- 1School of Earth and Environment, University of Leeds, Leeds, United Kingdom
- 2Department of Earth Sciences, University of Cambridge, Cambridge, United Kingdom
- 3GFZ German Research Centre for Geosciences, Potsdam, Germany
- 4Institute of Geosciences, University of Potsdam, Potsdam, Germany
- 5EarthByte Group, School of Geosciences, The University of Sydney, Sydney, NSW, Australia
Carbon is a key control on the surface chemistry and climate of Earth. Significant volumes of carbon are input to the oceans and atmosphere from deep Earth in the form of degassed CO2 and are returned to large carbon reservoirs in the mantle via subduction or burial. Different tectonic settings (e.g., volcanic arcs, mid-ocean ridges, and continental rifts) emit fluxes of CO2 that are temporally and spatially variable, and together they represent a first-order control on carbon outgassing from the deep Earth. A change in the relative importance of different tectonic settings throughout Earth’s history has therefore played a key role in balancing the deep carbon cycle on geological timescales. Over the past 10 years the Deep Carbon Observatory has made enormous progress in constraining estimates of carbon outgassing flux at different tectonic settings. Using plate boundary evolution modeling and our understanding of present-day carbon fluxes, we develop time series of carbon fluxes into and out of the Earth’s interior through the past 200 million years. We highlight the increasing importance of carbonate-intersecting subduction zones over time to carbon outgassing, and the possible dominance of carbon outgassing at continental rift zones, which leads to maxima in outgassing at 130 and 15 Ma. To a first-order, carbon outgassing since 200 Ma may be net positive, averaging ∼50 Mt C yr–1 more than the ingassing flux at subduction zones. Our net outgassing curve is poorly correlated with atmospheric CO2, implying that surface carbon cycling processes play a significant role in modulating carbon concentrations and/or there is a long-term crustal or lithospheric storage of carbon which modulates the outgassing flux. Our results highlight the large uncertainties that exist in reconstructing the corresponding in- and outgassing fluxes of carbon. Our synthesis summarizes our current understanding of fluxes at tectonic settings and their influence on atmospheric CO2, and provides a framework for future research into Earth’s deep carbon cycling, both today and in the past.
Introduction
The deep carbon cycle of the Earth connects the exospheric (atmosphere, hydrosphere, biosphere) reservoirs of carbon to those in the crust and mantle (e.g., Dasgupta and Hirschmann, 2010). The non-anthropogenic concentration of atmospheric CO2 is modulated by outgassing from the crust and mantle at plate boundary and intraplate settings (e.g., Self et al., 2005; van der Meer et al., 2014) and metamorphic carbon release (e.g., Skelton, 2011). Ingassing returns carbon to the crust and mantle through processes such as silicate weathering (e.g., Dessert et al., 2003) and subduction of altered oceanic crust, serpentinized lithospheric mantle, and seafloor sediments (e.g., Alt and Teagle, 1999; Alt et al., 2013; Plank, 2014).
Past work on links between tectonic parameters and carbon fluxes have often focused solely on the present day (e.g., Dasgupta and Hirschmann, 2010; Kelemen and Manning, 2015), or on the importance of specific tectonic settings through time (e.g., van der Meer et al., 2014; McKenzie et al., 2016; Brune et al., 2017). Early attempts at reconciling both present-day fluxes and past tectonically driven carbon cycling have been qualitative due to the immense uncertainties in quantifying present-day carbon degassing (e.g., Kerrick, 2001), or have attempted to tackle carbon cycling on a coarse scale for the entirety of Earth history (e.g., Dasgupta, 2013).
Through the DECADE program of the Deep Carbon Observatory, we now have a much improved understanding of the present-day outgassing rate of carbon, and its variability, in a range of tectonic settings. In tandem with the rapid increase in observations of carbon outgassing, progress has been made in modeling plate tectonics over geological time, e.g., through the development of open-source and community tools like GPlates (e.g., Gurnis et al., 2012; Müller et al., 2018), which allow reconstruction and quantification of tectonic plate boundary lengths, slab fluxes, and other tectonic parameters during much of the Phanerozoic (e.g., Matthews et al., 2016). In this study, we compile and review estimates of carbon fluxes into and out of the mantle over the past 200 Ma at plate boundaries, by combining our understanding of present-day fluxes with plate tectonic reconstructions. In doing so, we assess the effects of plate tectonics on the deep carbon cycle, and the implications for the surface environment.
Background and Methods
Carbon enters the mantle through the subduction of carbon-bearing phases in the oceanic lithosphere at subduction zones, and is returned to Earth’s surface during magmatic or tectonic activity (Figure 1). Therefore, the global distribution of plate boundaries is a primary control on the magnitude of solid Earth carbon degassing. To model tectonic parameters over geological time, we use GPlates, an open-source software for plate reconstructions (Müller et al., 2018 and references therein). In addition to illustrating past tectonic plate distributions up to 1000 Ma (e.g., Matthews et al., 2016; Müller et al., 2016; Merdith et al., 2017, 2019), GPlates has been previously used as a basis to extrapolate paleogeography (Cao et al., 2017b), past seafloor sediment thicknesses (Dutkiewicz et al., 2017), and the distribution of continental flood basalts and oceanic plateaus (Johansson et al., 2018). We utilize the open access global plate motion model of Müller et al. (2016) to analyze plate boundary length (Figure 2), which captures the past 200 million years of plate tectonic evolution based on paleomagnetic data, seafloor isochrons, and other plate motion indicators (e.g., ocean island chains). Alongside this model we use the subduction history data of East et al. (2019) to determine subduction flux parameters. Plate boundary lengths and other parameters from these models provide a basis for our tectonic carbon flux estimates in 1 Myr intervals. These parameters are provided in Supplementary Table S1. Uncertainties are determined through standard error propagation of uncertainties within present-day carbon flux measurements (see Table 1) and the standard deviation of computed tectonic parameters.
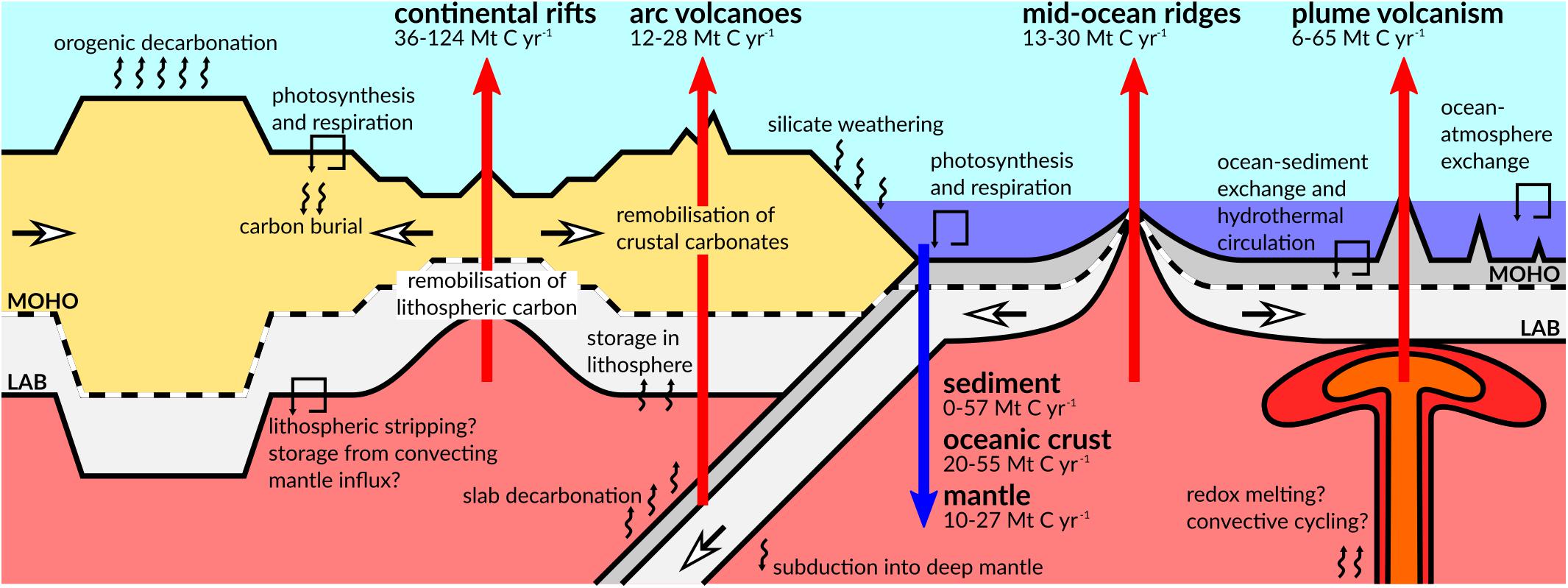
Figure 1. Cartoon illustrating major fluxes of carbon to, from, and within the Earth’s exogenic and endogenic systems. The fluxes discussed in this paper are highlighted as large colored arrows; the small arrows represent other processes occurring within each system that are not considered in this study. Values give the maximum and minimum fluxes since 200 Ma for each setting discussed. The two major boundaries highlighted are the Mohorovičić discontinuity (crust-mantle boundary; Moho) and the lithosphere-asthenosphere boundary (LAB).
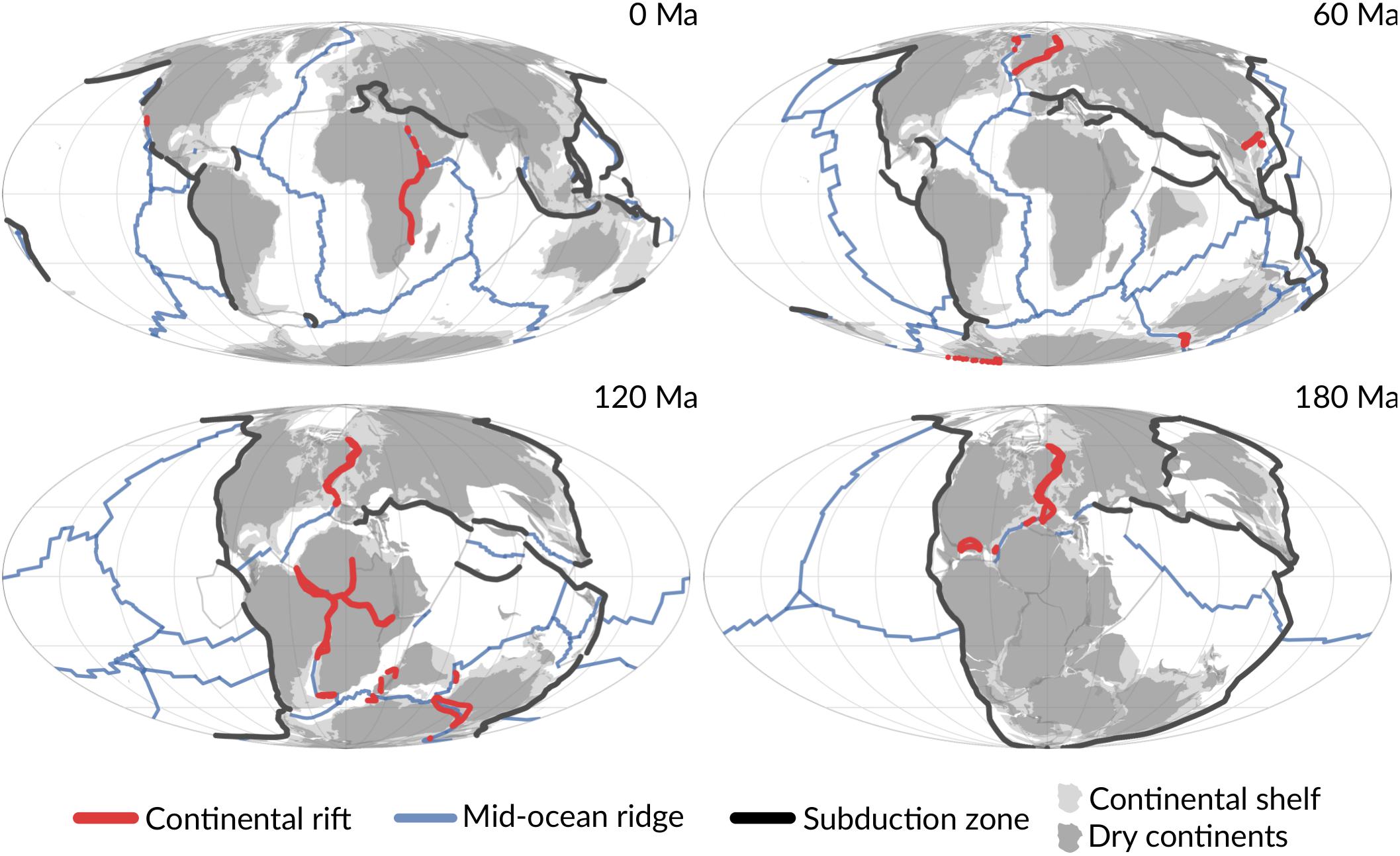
Figure 2. Plate tectonic reconstructions of key plate boundary types at 60 Myr intervals from the present-day using the model of Müller et al. (2016).
In this synthesis, we contrast the quantity of carbon that is outgassed at various tectonic settings against the carbon returned to deep Earth at subduction zones (Figure 1). Hence, processes such as decarbonation efficiency in the subducted slab beneath the crust-mantle boundary (e.g., Johnston et al., 2011), biogeochemical cycling (e.g., Lenton et al., 2018), and carbon drawdown from silicate weathering at the surface Earth (e.g., Dessert et al., 2003; Johansson et al., 2018) are not considered.
Results
Carbon Ingassing
The carbon entering the mantle at subduction zones can be subdivided into three constituent components which comprise the total carbon in a subducting oceanic lithosphere slab: the carbon locked within altered oceanic crust, the carbon present within serpentinized mantle, as well as organic and inorganic carbon present in deep-sea sediments (Kelemen and Manning, 2015). During subduction, carbon may be liberated from the slab through metamorphic decarbonation (e.g., Kerrick and Connolly, 2001), by dissolution of carbonate phases directly into a supercritical fluid (Manning, 2004; Facq et al., 2014) or through the formation of carbonatitic melts (Poli, 2015), thereby entering the mantle wedge. Carbon may also be subducted deeper into the mantle (e.g., Thomson et al., 2014, 2016).
Liberated slab carbon, alongside other volatile components, enters the overlying mantle as a low density, volatile-rich fluid phase. Such fluids depress the solidus of peridotite, and can promote melting in asthenospheric mantle (e.g., Dasgupta and Hirschmann, 2006, 2010), in which case carbon partitions into the melt and is later released as CO2 gas during volcanic eruptions. However, carbon-bearing fluids may also stall in the lithosphere as a result of this solidus depression, and subsequently freeze to form metasomatic layers and veins beneath the continents (e.g., McKenzie, 1989; Rooney et al., 2017).
The presence of surface-derived carbon in the deep mantle is inferred from the isotopic composition of superdeep diamonds (from sub-lithospheric depths of >250 km). Many superdeep diamonds host carbon and oxygen isotope ratios indicative of a biogenic endmember (Thomson et al., 2014; Burnham et al., 2015) and mafic inclusions with similar compositions to mid-ocean ridge basalt (MORB) (Thomson et al., 2016), which may suggest the existence of a thermal/compositional barrier to deep carbon subduction. While light carbon isotope ratios can arise from fractionation both prior to and during diamond growth (e.g., Cartigny et al., 2001), Smart et al. (2011) demonstrated that Rayleigh fractionation cannot produce extreme δ13C values, instead invoking the presence of recycled organic matter during diamond synthesis. Material subducted into the convecting mantle can therefore return to the surface, illustrating the vast scale of deep carbon cycling.
Altered Oceanic Crust
Carbon, sourced from magmatic fluids and dissolved inorganic and organic components in seawater, is fixed into oceanic crust as carbonate and carbon-bearing minerals during hydrothermal alteration at mid-ocean ridges largely during the first few tens of millions of years after crust formation (Alt and Teagle, 1999; Shilobreeva et al., 2011; Coogan et al., 2016). The amount of carbon fixed into oceanic crust and accumulated in the overlying sediments is a function of multiple variables. The spreading rate of a mid-ocean ridge determines the rate at which carbonate from sedimentary material leaches into crust; older oceanic crust will host more carbonate than younger crust; higher bottom water temperatures change the chemistry of seawater, promoting higher seawater Ca concentrations and encouraging carbonate precipitation (Gillis and Coogan, 2011; Coogan and Gillis, 2013; Coogan and Dosso, 2015). In addition, erupted basalts in the uppermost part of oceanic crust gain higher carbon concentrations than crustal gabbros through this mechanism (Staudigel et al., 1989; Gillis and Coogan, 2011).
Our understanding of carbon in altered oceanic crust results from ocean crust drilling (e.g., Staudigel et al., 1989; Alt and Teagle, 1999). Using the data collected from such programs, Müller and Dutkiewicz (2018) determined the magnitude of subducted carbon in oceanic crust by determining oceanic crust carbon concentrations as a function of crustal age, and bottom water temperature. From this parameterization of crustal carbon concentrations, the mean oceanic carbon concentration and its standard deviation can be determined for the past 200 Myrs. This model is used in our synthesis to account for subducted crustal carbon (Figure 3).
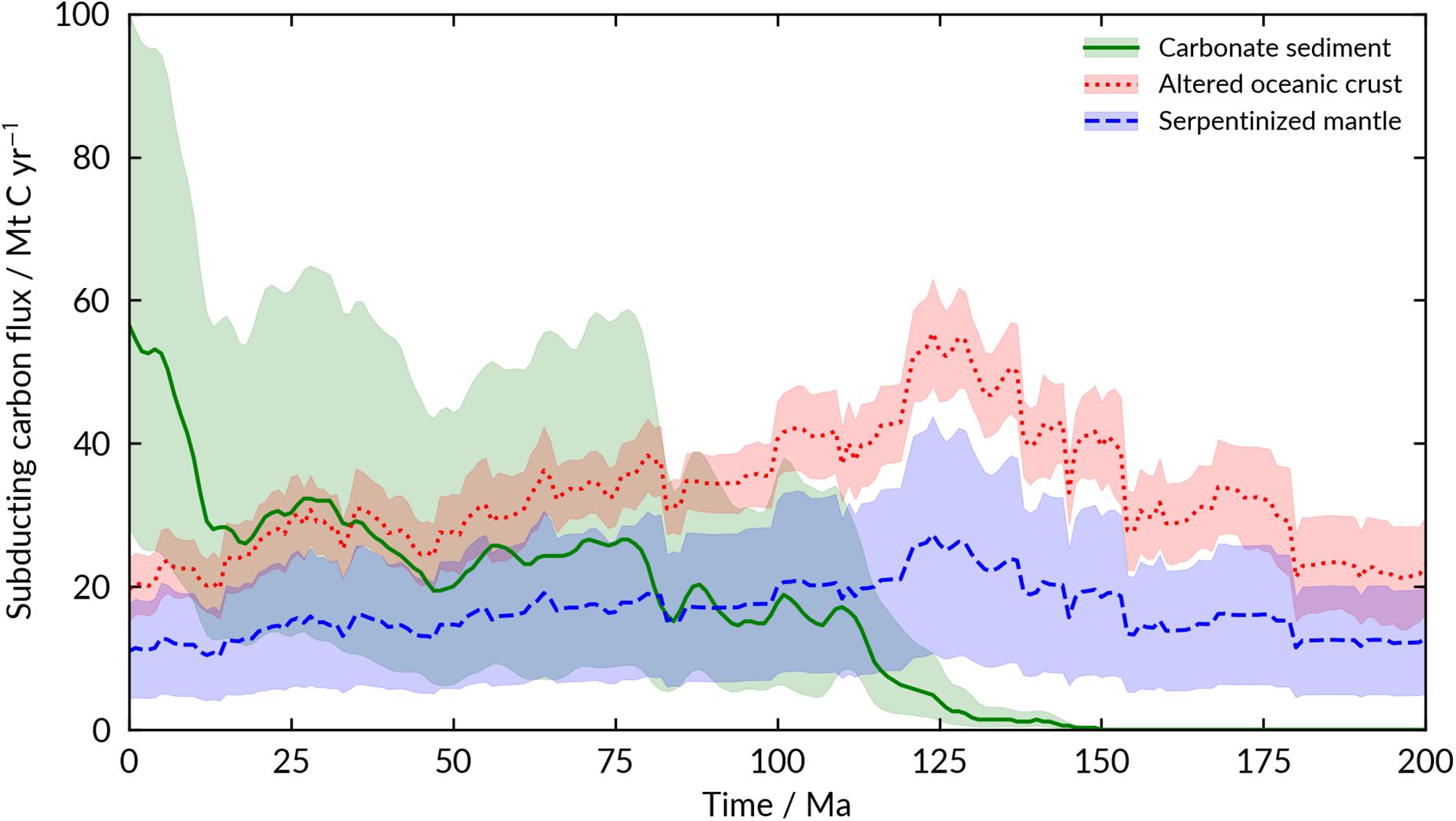
Figure 3. Estimated input of carbon into subduction zones since 200 Ma, divided into the different source lithologies (altered crust, sedimentary carbonate, and serpentinized mantle). The time series for altered crust and sedimentary carbonate are from Müller and Dutkiewicz (2018) and Dutkiewicz et al. (2018), respectively. The increase in sedimentary carbonate arising from the Mesozoic Marine Revolution is included from 150 Myr onward, when sedimentary carbonate input commenced due to an evolutionary radiation of biomineralizing metazoans (e.g., Edmond and Huh, 2003).
