- 1Institute of Earth Sciences, The Hebrew University of Jerusalem, Jerusalem, Israel
- 2Department of Earth Sciences, Royal Holloway University of London, Egham, United Kingdom
- 3Institute of Geosciences, Goethe-University, Frankfurt, Germany
Two species of Amphistegina were cultured under four variable DIC concentrations (2340–2570 μM). The variability of trace elements within the foraminiferal shells was measured in the knob area of three individuals for each DIC treatment using LA-ICPMS. In individuals that showed significant growth (identified via 135Ba-enriched seawater), B, Na, and Sr showed an increase with DIC, while K and Mg were slightly lower or unchanged. Sharp transition zones between natural 135Ba and the ∼10-fold increased 135Ba in the shells represent one quarter of a new additional chamber, which occurs roughly once a week. The shape of the transition zone is best described by a logistic equation for population growth. We propose that this reflects the dynamics of seawater vacuoles that serve the biomineralization process and provide Ca and DIC for calcification of Amphistegina as described in previous publications (e.g., Bentov et al., 2009). LA-ICPMS profiles in the central knob (∼70 μm depth) also revealed previously described cyclical changes in concentration of Mg, each apparently representing a growth of a new chamber. Additional elements such as K, Na and U showed similar cycles with the same frequency and phase as the Mg cycles. Sr showed variability with similar frequency but not in-phase to those of the Mg. These multi-element cycles were found both in the newly grown calcite (elevated-135Ba) and in the natural skeleton regardless of the DIC treatments. These high Mg and multi-element cycles seem to be an essential part of the calcification process. They may originate from the interaction with the organic matrix resulting in elevated Mg and other elements in primary calcite while secondary calcite of the lamination process shows lower concentrations. It is also possible that primary calcite is enriched in trace elements if an Amorphous CaCO3 (ACC) or vaterite precursors are involved. In addition, Rayleigh fractionation from a semi-closed reservoir, the presence of high Mg in the lattice or any combination of the previous causes may explain the trace elements enrichment. Changes in the DIC did not affect the pattern of elemental cycles in these foraminifera, suggesting that this variability is inherent to the biomineralization process.
Introduction
Foraminifera shells are well-known archives for paleoceanography and paleoclimate reconstructions. In addition to the use of foraminifera for biostratigraphy and paleoecology (e.g., Climap project, 1976; Crowley, 2000), stable isotopes (δ18O and δ13C), trace elements and their isotopes (Cd/Ca, Mg/Ca, U/Ca, δ11B, and more) are successfully used for studying past ocean chemistry and paleocirculation (e.g., Emiliani and Shackleton, 1974; Sanyal et al., 1996; Lea, 1999; Nürnberg, 2000; Barker and Elderfield, 2002; Lear et al., 2002; Katz et al., 2010; Allen et al., 2016; Foster and Rae, 2016). Recently it has been proposed that Na/Ca could be used to reconstruct past ocean calcium concentrations (Hauzer et al., 2018). However, different species of foraminifera at the same location show different shell chemistries and isotopic compositions, which are attributed to “vital effects” representing deviations from expected thermodynamic equilibrium (e.g., Erez, 1978). These deviations are mostly associated with the calcification process that is biologically controlled and thus may affect the incorporation of trace and minor elements and their isotopes into the calcite shells (e.g., Erez, 1978, 2003; Elderfield et al., 1996; Bentov and Erez, 2006; Zeebe et al., 2008; de Nooijer et al., 2014; Gussone et al., 2016). One of the main factors that control foraminiferal calcification is the carbonate system in seawater (e.g., ter Kuile et al., 1989; Spero et al., 1997; Erez, 2003). It is therefore expected that the increase in atmospheric CO2 (pCO2), causing ocean acidification, may reduce foraminiferal calcification as well as affect their shell chemistry (e.g., Erez, 2003; Kuroyanagi et al., 2009; Dias et al., 2010; Fujita et al., 2011; Vogel and Uthicke, 2012; McIntyre-Wressnig et al., 2013). For example Mg/Ca in planktic foraminifera shows species-specific sensitivity to the carbonate system (e.g., Russell et al., 2004; Kisakürek et al., 2008; Allen et al., 2016; Evans et al., 2016, 2018; Holland et al., 2017; Gray and Evans, 2019).
An additional complication in the study of foraminiferal proxies is the intra-shell compositional variability (or banding) within individual specimens of both planktic and benthic foraminifera. This has been demonstrated in both trace elements and stable isotopes (e.g., Erez, 2003; Eggins et al., 2004; Rollion-Bard et al., 2008; Hathorne et al., 2009; Branson et al., 2015; Spero et al., 2015; Jonkers et al., 2016; Fehrenbacher et al., 2017; van Dijk et al., 2017a, 2019; Geerken et al., 2019; Davis et al., 2020). Intensive experimental work (Eggins et al., 2004; Spero et al., 2015; Jonkers et al., 2016; Fehrenbacher et al., 2017) on planktic foraminifera demonstrated that Mg-rich bands are deposited during the night hours while low-Mg bands are precipitated during the daytime, perhaps connected with mitochondrial activity. Erez (2003) proposed that in large benthic foraminifera banding occurs when a new chamber is created in a two-step process: the first layer of organic-rich matrix (primary calcite) is associated with high concentrations of trace elements, while the secondary thick layer, often termed lamination, covers the existing exposed chambers and is composed of low trace element calcite (secondary calcite). The alteration between high and low elemental bands may thus be attributed to the process of sequential chamber formation (Erez, 2003; Bentov and Erez, 2005, 2006). While this may explain the daily banding in planktic foraminifera that add a chamber every day, the banding phenomena overall are not well-understood. Furthermore, the effect of ocean acidification on the element banding is not known.
In this study, we measured the intra-shell variability of trace elements (B, Mg, Na, K, Sr, Ba, and U) in the two benthic foraminifera species Amphistegina lobifera and A. lessonii, cultured at four DIC concentrations (2,340, 2,420, 2,440, and 2,570 μM). These correspond to four pCO2 levels of 430, 560, 740, and 1,390 μatm. These two species are commonly found in coral-reef environments of the Gulf of Eilat, and as such they are an important component of the carbonate sediments in this marine environment (Reiss and Hottinger, 1984).
