Tier 3 approach.
The quality of the different mapping techniques was further tested using the data from the 29 systems where backscatter data were available (Figure 5). When the estimated seabed coverage for the 24 Scottish fjords are compared, the Tier 1 mapping underestimated the rock (0.52%), gravel (4.41%) and sand (5.07%) and overestimated the coarse sediment (4.70%), mixed sediment (4.70%) and the mud and muddy sands (0.59%) when compared to the Tier 3 estimate of seabed coverage. The five Irish systems exhibit greater variation between Tier 1 and 3 mapping approaches. The Tier 1 maps underestimated the coverage of rock (0.61%), mixed sediment (5.07%) and the mud and muddy sands (0.48%) and overestimated the coverage of gravel (0.2%) in comparison to the Tier 3 maps. These values are broadly similar but the two mapping techniques differ significantly, overestimating sand by 25% and underestimating coarse sediments by 19%. The largest differences between these two mapping techniques is between the sand and the coarse sediment, probably because sand is a significant component of coarse sediments. The broad similarity in these sediments is potentially the result of misidentification of these sediment types that leads to differing estimates of sediment coverage between the mapping approaches. However, as the mud and muddy sands hold the greatest quantity of OC (Figure 3), the fact that we only see between −0.59 and 0.48% variation in coverage between the different mapping approaches is encouraging. A final test was undertaken using samples collected from the research cruises to ground-truth the sediment maps. The test used the sediment description from these samples and compared them to the Tier 1 and, where available, the Tier 3 maps. In total 84% of the 356 samples had matching sediment descriptions. The remaining 16% of these samples were mismatches exclusively between the coarser sediments (sand and coarse sediments). This multifaceted analysis indicates that for the OC rich sediments, all mapping approaches perform well and allow sediment type to be mapped across data poor and rich systems; however, caution should be applied when interpreting the coarser sedimentary types, especially in areas where sand and coarse sediment are collocated.
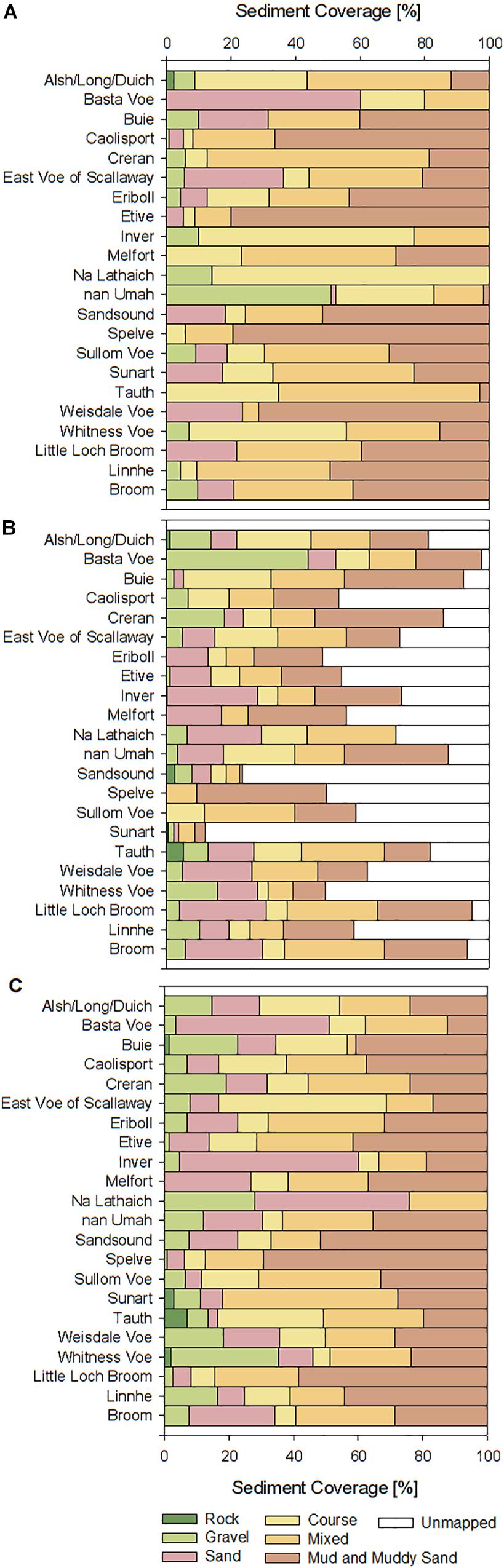
Figure 5. Visualization of the difference between the three-tiered mapping approach across the Scottish fjords with all data types. (A) Tier 1, point observations, (B) Tier 2, backscatter, and (C) Tier 3 Composite.
The mean errors calculated through these comparisons were applied to calculate the areal coverage (km2) of the different sediment types allowing the surficial OC stocks and their uncertainties to be estimated. The full results from the comparison between mapping approaches can be found in Supplementary Tables 5, 6.
A National Overview (Sediment Coverage)
The results of the mapping reveal distinct difference in the regional sedimentary composition of fjords in both Scotland and Ireland (Figure 6). The mean composition of the fjords of mainland Scotland are dominated by mixed and muddy sediments, with the fjord sediments elsewhere tending to be coarser in nature. This pattern is most apparent in the fjards of the Outer Hebrides where gravels and coarse sediments significantly overshadow other sediment types. This may reflect both a diminished sediment supply and the shallow, flat bottomed nature of these fjards, where coarse material is retained within the fjord and finer-grained sediments are flushed out to sea. The sediments from the fjords of the Inner Hebrides and the Shetland Islands are distributed over the different classes more equally than the mainland fjords, where mixed and muddy sediments dominate. The only mapped occurrence of biogenic/carbonate rich sediments were in the fjords of Shetland; samples collected from the MV Moder Dy confirm the presence of large quantities of broken shell material (Lo Giudice Cappelli et al., 2019 in review). The sediment coverage of the seabed of both the Irish fjords and sea loughs is far more diverse than that observed in Scotland’s fjords (Figure 6). The sediment in these systems contain a far larger sand component as well as generally coarser particles than their Scottish counterparts. There are similarities between the Irish systems and those of the Scottish Islands. The shallow nature of these systems may be conducive to the capture of coarser material that also hinders the accumulation of the finer OC rich sediments. In addition, the coastal and shelf sediments surrounding Ireland are dominated by sand and coarse sediments (Kaskela et al., 2019), the transport of this material to the fjords and sea loughs may be a secondary cause for the sediment composition in these systems.