Serpentinized Mantle
Bending of a plate at the outer rise before subduction at the trench produces normal faults that cut through the brittle lithosphere (e.g., Christensen and Ruff, 1988). These faults permit the infiltration of water into the lithospheric mantle and promote hydrous alteration and serpentinization (Ranero et al., 2003; Lefeldt et al., 2012); such alteration is evident from lower seismic velocities than those measured in unaltered mantle (e.g., van Avendonk et al., 2011). Models of outer rise serpentinization have shown that the extent of faulting, and hence serpentinization, are dependent on multiple parameters including crustal age, plate velocity, and coupling between the downgoing and overriding plate (Rüpke et al., 2004; Naliboff et al., 2013).
Serpentinites may host a significant amount of carbon (Alt et al., 2013). Present-day serpentinized mantle carbon ingassing flux is likely of the same order of magnitude to carbon subducted in altered crust (Alt et al., 2013; Dasgupta, 2013; Kelemen and Manning, 2015), and is hence an important contributing factor to the total carbon ingassing flux. We adopt the average serpentinite carbon concentration value of 681 ± 45 ppm of Kelemen and Manning (2015) to estimate subducted serpentinized mantle carbon through time using the model of Müller et al. (2016) (Figure 3). In addition, we take a serpentinization extent of 20 ± 10% and a serpentinized mantle thickness of 10.5 ± 3.5 km to determine total altered mantle contributions during subduction (Kelemen and Manning, 2015).
In addition to serpentinization immediately prior to subduction, mantle peridotite may also be serpentinized near mid-ocean ridges. Mantle peridotite is exposed at slow- and ultra-slow spreading ridges (White et al., 2001), and constitutes as much as 25% of oceanic crust produced at these settings (Alt et al., 2013). Carbon fixation into exposed seafloor serpentinite has been estimated at 1.1–2.7 Mt C yr–1 (Schwarzenbach et al., 2013). However, plate reconstructions suggest that the global root-mean-square ridge spreading rates in the past 200 Ma were rarely lower than the present-day (Seton et al., 2012; Müller et al., 2016). The subduction of exposed serpentinized peridotite has therefore contributed only a small and likely unchanging proportion of carbon to the ingassing flux, such that the contribution from serpentinized peridotites at slow-spreading ridges lies within calculated error of our flux from altered crust and serpentinized mantle estimates. More detailed work is required to reduce the uncertainties related to serpentinization processes throughout the plate reconstruction timeframe.
Carbonate Sediments
The composition and distribution of pelagic ocean sediments are highly variable (Plank and Langmuir, 1998; Plank, 2014; Dutkiewicz et al., 2015, 2016; Clift, 2017). A significant proportion of seafloor sediments are carbon-bearing (e.g., Plank, 2014), and are derived from the weathering of continental crust, accumulation of the skeletal components of biomineralizing organisms, and unreduced biological material (e.g., Dutkiewicz et al., 2015). A major control on the quantity of carbon sequestered within sediments is the carbonate compensation depth (CCD), the water depth at which carbonate dissolution exceeds carbonate deposition (e.g., van Andel, 1975; Ridgwell and Zeebe, 2005). The CCD is known to be well-buffered against carbon cycle perturbations, but may be subject to major changes in carbon cycle dynamics (Pälike et al., 2012). One such change during the Oligocene resulted in a deepening of the global CCD of more than a kilometer (van Andel, 1975).
The depth of the CCD is dependent on several factors, including the rate and composition of terrestrial sediment flux (Walling and Fang, 2003), biological productivity (Kumar et al., 1995; Sarmiento et al., 2004), and sea-surface temperature and salinity (Dutkiewicz et al., 2016). Sediment composition can additionally be considered a function of latitude as a result of increased biological productivity and continental weathering at lower latitudes, which has been suggested to have a direct influence on the CO2 degassed from arc volcanism (Plank, 2014; Aiuppa et al., 2017; Clift, 2017). The compositional and lateral heterogeneity of sediments affects the role subducted sediment can play in the deep carbon cycle.
In addition, the evolution of ocean phytoplankton must be considered. Although carbonate-biomineralizing metazoans are known from the Neoproterozoic (e.g., Grant, 1990), it was not until the mid-Mesozoic that the marine carbonate cycle became more representative of the present-day. The Mesozoic Marine Revolution (MMR) at ∼150 Ma saw an evolutionary radiation in biomineralizing faunas and calcareous plankton which affected the operation of ocean geochemistry (Falkowski et al., 2004; Ridgwell, 2005; Erba, 2006). Edmond and Huh (2003) suggest that prior to the MMR little sedimentary carbonate could be contributed to subduction zones, with sedimentation instead dominated by opal.
Carbon fractions and mass fluxes in subduction zone sediments have been characterized through ocean drilling programs (Rea and Ruff, 1996; Plank and Langmuir, 1998; Plank, 2014). The average present-day subducted sediment is taken as GLOSS II by Plank (2014), which comprises 3.07 ± 0.23 wt% CO2 (0.84 ± 0.06 wt% C). Carbon and helium isotope systematics have also previously been used to differentiate between sources of carbon contributing to arc volcanic CO2 emissions (Sano and Williams, 1996; Hilton et al., 2002). This method considers three principal sources of carbon at subduction zones: the mantle, marine carbonates, and organic carbon, however, it does not distinguish the role of carbon present in arc crust (Mason et al., 2017).
Such methods have yielded estimates of carbonate subduction at the present-day. The review of Kelemen and Manning (2015) utilizes a flux of 13–23 Mt C yr–1 at the present-day after Plank (2014). Clift (2017), on the other hand, suggests a much larger present-day flux of ∼60 Mt C yr–1 which additionally accounts for subduction accretion processes, of which ∼48 Mt C yr–1 is carbonate and the rest, organic carbon. By considering the factors controlling the position of the CCD and hence the proportion of carbon within ocean sediments, Dutkiewicz et al. (2018) determined subducted sedimentary carbonate fluxes for the past 120 Myr, including a present-day estimate of 57 Mt C yr–1. Here, we adopt the model of Dutkiewicz et al. (2018) to estimate carbonate subduction through geological time (Figure 3). To account for the MMR, we assume that the sedimentary carbonate flux prior to 150 Ma is negligible as an extreme case.
Like Dutkiewicz et al. (2018), we do not consider accretion of sediments onto arc crust. While accretionary prism growth could account for removal of as much as 70% of sediment entering the trench at present-day subduction zones (von Huene and Scholl, 1991), Dutkiewicz et al. (2018) note that accretionary processes are difficult to constrain for the geological past. We therefore follow their reasoning in assuming all sediment entering subduction zone trenches enters the mantle. Modeling organic carbon sediment subduction is beyond the scope of this paper, but can be considered significant; organic carbon may comprise as much as 1 wt% of carbonate-free sediments (e.g., Kelemen and Manning, 2015). The present-day organic carbon flux is thought to contribute ∼20% of the total subducting carbon sediment flux (Clift, 2017). While our percentage uncertainties for carbonate subduction are typically greater than 20% and may encompass the uncertainty in organic carbon subduction, we acknowledge that the total carbon subducted within sediments has not been completely accounted for.
Carbon Outgassing
Volcanoes are traditionally considered to be the principal natural sources of carbon to the atmosphere, whereas tectonic degassing along fault systems is considered to be an additional, possibly substantial source of CO2 (Kerrick, 2001). Over the past few decades, significant progress has been made in estimating the total flux and provenance of carbon from volcanic regions (e.g., Burton et al., 2013; Aiuppa et al., 2017, 2019; Werner et al., 2019), which has allowed the semi-permanent instrumentation of >20 of the top outgassing volcanoes worldwide (Carn et al., 2016, 2017), as well as large number of campaign measurements (e.g., Aiuppa et al., 2017, 2019). Recent studies highlight the importance of diffuse carbon outgassing away from volcanic centers for the outgassing carbon budget (Lee et al., 2016; Tamburello et al., 2018). In this case, carbon-bearing fluids are transported through faults and fractures. This link between faulting, fluid migration and carbon degassing has been documented in continental rifts and along transform faults (Kennedy et al., 1997; Ring et al., 2016) and is further supported by the occurrence of deep earthquake swarms detected in continental rifts worldwide (Ibs-von Seht et al., 2008).
Volcanic Degassing
There are numerous challenges associated with the estimation of volcanic carbon fluxes (Burton et al., 2013; Werner et al., 2019). Owing to the large background concentration of CO2 in our atmosphere, CO2 fluxes from volcanoes cannot yet be measured directly. If SO2 is also emitted from the volcano, the SO2 flux can be measured using spectroscopy (e.g., UV camera, Differential Optical Absorption Spectroscopy), then combined with the molar C/S in volcanic gases to yield CO2 flux (Aiuppa et al., 2006, 2017, 2019; Carn et al., 2017). If there is no SO2 (e.g., in low temperature, geothermal areas, or areas of diffuse CO2 emissions), then spatially integrated methods of CO2 flux estimation must be used (Chiodini et al., 1998; Werner et al., 2019), or methods that consider the petrology of erupted magmas (Aiuppa et al., 2019). Fluxes must be based, in many cases, on short timescale periods of measurement, over just a segment of a volcanic region (rift or arc). Each carbon flux measurement captures only a snapshot of the outgassing behavior of each volcano, and may not be representative of the flux averaged over longer timescales.
Magmatic CO2 can be constrained through study of melt inclusions in mineral phases (e.g., Wallace, 2005; Blundy et al., 2010), however, the concentration of carbon trapped in magmas is dependent on several factors, such as the pressure at which CO2 begins to degas, and also the degree to which the magma has undergone crystallization. Melt inclusions can therefore provide only a minimum bound to arc carbon fluxes (Wallace, 2005; Wallace et al., 2015). Estimates from melt inclusions are further complicated by the spatial heterogeneity of carbon present in the Earth’s upper mantle which is sampled during magmatism, resulting in variable concentrations of CO2 in mantle-derived magmas (Helo et al., 2011; Le Voyer et al., 2017; Hauri et al., 2019). Carbon fluxes and concentrations averaged both over time and large spatial regions (e.g., over entire arc systems) therefore provide the most robust representation of that region’s relative contribution to total carbon outgassing as a whole.
Finally, both active and diffuse degassing of volcanic regions must be considered. A significant proportion of carbon emissions is expected to be released away from active volcanic vents and plumes at both actively erupting and dormant settings by diffuse porous flow (e.g., Armstrong McKay et al., 2014; Werner et al., 2019). Furthermore, the proportion of CO2 that is absorbed into groundwater in volcanic regions is largely unknown, yet contributes significantly to surface carbon reservoirs (Tamburello et al., 2018).
Alongside CO2, carbon is degassed as other species. The role of methane (CH4) in the global carbon cycle has been highlighted by Etiope et al. (2008). Methane emitted from volcanic settings is minor compared to CO2 (typically more than two orders of magnitude lower (e.g., Fischer, 2008), so we consider here only the CO2 emissions from volcanic settings. Other carbon-bearing species (e.g., CO, OCS, CS2) contribute negligible amounts of carbon to the atmosphere and may be ignored (Burton et al., 2013).
The total CO2 flux from volcanoes and volcanic regions is estimated to lie in the range 250–375 Mt CO2 yr–1 (70 to 100 Mt C yr–1, Werner et al., 2019), which is slightly lower than that estimated by Burton et al. (2013) (540 Mt CO2 yr–1; 150 Mt C yr–1). We consider below the individual contributions from magmatism at continental rifts, mid-ocean ridges, plume volcanoes, and subduction zones.
Continental Rifts
The importance of continental rifting to the deep carbon cycle, especially rifting occurring in East Africa, has been highlighted by several authors (Lee et al., 2016; Brune et al., 2017; Foley and Fischer, 2017; Hunt et al., 2017). Magmatism during rifting liberates carbon and other volatiles stored in sub-continental lithospheric mantle (e.g., Rooney et al., 2017) and continental crust (e.g., Parks et al., 2013), allowing it to be transported to the surface. Extensional faults may then become localized within rifting zones as deformation continues (Muirhead et al., 2016); these faults can facilitate and focus the transport of magma and exsolved CO2 to the surface from the mantle (e.g., Faulkner et al., 2010; Tamburello et al., 2018).
Sources of outgassing carbon may be differentiated through carbon and helium isotope ratios, as each source component has a distinct isotopic composition. Carbon isotope studies by Lee et al. (2016) on the Magadi-Natron Basin of the Eastern branch of the East African Rift suggest that the mantle is the dominant source of carbon during the early stages of rifting; this is supported by similar results from the Western branch (Botz and Stoffers, 1993) and from the Central European Eger Rift (Weinlich et al., 1999; Kämpf et al., 2013; Bräuer et al., 2018). The destabilization and degassing of mantle-derived carbon is hence an important contributor during continental rifting.
Continental rifts show a large degree of spatial variability as they transition from fault-bounded basins to oceanic spreading ridges (e.g., Ebinger, 2005). The diffuse carbon flux from a continental rift may match this variability along its length; previous estimates of carbon fluxes from the East African Rift, for example, based on extrapolation, vary from 5.0 ± 3.9 to 19.4 ± 9.0 Mt C yr–1 depending on the rift sector sampled (Lee et al., 2016; Hunt et al., 2017). Individual sectors of a continental rift may show significant intrinsic heterogeneity in their carbon fluxes both along and across rift strike (Hunt et al., 2017). Finally, the many influences on continental rift development result in a considerable range of continental rift basin areal fluxes of 3–120 t C km–2 yr–1 worldwide (Brune et al., 2017 and references therein). This variability might be related to several processes and parameters: the amount of decompression melting as a function of extension rate (Bown and White, 1995) or the existence of a mantle plume beneath the rift (White and McKenzie, 1995), the age of the lithosphere and maturity of the rift (Foley and Fischer, 2017), spatio-temporal changes of the stress field (Tamburello et al., 2018), or local episodes of magmatic and seismic activity (Bräuer et al., 2018). Identifying the relative impact of these controls at specific sites, which is expected to lead to a reduction of the uncertainty on the CO2 flux, is a topic of ongoing research, which goes beyond the scope of this paper.
Brune et al. (2017) give rift mean areal fluxes and rift flux standard deviations of 49 ± 53 t C km–2 yr–1, or 63 ± 44 t C km–2 yr–1 if both mean and standard deviation are weighted by rift basin area. If the recent Main Ethiopian Rift estimate of Hunt et al. (2017) is included (an areal flux of 122 t CO2 km–2 yr–1 over 20,000 km2), the mean and area-weighted mean are 46 ± 46 and 52 ± 38 t C km–2 yr–1, respectively. We choose an areal flux of 50 ± 40 t C km–2 yr–1 for rift basins to capture the full uncertainty in present-day continental rift carbon flux and rifting styles (Figure 4). Continental rift length histories are based on the plate tectonic model of Müller et al. (2016), complemented by a compilation of rifts preserved in the geological record (Şengör and Natal’in, 2001). We retain the assumed continental rift basin width of 50 km used by Brune et al. (2017) as such a width is typical of a developing rift zone on strong continental lithosphere (e.g., Copley and Woodcock, 2016).
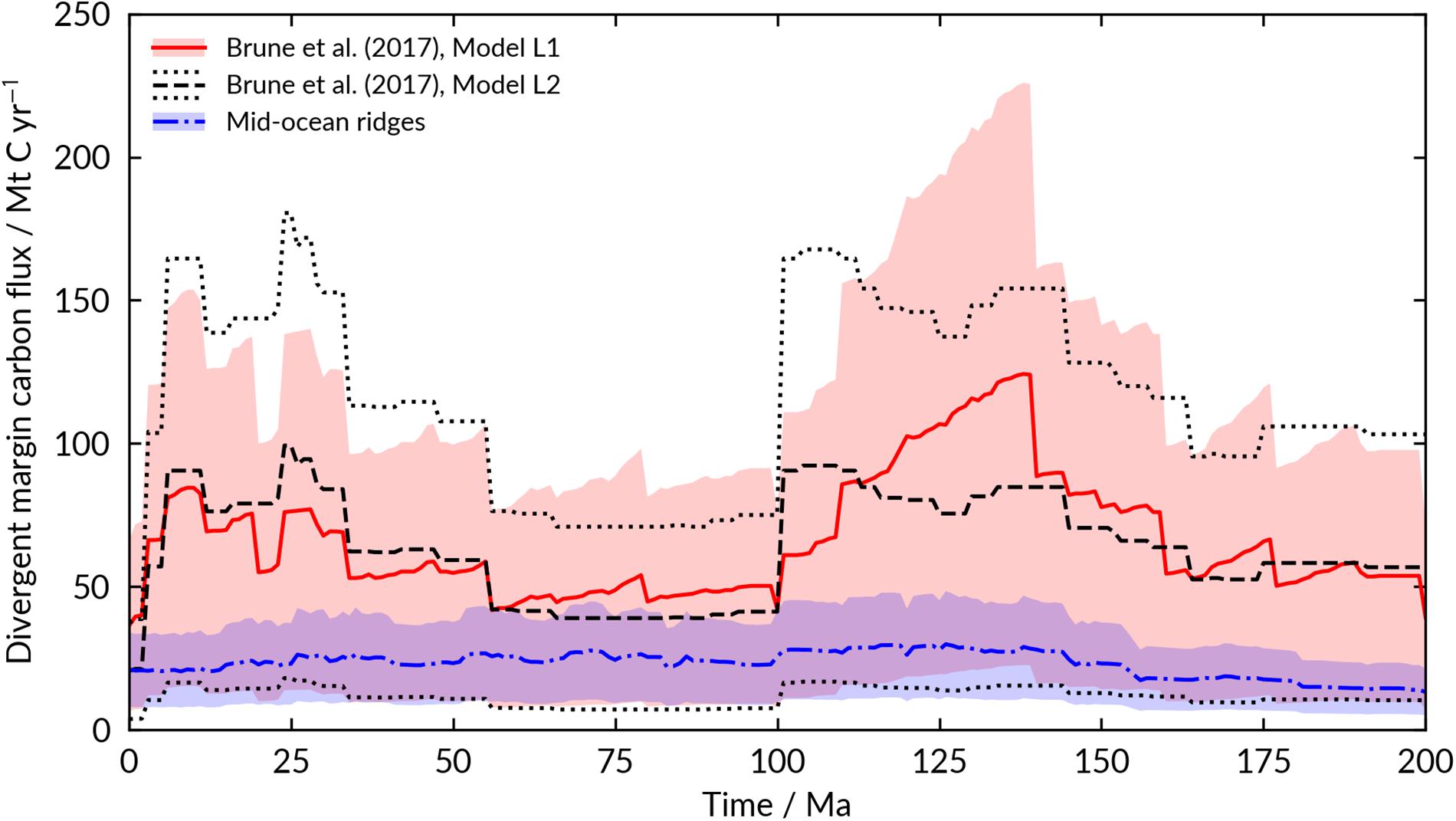
Figure 4. The carbon flux and uncertainty from divergent plate boundaries since 200 Ma. For continental rifts, the two models from Brune et al. (2017) are used, where L1 uses continent-scale rifts in concordance with rifts from the geological record, while L2 offers an alternate model using geologically inferred rifts alone. Mid-ocean ridge carbon outgassing flux is determined using a present-day estimate of 21 ± 13 Mt C yr–1, which encompasses most recent estimates and their uncertainties.