Materials and Methods
Two sediment-producing benthic foraminifera A. lobifera and A. lessonii were selected for this study. These two species are common in the Gulf of Eilat, and are an important component in carbonate sediment in this marine environment (Erez, 2003) particularly in the area of the coral reef. Both species produce high-Mg calcite shells at approximately 3–4 mol% MgCO3 (Erez, 2003). The foraminifera were collected from stones covered with turf algae in the Gulf of Eilat (Aqaba) at 3–5 m water depth. They were sieved and the 400–600 μm fraction of approximately 1400 live individuals of each species was used for the experiment. Initially the foraminifera were cultured in seawater with Calcein dye (Sigma-Aldrich, 40 μM/L) for 3 days and then washed with seawater (Eilat seawater) and divided to six groups (four experimental groups and two control groups). Both species were cultured in seawater sealed 120 ml Erlenmeyer flasks in four different DIC treatments. During the experiment (14 weeks) the Erlenmeyer flasks were kept at constant temperature of 25°C and light intensity of 60 μmol photons m–2 s–1, with a diurnal cycle of 12 h light. Once a week water was exchanged and sampled for oxygen, DIC, alkalinity, and pH measurements (methods and results will be reported in a separate publication). The salinity was constant at 40.6%, the normal salinity of Eilat seawater.
Water Preparation
Initially 20 liters of Eilat seawater was filtered throughout a 0.22-μm filter and spiked with 135Ba (Oak Ridge National Laboratory, United States) to reach a concentration of factor of ∼10 from seawater that unequivocally allows the identification of post-culture growth segments by LA-ICPMS (Laser Ablation Inductively Coupled Plasma Mass Spectrometry). Five liters were transferred to a CO2 bubbling system to prepare four pCO2 concentrations, 430, 560, 740, and 1390 ppm. The prepared waters were transferred to sealed bags (Supelco 30229-U) from which the actual experimental water were used for periodic water exchange (∼ once per week). The alkalinity, pH, oxygen and DIC of the reservoirs were measured four times during the experiment. During the experiment, the DIC in the sealed reservoirs increased in all bags (in part due to microbial oxidation of DOM with oxygen depletion). The alkalinity values remained constant and averaged 2411 ± 17 μmol kg–1 while the pH decreased. The exact values and their effect on the physiology (photosynthesis, calcification and respiration) of these foraminifera will be described elsewhere. The average DIC valued for the whole experiment are 2340 μmol (low DIC marked as L), 2420 μmol (intermediate low, IL), 2440 μmol (intermediate high, IH) and 2570 μmol (high, H). Here we report mainly on the shell chemistry recorded in the knob area of these foraminifera.
Sample Preparation and Analysis
At the end of the experiments, the cleaning process was done in two phases. The foraminifera from each treatment were washed five times with distilled water and dried in the oven (50°C, 24 h) then counted, weighted (total weight) and transferred to the organic matter cleaning procedure, by soaking the specimens in sodium hypochlorite (Sigma-Aldrich, 1:10) overnight while shaking gently. Specimens were washed five times again in distilled water, dried, and weighed again (shell weight).
LA-ICPMS
From each treatment, three foraminifers of each species were selected for laser ablation ICPMS analyses. We selected specimens of approximately equal shell size and close to the mean size of each treatment in order to limit the size dependency of the shell chemistry (Elderfield et al., 2002; Friedrich et al., 2012). In addition we examined their growth (the presence of several non-fluorescent chambers past the Calcein stain, see SI). Foraminifers were loosely fixed on top of Blu-Tak inside 1 inch stainless steel rings and analyzed using the RESOlution M-50 LA system coupled to an Agilent 8800 triple-quadrupole ICPMS at Royal Holloway University of London (Müller et al., 2009). The analytical method was optimized for the determination of trace elements via slow depth-profiling (for details see Griffiths et al., 2013 and Evans et al., 2015). Laser spot size of 74 μm for A. lessonii and 96 μm for A. lobifera with repetition rate of 2 Hz was used to ablate into the knob with an approximate vertical drill rate of ∼0.15 μm/pulse. To improve sensitivity, 6 ml min–1 H2 was used as an additional diatomic gas, added to He downstream of the LA cell. Each specimen was ablated once for 240 s, which reached an ablation depth of ∼50–70 μm (Figure 1).
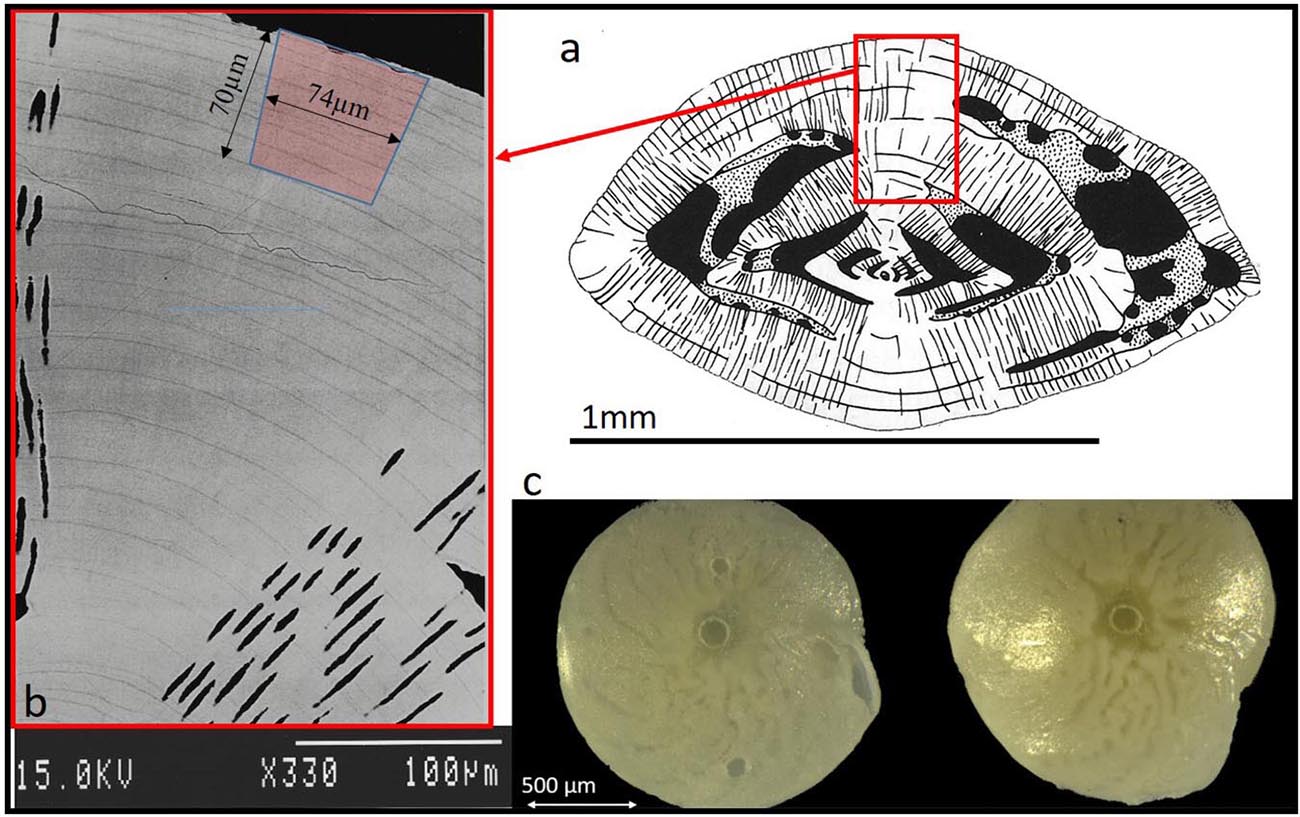
Figure 1. (a) Schematic diagram of Amphistegina lobifera vertical cross section (Adapted from Reiss and Hottinger, 1984). The knob is an imperforated structure build like multi layers concentric dome. (b) Back scatter SEM image of a polished vertical section through the knob of A. lobifera (see Erez, 2003), note the thin dark layers representing ∼weekly chamber growth lines. These dark layers are rich in Mg and K as well as other trace elements (see Figs below). The laser drilling marked in red is roughly 70 mm deep. (c) Images of the actual ablation hole in the knob of A. lessonii (left) and A. lobifera (right).