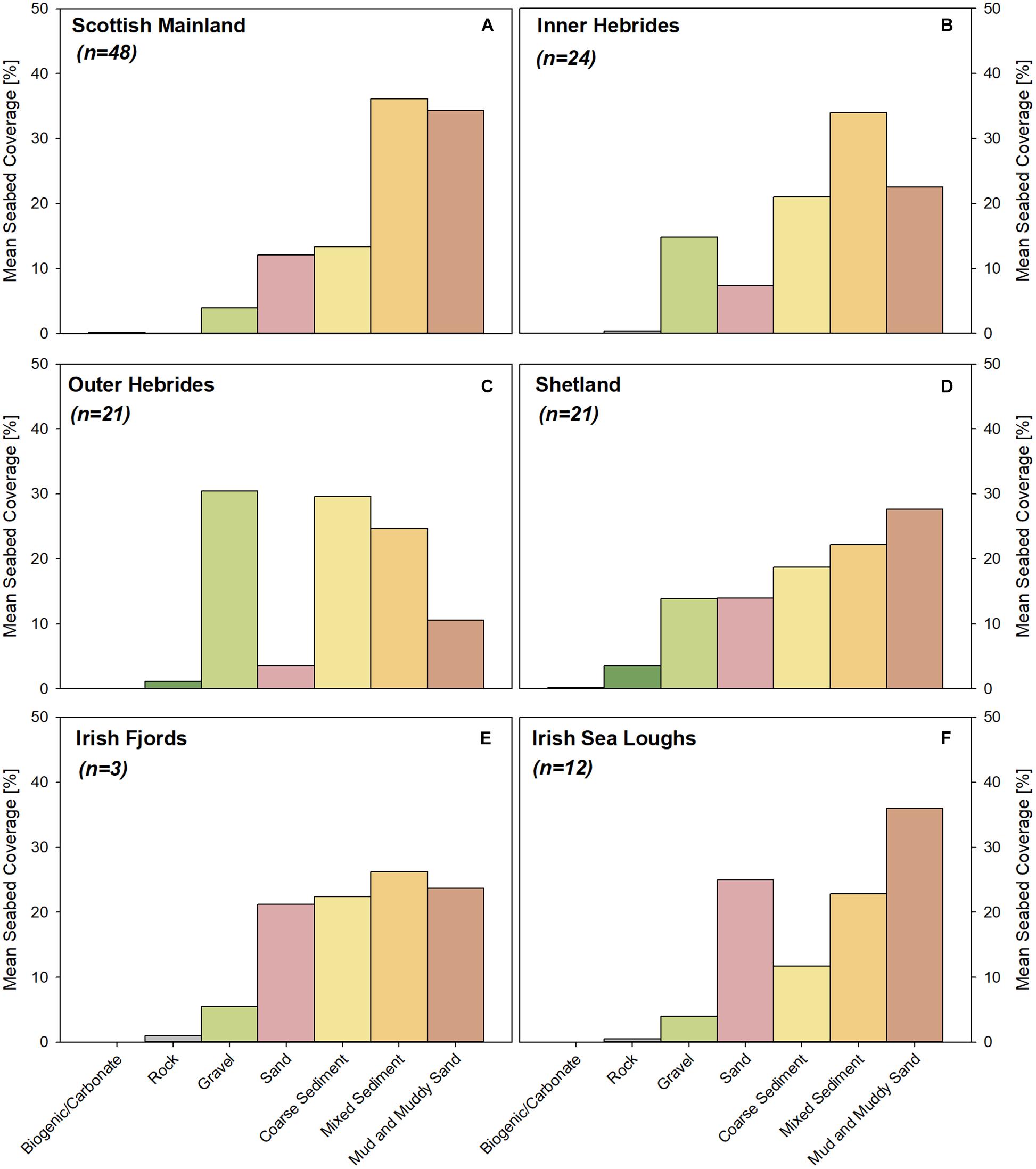
Figure 6. Regional differences in mean seabed coverage (%) of the seven different sediment types across the fjords of (A) Mainland Scotland, (B) the Inner Hebrides, (C) the Outer Hebrides, (D) the Shetland Islands, (E) the fjords, and (F) sea loughs of Ireland.
When the sediment type coverage of the seabed is converted to areal extent (km2), the mud and muddy sand class is estimated to cover the greatest area in both Scotland and Ireland (Figure 7). The extent of the different sediment classes in Ireland mirror the pattern observed in the calculated% seabed coverage estimates, but the Scottish fjords differ. In Scotland (mainland and island systems), the average fjord sediment composition is dominated by mixed sediments (Figure 6); and when areal extent is calculated, the mud and muddy sands are the dominate class. This difference is driven by the larger systems such as Loch Linnhe’s seabed being dominate by mud and muddy sands (Table 1).
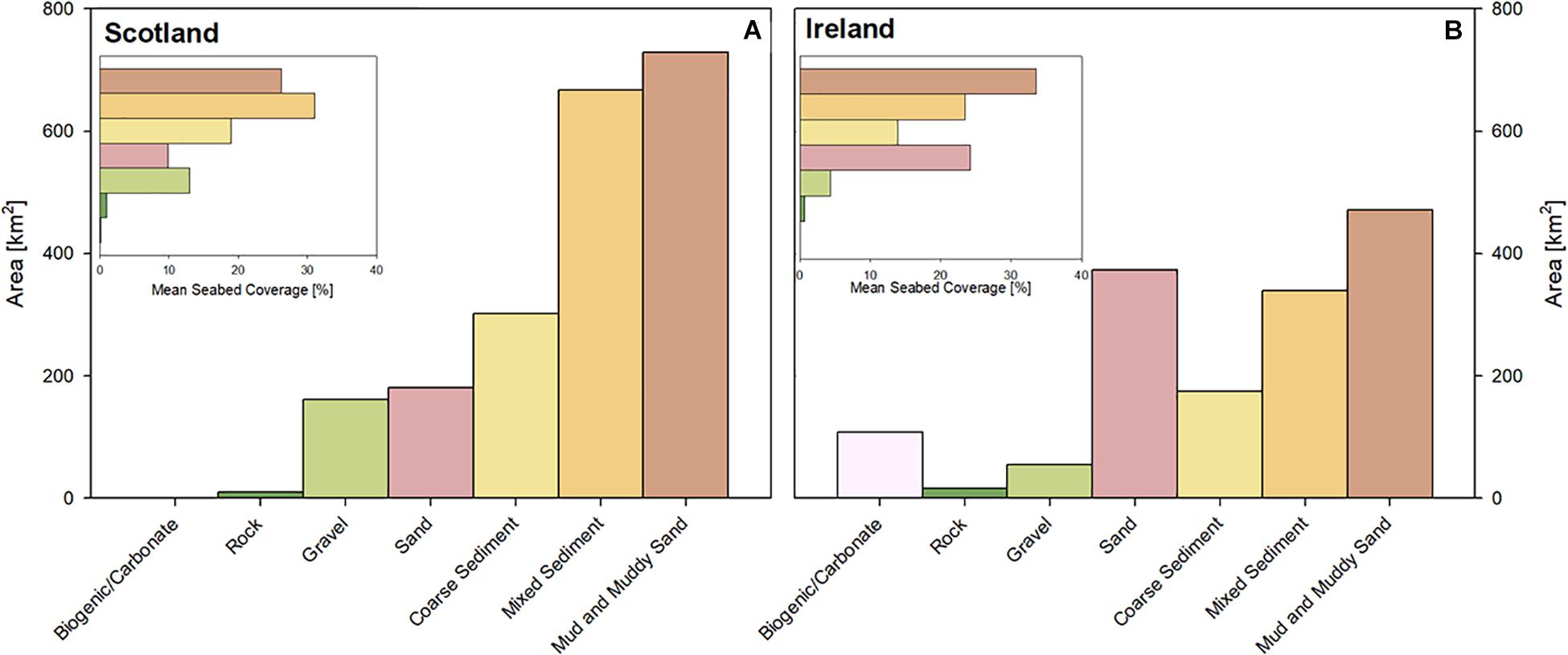
Figure 7. Areal extent of the different sediment types across (A) Scotland and (B) Ireland. Inset: Mean seabed coverage for Scotland and Ireland.