We do not account for the individual active fluxes of rift volcanoes such as Nyiragongo and Nyamuragira, two rift volcanoes that are known to be major carbon degassers (e.g., Aiuppa et al., 2019). Their combined flux of 1.6 ± 1.1 Mt C yr–1 (Aiuppa et al., 2019) is minor compared to the overall diffuse flux, and is accounted for by the uncertainty in the diffuse estimate.
Mid-Ocean Ridges
The flux of CO2 from mid-ocean ridges is almost entirely delivered into ocean water (Le Voyer et al., 2018). A fraction of this CO2 is precipitated into seafloor lithologies in the form of carbonate in crustal veins and serpentinites, or fixed into organic matter during photosynthesis (e.g., Alt and Teagle, 1999; Le Voyer et al., 2018); in addition, oceanic carbon reaches equilibrium with atmospheric carbon over short timescales (e.g., Zeebe, 2012). We must therefore consider mid-ocean ridge fluxes through time, as ridges are a significant contributor to Earth’s exogenic reservoirs.
There is a substantial range in previously published estimates of carbon fluxes from mid-ocean ridges (Figure 5). This is due to the challenge of estimating carbon fluxes in mid-ocean ridge settings with much less accessibility relative to other volcanic settings, and the subsequent difficulty in measurement of ridge carbon directly. Variability in spreading rate, mantle carbon concentrations and magma flux also produce notable differences in carbon flux along different mid-ocean ridge segments; CO2 fluxes are known to vary by at least two orders of magnitude as a result of this (Le Voyer et al., 2017, 2018; Hauri et al., 2019). In addition, there is uncertainty as to whether mantle volatiles liberated during decompression melting beneath mid-ocean ridges are focused toward the ridge axis or distributed along the base of the lithosphere (e.g., Keller et al., 2017). The method we apply must therefore account for uncertainty in past estimates and spatial heterogeneities in present-day ridge carbon fluxes.
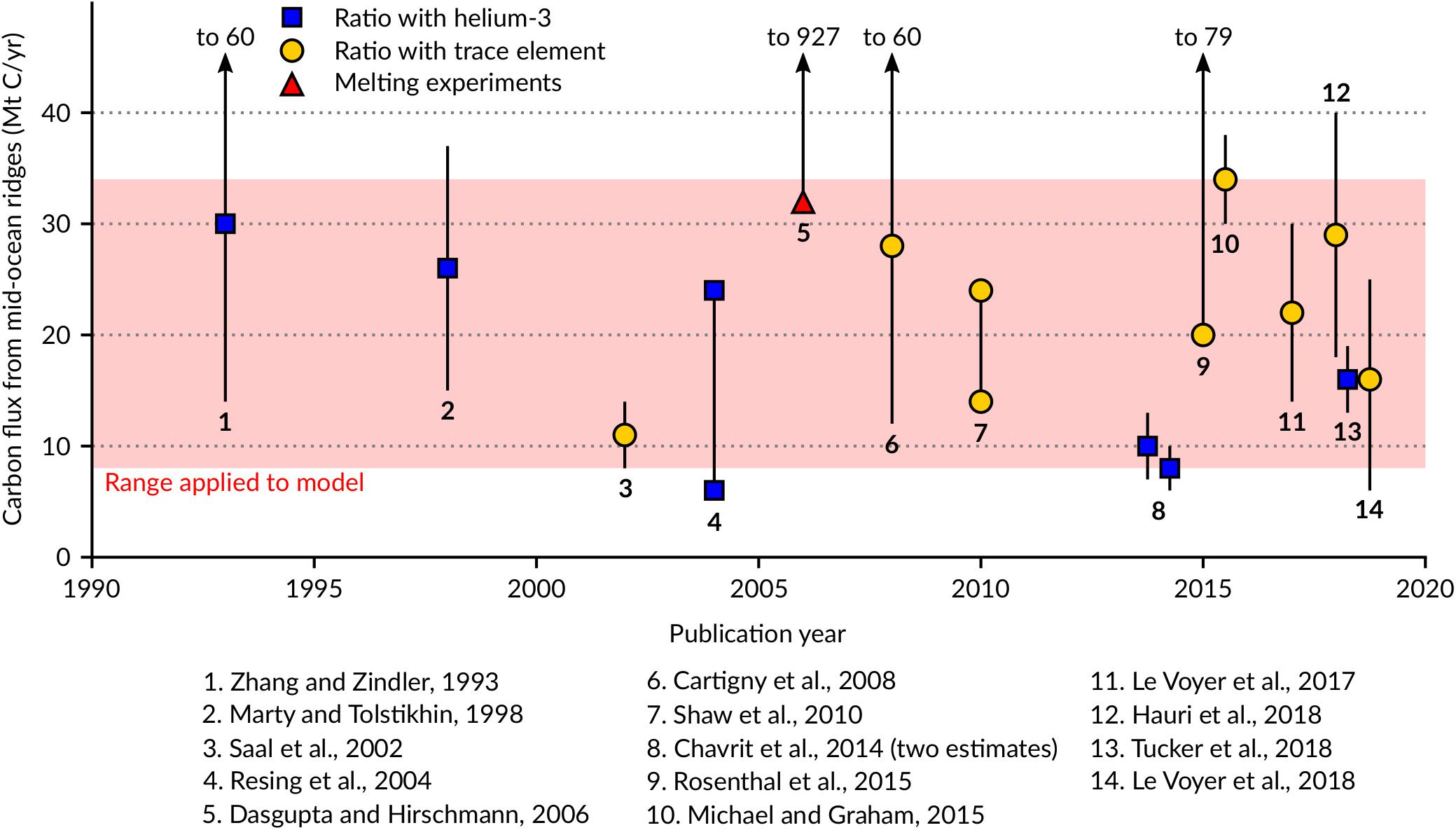
Figure 5. Comparison of previous ridge carbon flux estimates, plotted against year of publication (symbols and lines), and the range employed in this paper (red area). The symbols denote the method used to derive the estimate. Where two symbols are given a range of estimates is reported; a line to one or both sides of a point marks the uncertainty in the estimate. The flux estimates of Javoy and Pineau (1991) and Aubaud et al. (2004) are not shown as they extend beyond the range of the figure (180 Mt C yr–1 and 160+60/–30 Mt C yr–1 respectively), as do the uncertainties of Zhang and Zindler (1993) and Cartigny et al. (2008), and the maximum bounds of Dasgupta and Hirschmann (2006) and Rosenthal et al. (2015). Chavrit et al. (2014) provide a minimum and maximum estimate and error based on the geochemical data availability of the mid-ocean ridge samples analyzed. The other literature estimates compiled are Marty and Tolstikhin (1998), Saal et al. (2002), Resing et al. (2004), Shaw et al. (2010), Michael and Graham (2015), Hauri et al. (2017), Le Voyer et al. (2017, 2018), Tucker et al. (2018).
The low solubility of carbon in mid-ocean ridge basalts (e.g., Dixon et al., 1995) means that few samples collected at the surface are undegassed. Carbon flux estimates for MOR are therefore obtained through the measurement of ratios of CO2 in basaltic glass relative to lithophile trace elements that partition in a similar manner during melting and crystallization, such as Ba, Nb, and Rb, as this allows for the correction of degassed samples assuming a constant CO2 to incompatible element ratio (e.g., Saal et al., 2002; Rosenthal et al., 2015). However, Matthews et al. (2017) suggest that CO2/Ba and CO2/Nb ratios may also be influenced by the pressure at which degassing occurs, followed by mixing. Other approaches linking CO2 to 3He in mid-ocean ridge basalts must additionally correct for degassing of He from mantle-derived melts (e.g., Marty and Tolstikhin, 1998).
As it is difficult to account for uncertainty in these methods and results, we consider a simple approach in extrapolating mid-ocean ridge flux through time. We take the modern mid-ocean ridge flux and divide it by modern mid-ocean ridge length to obtain a mean carbon flux per unit length of ridge. This constant is then multiplied by ridge length as computed according to our reference plate tectonic model. This approach allows for the extrapolation of present-day carbon fluxes through geological time, but neglects both magma production fluxes and primary mantle carbon concentrations (Le Voyer et al., 2017, 2018; Hauri et al., 2019). We select a present-day total mid-ocean ridge carbon flux of 21 ± 13 Mt C yr–1, which overlaps with most previous estimates and their uncertainties (Figure 5). In addition, this estimate accounts for the range suggested by the recent estimate of Le Voyer et al. (2018), who sum individual ridge segment fluxes across the entire global ridge system based on MORB glass data. This extrapolation is shown in Figure 4.
Plume-Related Volcanism
Volcanism related to active mantle upwelling is a significant contributor of carbon to exogenic Earth. As an example, recent measurements of carbon fluxes from Katla volcano on Iceland suggest that basaltic plume-related volcanoes may constantly contribute a significant quantity of carbon to the atmosphere (∼5 kt C day–1, or ∼2 Mt C yr–1, Ilyinskaya et al., 2018). However, it remains uncertain whether such high emissions are representative of steady state diffuse degassing or pre-eruptive melt degassing; increased CO2 fluxes prior to eruption are known to correlate with an increase in magmatic flux, complicating our ability to estimate an “average” flux from plume volcanoes (e.g., CO2 fluxes reached >20 kt day–1 prior to the 2008 summit eruption at Kilauea Volcano, rising above a “background” flux of ≤300 t day–1 during the 1995 East Rift eruption; Gerlach et al., 2002; Poland et al., 2012).
The carbon released at plume settings is of uncertain origin, and is likely derived from multiple sources in the crust and mantle (Black and Gibson, 2019). While radiogenic isotope ratios (e.g., 3He/4He) suggest that mantle plume material may originate from a deep, primordial carbon reservoir (e.g., Marty et al., 2013), convecting mantle materials such as subducted slabs are likely to be entrained during plume upwellings, additionally contributing to the chemical composition of the plume and its carbon contribution (e.g., Sobolev et al., 2011). Plume-related magmas often indicate a geochemical contribution from lithospheric mantle (e.g., Gibson et al., 2006; Rooney, 2017), a known sink and source for mantle carbon (e.g., Foley and Fischer, 2017). Finally, crustal carbon-bearing rocks may be decarbonated during the emplacement of intrusive or extrusive plume magmas, which can cause extensive release of CO2 and CH4 (e.g., Ganino and Arndt, 2009; Svensen and Jamtveit, 2010).
The carbon liberated by a plume is likely to vary over its lifetime as a result of the temporal and spatial evolution it undergoes (e.g., Black and Gibson, 2019). In addition, neither the intrinsic properties of a mantle plume (e.g., potential temperature, buoyancy flux) nor the tectonic controls of melt generation (e.g., lithospheric thickness) appear to correlate with magmatic flux and degree of melting (Sleep, 1990), thereby affecting plume carbon fluxes, which, at the present-day, may differ between active plumes by several orders of magnitude (Werner et al., 2019). One possible method of generalizing plume fluxes is to establish a “baseline plume flux” at the present-day, and assume that this baseline has remained somewhat constant over the 200 Myr time period considered in this study.
Marty and Tolstikhin (1998) theorized that plume-related volcanism may contribute similar amounts of CO2 to the atmosphere as mid-ocean ridges; subsequent estimates have suggested that the flux from plume volcanoes may be much smaller than mid-ocean ridge fluxes (e.g., Dasgupta and Hirschmann, 2010; Werner et al., 2019). A compilation of some plume-related volcanic carbon fluxes by Barry et al. (2014) suggests that 3–8 Mt C yr–1 may be degassed by plume-related volcanism. Hauri et al. (2019) suggest a Hawaiian flux of 0.9 Mt C yr–1 from melt inclusions, comparable to the flux from the Kilauea plume of 0.8 Mt C yr–1 (Werner et al., 2019). By reviewing melt inclusions from other ocean islands, Hauri et al. (2019) suggest an estimate of 2–4 Mt C yr–1 from ocean intraplate volcanism, which is smaller, but comparable, to mid-ocean ridge flux. The volcanic flux review of Werner et al. (2019) provides an estimate of 2–7 Mt C yr–1 for intraplate volcanism including Yellowstone; including the Ilyinskaya et al. (2018) Katla estimate, this becomes 3–9 Mt C yr–1. Considering these estimates we suggest a present-day plume flux of 6 ± 3 Mt C yr–1, which is dominated by the fluxes of Yellowstone and Katla, but does not account for all plume volcanoes. If we assume that the passive carbon flux from mantle plumes remains roughly constant through geological time (implying either that the number of mantle plumes has remained roughly constant over the past 200 Ma, or that the variable conditions affecting each plume average out to present-day values), we can use this present-day estimate as a baseline mantle plume carbon flux (Figure 6).
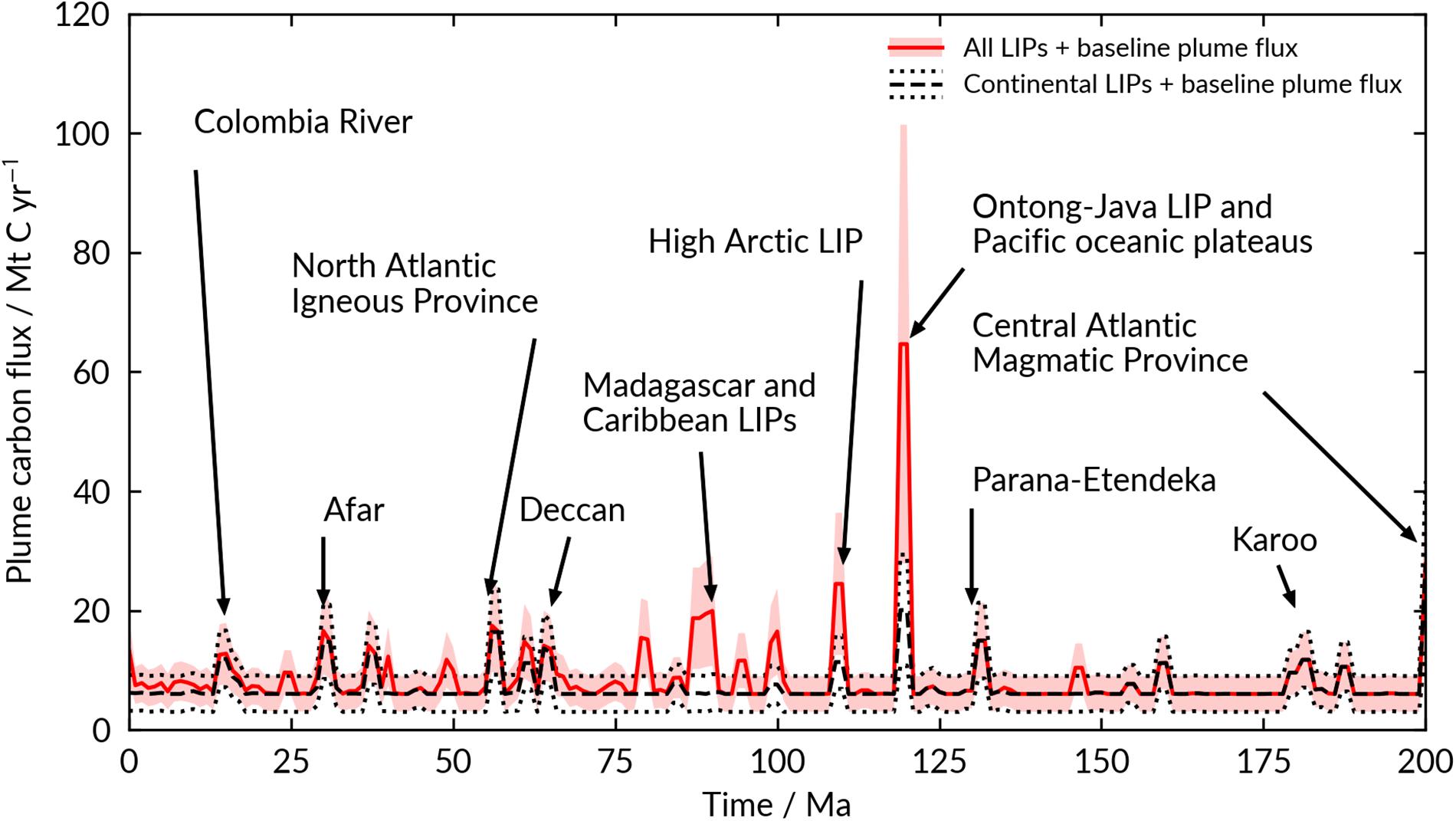
Figure 6. Volcanic carbon flux from continental and oceanic plume volcanoes (black dashed line and dotted line uncertainty) and all plume volcanism (red and shaded red area) since 200 Ma. A baseline flux of 6 ± 3 Mt yr–1 is assumed from recent volcanic flux reviews, and including the recent flux measurement from Katla (Ilyinskaya et al., 2018). Major flood basalt eruptions are annotated. The plume eruptive volcanic volumes are derived from Johansson et al. (2018).
Plume-derived carbon fluxes are further complicated by temporal variability in volcanism, especially at their arrival at the surface. Large Igneous Provinces (LIPs) and flood-basalt volcanism are often associated with the impact of a plume head with the base of the lithosphere (e.g., Richards et al., 1989; White and McKenzie, 1989). Emplacement of LIPs into and onto continental and oceanic crust are often associated with large changes in atmospheric CO2 and SO2 resulting in mass extinction events (e.g., Self et al., 2005, 2006; Ernst, 2014; Percival et al., 2015, 2017, 2018; Black and Manga, 2017; Johansson et al., 2018); these changes, much like the duration of volcanism, are short-term (1–5 Ma or less, e.g., Bryan and Ferrari, 2013) and associated spikes in atmospheric CO2 concentration are buffered by rapid weathering feedbacks acting on time scales of ∼1 Myr (Dessert et al., 2001). Nevertheless, during periods of flood basalt emplacement, these volcanic eruptions provide substantial CO2 contributions to the atmosphere.
Assimilation of carbon-rich material is additionally possible by LIP-related intrusions. Volatilization of organic carbon present in shales intruded by large igneous provinces could contribute significant proportions of CO2 and CH4 to the atmosphere over very short timescales, resulting in rapid climate change (Svensen et al., 2009; Svensen and Jamtveit, 2010; Jones et al., 2016 and references therein). While we note the importance of this assimilation to overall plume contributions, we do not consider them further in this study, as the timescales of these processes are likely too short for our modeling (∼100,000 year timescales, Svensen and Jamtveit, 2010).