Data Analysis
Data reduction was done with Iolite software (Paton et al., 2011), trace element DRS method (Woodhead et al., 2007) with NIST SRM 612 (Jochum et al., 2011) as external standard. Data processing performed off-line using a commercial software package (MATLAB R2017a, The MathWorks Inc., Natick, MA, 2000).
Results
Growth of Foraminifera
All four groups showed a positive growth based both on weekly alkalinity depletions and on final weighing as follows: Groups L, IL, IH, H (2340, 2420, 2440, 2570 μM DIC) increased their CaCO3 weight by ∼100, ∼70, ∼78, and ∼30%, respectively. As mentioned previously, a detailed report on the calcification and symbionts photosynthesis will be reported elsewhere. Here we concentrate on the variability of the internal shell chemistry as observed mainly with the LA-ICPMS.
As shown in Figure 1, LA profiles with high vertical resolution of 0.15 mm in the solid CaCO3 of the knob area were performed. 135Ba/Ca ratios in the knob of A. lobifera and in A. lessonii were used as a chemical marker for the start of new shell deposition during the experiment (Figures 2, 3). The transition zone is very clear for most of the specimens and reach a final 135Ba/Ca value of ∼0.2 μmol/mol that is similar in both A. lobifera and A. lessonii. This value is roughly 10 times higher than the natural ratio for this isotope. The transition zone is ∼15–20 μm wide in A. lessonii and ∼5–10 μm in A. lobifera. The new skeleton thickness shows large variability between individuals of the same treatment (i.e,. DIC values) that mask to a large extent the variability between the treatments (see further details in the section “Discussion”).
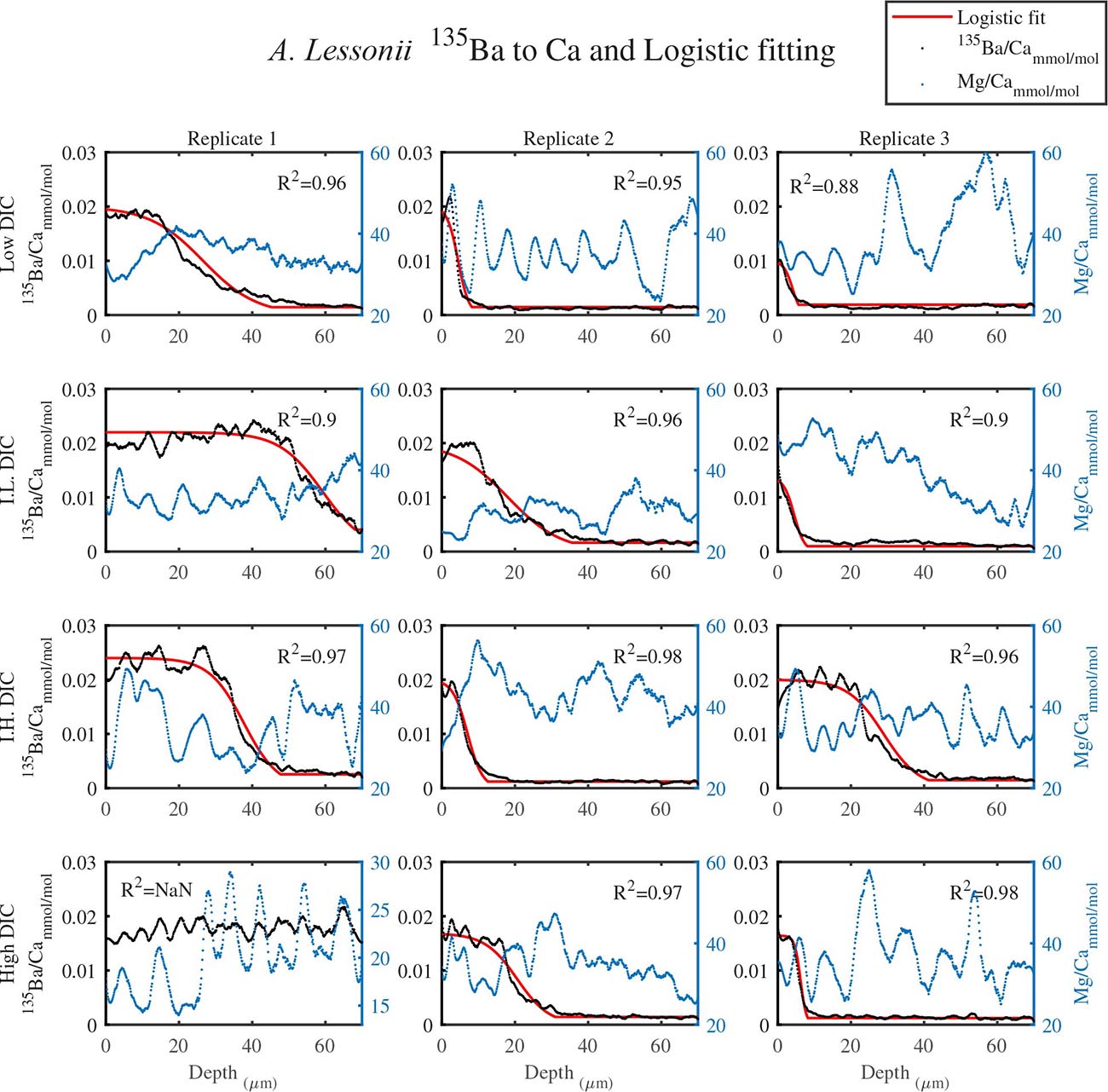
Figure 2. The transition zone of 135Ba/Ca from natural to experimental calcite as obtained from LA-ICPMS in the knob area of A. lessonii (dotted black). A logistic population function was fitted to the data (red solid line). The correlation coefficients of the fitted lines are very high (R2 > 0.9). The dotted blue line is the Mg/Ca ratio and the high peaks represent new chambers growth. Note that there is no correlation between the newly grown calcite (high 135Ba) and the experimental DIC conditions. Replicates L3 and IL3 stopped growing in the middle of the transition zone and replicate H1 shows only new growth.