The regional differences in sediment coverage within these systems emphasizes the importance of understanding the seabed conditions within fjords, and in the marine environment more generally, highlighting that not all mid-latitude fjord systems are equivalent depositional environments.
Sedimentary OC Heterogeneity
The best available sediment map (i.e., Tier 1 or 3) for each fjord was combined with the OC data (Figure 3) to map the spatial distribution of the OC at 0.5% OC increments (high resolution maps included in the Supplementary Material). Using the Loch Linnhe complex as a case study (Figure 8), the greatest concentrations of OC is observed in the muddy sediments, typically occupying the upper portions and the deep central basins of the fjords. The fjord edges and sill regions, in contrast, are low in OC due to the coarse/rocky nature of the seabed in these locations. It is important to highlight that the muddy sediments are mapped as having an OC content of >2% and that the actual OC content of these sediments can be lower and also far exceed these values (Figure 3). Therefore, the maps outlining the spatial distribution of sediment OC are indicative, highlighting the location of OC “hotspots” rather than definitive measures of OC content.
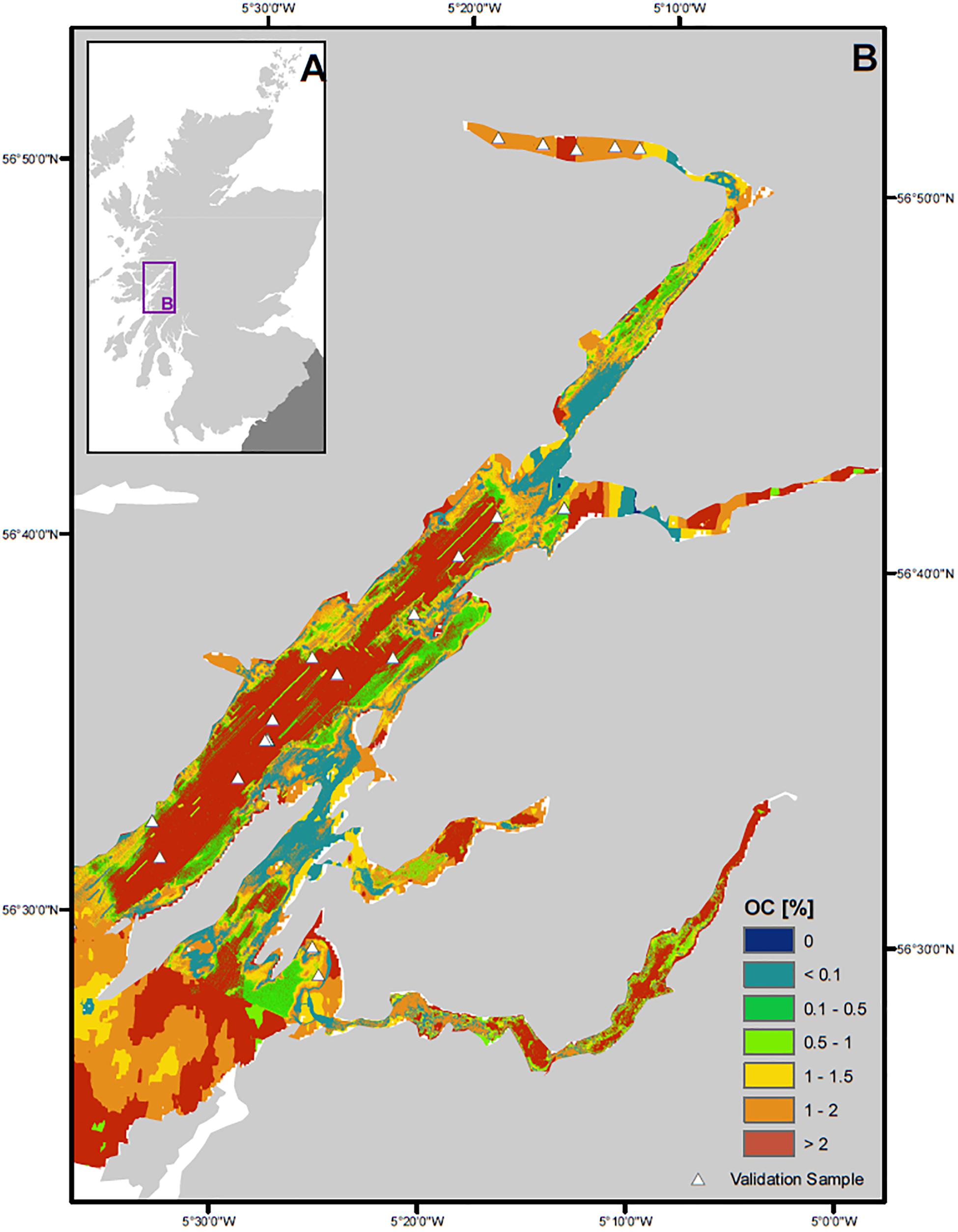
Figure 8. Spatial distribution of OC in surficial sediments (top 10cm). (A) The Loch Linnhe fjord complex in context of the United Kingdom and (B) surficial sediment OC content (%).