Flood basalts likely degas completely as erupted products retain minimal carbon; by estimating the mass of erupted products we can estimate a carbon flux from estimated primary carbon concentrations (e.g., Self et al., 2006; Jones et al., 2016). However, the concentration of CO2 in LIP magmas is known to range from 0.1 to 2 wt% (Black and Gibson, 2019.), limiting the resolution of our approach. We adapt the LIP erupted area per 1 Myr of Johansson et al. (2018) to determine an average annual area flux, which is converted into a corresponding LIP eruption volume flux using an average flood basalt thickness of 2 ± 1 km (which fits the average thickness of most flood basalts, Courtillot and Renne, 2003; Ross et al., 2005; Ernst, 2014). To predict the carbon which is degassed, we select an average intraplate primary CO2 concentration of 0.8 ± 0.3 wt% (Hauri et al., 2019; Figure 6); this CO2 concentration lies within the range suggested by Black and Gibson (2019). For our analysis we assume that oceanic and continental LIP basalts degas the entirety of their CO2 on eruption.
Arc Volcanism
Carbon degassed at continental arc volcanoes is sourced from the mantle via fluids liberated from subducted slabs, from the mantle wedge, and from assimilation in the shallow crust (Marty et al., 2013; Aiuppa et al., 2017; Mason et al., 2017). There is an abundance of evidence to show that carbon from subducted material (sediments and crust) influences the carbon output of volcanoes in subduction zones (Sano and Marty, 1995; Sano and Williams, 1996; Hilton et al., 2002; Shaw et al., 2003; Fischer, 2008; Aiuppa et al., 2017). This is exemplified by the Central American Volcanic Arc, which displays along-arc trends in volatile emissions linked to the composition of slab material and fluids (Aiuppa et al., 2014; de Moor et al., 2017).
High latitude volcanoes, such as those of the Aleutian Arc, outgas low fluxes of carbon (Fischer, 2008; Aiuppa et al., 2017; Lopez et al., 2018); these volcanoes are associated with the subduction of seafloor sediments dominated by carbon-poor diatomaceous and terrigenous material (Johnston et al., 2011). The sedimentary composition is reflected in the carbon isotopic composition, which lies within mantle values (Kodosky et al., 1991; Symonds et al., 2003; Fischer and Lopez, 2016). Latitude, a key control on sediment compositions, may therefore modulate the magnitude of carbon outgassing from arc volcanoes.
As well as carbon sourced from the subducting slab, it has become increasingly clear that some carbon is sourced from the overlying crust. The evolution of biomineralizing life led to growth and development of carbonate platforms built on continental margins, and the potential of these platforms to interact with percolating arc magmas at subduction zones (Pall et al., 2018; Lee et al., 2019). Models of magma intrusion into lower crustal hot zones predict substantial amounts of crustal melting where basaltic sills intrude amphibolite and other crustal rocks (Annen, 2011; Solano et al., 2012). This interaction provides the basis for assimilation of host rock chemistry, and is consistent with widespread geochemical signatures of assimilation (e.g., Hildreth and Moorbath, 1988; Goff et al., 2001; Chadwick et al., 2007; Iacono-Marziano et al., 2009). The decarbonation of crustal carbonates interacting with silicate magmas generates increased volumes of CO2 relative to non-carbonate-intersecting arcs; this enhanced outgassing is observed in experiments (Iacono-Marziano et al., 2008, 2009; Deegan et al., 2010; Carter and Dasgupta, 2015, 2016, 2018), and is evident from isotopic signatures of shallow crustal assimilation of carbonate measured in arc volcanic gas emissions (Mason et al., 2017). The co-evolution of continental arc lengths and carbonate platforms must therefore be considered when considering arc carbon fluxes. Increased volcanic carbon outgassing fluxes are expected when continental arc lengths are longer than at the present-day, as the potential of crustal carbonate intersection is greater (Lee et al., 2013, 2015; Lee and Lackey, 2015; Pall et al., 2018).
We use subduction zone length as a proxy for arc length using as in Pall et al. (2018); this method permits us to capture periods of arc history that may not be considered through other methods (e.g., mantle tomography, van der Meer et al., 2014; detrital zircon age statistics, McKenzie et al., 2014, 2016; exposure of arc-related granitoid bodies, Cao et al., 2017a). The data for carbonate platform development through time from Pall et al. (2018) is also used for modeling the degree of carbonate intersection. In the model of Pall et al. (2018), multiple crustal decarbonation events are not considered. It is likely that a second magmatic intrusion in the same position as a prior intrusion will not liberate as much CO2 as a first event, however, multiple factors (e.g., temperature, pressure, melt composition, carbonate platform thickness) affect the efficiency of decarbonation and hinder the simplicity of modeling multiple decarbonation events (Johnston et al., 2011).
It should be noted that the influence of carbonate, both in the shallow crust and from the subducted slab, is not the only significant control on volcanic carbon degassing. The specific dynamics of degassing play a critical role in the fluxes of all magmatic gases released at volcanoes. The highest carbon flux (Nyiragongo, Le Guern, 1987) recorded thus far was measured at an intra-plate (associated with a continental rift) open vent basaltic system with no evidence of shallow carbonate assimilation. Furthermore, the effect of localized mantle upwelling (as hypothesized under Italian volcanoes, e.g., Schiano et al., 2001), should be considered, as this will affect our extrapolation of arc fluxes through time when based solely on carbonate intersection. Understanding and integrating the complexity the above factors introduce through time is, however, beyond the scope of this work.
Kelemen and Manning (2015) estimate arc volcanic fluxes at the present-day as 18–43 Mt C yr–1. Recent reviews of active and diffuse carbon fluxes places currently known arc emissions at 8–12 Mt C yr–1 (Aiuppa et al., 2019; Werner et al., 2019); this value does not account for total volcanic arc degassing, instead providing a minimum bound from arc volcano carbon fluxes which have been measured or monitored. Recent methods considering concentrations of CO2 within magmatic material added to continental arcs have produced similar values at the present-day of 7.8 Mt C yr–1 (Ratschbacher et al., 2019), but do not account for differences in the carbon content of the subducted slab and overlying crust. We therefore use a present-day arc output of 27 ± 16 Mt C yr–1, taking into account both recent estimates and the range suggested by Kelemen and Manning (2015). Werner et al. (2019) also note that the higher estimates may be skewed toward eruptive periods when active degassing is enhanced; we use this value as a suitable upper constraint for arc degassing.
In our study we distinguish between arcs that intersect crustal carbonates and those that do not. Figure 7 shows the result of applying the average endmember values of 108.0 mol km–1 yr–1 degassed from carbonate-intersecting arcs and 106.3 mol km–1 yr–1 degassed from non-carbonate intersecting arcs to the model of Pall et al. (2018; see Supplementary Material for more details). Our results show that if arcs are treated as undifferentiated over time, then the carbon fluxes from continental arcs have remained relatively constant through the past 200 Ma, varying from 93 to 123% of the present-day value at most. If, however, we differentiate arcs into those that intersect carbonate and those that do not, we predict that carbon arc fluxes have increased over time as a result of increasing carbonate-intersection (from 43% of present-day values at the start of the Jurassic).
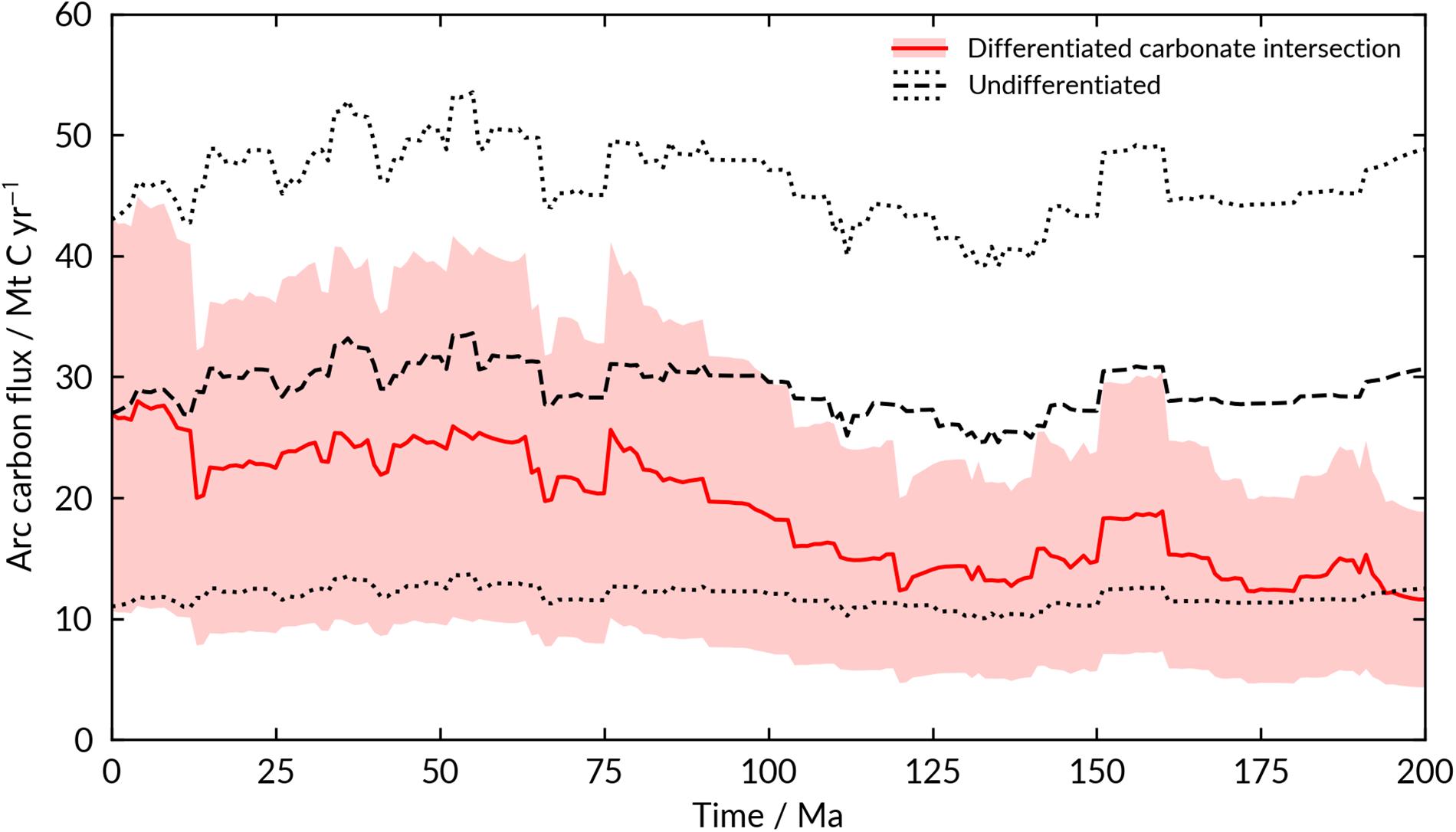
Figure 7. Minimum, maximum, and mean arc carbon flux for volcanic arcs since 200 Ma. The red line and area illustrate the case where carbonate-intersecting subduction zone arcs and those that do not intersect carbonates are differentiated, producing 108.0 mol km–1 yr–1 and 106.3 mol km–1 yr–1 of carbon, respectively. The case where all arc lengths produce the same carbon flux (arcs are not differentiated into carbonate-intersecting and non-carbonate-intersecting) is included as the black dashed line and dotted uncertainty.
Our approach does not consider the influence of significant carbon-degassing volcanoes such as Etna and Popocatepétl, which together contribute at least 2 Mt C yr–1 to present-day global arc flux, or 5–15% (Werner et al., 2019). Both volcanoes are located on arcs intersecting crustal carbonates (Etna on the Calabrian Arc; Popocatepétl on the Trans-Mexican Volcanic Belt). de Moor et al. (2017) propose that the dominance of such volcanoes may indicate either immense short-scale compositional or geophysical variability in carbon subduction along arc segments or short-term variability in arc carbon fluxes; in addition we suggest that the lateral distribution of crustal carbonate may also be a factor in determining local carbon degassing rates.
Discussion
Net Carbon Flux
In this analysis we have considered the balance of carbon degassed at different tectonic settings and the amount of carbon that is subducted into the deep Earth (Figure 8). The result of the analysis presented here is that while net outgassing flux has stayed close to zero for the last 200 Myr, for the majority of this time net outgassing of CO2 has been slightly positive, at around 25–75 Mt C yr–1 (the equivalent of the CO2 degassing of 5–15 Mount Etnas, Burton et al., 2013). This result therefore suggests that the carbon released from Earth’s mantle is larger than the carbon returned to the mantle at subduction zones, and that there has been a net outgassing of carbon to the Earth’s surface since 200 Ma.
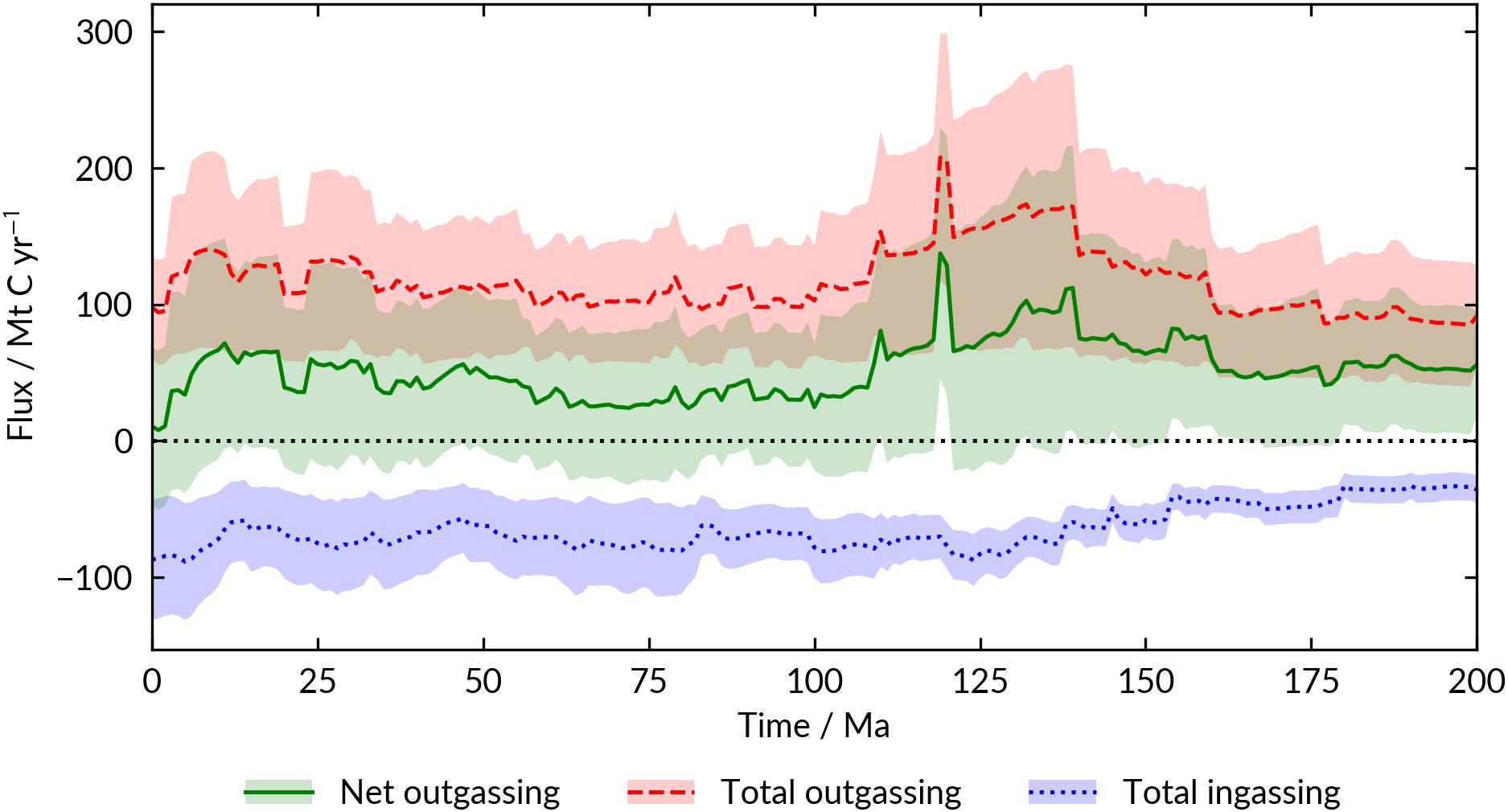
Figure 8. Total outgassing (red), total ingassing (blue), and net outgassing (green) plotted against time. Total ingassing curve drawn assuming a symmetric uncertainty represented by the difference between the maximum carbonate sediment subduction and the mean carbonate sediment subduction, as opposed to the modeled asymmetric distribution. Total outgassing curve drawn assuming the continent scale rift history of Brune et al., 2017 (L1), and the differentiation of volcanic arcs into carbonate-intersecting and non-intersecting (Pall et al., 2018).
We acknowledge that this first-order net degassing curve does not account for the subduction of organic carbon within sediments (as mentioned in section “Carbonate Sediments”), which may constitute a significant proportion of subducting carbon, however, we note that organic carbon subduction, if similar to the ∼20% of subducted sediment at the present-day (Clift, 2017), would not be sufficient to account for the difference between ingassing and outgassing fluxes. We additionally make note of the potential release of stored carbon within the crust and mantle during volcanism, such as at carbonate-intersecting arcs, which may bolster the net carbon outgassing curve.
Figure 9 shows the relative contribution of each tectonic setting to total degassing. Our results agree to a first order with those of Marty and Tolstikhin (1998), who suggest that the contributions from mid-ocean ridges, arcs, and plumes may contribute comparable fluxes of carbon; at the present-day and over the past 200 Ma the contributions from mid-ocean ridges, arcs, and plumes have been similar. More interestingly, our results highlight the vital role of rifting to the deep carbon cycle, consistent with previous studies (Brune et al., 2017; Foley and Fischer, 2017). Of the four plate tectonic settings considered in this study, continental rifts account for a significant portion of degassed carbon (Figure 9); the mean areal flux of 50 ± 40 t C km–2 yr–1 adopted suggests that continental rifts may consistently supply 50% of the total outgassing carbon from the deep Earth, with a maximum at ∼130 Ma linked to the rifting of the Pangaean supercontinent. However, such a large quantity may be an artifact of the uncertainties in capturing rift carbon fluxes; the minimum and maximum rift values would suggest consistent carbon contributions of 20 and 70%, respectively to Earth’s surface (Figure 9). This observation highlights the necessity for a better understanding of carbon degassing within continental rift zones, and demonstrates that continental rifts may play the most important role in delivering non-anthropogenic carbon to the atmosphere. Oceanic divergent boundaries contribute a smaller, but not insubstantial amount of ∼20%, which remains fairly constant over the past 200 Myr.