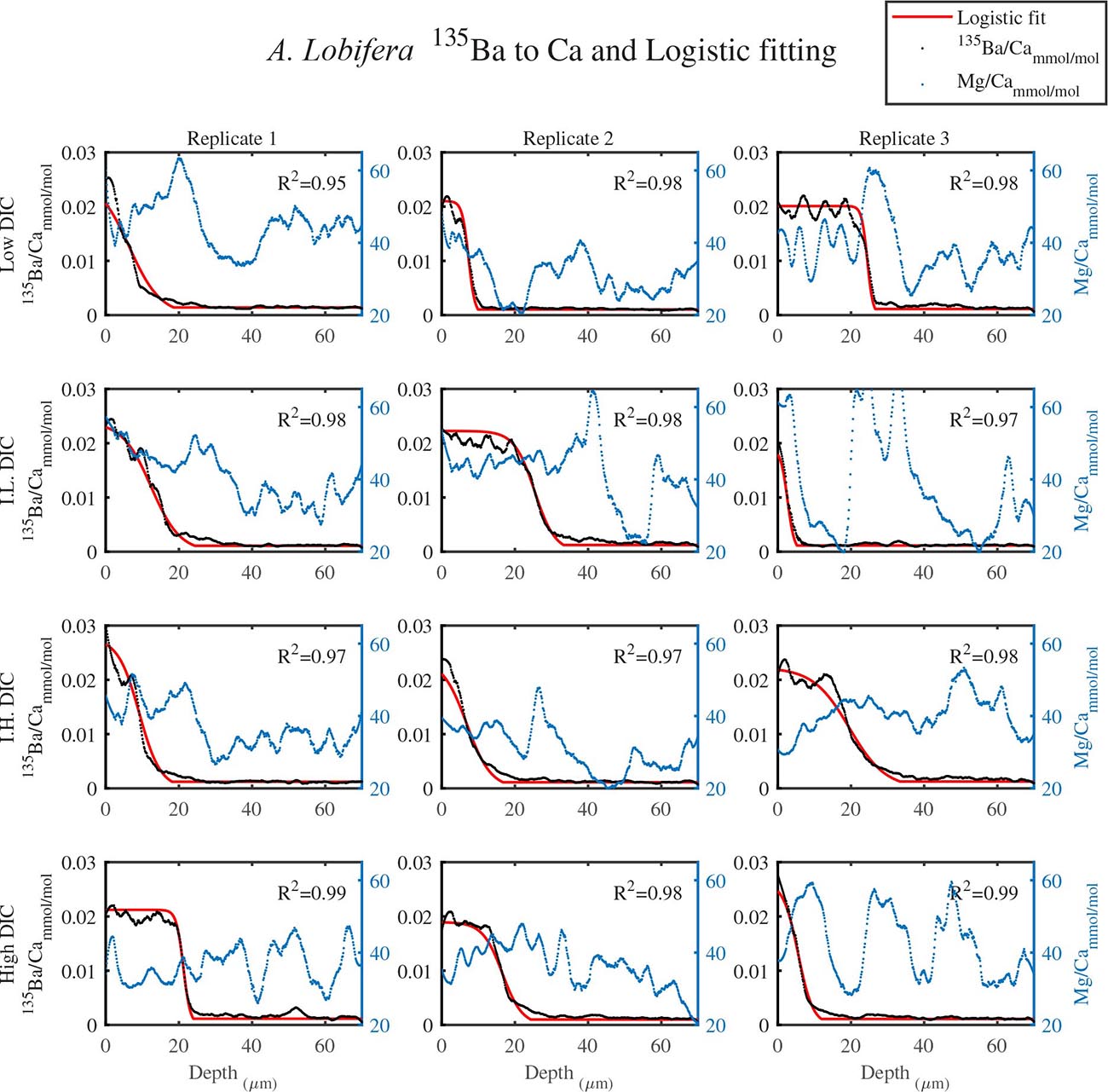
Figure 3. The transition zone of 135Ba/Ca from natural to experimental calcite as obtained from LA-ICPMS in the knob area of A. lobifera (dotted black). A logistic function was fitted to the data (red solid line). The correlation coefficients of the fitted lines are very high (R2 > 0.9). The dotted blue line is the Mg/Ca ratios and the high peaks represent new chambers growth. Note that there is no correlation between the newly grown calcite (high 135Ba) and the experimental DIC conditions.
Based on the identity of the newly grown shell we describe below the elemental cycles within the knob and the overall average ratios of several elements in the new and the natural shell.
Element to Ca Variability in the Knob Profiles at Different DIC
For all the specimens we observe the previously described high and low Mg bands in the knob area (Erez, 2003). Most of the profiles (∼70 μm in length) comprise 7–8 bands of Mg rich layers that equals to 60–70% of the last growth circular whorl that contain ∼11–12 chambers in these organisms. Every high-Mg band represents a new chamber and is followed by a low-Mg band (Figures 4, 5). There is no apparent changes in the Mg banding (frequency and phase) as a function of the DIC treatment for both species, i.e., the newly precipitated cycles show continuation of the natural cycles. Here again the variability within treatment as large as the variability between DIC treatments (Figures 4, 5). A. lobifera showed Mg/Ca range of 20–75 mmol/mol, and A. lessoni shows lower range of 14 to 60 mmol/mol. The Mg/Ca cycles were used as a template for comparison to the other elements. The K/Ca cycles throughout the knob are similar to those of the Mg/Ca, both frequency and phase regardless of the DIC treatment (Figure 4). The K/Ca values ranged from 0.45 to 0.35 mmol/mol in A. lobifera and 0.3 to 0.42 mmol/mol in A. lessoni. The Na/Ca (Figure 5) varied from 7 to 14 in A. lobifera and from 6 to 14 mmol/mol in A. lessoni showing a decrease from the old shell (high values) to the new part of the shell (low values), the cycles however, are similar to the Mg/Ca regardless of the DIC treatment. Sr/Ca show the same trend of decreasing values from 1.2 to 2.1 mmol/mol and 1.25 to 2.4 mmol/mol, respectively. The Sr/Ca show the same number of cycles of the Mg/Ca but with a phase shift of ∼1/4 cycle (Figure 6). The U/Ca cycles are very similar to those of the Mg both in frequency and in phase, but has a general trend of increase in the new shell in all treatments (Figure 5).