The accuracy of these OC maps was tested by comparing them to the independent OC data (see Section Organic Carbon and Grain Size Data). Within the Loch Linnhe complex, there were 20 independent samples (Figure 8), 80% of these fell within the mapped OC unit appropriate for the measured OC concentration. The remaining 20% were samples associated with the sand and coarse sediments, where the measured OC content of the samples did not match the predicted values, of these sample only one overestimated the %OC. The error between the measured OC concentration and the mapped value rarely exceeds the mapped OC increments (i.e., 0.5% OC). These error is likely due to the co-location of multiple sediment types and the influx of multiple sources of OC. The pattern observed in Loch Linnhe is mirrored throughout Scotland and Ireland when the mapped OC predictions are compared to the ground-truthing samples (Supplementary Figure 4). In Scotland as a whole, 72% of the ground-truthing samples correspond to the mapped OC values while 64% of the Irish samples match the mapped OC concentration data. It should be noted that the low OC values around the sills and edges of the fjords are difficult to ground-truth as there is a general lack of samples from these areas. However, these low OC zones are probably appropriate because we would expect coarse materials, such as gravel, to accumulate in these high energy areas (Inall et al., 2004; Stigebrandt, 2012; Staalstrøm and Røed, 2016). The larger errors of OC prediction observed within the Irish systems is most likely due to the fact that the calibrated sediment type and associated OC content originate solely from Scottish fjords.
Surficial OC Stocks
OC stocks were calculated for the top 10 cm of sediment in both the Irish and Scottish systems to allow comparisons to other studies but it is important to highlight that the organic matter at this depth is still actively degrading. Therefore, the OC held within the top 10 cm can only be considered a stock rather than a store of OC and caution should be applied when using such shallow depths to estimate OC stocks of fjord and continental shelf sediments.
Nevertheless, it was estimated that in the top 10 cm of sediment in Scotland’s fjords contain 4.16 ± 0.5 Mt OC with the muddy sediments holding 63% of that OC (Table 2). Of the 4.16 ± 0.5 Mt OC 72% is held in the sediments of mainland Scotland. The systems of Northern Ireland and the Republic of Ireland hold 0.94 ± 0.12 Mt OC and 1.15 ± 0.14 Mt OC respectively, again with the greatest proportion of this total held within the muddy sediments. Previous assessments of surficial sediment of Scottish fjords estimated the OC stock to be 0.33Mt (Burrows et al., 2014). The results presented in Table 1 suggest these previous estimates significantly underestimated the OC held within the top 10 cm of sediment in these systems. The United Kingdom/North sea continental shelf surficial sediments (top 10 cm) are estimated to hold 205 – 592 Mt OC (Burrows et al., 2014, 2017; Diesing et al., 2017; Luisetti et al., 2019) in comparison the fjord OC stocks are relatively small in magnitude but it must be remembered that there is a significant difference in areal coverage.
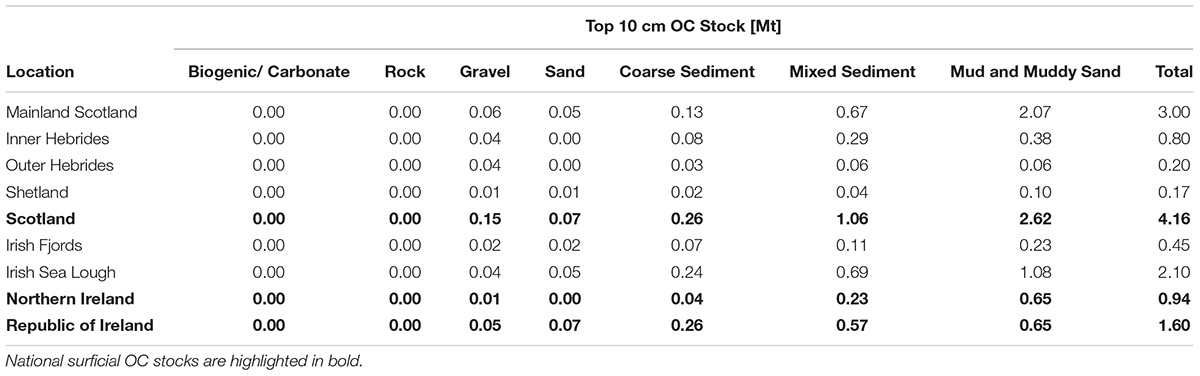
Table 2. Surficial (top 10 cm) OC stocks for fjords for Scotland and Ireland broken down to regions.
The top 10 cm of sediment in fjords hold a relatively small amount of OC in comparison to shelf sediments; yet when normalized for area the fjords are a significantly more effective at trapping OC in the surficial sediments. The Scottish and Irish fjords have an OC density of 2027 ± 367 and 1844 ± 94 tonnes OC km–2 respectively (Figure 9), which far exceeds that observed on the continental shelf with estimates ranging between 428 and 1259 tonnes OC km–2 (Burrows et al., 2014, 2017; Diesing et al., 2017; Luisetti et al., 2019). The fjords of mainland Scotland have the highest OC density followed by the fjords of the Inner Hebrides and the Shetland Islands with the Outer Hebrides having the lowest OC density in Scotland (Figure 9). This pattern is likely due to the submarine geomorphology and supply of OC to the fjords. The mainland systems are deeply glaciated and tend to have larger catchment thus supplying greater quantities of OC. In contrast, the Outer Hebrides systems are shallow and have small catchments resulting terrestrial OC likely being flushed out to sea. This pattern was observed by Smeaton et al. (2017) where the postglacial sedimentary OC stocks of the mainland fjords of Scotland far exceeded that of other Scottish locations. The Irish fjords and loughs are comparable to that the fjards found on the Scottish Islands (Figure 9). The Republic and Northern Irish sea loughs have the highest OC density, due to these systems having a more “fjord like” geomorphology (i.e., restricted exchange) compared to the more estuarine like loughs of the Republic of Ireland.