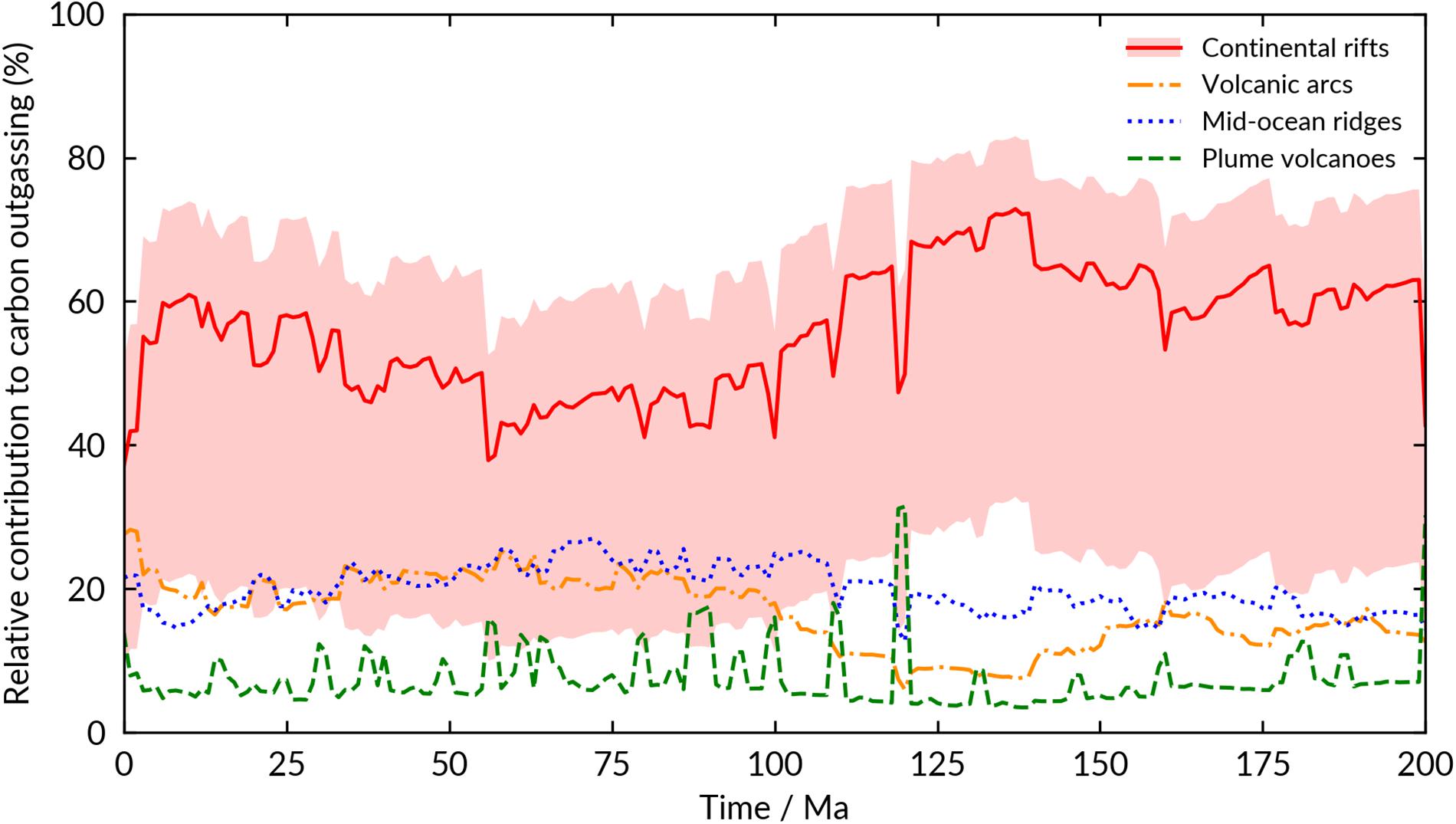
Figure 9. Ratioed outgassing contributions from different tectonic settings. Rift maximum and minimum degassing is shown as the shaded red area. The contributions from arcs, plumes, and ridges are mean calculated values; uncertainty envelopes for these contributions are not shown.
Volcanic arc contributions to total outgassing have fluctuated between 10 and 30% of total net outgassing through time, reaching a maximum at the present-day, coincident with a minimum in rift carbon (Figure 9). This could illustrate the effect of millions of years of carbonate accumulation in the crust through geological time, and hence increasing arc carbonate assimilation; this could also be a result of the limitations of both the Pall et al. (2018) model and our own assumptions, which both do not account for carbonate platform thicknesses or the effect of multiple decarbonation events.
Mantle plume volcanism contributes ∼10% of the total flux, with increased outgassing during LIP emplacement events (Figure 9). Our LIP carbon fluxes are in agreement with past estimates for carbon degassing for individual LIPs, including the Columbia River Basalt Group eruptions estimate of Armstrong McKay et al. (2014) (7.5–10 Mt C yr–1) and the Deccan Traps estimate of Caldeira and Rampino (1990) (6 to 20 × 1016 moles over several hundred thousand years, or ∼7–20 Mt C yr–1).
Figures 8, 9 therefore highlight the role of arc and rift magmatism in liberating vast quantities of carbon stored within the crust and mantle. Volatilized carbon-bearing phases at these settings have played a vital role in tipping the balance between carbon subduction and degassing over the last 200 Myr, and new insights into the remobilization of stored carbon in continental crust and metasomatized lithospheric mantle will likely continue to prove a fruitful avenue for future research into the deep carbon cycle.
Links Between Carbon Fluxes and Atmospheric CO2
Our total net outgassing curve is plotted against the proxy atmospheric CO2 record of Foster et al. (2017) in Figure 10. There appears to be minimal first-order correlation between the two curves, albeit for occasional spikes corresponding to LIP eruptions (e.g., at 100–120 Ma). Similarly, there is little correlation between the outgassing/ingassing ratio and atmospheric CO2 (Figure 11A). The noticeable lack of correlation between total net outgassing fluxes and atmospheric CO2 concentrations highlights the importance of carbon cycling and storage within surface reservoirs, thereby maintaining Earth’s climate.
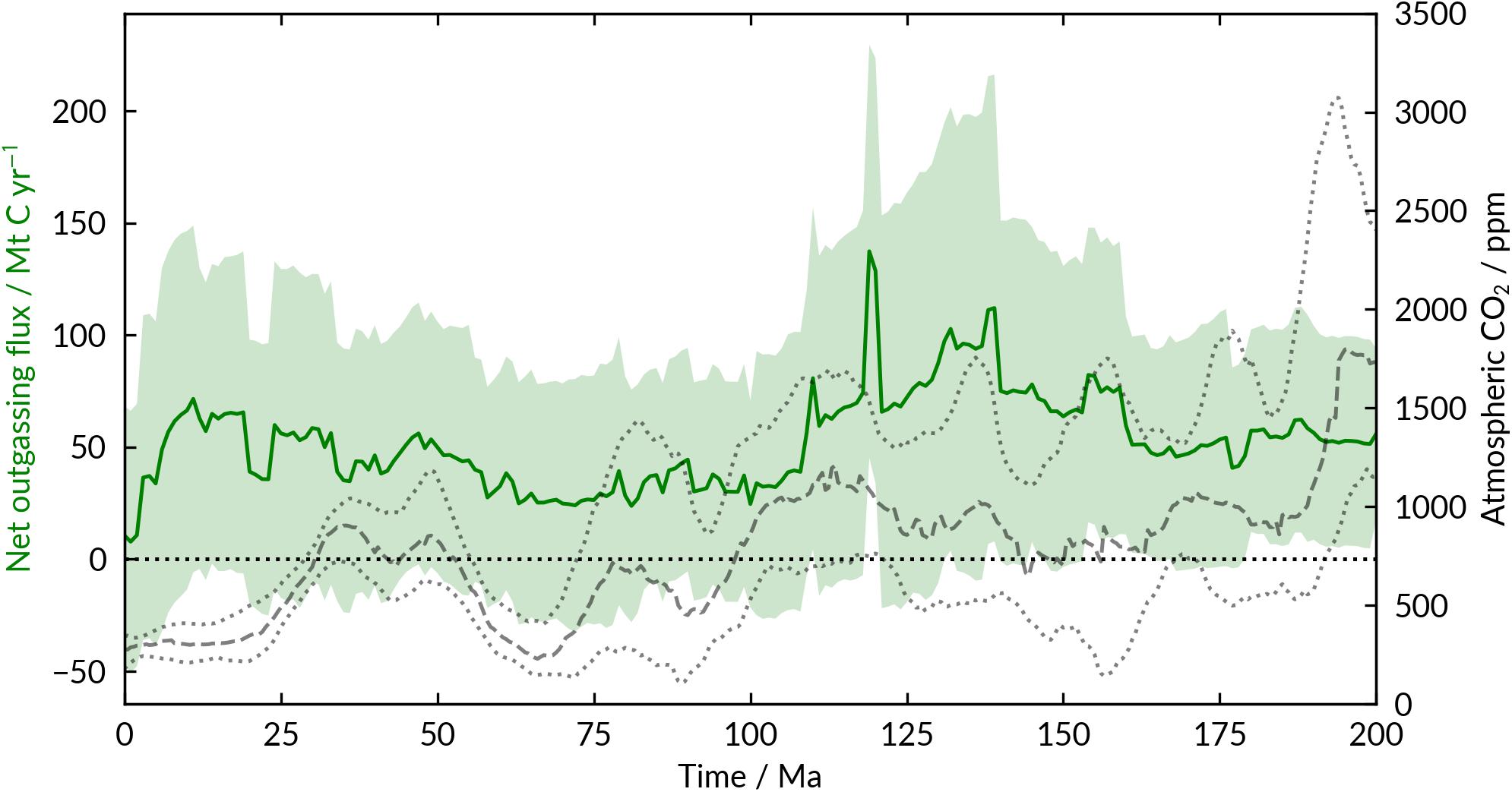
Figure 10. Net carbon flux plotted against time (green line and green shaded area uncertainty). Atmospheric CO2 curve of Foster et al. (2017) included (dashed gray line) with 2σ uncertainty (dotted gray lines).
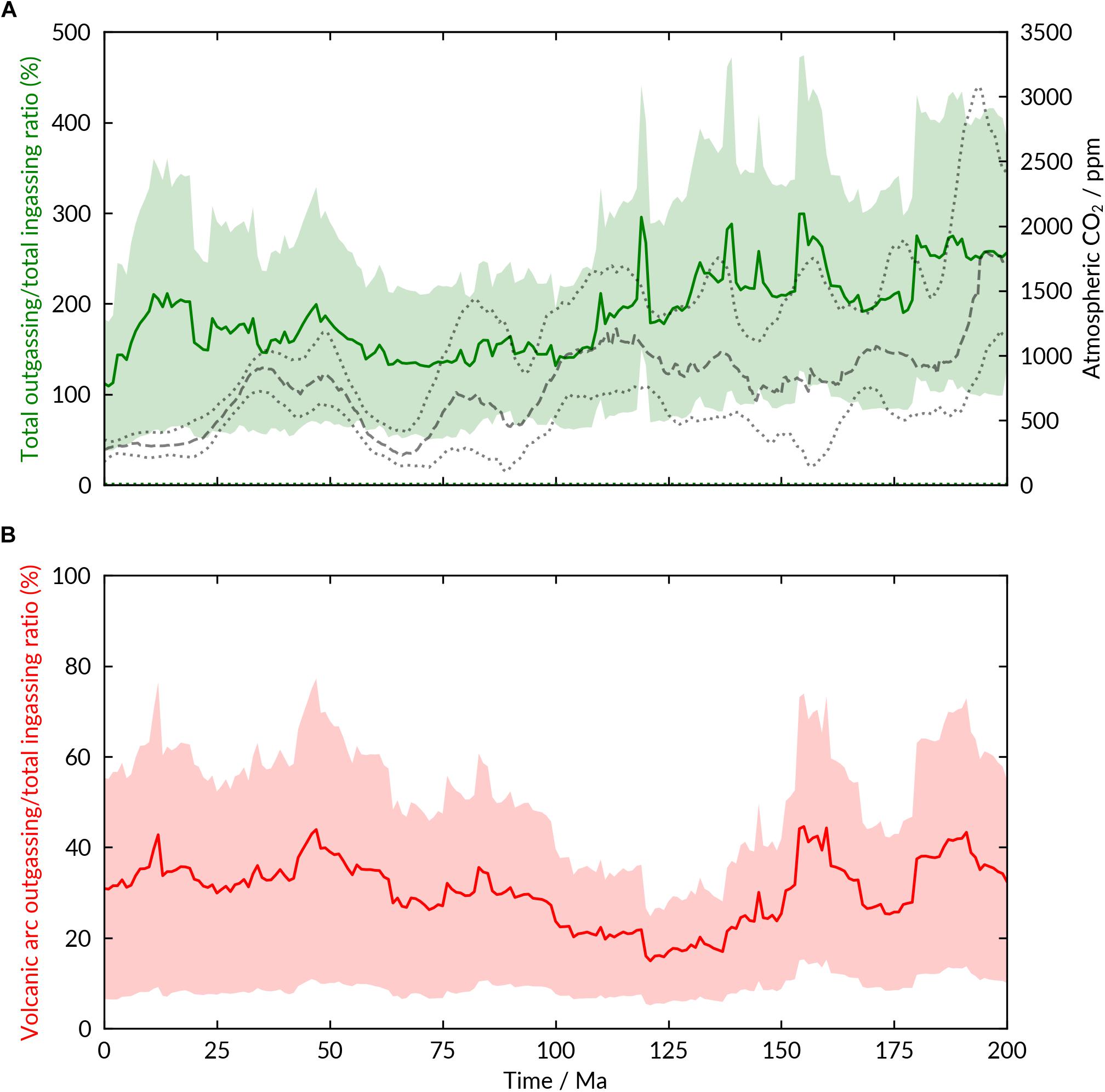
Figure 11. (A) Gross carbon outgassing/ingassing ratio as percentage ingassing plotted against time (green line and shaded area). The atmospheric CO2 curve and uncertainty of Foster et al. (2017) is included as the dashed and dotted gray lines. (B) Percentage of subducted material accounted for by outgassing at volcanic arcs, and uncertainty envelope.
One of the major surface processes we have not considered is carbon drawdown resulting from silicate weathering. Silicate weathering provides a negative feedback to elevated atmospheric CO2 concentrations (e.g., Dessert et al., 2003; Jones et al., 2016). Carbon can chemically sequester in silicate materials to form clay minerals, which are preferentially removed during erosion and transported to the oceans (Colbourn et al., 2015). The drawdown of atmospheric CO2 is dependent on available surface area for weathering, local erosion rates, and land surface reactivity (Rugenstein et al., 2019). Temperature, humidity, precipitation rates and acidity of the weathering environment all control erosion rates and are in turn controlled by climate; greater erosional rates and CO2 drawdown is expected at lower latitudes where precipitation rates and temperature are higher (e.g., Amiotte Suchet et al., 2003). CO2 drawdown by silicate weathering is further enhanced by the emplacement of large surface areas of mafic material onto the Earth’s surface, which weather 5–10 times faster than granitic or gneissic material (Dessert et al., 2001, 2003). LIPs and basaltic eruptions therefore provide an immense sink for atmospheric carbon, considered a net sink by some studies (e.g., Jones et al., 2011), with a drawdown flux of ∼1.8 ± 2.2 Mt C km–2 yr–1 (average basaltic watershed CO2 consumption rate, Dessert et al., 2003). The link between flood basalt area within low latitudes and atmospheric CO2 has been explored previously by Johansson et al. (2018), who link downward atmospheric CO2 trends to the transit and residence of major LIPs in the near-equatorial humid belt.
In addition, we have not considered the exsolution of CO2 during the metamorphism of carbon-bearing rocks, such as limestones or shales (Bickle, 1996; Evans, 2011). The thermal breakdown of carbonates can liberate CO2 contained within the carbonate structure, as can reaction with silica (e.g., Ague and Nicolescu, 2014). Vast amounts of CO2 are therefore expected to be released during regional metamorphism in orogenic belts, such as the Himalayas (Kerrick and Caldeira, 1998; Evans et al., 2008), where reactions from greenschist facies onward can permit subsolidus decarbonation. Direct measurement of metamorphic carbon dioxide is often possible in orogenic belts through the analysis of riverine water and groundwaters (e.g., Becker et al., 2008; Evans et al., 2008; Frondini et al., 2008), chromatographic modeling of fluid reaction fronts (Skelton, 2011), and metamorphic phase diagram modeling (Groppo et al., 2017). Svensen and Jamtveit (2010) argue that owing to the long timescales related to orogenic processes there are unlikely to be substantial climate changes linked to orogenic decarbonation; however, present-day estimates of orogenic degassing are on a similar order of magnitude to that of mid-ocean ridges and plumes. As an example, measured Himalayan metamorphic carbon fluxes range from 0.132 to 0.408 Mt C yr–1 (Groppo et al., 2017) to 11 Mt C yr–1 (Becker et al., 2008). On the other hand, the erosion of orogenic belts has been linked to immense atmospheric drawdown as a result of silicate weathering (e.g., Raymo and Ruddiman, 1992).
The question as to whether total metamorphic degassing is balanced by drawdown resulting from silicate weathering of mountain belts remains a subject of debate. Some authors suggest that decarbonation and silicate weathering are in balance as a result of increased sedimentation expected from erosion of orogenic belts, and hence increased organic carbon burial (Galy et al., 2007; Gaillardet and Galy, 2008). Metamorphic contributions to carbon fluxing could therefore be ignored if this was indeed the case. In contrast, Huh (2010) estimates that the drawdown flux of the Tibetan Plateau at the present-day is substantially lower than suggested degassing rates listed above (e.g., Skelton, 2011), implying that metamorphic degassing and drawdown are decoupled (Evans, 2011).
Metamorphic degassing is dependent on the pressure and temperature of reaction, the initial composition of the rock and its volatile content, the porosity of the rock and the ease of degassing, and the rate at which metamorphic rocks undergo reaction (e.g., France-Lanord and Derry, 1997; Evans et al., 2008; Groppo et al., 2017). We therefore expect significant variations in metamorphic CO2 degassing both across a single mountain range and between different ranges. In addition we anticipate large temporal variability in carbon exsolution over the lifetime of an orogenic belt. Without considering this full range in parameters it is difficult to accurately determine past metamorphic fluxes using our present-day analogs. One future avenue of research may be to determine orogenic belt areas from paleogeographical models (e.g., Cao et al., 2017b), and utilize them alongside the rock record in estimating protolith compositions and hence metamorphic decarbonation and drawdown fluxes, however, such modeling is beyond the scope of this paper.
What Goes Down Is Mostly What Comes Up – The Norm or the Exception?
Kelemen and Manning (2015) suggest that ingassing and degassing carbon fluxes at present-day subduction zones are approximately in balance, or perhaps that outgassing fluxes are larger, implying that there is very little return of carbon to the deep mantle, and that some carbon sourced from the subducting slab may be stored in the lithosphere of the overriding plate. Other authors have argued that carbon subduction and mantle outgassing are in steady state over long timescales (e.g., Jarrard, 2003).
Our analysis suggests that at the present-day, most of the carbon entering the mantle at subduction zones is unaccounted for; only about 20–40% of subducted carbon is outgassed at arc volcanoes, implying that the remaining 60–80% of subducted material is returned to the mantle or trapped in lithospheric reservoirs (Figure 11B). Within the mantle, this 60% is likely either recycled back into the deep mantle or stored in the mantle lithosphere. In either case, convection, plumes, and continental rifting may liberate carbon from the mantle and return it to the surface. Our results additionally indicate that there has not been a period in the past 200 Myrs during which subduction fluxes have been perfectly balanced by arc emissions. Arc emissions have, for this time period, constituted less than 50% of the total subducted carbon. This result not only illustrates the relatively low efficiency of arcs in outgassing their subducted carbon, but also the role of continental rifts and mid-ocean ridges in maintaining the Earth’s ingassing and outgassing balance.
The difference between our conclusions and those of Kelemen and Manning (2015) at the present-day is partly a result of new estimates of carbonate subduction. Recent estimates of carbonate subduction at the present-day of 48 Mt C yr–1 (Clift, 2017) and 57 Mt C yr–1 (Dutkiewicz et al., 2018) are significantly larger than the 13–23 Mt C yr–1 assumed for carbon-bearing sediments by Kelemen and Manning (2015). Likewise, present-day estimates for arc degassing are significantly lower than the values adopted by Kelemen and Manning (2015), Aiuppa et al. (2019) estimate a value of 8.0 ± 0.6 Mt C yr–1, and the value we use of 27 ± 16 Mt C yr–1 to capture the range of estimates reflects these recent developments.