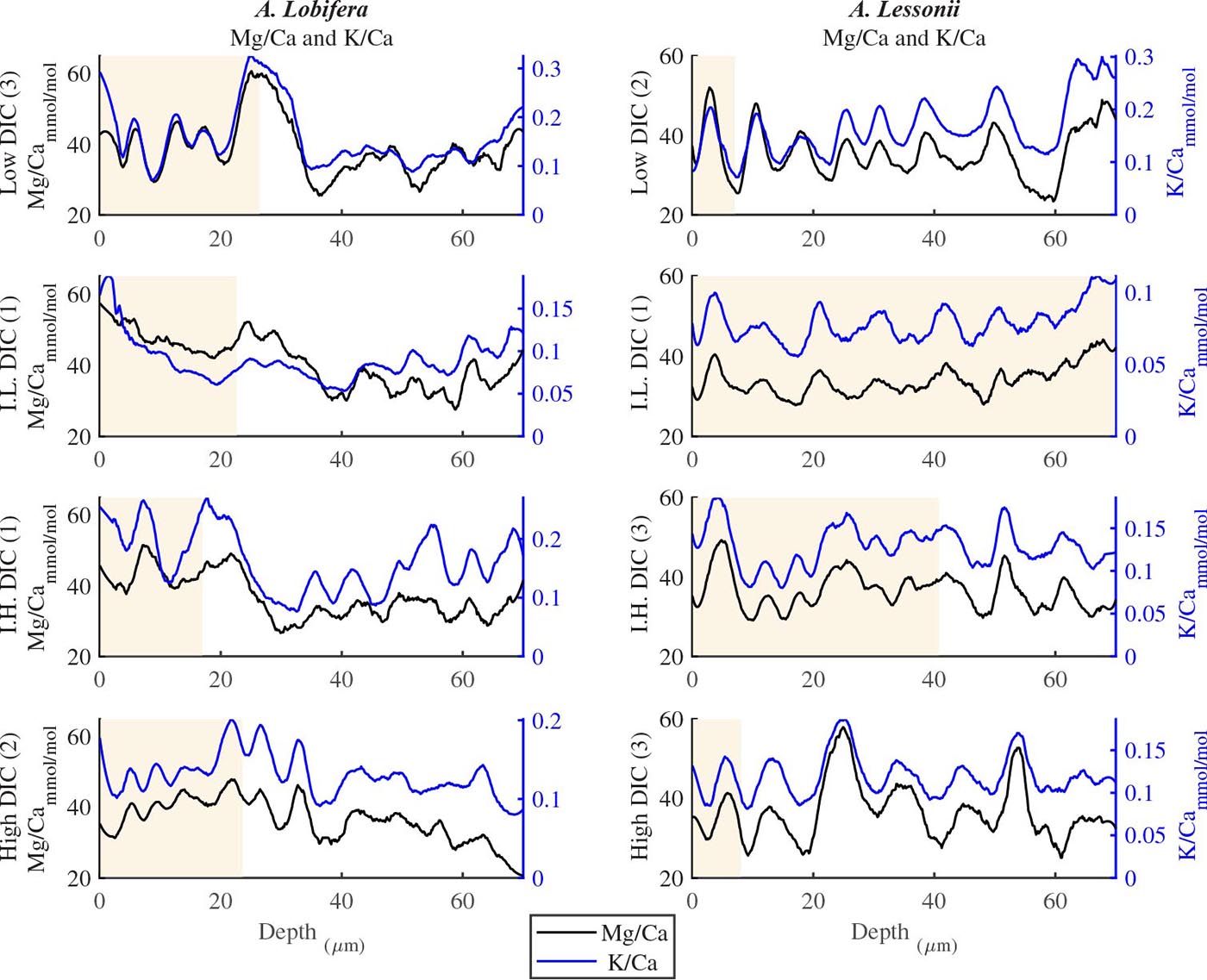
Figure 4. K/Ca and Mg/Ca ratios in the knob profile of A. lessonii and A. lobifera for one selected specimen from each DIC treatment (data for all the specimens are given in the Supplementary Material). Note that the K bands is following the Mg ones with high fidelity in both frequency and amplitude. These high Mg-K bands represent the dark layers shown in Fig 1b and are associated with the organic rich primary calcite while the thick low Mg-K bands represent the secondary calcite of the lamination process. The DIC treatment did not affect the elemental cycles.
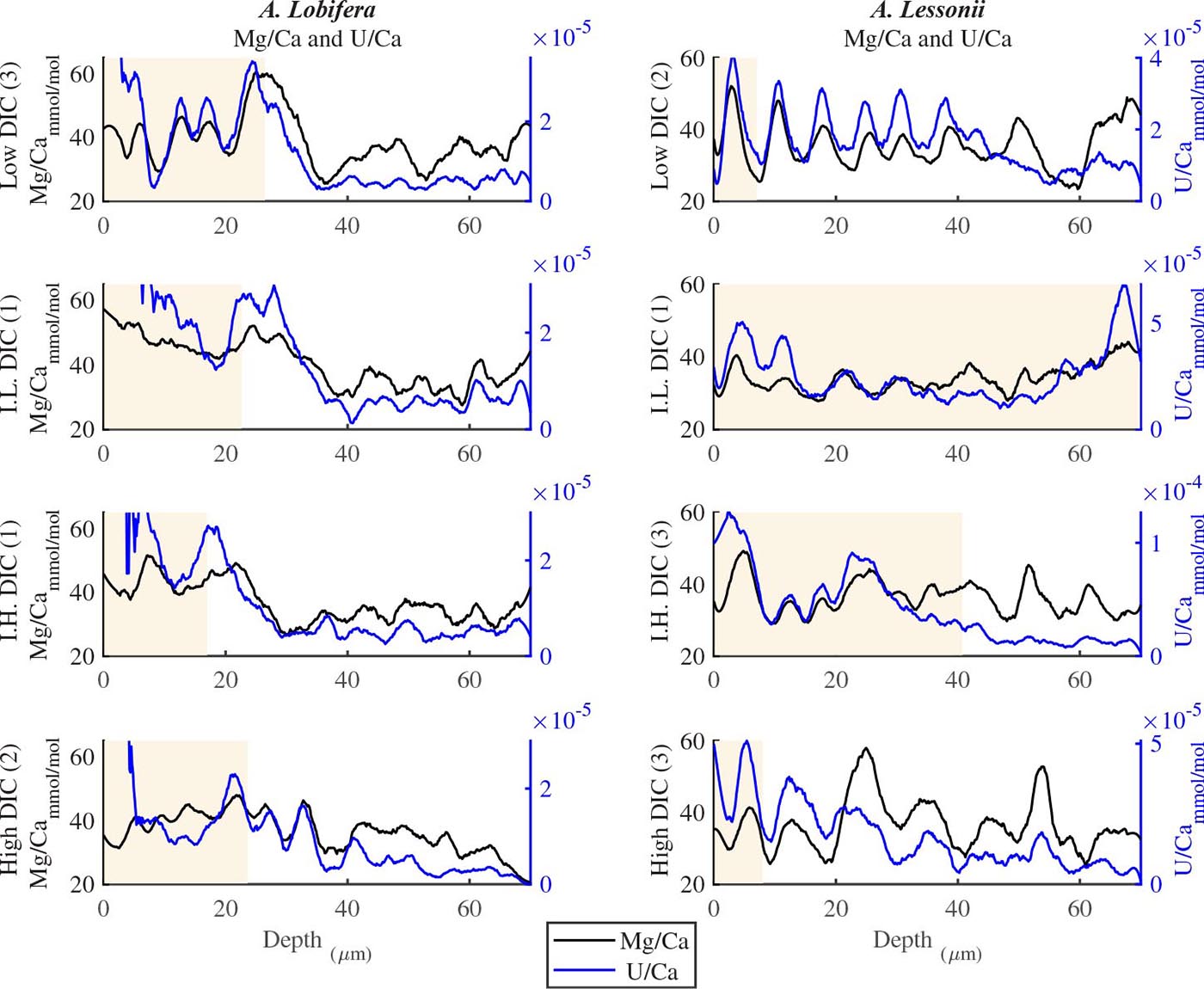
Figure 5. U/Ca and Mg/Ca ratios in the knob profile of A. lessonii and A. lobifera for one selected specimen for the four DIC treatments (all the data are given in the Supplementary Material). The two elements show similar cycles in both frequency and amplitude. In that respect, this is similar to the K/Ca ratio shown in Figure 4. The DIC treatment did not affect the elemental cycles.