When the surficial OC stocks are ranked (Table 3) it is clear that a small number of systems hold much of the 4.16 ± 0.5 Mt OC (Table 2). This pattern is also observed in the postglacial OC stocks where the 14 largest fjords hold 65% of the sedimentary OC (Smeaton et al., 2017). When the top 10 rankings of both datasets are compared the same fjords appear on the list though in a slightly different order, with the exception of Loch Etive which is replaced by Loch Scridian. Loch Etive holds the sixth largest postglacial OC store but only ranks 17th when considering the 10 cm stock, in comparison Loch Scridian is ranked 8th (Surficial OC Stock) and 11th (Postglacial OC Stock). The cause of this discrepancy is due to the bottom waters in the upper basin of Loch Etive which are semi-permanently hypoxic (Friedrich et al., 2014). Low oxygen conditions are recognized as an important factor in governing the burial and preservation of OC (Woulds et al., 2007; Middelburg and Levin, 2009), these conditions preserve a greater quantity of OC in-turn increasing the magnitude of the postglacial OC store and allowing Loch Etive to rank higher.
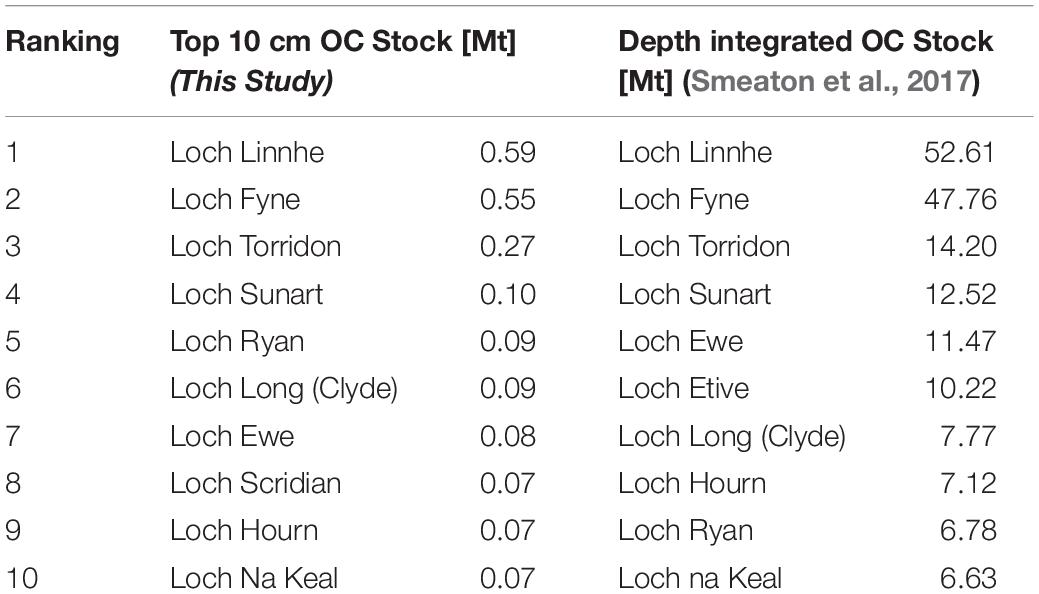
Table 3. Surficial (top 10 cm) OC stocks ranked in magnitude (Mt) compared to fully depth-integrated postglacial OC stocks (Smeaton et al., 2017).
Beyond regional differences in OC density, the sediment classes differ significantly with the muddy surficial sediments in Scottish and Irish systems holding 3596 ± 172 tonnes of OC km–2 respectively. These results highlight that fjords are “hotspots” for the burial and potential storage of OC; more so the results highlight that the muddy sediments themselves are OC hotspots within the fjords. By mapping the OC content of the surficial sediments (10 cm) we provide a tool for policy makers to potentially target areas for protection and or management of these important OC resources. For example, Figure 9 highlights that the mud and muddy sands within Scotland’s mainland fjords are where the greatest surficial OC stock are held and would be the areas where targeted management (i.e., protection from benthic disturbance) would have the most C benefits.
Revisiting Global OC Burial
While a focus on OC burial is not the purpose of this work, the results from the sediment and OC mapping highlight the need to revisit the current estimates of OC burial in fjords globally, particularly in light of our improved understanding of seabed heterogeneity. It is currently estimated that 21–31 Mt OC is buried in fjords sediments each year (Smith et al., 2015; Cui et al., 2016). But as previously stated, these calculations are based on the assumption that the seabed of these fjords are homogenous and covered in muddy sediments; an issue which impacts many marine OC stock estimates (Berner, 1982; Hedges et al., 1997). The mapping undertaken in this study estimates that 26–33% of the seabed of Scotland’s and Ireland’s fjords are dominated by muddy sediments (i.e., mud and muddy sands) which cover an area of 1,159 km2. Using these systems as a proxy for fjords globally we can calculate the muddy sediments within fjords potentially bury between 5.46 and 10.23 Mt OC yr–1. Globally fjords are estimated to cover an area of 455000 km2 (Dürr et al., 2011) if we assume that the areal coverage of the muddy sediments matches that of the Scottish systems it is estimated that globally the muddy sediments bury between 57 and 107 tonnes OC km–2 yr–1 which far exceeds all other marine environments (Berner, 1982; Hedges et al., 1997; Smith et al., 2015) with the upper range being similar to the OC rich intertidal environments (Duarte et al., 2013).
These estimates are highly speculative as there are significant differences between fjord systems around the world. Yet the results do emphasize that the muddy sediments within fjords are globally significant habitats for the capture and potential storage of OC. Perhaps more significantly, these results highlight that we know very little about the non-muddy sediments which potentially represent up to 74% of the seabed area within fjords. To better understand OC burial in these coarser sediments will require a concerted effort by the research community to sample the coarser sediments; these sediments have traditionally been overlooked in favor of finer grain sediments better suited to geochemical and paleo study.
This research outlines an approach to better understand the spatial heterogeneity of the type and OC content of fjordic and marine sediments more generally. If this methodological approach can be applied to fjord systems globally, it will provide a robust foundation from which to revisit and better constrain the current estimates of OC burial rates in these environments.
Conclusion
Understanding the spatial heterogeneity of seabed sediments in fjords is a crucial step toward quantifying OC stocks, the formation of long-term OC stores and any associated rates of change in these globally significant OC burial hotspots. There is now a growing international awareness that natural climate solutions exist which can deliver negative emissions potential for managing our atmospheric greenhouse gases. A fundamental opportunity therefore presents itself to society, namely to manage and protect these significant OC hotspots because of their potentially significant role in regulating global climate. We have demonstrated that when combined with the tiered mapping approach, both readily available point observation and backscatter data can produce equally robust predictions of sediment type and surficial sediment OC content. The results highlight that the seafloor of fjords are highly heterogeneous which is reflected in the distribution of the surficial OC within these systems. In addition, by improving our understanding of the seabed sediment, it has been possible to refine sedimentary OC stock (top 10 cm) estimates. Scotland’s fjord sediments are estimated to hold 4.16 ± 0.5 Mt OC; this is an order of magnitude higher than previous estimates (Burrows et al., 2014). The maps depicting the spatial distribution of sediment type and OC content are potentially powerful tools for policy makers and in support of coastal management decision making, allowing a future targeted approach to the protection of these important OC hotspots. Analysis of the mapped outputs highlight that the muddy sediments within the fjords of mainland Scotland hold the majority of this sedimentary OC stock and might therefore benefit most from targeted management measures to protect and preserve these long-term OC stores from anthropogenic disturbance.