Finally, recent investigations into carbon sequestration in the Costa Rica subduction zone suggests that a significant portion of carbon may be stored as subsurface crustal calcite in the forearc region, implying that relatively little subducted carbon is then consumed and degassed at subduction zones (Barry et al., 2019). An alternate conclusion from our results could therefore be that a significant proportion of slab-derived carbon is removed by forearc processing prior to outgassing at subduction zones, however, further understanding of such processes will be necessary to differentiate between the effects of forearc storage and deep mantle subduction.
Conclusion
The relationships between the temporal change in plate boundary lengths and deep Earth carbon outgassing and ingassing have been the subject of several recent studies (Brune et al., 2017; Müller and Dutkiewicz, 2018; Pall et al., 2018). This study summarizes our understanding of carbon degassing at tectonic settings at the present-day, and the implications of current present-day estimates to temporal atmospheric carbon variability and climate change since the start of the Jurassic. We have highlighted recent advances made, particularly in the fields of plate reconstruction modeling and measurements of carbon degassing, but also the significant uncertainties and debates that are ongoing in the carbon cycling community. With this in mind a number of broad conclusions may be drawn:
(1) Net outgassing has been positive over the last 200 Ma, averaging ∼50 Mt C yr–1 more than ingassing at subduction zones (Figure 8). This result highlights the importance of surface sinks in regulating atmospheric CO2, or the necessity of reactivation of sub-continental lithospheric storage, but may be accounted for when other carbon sequestering mechanisms are incorporated (e.g., silicate weathering, etc.).
(2) We build on previous work (e.g., Brune et al., 2017; Foley and Fischer, 2017) to show that rifts appear to be the dominant tectonic setting in terms of carbon degassing fluxes over the last 200 Ma (Figure 9), although significant uncertainty remains. However, the influence of carbonate-intersecting arcs increases with time (see Figure 7). The influence that this increase may or may not have had on atmospheric CO2 over the last 200 Ma remains unclear. An implication of this conclusion is that outgassing at volcanic arcs does not completely account for the carbon ingassed at subduction zones (Figure 11B), and that some material is recycled back into the mantle, where it later contributes to carbon outgassed at rifts, ridges, and plume volcanoes.
(3) The net outgassing values (Figure 10) do not correlate well with atmospheric CO2 concentrations over the last 200 Ma (Figures 10, 11A). This should not be taken as implying that the approach of this work is incorrect, but that there are “missing” components in the model of ingassing and outgassing presented here.
(4) Our first-order outgassing curve is limited by our present-day understanding of deep carbon cycling processes. Further understanding of the mechanisms behind carbon production and release will be necessary to constrain carbon estimates at the present day. We highlight the following critical components which will be essential in understanding the global carbon balance through deep time: (a) Integration of orogenic controls on the deep carbon cycle, including metamorphic degassing and silicate weathering; (b) Sub-lithospheric storage of carbon, which may be critical for understanding the distribution of carbon on Earth but is yet unaccounted for in most models (including the one presented here); (c) Constraining the uncertainty in carbon fluxes arising from continental rifts.
Data Availability Statement
The datasets generated for this study are available on request to the corresponding author.
Author Contributions
SB, MrE, and SZ conceived the study. KW and EM performed the literature review with support from SB, MrE, and SZ. KW assembled net outgassing curve. MdE and SZ contributed data from GPlates. All authors contributed to interpretation and writing of the manuscript.
Funding
This work arose from a workshop funded by the Alfred P. Sloan Foundation at the University of Cambridge in 2018, under the auspices of the Deep Carbon Observatory. SB was supported through the Helmholtz Young Investigators Group CRYSTALS (VH-NG-1132). SZ and MdE were supported by Alfred P. Sloan grants G-2017-9997 and G-2018-11296 through the Deep Carbon Observatory, and SZ was also supported by Australian Research Council grant IH130200012.
Conflict of Interest
The authors declare that the research was conducted in the absence of any commercial or financial relationships that could be construed as a potential conflict of interest.
Acknowledgments
We thank R. Dietmar Müller for constructive comments on a draft of the manuscript, and feedback from colleagues who attended the Janet Watson Meeting at the Geological Society of London in 2019 and participate in the University of Leeds’ Rocks, Melts, Fluids study group.
Supplementary Material
The Supplementary Material for this article can be found online at: https://www.frontiersin.org/articles/10.3389/feart.2019.00263/full#supplementary-material
References
Ague, J. J., and Nicolescu, S. (2014). Carbon dioxide released from subduction zones by fluid-mediated reactions. Nat. Geosci. 7, 355–360. doi: 10.1038/ngeo2143
Aiuppa, A., Federico, C., Giudice, G., Gurrieri, S., Liuzzo, M., Shinohara, H., et al. (2006). Rates of carbon dioxide plume degassing from mount etna volcano. J. Geophys. Res. Solid Earth 111:B09207. doi: 10.1029/2006JB004307
Aiuppa, A., Fischer, T. P., Plank, T., and Bani, P. (2019). CO2 flux emissions from the Earth’s most actively degassing volcanoes, 2005–2015. Sci. Rep. 9:5442. doi: 10.1038/s41598-019-41901-y
Aiuppa, A., Fischer, T. P., Plank, T., Robidoux, P., and Di Napoli, R. (2017). Along-arc, inter-arc and arc-to-arc variations in volcanic gas CO2/ST ratios reveal dual source of carbon in arc volcanism. Earth Sci. Rev. 168, 24–47. doi: 10.1016/j.earscirev.2017.03.005
Aiuppa, A., Robidoux, P., Tamburello, G., Conde, V., Galle, B., Avard, G., et al. (2014). Gas measurements from the costa rica–nicaragua volcanic segment suggest possible along-arc variations in volcanic gas chemistry. Earth Planet. Sci. Lett. 407, 134–147. doi: 10.1016/j.epsl.2014.09.041
Alt, J. C., Schwarzenbach, E. M., Früh-Green, G. L., Shanks, W. C., Bernasconi, S. M., Garrido, C. J., et al. (2013). The role of serpentinites in cycling of carbon and sulfur: seafloor serpentinization and subduction metamorphism. Lithos 178, 40–54. doi: 10.1016/j.lithos.2012.12.006
Alt, J. C., and Teagle, D. A. H. (1999). The uptake of carbon during alteration of ocean crust. Geochim. Cosmochim. Acta 63, 1527–1535. doi: 10.1016/S0016-7037(99)00123-4
Amiotte Suchet, P., Probst, J.-L., and Ludwig, W. (2003). Worldwide distribution of continental rock lithology: implications for the atmospheric/soil CO2 uptake by continental weathering and alkalinity river transport to the oceans. Glob. Biogeochem. Cycles 17, 1038–1051. doi: 10.1029/2002GB001891
Annen, C. (2011). Implications of incremental emplacement of magma bodies for magma differentiation, thermal aureole dimensions and plutonism–volcanism relationships. Tectonophysics 500, 3–10. doi: 10.1016/j.tecto.2009.04.010
Armstrong McKay, D. I., Tyrrell, T., Wilson, P. A., and Foster, G. L. (2014). Estimating the impact of the cryptic degassing of large igneous provinces: a mid-miocene case-study. Earth Planet. Sci. Lett. 403, 254–262. doi: 10.1016/j.epsl.2014.06.040
Aubaud, C., Pineau, F., Jambon, A., and Javoy, M. (2004). Kinetic disequilibrium of C, He, Ar and carbon isotopes during degassing of mid-ocean ridge basalts. Earth Planet. Sci. Lett. 222, 391–406. doi: 10.1016/j.epsl.2004.03.001
Barry, P. H., Hilton, D. R., Füri, E., Halldórsson, S. A., and Grönvold, K. (2014). Carbon isotope and abundance systematics of Icelandic geothermal gases, fluids and subglacial basalts with implications for mantle plume-related CO2 fluxes. Geochim. Cosmochim. Acta 134, 74–99. doi: 10.1016/j.gca.2014.02.038
Barry, P. H., Moor, J. M., de Giovannelli, D., Schrenk, M., Hummer, D. R., Lopez, T., et al. (2019). Forearc carbon sink reduces long-term volatile recycling into the mantle. Nature 568:487. doi: 10.1038/s41586-019-1131-5
Becker, J. A., Bickle, M. J., Galy, A., and Holland, T. J. B. (2008). Himalayan metamorphic CO2 fluxes: quantitative constraints from hydrothermal springs. Earth Planet. Sci. Lett. 265, 616–629. doi: 10.1016/j.epsl.2007.10.046
Bickle, M. J. (1996). Metamorphic decarbonation, silicate weathering and the long-term carbon cycle. Terra Nova 8, 270–276. doi: 10.1111/j.1365-3121.1996.tb00756.x
Black, B. A., and Gibson, S. A. (2019). Deep carbon and the life cycle of Large Igneous Provinces. Elements 15, 319–324. doi: 10.2138/gselements.15.5.319
Black, B. A., and Manga, M. (2017). Volatiles and the tempo of flood basalt magmatism. Earth Planet. Sci. Lett. 458, 130–140. doi: 10.1016/j.epsl.2016.09.035
Blundy, J., Cashman, K. V., Rust, A., and Witham, F. (2010). A case for CO2-rich arc magmas. Earth Planet. Sci. Lett. 290, 289–301. doi: 10.1016/j.epsl.2009.12.013
Botz, R. W., and Stoffers, P. (1993). Light hydrocarbon gases in lake tanganyika hydrothermal fluids (East-Central Africa). Chem. Geol. 104, 217–224. doi: 10.1016/0009-2541(93)90152-9
Bown, J. W., and White, R. S. (1995). Effect of finite extension rate on melt generation at rifted continental margins. J. Geophys. Res. Solid Earth 100, 18011–18029. doi: 10.1029/94JB01478
Bräuer, K., Kämpf, H., Niedermann, S., and Strauch, G. (2018). Monitoring of helium and carbon isotopes in the western eger rift area (Czech republic): relationships with the 2014 seismic activity and indications for recent (2000–2016) magmatic unrest. Chem. Geol. 482, 131–145. doi: 10.1016/j.chemgeo.2018.02.017
Brune, S., Williams, S. E., and Müller, R. D. (2017). Potential links between continental rifting, CO2 degassing and climate change through time. Nat. Geosci. 10, 941–946. doi: 10.1038/s41561-017-0003-6
Bryan, S. E., and Ferrari, L. (2013). Large igneous provinces and silicic large igneous provinces: progress in our understanding over the last 25 years. GSA Bull. 125, 1053–1078. doi: 10.1130/B30820.1
Burnham, A. D., Thomson, A. R., Bulanova, G. P., Kohn, S. C., Smith, C. B., and Walter, M. J. (2015). Stable isotope evidence for crustal recycling as recorded by superdeep diamonds. Earth Planet. Sci. Lett. 432, 374–380. doi: 10.1016/j.epsl.2015.10.023
Burton, M. R., Sawyer, G. M., and Granieri, D. (2013). Deep carbon emissions from volcanoes. Rev. Mineral. Geochem. 75, 323–354. doi: 10.2138/rmg.2013.75.11
Caldeira, K., and Rampino, M. R. (1990). Carbon dioxide emissions from deccan volcanism and a K/T boundary greenhouse effect. Geophys. Res. Lett. 17, 1299–1302. doi: 10.1029/GL017i009p01299
Cao, W., Lee, C.-T. A., and Lackey, J. S. (2017a). Episodic nature of continental arc activity since 750 Ma: a global compilation. Earth Planet. Sci. Lett. 461, 85–95. doi: 10.1016/j.epsl.2016.12.044
Cao, W., Zahirovic, S., Flament, N., Williams, S. E., Golonka, J., and Müller, R. D. (2017b). Improving global paleogeography since the late paleozoic using paleobiology. Biogeosciences 14, 5425–5439. doi: 10.5194/bg-14-5425-2017
Carn, S. A., Clarisse, L., and Prata, A. J. (2016). Multi-decadal satellite measurements of global volcanic degassing. J. Volcanol. Geotherm. Res. 311, 99–134. doi: 10.1016/j.jvolgeores.2016.01.002
Carn, S. A., Fioletov, V. E., McLinden, C. A., Li, C., and Krotkov, N. A. (2017). A decade of global volcanic SO2 emissions measured from space. Sci. Rep. 7:44095. doi: 10.1038/srep44095
Carter, L. B., and Dasgupta, R. (2015). Hydrous basalt–limestone interaction at crustal conditions: implications for generation of ultracalcic melts and outflux of CO2 at volcanic arcs. Earth Planet. Sci. Lett. 427, 202–214. doi: 10.1016/j.epsl.2015.06.053
Carter, L. B., and Dasgupta, R. (2016). Effect of melt composition on crustal carbonate assimilation: implications for the transition from calcite consumption to skarnification and associated CO2 degassing. Geochem. Geophys. Geosyst. 17, 3893–3916. doi: 10.1002/2016GC006444
Carter, L. B., and Dasgupta, R. (2018). Decarbonation in the Ca-Mg-Fe carbonate system at mid-crustal pressure as a function of temperature and assimilation with arc magmas – Implications for long-term climate. Chem. Geol. 492, 30–48. doi: 10.1016/j.chemgeo.2018.05.024
Cartigny, P., Harris, J. W., and Javoy, M. (2001). Diamond genesis, mantle fractionations and mantle nitrogen content: a study of δ13C–N concentrations in diamonds. Earth Planet. Sci. Lett. 185, 85–98. doi: 10.1016/S0012-821X(00)00357-5
Cartigny, P., Pineau, F., Aubaud, C., and Javoy, M. (2008). Towards a consistent mantle carbon flux estimate: insights from volatile systematics (H2O/Ce, δD, CO2/Nb) in the North Atlantic mantle (14° N and 34° N). Earth Planet. Sci. Lett. 265, 672–685. doi: 10.1016/j.epsl.2007.11.011
Chadwick, J. P., Troll, V. R., Ginibre, C., Morgan, D., Gertisser, R., Waight, T. E., et al. (2007). Carbonate assimilation at merapi volcano, java, indonesia: insights from crystal isotope stratigraphy. J. Petrol. 48, 1793–1812. doi: 10.1093/petrology/egm038
Chavrit, D., Humler, E., and Grasset, O. (2014). Mapping modern CO2 fluxes and mantle carbon content all along the mid-ocean ridge system. Earth Planet. Sci. Lett. 387, 229–239. doi: 10.1016/j.epsl.2013.11.036
Chiodini, G., Cioni, R., Guidi, M., Raco, B., and Marini, L. (1998). Soil CO2 flux measurements in volcanic and geothermal areas. Appl. Geochem. 13, 543–552. doi: 10.1016/S0883-2927(97)00076-0
Christensen, D. H., and Ruff, L. J. (1988). Seismic coupling and outer rise earthquakes. J. Geophys. Res. Solid Earth 93, 13421–13444. doi: 10.1029/JB093iB11p13421
Clift, P. D. (2017). A revised budget for Cenozoic sedimentary carbon subduction. Rev. Geophys. 55, 97–125. doi: 10.1002/2016RG000531
Colbourn, G., Ridgwell, A., and Lenton, T. M. (2015). The time scale of the silicate weathering negative feedback on atmospheric CO2. Glob. Biogeochem. Cycles 29, 583–596. doi: 10.1002/2014GB005054
Coogan, L. A., and Dosso, S. E. (2015). Alteration of ocean crust provides a strong temperature dependent feedback on the geological carbon cycle and is a primary driver of the Sr-isotopic composition of seawater. Earth Planet. Sci. Lett. 415, 38–46. doi: 10.1016/j.epsl.2015.01.027
Coogan, L. A., and Gillis, K. M. (2013). Evidence that low-temperature oceanic hydrothermal systems play an important role in the silicate-carbonate weathering cycle and long-term climate regulation. Geochem. Geophys. Geosyst. 14, 1771–1786. doi: 10.1002/ggge.20113
Coogan, L. A., Parrish, R. R., and Roberts, N. M. W. (2016). Early hydrothermal carbon uptake by the upper oceanic crust: insight from in situ U-Pb dating. Geology 44, 147–150. doi: 10.1130/G37212.1
Copley, A., and Woodcock, N. (2016). Estimates of fault strength from the variscan foreland of the northern UK. Earth Planet. Sci. Lett. 451, 108–113. doi: 10.1016/j.epsl.2016.07.024
Courtillot, V. E., and Renne, P. R. (2003). On the ages of flood basalt events. C. R. Geosci. 335, 113–140. doi: 10.1016/S1631-0713(03)00006-3
Dasgupta, R. (2013). Ingassing, storage, and outgassing of terrestrial carbon through geologic time. Rev. Mineral. Geochem. 75, 183–229. doi: 10.2138/rmg.2013.75.7
Dasgupta, R., and Hirschmann, M. M. (2006). Melting in the Earth’s deep upper mantle caused by carbon dioxide. Nature 440, 659–662. doi: 10.1038/nature04612
Dasgupta, R., and Hirschmann, M. M. (2010). The deep carbon cycle and melting in Earth’s interior. Earth Planet. Sci. Lett. 298, 1–13. doi: 10.1016/j.epsl.2010.06.039
de Moor, J. M., Kern, C., Avard, G., Müller, C., Aiuppa, A., Saballos, A., et al. (2017). A new sulfur and carbon degassing inventory for the southern central american volcanic arc: the importance of accurate time-series data sets and possible tectonic processes responsible for temporal variations in arc-scale volatile emissions. Geochem. Geophys. Geosyst. 18, 4437–4468. doi: 10.1002/2017GC007141
Deegan, F. M., Troll, V. R., Freda, C., Misiti, V., Chadwick, J. P., McLeod, C. L., et al. (2010). Magma–carbonate interaction processes and associated CO2 release at merapi volcano, indonesia: insights from experimental petrology. J. Petrol. 51, 1027–1051. doi: 10.1093/petrology/egq010
Dessert, C., Dupré, B., François, L. M., Schott, J., Gaillardet, J., Chakrapani, G., et al. (2001). Erosion of deccan traps determined by river geochemistry: impact on the global climate and the 87Sr/86Sr ratio of seawater. Earth Planet. Sci. Lett. 188, 459–474. doi: 10.1016/S0012-821X(01)00317-X
Dessert, C., Dupré, B., Gaillardet, J., François, L. M., and Allègre, C. J. (2003). Basalt weathering laws and the impact of basalt weathering on the global carbon cycle. Chem. Geol. 202, 257–273. doi: 10.1016/j.chemgeo.2002.10.001
Dixon, J. E., Stolper, E. M., and Holloway, J. R. (1995). An experimental study of water and carbon dioxide solubilities in Mid-Ocean ridge basaltic liquids. Part I: calibration and solubility models. J. Petrol. 36, 1607–1631. doi: 10.1093/oxfordjournals.petrology.a037267
Dutkiewicz, A., Müller, R. D., Cannon, J., Vaughan, S., and Zahirovic, S. (2018). Sequestration and subduction of deep-sea carbonate in the global ocean since the early cretaceous. Geology 47, 91–94. doi: 10.1130/G45424.1
Dutkiewicz, A., Müller, R. D., O’Callaghan, S., and Jónasson, H. (2015). Census of seafloor sediments in the world’s ocean. Geology 43, 795–798. doi: 10.1130/G36883.1
Dutkiewicz, A., Müller, R. D., Wang, X., O’Callaghan, S., Cannon, J., and Wright, N. M. (2017). Predicting sediment thickness on vanished ocean crust since 200 Ma. Geochem. Geophys. Geosyst. 18, 4586–4603. doi: 10.1002/2017GC007258
Dutkiewicz, A., O’Callaghan, S., and Müller, R. D. (2016). Controls on the distribution of deep-sea sediments. Geochem. Geophys. Geosyst. 17, 3075–3098. doi: 10.1002/2016GC006428
East, M., Müller, R. D., Williams, S. E., Zahirovic, S., and Heine, C. (2019). Subduction history reveals Cretaceous slab superflux as a possible cause for the mid-Cretaceous plume pulse and superswell events. EarthArXiv
Ebinger, C. (2005). Continental break-up: the east african perspective. Astron. Geophys. 46, 16–12. doi: 10.1111/j.1468-4004.2005.46216.x
Edmond, J. M., and Huh, Y. (2003). Non-steady state carbonate recycling and implications for the evolution of atmospheric pCO2. Earth Planet. Sci. Lett. 216, 125–139. doi: 10.1016/S0012-821X(03)00510-7
Erba, E. (2006). The first 150 million years history of calcareous nannoplankton: biosphere–geosphere interactions. Palaeogeogr. Palaeoclimatol. Palaeoecol. 232, 237–250. doi: 10.1016/j.palaeo.2005.09.013
Etiope, G., Lassey, K. R., Klusman, R. W., and Boschi, E. (2008). Reappraisal of the fossil methane budget and related emission from geologic sources. Geophys. Res. Lett. 35. doi: 10.1029/2008GL033623
Evans, K. (2011). Metamorphic carbon fluxes: how much and how fast? Geology 39, 95–96. doi: 10.1130/focus012011.1
Evans, M. J., Derry, L. A., and France-Lanord, C. (2008). Degassing of metamorphic carbon dioxide from the Nepal Himalaya. Geochem. Geophys. Geosyst. 9:Q04021. doi: 10.1029/2007GC001796
Facq, S., Daniel, I., Montagnac, G., Cardon, H., and Sverjensky, D. A. (2014). In situ Raman study and thermodynamic model of aqueous carbonate speciation in equilibrium with aragonite under subduction zone conditions. Geochim. Cosmochim. Acta 132, 375–390. doi: 10.1016/j.gca.2014.01.030
Falkowski, P. G., Katz, M. E., Knoll, A. H., Quigg, A., Raven, J. A., Schofield, O., et al. (2004). The evolution of modern eukaryotic phytoplankton. Science 305, 354–360. doi: 10.1126/science.1095964
Faulkner, D. R., Jackson, C. A. L., Lunn, R. J., Schlische, R. W., Shipton, Z. K., Wibberley, C. A. J., et al. (2010). A review of recent developments concerning the structure, mechanics and fluid flow properties of fault zones. J. Struct. Geol. 32, 1557–1575. doi: 10.1016/j.jsg.2010.06.009
Fischer, T. P. (2008). Fluxes of volatiles (H2O, CO2, N2, Cl, F) from arc volcanoes. Geochem. J. 42, 21–38. doi: 10.2343/geochemj.42.21
Fischer, T. P., and Lopez, T. M. (2016). First airborne samples of a volcanic plume for δ13C of CO2 determinations. Geophys. Res. Lett. 43, 3272–3279. doi: 10.1002/2016GL068499
Foley, S. F., and Fischer, T. P. (2017). An essential role for continental rifts and lithosphere in the deep carbon cycle. Nat. Geosci. 10, 897–902. doi: 10.1038/s41561-017-0002-7
Foster, G. L., Royer, D. L., and Lunt, D. J. (2017). Future climate forcing potentially without precedent in the last 420 million years. Nat. Commun. 8:14845. doi: 10.1038/ncomms14845
France-Lanord, C., and Derry, L. A. (1997). Organic carbon burial forcing of the carbon cycle from Himalayan erosion. Nature 390, 65–67. doi: 10.1038/36324
Frondini, F., Caliro, S., Cardellini, C., Chiodini, G., Morgantini, N., and Parello, F. (2008). Carbon dioxide degassing from tuscany and Northern Latium (Italy). Glob. Planet. Chang. 61, 89–102. doi: 10.1016/j.gloplacha.2007.08.009
Gaillardet, J., and Galy, A. (2008). Himalaya–carbon sink or source? Science 320:1727. doi: 10.1126/science.1159279
Galy, V., France-Lanord, C., Beyssac, O., Faure, P., Kudrass, H., and Palhol, F. (2007). Efficient organic carbon burial in the Bengal fan sustained by the Himalayan erosional system. Nature 450:407. doi: 10.1038/nature06273
Ganino, C., and Arndt, N. T. (2009). Climate changes caused by degassing of sediments during the emplacement of large igneous provinces. Geology 37, 323–326. doi: 10.1130/G25325A.1
Gerlach, T. M., McGee, K. A., Elias, T., Sutton, A. J., and Doukas, M. P. (2002). Carbon dioxide emission rate of Kîlauea Volcano: implications for primary magma and the summit reservoir. J. Geophys. Res. Solid Earth 107, ECV 3-1–ECV 3-15. doi: 10.1029/2001JB000407
Gibson, S. A., Thompson, R. N., and Day, J. A. (2006). Timescales and mechanisms of plume–lithosphere interactions: 40Ar/39Ar geochronology and geochemistry of alkaline igneous rocks from the Paraná–Etendeka large igneous province. Earth Planet. Sci. Lett. 251, 1–17. doi: 10.1016/j.epsl.2006.08.004
Gillis, K. M., and Coogan, L. A. (2011). Secular variation in carbon uptake into the ocean crust. Earth Planet. Sci. Lett. 302, 385–392. doi: 10.1016/j.epsl.2010.12.030
Goff, F., Love, S. P., Warren, R. G., Counce, D., Obenholzner, J., Siebe, C., et al. (2001). Passive infrared remote sensing evidence for large, intermittent CO2 emissions at popocatépetl volcano, Mexico. Chem. Geol. 177, 133–156. doi: 10.1016/S0009-2541(00)00387-9
Grant, S. W. (1990). Shell structure and distribution of Cloudina, a potential index fossil for the terminal proterozoic. Am. J. Sci. 290, 261–294.