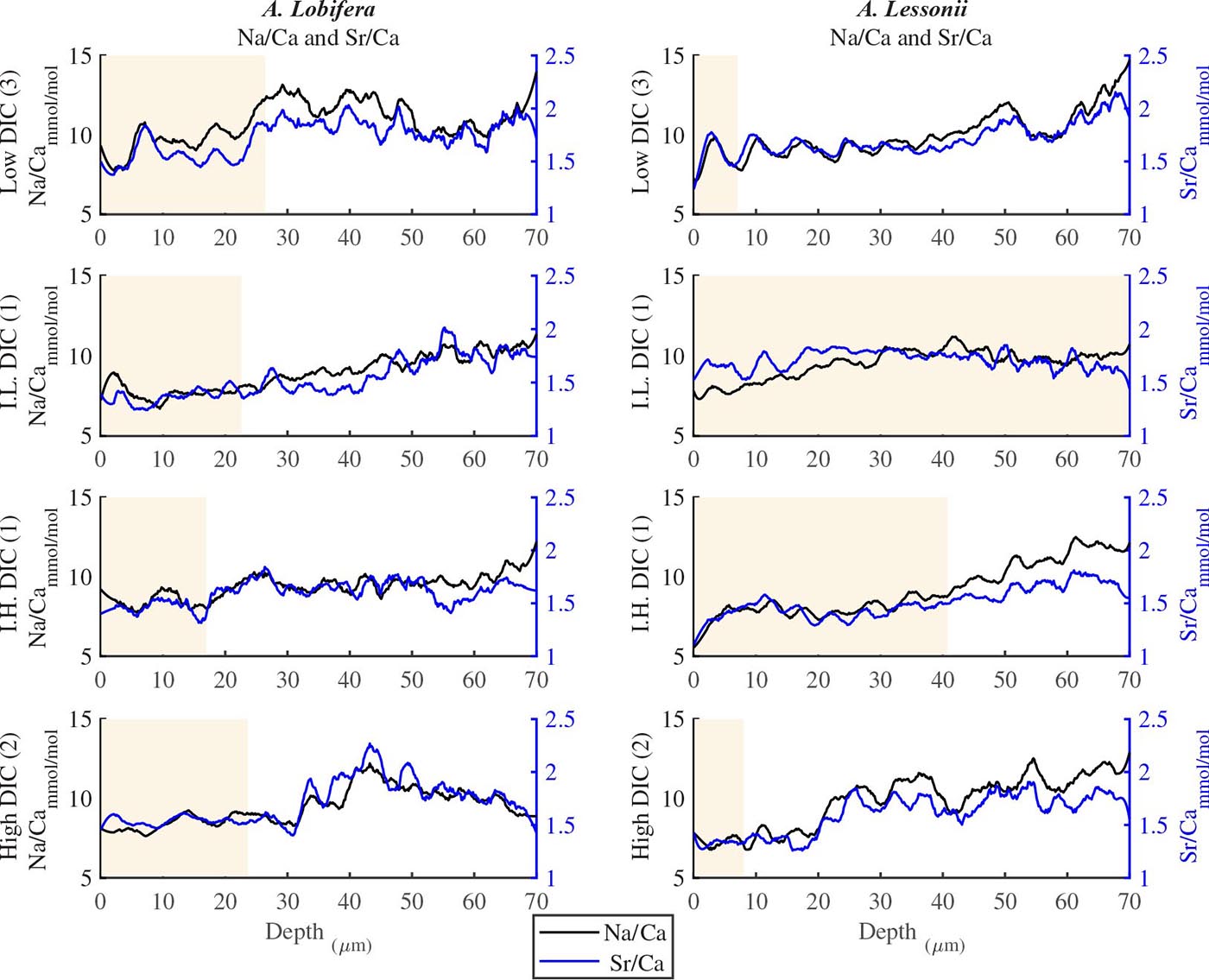
Figure 6. Na/Ca and Sr/Ca ratios in the knob profile of A. lessonii and A. lobifera for one selected specimen for each DIC treatments (all the data are given in the Supplementary Material). It should be noted that these two elements show less similarity to the Mg-K-U cycles shown in Figures 4, 5. On the whole, however, the number of cycles (i.e., the frequency) is similar to the those of Mg. The DIC treatment did not affect the elemental cycles.
Average Changes in Shell Composition at Different DIC
The average element to Ca ratios between the newly grown shell and the original natural shell are given in Table 1 and Figure 7 (A. lobifera) and 8 (A. lessoni). Significant differences between the new and natural shells were tested using Two Sample t-test (Table 2). Error bars in Figures 7, 8 represent ± the average standard deviation for the new and natural parts of the shell for each experimental DIC.