Data Availability Statement
All datasets generated/analyzed for this study are included in the manuscript/Supplementary Files.
Author Contributions
CS led the conception and design of the study in conjunction with WA. CS undertook the research and wrote the first draft of the manuscript. Both authors contributed to the manuscript revision, and approved the submitted version.
Funding
This work was jointly supported by the Natural Environment Research Council (Grant Number NE/L501852/1) and Marine Scotland. Additional support from NERC/BBSRC (BB/M026620/1) and NERC Life Sciences Mass Spectrometry Facility (CEH_L_115_05_2018) allowed additional field and analytical work to be undertaken. BGS provided access to samples through there In-kind sample loan scheme (Loan: 237389).
Conflict of Interest
The authors declare that the research was conducted in the absence of any commercial or financial relationships that could be construed as a potential conflict of interest.
Acknowledgments
The authors would like to thank the captains, crews, and fellow scientists from the many research vessels used to collect the samples utilized in this study. The British Ocean Sediment Core Research Facility (BOSCORF) is thanked for supplying sediment samples. In addition, the authors would like to thank the staff of the BGS Core Store for their assistance in gaining access to samples. The authors would also like to thank the data managers at both BGS and UKHO for their help in gaining access to backscatter data and survey reports. Finally, the authors thank John Baxter and Ian Davies for comments on an early draft of the manuscript and the two reviewers for providing useful comments and guidance to improve the manuscript.
Supplementary Material
The Supplementary Material for this article can be found online at: https://www.frontiersin.org/articles/10.3389/feart.2019.00269/full#supplementary-material
References
Audsley, A., Arosio, R., and Howe, J. A. (2016). A geomorphological reconstruction of the deglaciation of loch etive during the loch lomond stadial. Scott. J. Geol. 52, 55–63. doi: 10.1144/sjg2015-004
Bao, R., Zhao, M., McNichol, A., Galy, V., McIntyre, C., Haghipour, N., et al. (2019). Temporal constraints on lateral organic matter transport along a coastal mud belt. Organ. Geochem. 128, 86–93. doi: 10.1016/j.orggeochem.2019.01.007
Bauer, J. E., Cai, W. J., Raymond, P. A., Bianchi, T. S., Hopkinson, C. S., and Regnier, P. A. (2013). The changing carbon cycle of the coastal ocean. Nature 504:61. doi: 10.1038/nature12857
Berner, R. A. (1982). Burial of organic carbon and pyrite sulfur in the modern ocean: its geochemical and environmental significance. Am. J. Sci. 282, 451–473. doi: 10.2475/ajs.282.4.451
Bockelmann, F. D., Puls, W., Kleeberg, U., Müller, D., and Emeis, K. C. (2018). Mapping mud content and median grain-size of North Sea sediments–A geostatistical approach. Mar. Geol. 397, 60–71. doi: 10.1016/j.margeo.2017.11.003
Brown, C. J., Beaudoin, J., Brissette, M., and Gazzola, V. (2019). Multispectral multibeam echo sounder backscatter as a tool for improved seafloor characterization. Geosciences 9:126. doi: 10.3390/geosciences9030126
Brown, C. J., and Collier, J. S. (2008). Mapping benthic habitat in regions of gradational substrata: an automated approach utilising geophysical, geological, and biological relationships. Estuar. Coast. Shelf Sci. 78, 203–214. doi: 10.1016/j.ecss.2007.11.026
Brown, C. J., Todd, B. J., Kostylev, V. E., and Pickrill, R. A. (2011). Image-based classification of multibeam sonar backscatter data for objective surficial sediment mapping of Georges Bank, Canada. Cont. Shelf Res. 31, S110–S119.
Burrows, M. T., Hughes, D. J., Austin, W. E. N., Smeaton, C., Hicks, N., Howe, J. A., et al. (2017). Assessment of Blue Carbon Resources in Scotland’s Inshore Marine Protected Area Network Scottish Natural Heritage Commissioned. Report. No. 957. St Andrews: University of St Andrews.
Burrows, M. T., Kamenos, N. A., Hughes, D. J., Stahl, H., Howe, J. A., and Tett, P. (2014). Assessment of Carbon Budgets and Potential Blue Carbon Stores in Scotland’s Coastal and Marine Environment Scottish Natural Heritage Commissioned. Report. No. 761. Scotland: Scottish Natural Heritage.
Calvert, J., Strong, J. A., Service, M., McGonigle, C., and Quinn, R. (2014). An evaluation of supervised and unsupervised classification techniques for marine benthic habitat mapping using multibeam echosounder data. ICES J. Mar. Sci. 72, 1498–1513. doi: 10.1093/icesjms/fsu223
Chiles, J. P., and Delfiner, P. (2009). Geostatistics: Modeling Spatial Uncertainty. Hoboken, NJ: John Wiley & Sons.
Cui, X., Bianchi, T. S., Savage, C., and Smith, R. W. (2016). Organic carbon burial in fjords: terrestrial versus marine inputs. Earth Planet. Sci. Lett. 451, 41–50. doi: 10.1016/j.scitotenv.2018.04.242
Dadey, K. A., Janecek, T., and Klaus, A. (1992). “Dry-bulk density: its use and determination,” in Proceedings of the Ocean Drilling Program, Scientific Results, College Station, TX.
Diesing, M., Kröger, S., Parker, R., Jenkins, C., Mason, C., and Weston, K. (2017). Predicting the standing stock of organic carbon in surface sediments of the North–West European continental shelf. Biogeochemistry 135, 183–200. doi: 10.1007/s10533-017-0310-4
Duarte, C. M., Losada, I. J., Hendriks, I. E., Mazarrasa, I., and Marbà, N. (2013). The role of coastal plant communities for climate change mitigation and adaptation. Nat. Clim. Chang. 3:961. doi: 10.1371/journal.pone.0044727
Dürr, H. H., Laruelle, G. G., van Kempen, C. M., Slomp, C. P., Meybeck, M., and Middelkoop, H. (2011). Worldwide typology of nearshore coastal systems: defining the estuarine filter of river inputs to the oceans. Estuar. Coast. 34, 441–458. doi: 10.1007/s12237-011-9381-y
Edwards, A., and Sharples, F. (1986). Scottish Sea Lochs: A Catalogue. Scottish Marine Biological Association. Peterborough: Nature Conservancy Council.