Groppo, C., Rolfo, F., Castelli, D., and Mosca, P. (2017). Metamorphic CO2 Production in collisional orogens: petrological constraints from phase diagram modeling of himalayan, scapolite-bearing, calc-silicate rocks in the NKC(F)MAS(T)-HC system. J. Petrol. 58, 53–83. doi: 10.1093/petrology/egx005
Gurnis, M., Turner, M., Zahirovic, S., DiCaprio, L., Spasojevic, S., Müller, R. D., et al. (2012). Plate tectonic reconstructions with continuously closing plates. Comput. Geosci. 38, 35–42. doi: 10.1016/j.cageo.2011.04.014
Hauri, E. H., Cottrell, E., Kelley, K. A., Tucker, J. M., Shimizu, K., Le Voyer, M., et al. (2019). “Carbon in the convecting mantle,” in Deep Carbon: Past to Present, eds B. Orcutt, R. Dasgupta, and I. Daniel (Cambridge: Cambridge University Press).
Hauri, E. H., Maclennan, J., McKenzie, D., Gronvold, K., Oskarsson, N., and Shimizu, N. (2017). CO2 content beneath northern Iceland and the variability of mantle carbon. Geology 46, 55–58. doi: 10.1130/G39413.1
Helo, C., Longpré, M.-A., Shimizu, N., Clague, D. A., and Stix, J. (2011). Explosive eruptions at mid-ocean ridges driven by CO2-rich magmas. Nat. Geosci. 4, 260–263. doi: 10.1038/ngeo1104
Hildreth, W., and Moorbath, S. (1988). Crustal contributions to arc magmatism in the andes of central chile. Contrib. Mineral. Petrol. 98, 455–489. doi: 10.1007/BF00372365
Hilton, D. R., Fischer, T. P., and Marty, B. (2002). Noble gases and volatile recycling at subduction zones. Rev. Mineral. Geochem. 47, 319–370. doi: 10.2138/rmg.2002.47.9
Huh, Y. (2010). “Estimation of atmospheric CO2 uptake by silicate weathering in the himalayas and the tibetan plateau: a review of existing fluvial geochemical data,” in Monsoon Evolution and Tectonic-Climate Linkage in Asia, eds P. D. Clift, R. Tada, and H. Zheng, (London: Geological Society).
Hunt, J. A., Zafu, A., Mather, T. A., Pyle, D. M., and Barry, P. H. (2017). Spatially variable CO2 degassing in the main Ethiopian rift: implications for magma storage, volatile transport, and rift-related emissions. Geochem. Geophys. Geosyst. 18, 3714–3737. doi: 10.1002/2017GC006975
Iacono-Marziano, G., Gaillard, F., and Pichavant, M. (2008). Limestone assimilation by basaltic magmas: an experimental re-assessment and application to Italian volcanoes. Contrib. Mineral. Petrol. 155, 719–738. doi: 10.1007/s00410-007-0267-8
Iacono-Marziano, G., Gaillard, F., Scaillet, B., Pichavant, M., and Chiodini, G. (2009). Role of non-mantle CO2 in the dynamics of volcano degassing: the mount vesuvius example. Geology 37, 319–322. doi: 10.1130/G25446A.1
Ibs-von Seht, M., Plenefisch, T., and Klinge, K. (2008). Earthquake swarms in continental rifts – A comparison of selected cases in America, Africa and Europe. Tectonophysics 452, 66–77. doi: 10.1016/j.tecto.2008.02.008
Ilyinskaya, E., Mobbs, S., Burton, R., Burton, M., Pardini, F., Pfeffer, M. A., et al. (2018). Globally significant CO2 emissions from Katla, a Subglacial Volcano in Iceland. Geophys. Res. Lett. 45, 332–310. doi: 10.1029/2018GL079096
Jarrard, R. D. (2003). Subduction fluxes of water, carbon dioxide, chlorine, and potassium. Geochem. Geophys. Geosyst. 4:8905. doi: 10.1029/2002GC000392
Javoy, M., and Pineau, F. (1991). The volatiles record of a “popping” rock from the Mid-atlantic ridge at 14°N: chemical and isotopic composition of gas trapped in the vesicles. Earth Planet. Sci. Lett. 107, 598–611. doi: 10.1016/0012-821X(91)90104-P
Johansson, L., Zahirovic, S., and Müller, R. D. (2018). The interplay between the eruption and weathering of large igneous provinces and the deep-time carbon cycle. Geophys. Res. Lett. 45, 5380–5389. doi: 10.1029/2017GL076691
Johnston, F. K. B., Turchyn, A. V., and Edmonds, M. (2011). Decarbonation efficiency in subduction zones: implications for warm cretaceous climates. Earth Planet. Sci. Lett. 303, 143–152. doi: 10.1016/j.epsl.2010.12.049
Jones, M. T., Hembury, D. J., Palmer, M. R., Tonge, B., Darling, W. G., and Loughlin, S. C. (2011). The weathering and element fluxes from active volcanoes to the oceans: a montserrat case study. Bull. Volcanol. 73, 207–222. doi: 10.1007/s00445-010-0397-0
Jones, M. T., Jerram, D. A., Svensen, H. H., and Grove, C. (2016). The effects of large igneous provinces on the global carbon and sulphur cycles. Palaeogeogr. Palaeoclimatol. Palaeoecol. 441, 4–21. doi: 10.1016/j.palaeo.2015.06.042
Kämpf, H., Bräuer, K., Schumann, J., Hahne, K., and Strauch, G. (2013). CO2 discharge in an active, non-volcanic continental rift area (Czech republic): characterisation (δ13C, 3He/4He) and quantification of diffuse and vent CO2 emissions. Chem. Geol. 339, 71–83. doi: 10.1016/j.chemgeo.2012.08.005
Kelemen, P. B., and Manning, C. E. (2015). Reevaluating carbon fluxes in subduction zones, what goes down, mostly comes up. Proc. Natl. Acad. Sci. U.S.A. 112, E3997–E4006. doi: 10.1073/pnas.1507889112
Keller, T., Katz, R. F., and Hirschmann, M. M. (2017). Volatiles beneath mid-ocean ridges: deep melting, channelised transport, focusing, and metasomatism. Earth Planet. Sci. Lett. 464, 55–68. doi: 10.1016/j.epsl.2017.02.006
Kennedy, B. M., Kharaka, Y. K., Evans, W. C., Ellwood, A., DePaolo, D. J., Thordsen, J., et al. (1997). Mantle fluids in the san andreas fault system, California. Science 278, 1278–1281. doi: 10.1126/science.278.5341.1278
Kerrick, D. M. (2001). Present and past nonanthropogenic CO2 degassing from the solid earth. Rev. Geophys. 39, 565–585. doi: 10.1029/2001RG000105
Kerrick, D. M., and Caldeira, K. (1998). Metamorphic CO2 degassing from orogenic belts. Chem. Geol. 145, 213–232. doi: 10.1016/S0009-2541(97)00144-7
Kerrick, D. M., and Connolly, J. A. D. (2001). Metamorphic devolatilization of subducted oceanic metabasalts: implications for seismicity, arc magmatism and volatile recycling. Earth Planet. Sci. Lett. 189, 19–29. doi: 10.1016/S0012-821X(01)00347-8
Kodosky, L. G., Motyka, R. J., and Symonds, R. B. (1991). Fumarolic emissions from Mount St. augustine, alaska: 1979–1984 degassing trends, volatile sources and their possible role in eruptive style. Bull. Volcanol. 53, 381–394. doi: 10.1007/BF00280228
Kumar, N., Anderson, R. F., Mortlock, R. A., Froelich, P. N., Kubik, P., Dittrich-Hannen, B., et al. (1995). Increased biological productivity and export production in the glacial Southern Ocean. Nature 378, 675–680. doi: 10.1038/378675a0
Le Guern, F. (1987). Mechanism of energy transfer in the lava lake of niragongo (Zaire), 1959–1977. J. Volcanol. Geotherm. Res. 31, 17–31. doi: 10.1016/0377-0273(87)90003-5
Le Voyer, M., Hauri, E. H., Cottrell, E., Kelley, K. A., Salters, V. J. M., Langmuir, C. H., et al. (2018). Carbon fluxes and primary magma CO2 contents along the global mid-ocean ridge system. Geochem. Geophys. Geosyst. 20, 1387–1424. doi: 10.1029/2018GC007630
Le Voyer, M., Kelley, K. A., Cottrell, E., and Hauri, E. H. (2017). Heterogeneity in mantle carbon content from CO2-undersaturated basalts. Nat. Commun. 8:14062. doi: 10.1038/ncomms14062
Lee, C.-T. A., Jiang, H., Dasgupta, R., and Torres, M. (2019). “A framework for understanding whole earth carbon cycling,” in Deep Carbon: Past to Present, eds B. Orcutt, R. Dasgupta, and I. Daniel (Cambridge: Cambridge University Press).
Lee, C.-T. A., and Lackey, J. S. (2015). Global continental arc flare-ups and their relation to long-term greenhouse conditions. Elements 11, 125–130. doi: 10.2113/gselements.11.2.125
Lee, C.-T. A., Shen, B., Slotnick, B. S., Liao, K., Dickens, G. R., Yokoyama, Y., et al. (2013). Continental arc–island arc fluctuations, growth of crustal carbonates, and long-term climate change. Geosphere 9, 21–36. doi: 10.1130/GES00822.1
Lee, C.-T. A., Thurner, S., Paterson, S., and Cao, W. (2015). The rise and fall of continental arcs: interplays between magmatism, uplift, weathering, and climate. Earth Planet. Sci. Lett. 425, 105–119. doi: 10.1016/j.epsl.2015.05.045
Lee, H., Muirhead, J. D., Fischer, T. P., Ebinger, C. J., Kattenhorn, S. A., Sharp, Z. D., et al. (2016). Massive and prolonged deep carbon emissions associated with continental rifting. Nat. Geosci. 9, 145–149. doi: 10.1038/ngeo2622
Lefeldt, M., Ranero, C. R., and Grevemeyer, I. (2012). Seismic evidence of tectonic control on the depth of water influx into incoming oceanic plates at subduction trenches. Geochem. Geophys. Geosyst. 13:Q05013. doi: 10.1029/2012GC004043
Lenton, T. M., Daines, S. J., and Mills, B. J. W. (2018). COPSE reloaded: an improved model of biogeochemical cycling over Phanerozoic time. Earth Sci. Rev. 178, 1–28. doi: 10.1016/j.earscirev.2017.12.004
Lopez, T. M., Fischer, T. P., Plank, T. A., Malinverno, A., Rizzo, A. L., Rasmussen, D. J., et al. (2018). Tracing volatile cycling from subduction to outgassing along the Aleutian Arc, in Proceedings of the V43I-0246 AGU Fall Meeting Abstracts, Washington, DC.