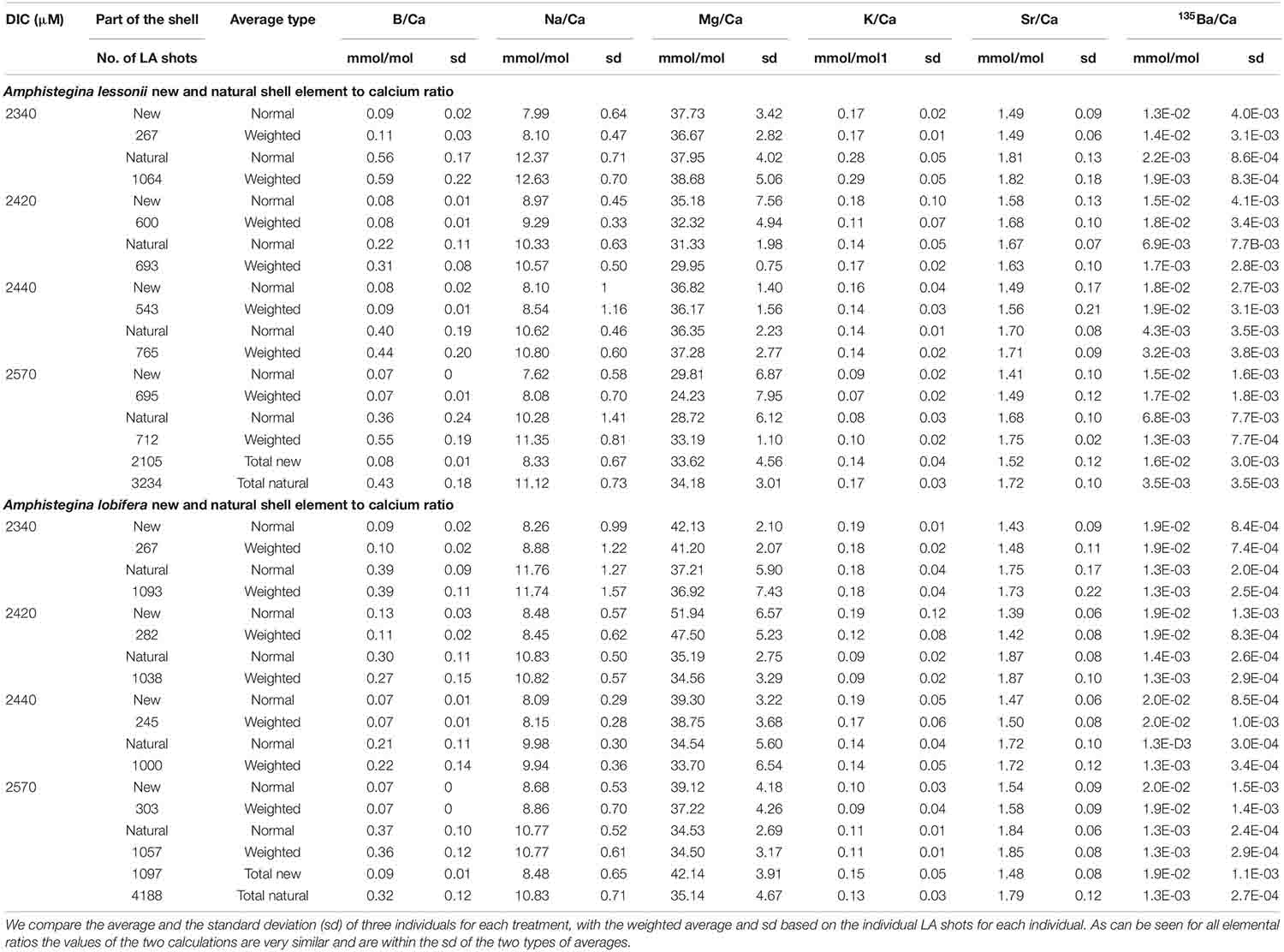
Table 1. Element to Ca ratios in the new shell precipitated under the experimental conditions and the natural original shell (Figures 7, 8).
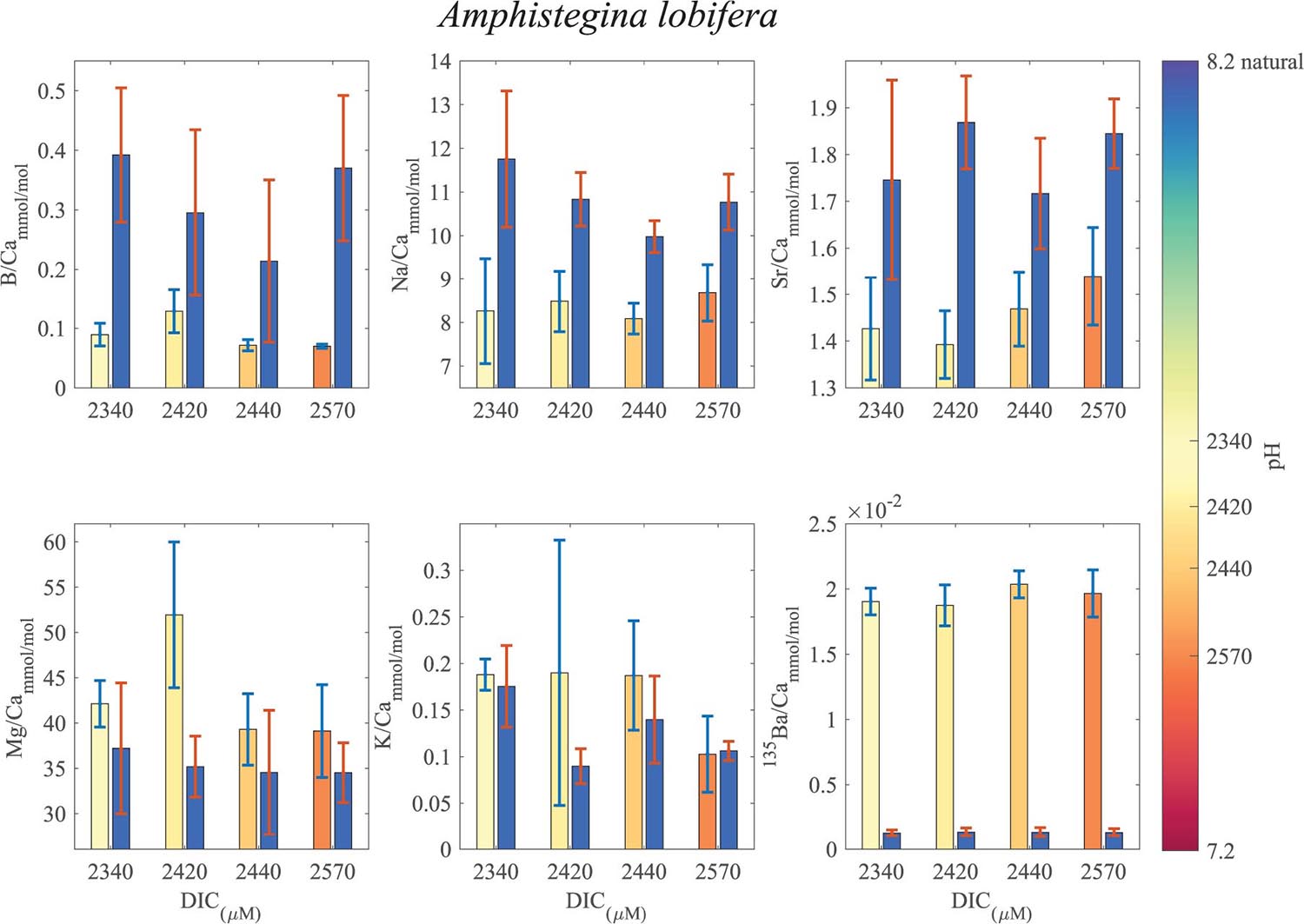
Figure 7. Averages of El/Ca ratios of the natural-original shells of Amphistegina lobifera in comparison with the newly grown shell under the DIC modified experimental conditions. For B/Ca, Na/Ca and Sr/Ca the new shell ratios are significantly lower than the original shell. For K/Ca and Mg/Ca the new shells show ratios that are significantly higher than the original shell (see Tables 1, 2 for statistics). 135Ba/Ca show the enrichment of the 135Ba that was added to the culture media to mark the newly precipitated calcite. The numbers on the colored pH bar represent the experimental average DIC measurements during the experiments.