Ehlers, J., Gibbard, P. L., and Rose, J. (1991). Glacial Deposits in Great Britain and Ireland. Rotterdam: Balkema.
Flemming, B. W., and Delafontaine, M. T. (2000). Mass physical properties of muddy intertidal sediments: some applications, misapplications and non-applications. Cont. Shelf Res. 20, 1179–1197. doi: 10.1016/s0278-4343(00)00018-2
Folk, R. L. (1954). The distinction between grain size and mineral composition in sedimentary-rock nomenclature. J. Geol. 62, 344–359. doi: 10.1086/626171
Friedrich, J., Janssen, F., Aleynik, D., Bange, H. W., Boltacheva, N. A., Çağatay, M. N., et al. (2014). Investigating hypoxia in aquatic environments: diverse approaches to addressing a complex phenomenon. Biogeosciences 11, 1215–1259. doi: 10.5194/bg-11-1215-2014
Guinan, J., Charlesworth, M., Service, M., and Oliver, T. (2001). Sources and geochemical constraints of polycyclic aromatic hydrocarbons (PAHs) in sediments and mussels of two Northern Irish Sea-loughs. Mar. Pollut. Bull. 42, 1073–1081. doi: 10.1016/s0025-326x(01)00077-7
Harris, D., Horwáth, W. R., and Van Kessel, C. (2001). Acid fumigation of soils to remove carbonates prior to total organic carbon or carbon-13 isotopic analysis. Soil Sci. Soc. Am. J. 65, 1853–1856.
Hedges, J. I., Keil, R. G., and Benner, R. (1997). What happens to terrestrial organic matter in the ocean? Organ. Geochem. 27, 195–212. doi: 10.1016/s0146-6380(97)00066-1
Howe, J. A., Austin, W. E., Forwick, M., Paetzel, M., Harland, R., and Cage, A. G. (2010). Fjord systems and archives: a review. Geol. Soc. Spec. Publ. 344, 5–15. doi: 10.1144/sp344.2
Howe, J. A., Shimmield, T., Austin, W. E., and Longva, O. (2002). Post-glacial depositional environments in a mid-high latitude glacially-overdeepened sea loch, inner Loch Etive, western Scotland. Mar. Geol. 185, 417–433. doi: 10.1016/s0025-3227(01)00299-7
Ierodiaconou, D., Monk, J., Rattray, A., Laurenson, L., and Versace, V. L. (2011). Comparison of automated classification techniques for predicting benthic biological communities using hydroacoustics and video observations. Cont. Shelf Res. 31, S28–S38.
Inall, M., Cottier, F., Griffiths, C., and Rippeth, T. (2004). Sill dynamics and energy transformation in a jet fjord. Ocean Dyn. 54, 307–314.
Kaskela, A. M., Kotilainen, A. T., Alanen, U., Cooper, R., Green, S., Guinan, J., et al. (2019). Picking up the pieces–Harmonising and collating seabed substrate data for European maritime areas. Geosciences 9:84. doi: 10.3390/geosciences9020084
Keil, R. G., Mayer, L. M., Quay, P. D., Richey, J. E., and Hedges, J. I. (1997). Loss of organic matter from riverine particles in deltas. Geochim. Cosmochim. Acta 61, 1507–1511. doi: 10.1016/s0016-7037(97)00044-6
Lark, R. M., Dove, D., Green, S. L., Richardson, A. E., Stewart, H., and Stevenson, A. (2012). Spatial prediction of seabed sediment texture classes by cokriging from a legacy database of point observations. Sediment. Geol. 281, 35–49. doi: 10.1016/j.sedgeo.2012.07.009
Lark, R. M., Marchant, B. P., Dove, D., Green, S. L., Stewart, H., and Diesing, M. (2015). Combining observations with acoustic swath bathymetry and backscatter to map seabed sediment texture classes: the empirical best linear unbiased predictor. Sediment. Geol. 328, 17–32. doi: 10.1016/j.sedgeo.2015.07.012
Lo Giudice Cappelli, E., Clarke, J. L., Smeaton, C., and Davidson, K. (2019). Organic carbon rich sediments: benthic foraminifera as bio-indicators of depositional environments. Biogeosciences 16, 3725–3746. doi: 10.5194/bg-2019-2125
Luisetti, T., Turner, R. K., Andrews, J. E., Jickells, T. D., Kröger, S., Diesing, M., et al. (2019). Quantifying and valuing carbon flows and stores in coastal and shelf ecosystems in the UK. Ecosyst. Serv. 35, 67–76. doi: 10.1016/j.ecoser.2018.10.013
Mayer, L. M. (1994). Relationships between mineral surfaces and organic carbon concentrations in soils and sediments. Chem. Geol. 114, 347–363. doi: 10.1016/0009-2541(94)90063-9
McBreen, F., Wilson, J. G., Mackie, A. S., and Aonghusa, C. N. (2008). Seabed mapping in the southern Irish Sea: predicting benthic biological communities based on sediment characteristics. Chall. Mar. Ecosyst. 606, 93–103. doi: 10.1007/978-1-4020-8808-7_9
McIntosh, A. D., Fryer, R. J., Webster, L., and Cundy, A. B. (2012). Long-term fate of polycyclic aromatic hydrocarbons (PAH) in sediments from loch leven after closure of an aluminium smelter. J. Environ. Monit. 14, 1335–1344. doi: 10.1039/c2em11006g
Middelburg, J. J., and Levin, L. A. (2009). Coastal hypoxia and sediment biogeochemistry. Biogeosciences 6, 1273–1293. doi: 10.5194/bg-6-1273-2009
Mitchell, P. J., Aldridge, J., and Diesing, M. (2019). Legacy data: how decades of seabed sampling can produce robust predictions and versatile products. Geosciences 9:182. doi: 10.3390/geosciences9040182
Nairn, R. (2005). Ireland’s Coastline: Exploring its Nature and Heritage. West Link Park: Collins Press.