Manning, C. E. (2004). The chemistry of subduction-zone fluids. Earth Planet. Sci. Lett. 223, 1–16. doi: 10.1016/j.epsl.2004.04.030
Marty, B., Alexander, C. M. O., and Raymond, S. N. (2013). Primordial origins of Earth’s carbon. Rev. Mineral. Geochem. 75, 149–181. doi: 10.2138/rmg.2013.75.6
Marty, B., and Tolstikhin, I. N. (1998). CO2 fluxes from mid-ocean ridges, arcs and plumes. Chem. Geol. 145, 233–248. doi: 10.1016/S0009-2541(97)00145-9
Mason, E., Edmonds, M., and Turchyn, A. V. (2017). Remobilization of crustal carbon may dominate volcanic arc emissions. Science 357, 290–294. doi: 10.1126/science.aan5049
Matthews, K. J., Maloney, K. T., Zahirovic, S., Williams, S. E., Seton, M., and Müller, R. D. (2016). Global plate boundary evolution and kinematics since the late Paleozoic. Glob. Planet. Chang. 146, 226–250. doi: 10.1016/j.gloplacha.2016.10.002
Matthews, S., Shorttle, O., Rudge, J. F., and Maclennan, J. (2017). Constraining mantle carbon: CO2-trace element systematics in basalts and the roles of magma mixing and degassing. Earth Planet. Sci. Lett. 480, 1–14. doi: 10.1016/j.epsl.2017.09.047
McKenzie, D. (1989). Some remarks on the movement of small melt fractions in the mantle. Earth Planet. Sci. Lett. 95, 53–72. doi: 10.1016/0012-821X(89)90167-2
McKenzie, N. R., Horton, B. K., Loomis, S. E., Stockli, D. F., Planavsky, N. J., and Lee, C.-T. A. (2016). Continental arc volcanism as the principal driver of icehouse-greenhouse variability. Science 352, 444–447. doi: 10.1126/science.aad5787
McKenzie, N. R., Hughes, N. C., Gill, B. C., and Myrow, P. M. (2014). Plate tectonic influences on neoproterozoic–early Paleozoic climate and animal evolution. Geology 42, 127–130. doi: 10.1130/G34962.1
Merdith, A. S., Collins, A. S., Williams, S. E., Pisarevsky, S., Foden, J. D., Archibald, D. B., et al. (2017). A full-plate global reconstruction of the neoproterozoic. Gondwana Res. 50, 84–134. doi: 10.1016/j.gr.2017.04.001
Merdith, A. S., Williams, S. E., Brune, S., Collins, A. S., and Müller, R. D. (2019). Rift and plate boundary evolution across two supercontinent cycles. Glob. Planet. Chang. 173, 1–14. doi: 10.1016/j.gloplacha.2018.11.006
Michael, P. J., and Graham, D. W. (2015). The behavior and concentration of CO2 in the suboceanic mantle: inferences from undegassed ocean ridge and ocean island basalts. Lithos 23, 338–351. doi: 10.1016/j.lithos.2015.08.020
Muirhead, J. D., Kattenhorn, S. A., Lee, H., Mana, S., Turrin, B. D., Fischer, T. P., et al. (2016). Evolution of upper crustal faulting assisted by magmatic volatile release during early-stage continental rift development in the East African Rift. Geosphere 12, 1670–1700. doi: 10.1130/GES01375.1
Müller, R. D., Cannon, J., Qin, X., Watson, R. J., Gurnis, M., Williams, S., et al. (2018). GPlates: building a virtual earth through deep time. Geochem. Geophys. Geosyst. 19, 2243–2261. doi: 10.1029/2018GC007584
Müller, R. D., and Dutkiewicz, A. (2018). Oceanic crustal carbon cycle drives 26-million-year atmospheric carbon dioxide periodicities. Sci. Adv. 4:eaaq0500. doi: 10.1126/sciadv.aaq0500
Müller, R. D., Seton, M., Zahirovic, S., Williams, S. E., Matthews, K. J., Wright, N. M., et al. (2016). Ocean basin evolution and global-scale plate reorganization events since pangea breakup. Ann. Rev. Earth Planet. Sci. 44, 107–138. doi: 10.1146/annurev-earth-060115-012211
Naliboff, J. B., Billen, M. I., Gerya, T., and Saunders, J. (2013). Dynamics of outer-rise faulting in oceanic-continental subduction systems. Geochem. Geophys. Geosyst. 14, 2310–2327. doi: 10.1002/ggge.20155
Pälike, H., Lyle, M. W., Nishi, H., Raffi, I., Ridgwell, A., Gamage, K., et al. (2012). A Cenozoic record of the equatorial Pacific carbonate compensation depth. Nature 488, 609–614. doi: 10.1038/nature11360
Pall, J., Zahirovic, S., Doss, S., Hassan, R., Matthews, K. J., Cannon, J., et al. (2018). The influence of carbonate platform interactions with subduction zone volcanism on palaeo-atmospheric CO2; since the devonian. Clim. Past 14, 857–870. doi: 10.5194/cp-14-857-2018
Parks, M. M., Caliro, S., Chiodini, G., Pyle, D. M., Mather, T. A., Berlo, K., et al. (2013). Distinguishing contributions to diffuse CO2 emissions in volcanic areas from magmatic degassing and thermal decarbonation using soil gas 222Rn–δ13C systematics: application to santorini volcano. Greece. Earth Planet. Sci. Lett. 37, 180–190. doi: 10.1016/j.epsl.2013.06.046
Percival, L. M. E., Jenkyns, H. C., Mather, T. A., Dickson, A. J., Batenburg, S. J., Ruhl, M., et al. (2018). Does large igneous province volcanism always perturb the mercury cycle? comparing the records of oceanic anoxic event 2 and the end-cretaceous to other mesozoic events. Am. J. Sci. 318, 799–860. doi: 10.2475/08.2018.01
Percival, L. M. E., Ruhl, M., Hesselbo, S. P., Jenkyns, H. C., Mather, T. A., and Whiteside, J. H. (2017). Mercury evidence for pulsed volcanism during the end-Triassic mass extinction. Proc. Natl. Acad. Sci. U.S.A. 114, 7929–7934. doi: 10.1073/pnas.1705378114
Percival, L. M. E., Witt, M. L. I., Mather, T. A., Hermoso, M., Jenkyns, H. C., Hesselbo, S. P., et al. (2015). Globally enhanced mercury deposition during the end-pliensbachian extinction and toarcian OAE: a link to the karoo–ferrar large igneous Province. Earth Planet. Sci. Lett. 428, 267–280. doi: 10.1016/j.epsl.2015.06.064
Plank, T. (2014). “4.17 - the chemical composition of subducting sediments,” in Treatise on Geochemistry, eds H. D. Holland, and K. K. Turekian, (Amsterdam: Elsevier).
Plank, T., and Langmuir, C. H. (1998). The chemical composition of subducting sediment and its consequences for the crust and mantle. Chem. Geol. 145, 325–394. doi: 10.1016/S0009-2541(97)00150-2
Poland, M. P., Miklius, A., Jeff Sutton, A., and Thornber, C. R. (2012). A mantle-driven surge in magma supply to Kîlauea Volcano during 2003–2007. Nat. Geosci. 5, 295–300. doi: 10.1038/ngeo1426
Poli, S. (2015). Carbon mobilized at shallow depths in subduction zones by carbonatitic liquids. Nat. Geosci. 8, 633–636. doi: 10.1038/ngeo2464
Ranero, C. R., Phipps Morgan, J., McIntosh, K., and Reichert, C. (2003). Bending-related faulting and mantle serpentinization at the middle America trench. Nature 425, 367–373. doi: 10.1038/nature01961
Ratschbacher, B. C., Paterson, S. R., and Fischer, T. P. (2019). Spatial and depth-dependent variations in magma volume addition and addition rates to continental arcs: application to global CO 2 fluxes since 750 Ma. Geochem. Geophys. Geosyst. 20, 2997–3018. doi: 10.1029/2018GC008031
Raymo, M. E., and Ruddiman, W. F. (1992). Tectonic forcing of late cenozoic climate. Nature 359, 117–122. doi: 10.1038/359117a0
Rea, D. K., and Ruff, L. J. (1996). Composition and mass flux of sediment entering the world’s subduction zones: implications for global sediment budgets, great earthquakes, and volcanism. Earth Planet. Sci. Lett. 140, 1–12. doi: 10.1016/0012-821X(96)00036-2
Resing, J. A., Lupton, J. E., Feely, A., and Lilley, M. D. (2004). CO2 and 3He in hydrothermal plumes: implications for mid-ocean ridge CO2 flux. Earth Planet. Sci. Lett. 226, 449–464. doi: 10.1016/j.epsl.2004.07.028
Richards, M. A., Duncan, R. A., and Courtillot, V. E. (1989). Flood basalts and Hot-spot tracks: plume heads and tails. Science 246, 103–107. doi: 10.1126/science.246.4926.103
Ridgwell, A. (2005). A mid mesozoic revolution in the regulation of ocean chemistry. Mar. Geol. 217, 339–357. doi: 10.1016/j.margeo.2004.10.036
Ridgwell, A., and Zeebe, R. E. (2005). The role of the global carbonate cycle in the regulation and evolution of the Earth system. Earth Planet. Sci. Lett. 234, 299–315. doi: 10.1016/j.epsl.2005.03.006
Ring, U., Tonguç Uysal, I., Yüce, G., Ünal-Ýmer, E., Italiano, F., Ýmer, A., et al. (2016). Recent mantle degassing recorded by carbonic spring deposits along sinistral strike-slip faults, south-central Australia. Earth Planet. Sci. Lett. 454, 304–318. doi: 10.1016/j.epsl.2016.09.017
Rooney, T. O. (2017). The Cenozoic magmatism of East-Africa: part I – flood basalts and pulsed magmatism. Lithos 28, 264–301. doi: 10.1016/j.lithos.2017.05.014
Rooney, T. O., Nelson, W. R., Ayalew, D., Hanan, B., Yirgu, G., and Kappelman, J. (2017). Melting the lithosphere: metasomes as a source for mantle-derived magmas. Earth Planet. Sci. Lett. 461, 105–118. doi: 10.1016/j.epsl.2016.12.010
Rosenthal, A., Hauri, E. H., and Hirschmann, M. M. (2015). Experimental determination of C, F, and H partitioning between mantle minerals and carbonated basalt, CO2/Ba and CO2/Nb systematics of partial melting, and the CO2 contents of basaltic source regions. Earth Planet. Sci. Lett. 412, 77–87. doi: 10.1016/j.epsl.2014.11.044
Ross, P.-S., Ukstins Peate, I., McClintock, M. K., Xu, Y. G., Skilling, I. P., White, J. D. L., et al. (2005). Mafic volcaniclastic deposits in flood basalt provinces: a review. J. Volcanol. Geotherm. Res. 145, 281–314. doi: 10.1016/j.jvolgeores.2005.02.003
Rugenstein, J. K. C., Ibarra, D. E., and von Blanckenburg, F. (2019). Neogene cooling driven by land surface reactivity rather than increased weathering fluxes. Nature 571, 99–102. doi: 10.1038/s41586-019-1332-y
Rüpke, L. H., Morgan, J. P., Hort, M., and Connolly, J. A. D. (2004). Serpentine and the subduction zone water cycle. Earth Planet. Sci. Lett. 223, 17–34. doi: 10.1016/j.epsl.2004.04.018
Saal, A. E., Hauri, E. H., Langmuir, C. H., and Perfit, M. R. (2002). Vapour undersaturation in primitive mid-ocean-ridge basalt and the volatile content of Earth’s upper mantle. Nature 419, 451–455. doi: 10.1038/nature01073
Sano, Y., and Marty, B. (1995). Origin of carbon in fumarolic gas from island arcs. Chem. Geol. 119, 265–274. doi: 10.1016/0009-2541(94)00097-R
Sano, Y., and Williams, S. N. (1996). Fluxes of mantle and subducted carbon along convergent plate boundaries. Geophys. Res. Lett. 23, 2749–2752. doi: 10.1029/96GL02260
Sarmiento, J. L., Gruber, N., Brzezinski, M. A., and Dunne, J. P. (2004). High-latitude controls of thermocline nutrients and low latitude biological productivity. Nature 427, 56–60. doi: 10.1038/nature02127
Schiano, P., Clocchiatti, R., Ottolini, L., and Busà, T. (2001). Transition of Mount Etna lavas from a mantle-plume to an island-arc magmatic source. Nature 412, 900–904. doi: 10.1038/35091056
Schwarzenbach, E. M., Früh-Green, G. L., Bernasconi, S. M., Alt, J. C., and Plas, A. (2013). Serpentinization and carbon sequestration: a study of two ancient peridotite-hosted hydrothermal systems. Chem. Geol. 351, 115–133. doi: 10.1016/j.chemgeo.2013.05.016
Self, S., Thordarson, T., and Widdowson, M. (2005). Gas fluxes from flood basalt eruptions. Elements 1, 283–287. doi: 10.2113/gselements.1.5.283
Self, S., Widdowson, M., Thordarson, T., and Jay, A. E. (2006). Volatile fluxes during flood basalt eruptions and potential effects on the global environment: a Deccan perspective. Earth Planet. Sci. Lett. 248, 518–532. doi: 10.1016/j.epsl.2006.05.041
Şengör, A. M. C., and Natal’in, B. A. (2001). “Rifts of the world,” in Mantle Plumes: Their Identification Through Time, eds R. Ernst, and K. Buchan, (Boulder, CO: Geological Society of America), 389–482.
Seton, M., Müller, R. D., Zahirovic, S., Gaina, C., Torsvik, T., Shephard, G., et al. (2012). Global continental and ocean basin reconstructions since 200Ma. Earth Sci. Rev. 113, 212–270. doi: 10.1016/j.earscirev.2012.03.002
Shaw, A. M., Behn, M. D., Humphris, S. E., Sohn, R. A., and Gregg, P. M. (2010). Deep pooling of low degree melts and volatile fluxes at the 85°E segment of the gakkel ridge: evidence from olivine-hosted melt inclusions and glasses. Earth Planet. Sci. Let. 289, 311–322. doi: 10.1016/j.epsl.2009.11.018
Shaw, A. M., Hilton, D. R., Fischer, T. P., Walker, J. A., and Alvarado, G. E. (2003). Contrasting He–C relationships in nicaragua and costa rica: insights into C cycling through subduction zones. Earth Planet. Sci. Lett. 214, 499–513. doi: 10.1016/S0012-821X(03)00401-1
Shilobreeva, S., Martinez, I., Busigny, V., Agrinier, P., and Laverne, C. (2011). Insights into C and H storage in the altered oceanic crust: results from ODP/IODP Hole 1256D. Geochim. Cosmochim. Acta 75, 2237–2255. doi: 10.1016/j.gca.2010.11.027
Skelton, A. (2011). Flux rates for water and carbon during greenschist facies metamorphism. Geology 39, 43–46. doi: 10.1130/G31328.1
Sleep, N. H. (1990). Hotspots and mantle plumes: some phenomenology. J. Geophys. Res. Solid Earth 95, 6715–6736. doi: 10.1029/JB095iB05p06715
Smart, K. A., Chacko, T., Stachel, T., Muehlenbachs, K., Stern, R. A., and Heaman, L. M. (2011). Diamond growth from oxidized carbon sources beneath the Northern Slave Craton, Canada: a δ13C–N study of eclogite-hosted diamonds from the jericho kimberlite. Geochim. Cosmochim. Acta 75, 6027–6047. doi: 10.1016/j.gca.2011.07.028
Sobolev, S. V., Sobolev, A. V., Kuzmin, D. V., Krivolutskaya, N. A., Petrunin, A. G., Arndt, N. T., et al. (2011). Linking mantle plumes, large igneous provinces and environmental catastrophes. Nature 477, 312–316. doi: 10.1038/nature10385
Solano, J. M. S., Jackson, M. D., Sparks, R. S. J., Blundy, J. D., and Annen, C. (2012). Melt segregation in deep crustal hot zones: a mechanism for chemical differentiation, crustal assimilation and the formation of evolved magmas. J. Petrol. 53, 1999–2026. doi: 10.1093/petrology/egs041
Staudigel, H., Hart, S. R., Schmincke, H.-U., and Smith, B. M. (1989). Cretaceous ocean crust at DSDP Sites 417 and 418: carbon uptake from weathering versus loss by magmatic outgassing. Geochim. Cosmochim. Acta 53, 3091–3094. doi: 10.1016/0016-7037(89)90189-0
Svensen, H., and Jamtveit, B. (2010). Metamorphic fluids and global environmental changes. Elements 6, 179–182. doi: 10.2113/gselements.6.3.179
Svensen, H., Planke, S., Polozov, A. G., Schmidbauer, N., Corfu, F., Podladchikov, Y. Y., et al. (2009). Siberian gas venting and the end-Permian environmental crisis. Earth Planet. Sci. Lett. 277, 490–500. doi: 10.1016/j.epsl.2008.11.015
Symonds, R. B., Poreda, R. J., Evans, W. C., Janik, C. J., and Ritchie, B. E. (2003). Mantle and Crustal Sources of Carbon, Nitrogen, and Noble Gases in Cascade-Range and Aleutian-Arc Volcanic Gases. USGS Open File Report No. 03–436. Reston, VA: USGS.
Tamburello, G., Pondrelli, S., Chiodini, G., and Rouwet, D. (2018). Global-scale control of extensional tectonics on CO2 earth degassing. Nat. Commun. 9:4608. doi: 10.1038/s41467-018-07087-z
Thomson, A. R., Kohn, S. C., Bulanova, G. P., Smith, C. B., Araujo, D., Walter, M. J., et al. (2014). Origin of sub-lithospheric diamonds from the Juina-5 kimberlite (Brazil): constraints from carbon isotopes and inclusion compositions. Contrib. Mineral. Petrol. 168:1081. doi: 10.1007/s00410-014-1081-8
Thomson, A. R., Walter, M. J., Kohn, S. C., and Brooker, R. A. (2016). Slab melting as a barrier to deep carbon subduction. Nature 529, 76–79. doi: 10.1038/nature16174
Tucker, J. M., Mukhopadhyay, S., and Gonnermann, H. M. (2018). Reconstructing mantle carbon and noble gas contents from degassed mid-ocean ridge basalts. Earth Planet. Sci. Lett. 496, 108–119. doi: 10.1016/j.epsl.2018.05.024
van Andel, T. H. (1975). Mesozoic/cenozoic calcite compensation depth and the global distribution of calcareous sediments. Earth Planet. Sci. Lett. 26, 187–194. doi: 10.1016/0012-821X(75)90086-2
van Avendonk, H. J. A., Holbrook, W. S., Lizarralde, D., and Denyer, P. (2011). Structure and serpentinization of the subducting Cocos plate offshore nicaragua and Costa Rica. Geochem. Geophys. Geosyst. 12:Q06009. doi: 10.1029/2011GC003592
van der Meer, D. G., Zeebe, R. E., van Hinsbergen, D. J. J., Sluijs, A., Spakman, W., and Torsvik, T. H. (2014). Plate tectonic controls on atmospheric CO2 levels since the Triassic. Proc. Natl. Acad. Sci. U.S.A. 111, 4380–4385. doi: 10.1073/pnas.1315657111
von Huene, R., and Scholl, D. W. (1991). Observations at convergent margins concerning sediment subduction, subduction erosion, and the growth of continental crust. Rev. Geophys. 29, 279–316. doi: 10.1029/91RG00969
Wallace, P. J. (2005). Volatiles in subduction zone magmas: concentrations and fluxes based on melt inclusion and volcanic gas data. J. Volcanol. Geotherm. Res. 140, 217–240. doi: 10.1016/j.jvolgeores.2004.07.023
Wallace, P. J., Kamenetsky, V. S., and Cervantes, P. (2015). Melt inclusion CO2 contents, pressures of olivine crystallization, and the problem of shrinkage bubbles. Am. Mineral. 100, 787–794. doi: 10.2138/am-2015-5029
Walling, D. E., and Fang, D. (2003). Recent trends in the suspended sediment loads of the world’s rivers. Glob. Planet. Chang. 39, 111–126. doi: 10.1016/S0921-8181(03)00020-1
Weinlich, F. H., Bräuer, K., Kämpf, H., Strauch, G., Tesaø, J., and Weise, S. M. (1999). An active subcontinental mantle volatile system in the western Eger rift, Central Europe: gas flux, isotopic (He, C, and N) and compositional fingerprints. Geochim. Cosmochim. Acta 63, 3653–3671. doi: 10.1016/S0016-7037(99)00187-8
Werner, C., Fischer, T. P., Aiuppa, A., Edmonds, M., Cardellini, C., Carn, S., et al. (2019). “Carbon dioxide emissions from subaerial volcanic regions: two decades in review,” in Deep Carbon: Past to Present, eds B. Orcutt, R. Dasgupta, and I. Daniel (Cambridge: Cambridge University Press).
White, R. S., and McKenzie, D. P. (1989). Magmatism at rift zones: the generation of volcanic continental margins and flood basalts. J. Geophys. Res. Solid Earth 94, 7685–7729. doi: 10.1029/JB094iB06p07685
White, R. S., and McKenzie, D. P. (1995). Mantle plumes and flood basalts. J. Geophys. Res. Solid Earth 100, 17543–17585. doi: 10.1029/95JB01585
White, R. S., Minshull, T. A., Bickle, M. J., and Robinson, C. J. (2001). Melt generation at very slow-spreading oceanic ridges: constraints from geochemical and geophysical data. J. Petrol. 42, 1171–1196. doi: 10.1093/petrology/42.6.1171
Zeebe, R. E. (2012). History of seawater carbonate chemistry, atmospheric Co2, and ocean acidification. Ann. Rev. Earth Planet. Sci. 40, 141–165. doi: 10.1146/annurev-earth-042711-105521
Keywords: deep carbon cycle, carbonate assimilation, solid Earth degassing, plate reconstructions, carbon dioxide, subduction zone
Citation: Wong K, Mason E, Brune S, East M, Edmonds M and Zahirovic S (2019) Deep Carbon Cycling Over the Past 200 Million Years: A Review of Fluxes in Different Tectonic Settings. Front. Earth Sci. 7:263. doi: 10.3389/feart.2019.00263
Received: 05 June 2019; Accepted: 24 September 2019;
Published: 11 October 2019.
Edited by:
Jacob B. Lowenstern, United States Geological Survey, United StatesReviewed by:
Esther M. Schwarzenbach, Freie Universität Berlin, GermanyBarbara Ratschbacher, California Institute of Technology, United States
Copyright © 2019 Wong, Mason, Brune, East, Edmonds and Zahirovic. This is an open-access article distributed under the terms of the Creative Commons Attribution License (CC BY). The use, distribution or reproduction in other forums is permitted, provided the original author(s) and the copyright owner(s) are credited and that the original publication in this journal is cited, in accordance with accepted academic practice. No use, distribution or reproduction is permitted which does not comply with these terms.
*Correspondence: Kevin Wong, ZWVrd0BsZWVkcy5hYy51aw==