O’Cofaigh, C., Weilbach, K., Lloyd, J. M., Benetti, S., Callard, S. L., Purcell, C., et al. (2019). Early deglaciation of the British-Irish ice sheet on the Atlantic shelf northwest of Ireland driven by glacioisostatic depression and high relative sea level. Quat. Sci. Rev. 208, 76–96. doi: 10.1016/j.quascirev.2018.12.022
Peters, J. L., Benetti, S., Dunlop, P., and Cofaigh, C. O. (2015). Maximum extent and dynamic behaviour of the last British–Irish ice sheet west of Ireland. Quat. Sci. Rev. 128, 48–68. doi: 10.1016/j.quascirev.2015.09.015
Russell, M., Robinson, C. D., Walsham, P., Webster, L., and Moffat, C. F. (2011). Persistent organic pollutants and trace metals in sediments close to scottish marine fish farms. Aquaculture 319, 262–271. doi: 10.1016/j.aquaculture.2011.06.030
Russell, M., Webster, L., Walsham, P., Packer, G., Dalgarno, E. J., McIntosh, A. D., et al. (2008). Composition and concentration of hydrocarbons in sediment samples from the oil producing area of the East Shetland Basin, Scotland. J. Environ. Monit. 10, 559–569. doi: 10.1039/b715765g
Serpetti, N., Heath, M., Rose, M., and Witte, U. (2012). High resolution mapping of sediment organic matter from acoustic reflectance data. Hydrobiologia 680, 265–284. doi: 10.1007/s10750-011-0937-4
Sinnot, A. M., and Devoy, R. J. N. (1992). The geomorphology of Ireland’s coastline: patterns, processes and future prospects. Hommes Terres Nord 3, 145–153. doi: 10.3406/htn.1992.2370
Smeaton, C., Austin, W., Davies, A., Baltzar, A., Abell, R. E., and Howe, J. A. (2016). Substantial stores of sedimentary carbon held in mid-latitude fjords. Biogeosciences 13, 5771–5787. doi: 10.5194/bg-13-5771-2016
Smeaton, C., and Austin, W. E. (2017). Sources, sinks, and subsidies: terrestrial carbon storage in mid-latitude fjords. J. Geophys. Res. Biogeosci. 122, 2754–2768. doi: 10.1002/2017jg003952
Smeaton, C., Austin, W. E., Davies, A., Baltzer, A., Howe, J. A., and Baxter, J. M. (2017). Scotland’s forgotten carbon: a national assessment of mid-latitude fjord sedimentary stocks. Biogeosciences 14, 5663–5674. doi: 10.5194/bg-14-5663-2017
Smith, R. W., Bianchi, T. S., Allison, M., Savage, C., and Galy, V. (2015). High rates of organic carbon burial in fjord sediments globally. Nat. Geosci. 8, 450–453. doi: 10.1038/ngeo2421
Staalstrøm, A., and Røed, L. P. (2016). Vertical mixing and internal wave energy fluxes in a sill fjord. J. Mar. Syst. 159, 15–32. doi: 10.1016/j.jmarsys.2016.02.005
Stigebrandt, A. (2012). “Hydrodynamics and circulation of fjords,” in Encyclopedia of Lakes and Reservoirs. Encyclopedia of Earth Sciences Series, eds L. Bengtsson, R. W. Herschy, and R. W. Fairbridge, (Dordrecht: Springer), 327–344. doi: 10.1007/978-1-4020-4410-6_247
Syvitski, J. P., Burrell, D. C., and Skei, J. M. (1987). Fjords: Processes and Products. Berlin: Springer Science & Business Media.
Syvitski, J. P., and Shaw, J. (1995). Sedimentology and geomorphology of fjords. Dev. Sedimentol. 53, 113–178. doi: 10.1016/s0070-4571(05)80025-1
Webster, L., Fryer, R. J., Dalgarno, E. J., Megginson, C., and Moffat, C. F. (2001). The polycyclic aromatic hydrocarbon and geochemical biomarker composition of sediments from voes and coastal areas in the Shetland and Orkney Islands. J. Environ. Monit. 3, 591–601. doi: 10.1039/B106408H
Webster, L., Fryer, R. J., Megginson, C., Dalgarno, E. J., McIntosh, A. D., and Moffat, C. F. (2004). The polycyclic aromatic hydrocarbon and geochemical biomarker composition of sediments from sea lochs on the west coast of Scotland. J. Environ. Monit. 6, 219–228.
Webster, L., McIntosh, A. D., Dalgarno, E. J., Megginson, C., Shepherd, N. J., and Moffat, C. F. (2003). The polycyclic aromatic hydrocarbon composition of mussels (Mytilus edulis) from Scottish coastal waters. J. Environ. Monit. 5, 150–159. doi: 10.1039/b208691n
Webster, L., McIntosh, A. D., Moffat, C. F., Dalgarno, E. J., Brown, N. A., and Fryer, R. J. (2000). Analysis of sediments from Shetland Island voes for polycyclic aromatic hydrocarbons, steranes and triterpanes. J. Environ. Monit. 2, 29–38. doi: 10.1039/a907556i
Wilson, R. J., Speirs, D. C., Sabatino, A., and Heath, M. R. (2018). A synthetic map of the north-west European shelf sedimentary environment for applications in marine science. Earth Syst. Sci. Data 10, 109–130. doi: 10.5194/essd-10-109-2018
Woulds, C., Bouillon, S., Cowie, G. L., Drake, E., Middelburg, J. J., and Witte, U. (2016). Patterns of carbon processing at the seafloor: the role of faunal and microbial communities in moderating carbon flows. Biogeosciences 13, 4343–4357. doi: 10.5194/bg-13-4343-2016
Keywords: fjord, carbon, sediment, spatial, Scotland, mid-latitude, organic carbon, mapping
Citation: Smeaton C and Austin WEN (2019) Where’s the Carbon: Exploring the Spatial Heterogeneity of Sedimentary Carbon in Mid-Latitude Fjords. Front. Earth Sci. 7:269. doi: 10.3389/feart.2019.00269
Received: 08 May 2019; Accepted: 30 September 2019;
Published: 24 October 2019.
Edited by:
Michael Andrew Clare, University of Southampton, United KingdomReviewed by:
Zhixiong Shen, Coastal Carolina University, United StatesValier Galy, Woods Hole Oceanographic Institution, United States
Copyright © 2019 Smeaton and Austin. This is an open-access article distributed under the terms of the Creative Commons Attribution License (CC BY). The use, distribution or reproduction in other forums is permitted, provided the original author(s) and the copyright owner(s) are credited and that the original publication in this journal is cited, in accordance with accepted academic practice. No use, distribution or reproduction is permitted which does not comply with these terms.
*Correspondence: Craig Smeaton, Y3MyNDRAc3QtYW5kcmV3cy5hYy51aw==