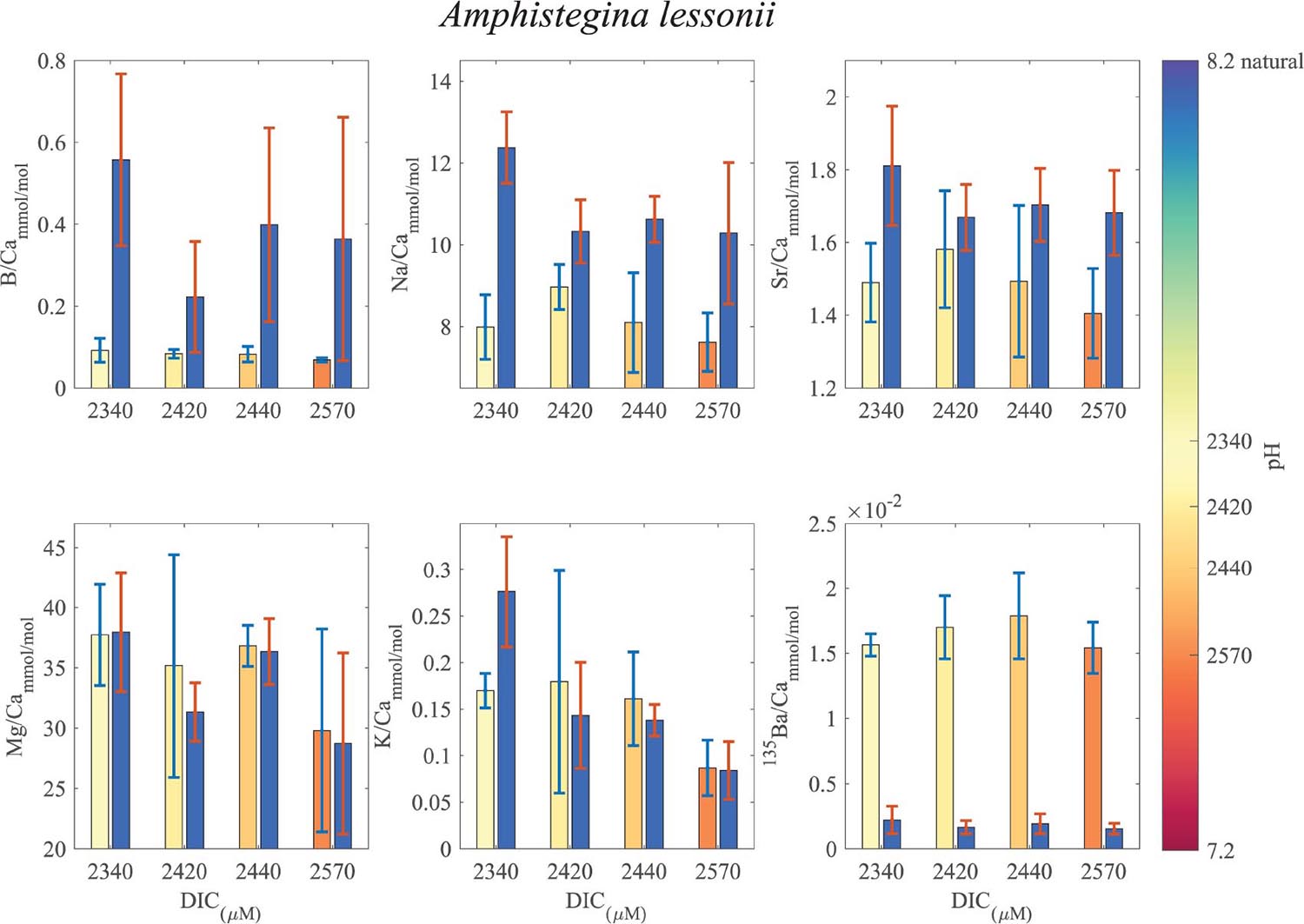
Figure 8. Averages of El/Ca ratios of the natural-original shells Amphistegina lessonii in comparison with the newly grown shell under the DIC modified experimental conditions. For B/Ca, Na/Ca and Sr/Ca the new shell ratios are significantly lower than the original shell. For K/Ca and Mg/Ca the new shells show ratios that are variable, slight lower or similar to the original shell (see Tables 1, 2 for statistics). 135Ba/Ca show the enrichment of the 135Ba that was added to the culture media to mark the newly precipitated calcite. The numbers on the colored pH bar represent the experimental average DIC measurements during the experiments.
B/Ca, Na/Ca and Sr/Ca in the new shell of A. lobifera are significantly lower than the natural shell. For Mg/Ca and K/Ca the new shells show ratios that are significantly higher than the natural shell (Figure 7 and Tables 1, 2). For A. lessoni B/Ca, Na/Ca and Sr/Ca in the new shell ratios are significantly lower than the natural shell. The Mg/Ca and K/Ca of the new shells show ratios that are variable, slightly lower or similar to the natural shell (Figure 8 and Tables 1, 2) thus not affected by the DIC increase.
Discussion
Transition Zone Dynamics
The increase of the 135Ba in the newly precipitated shell due to the spike in the experimental medium, give a marker for the start of the new growing phase. The part of the increase in the 135Ba concentration to a steady state value (i.e., transition zone) can be used to understand the calcification mechanism. The transition shape is rather uniform and can be fitted with a logistic equation (Verhulst, 1938) that investigates a standard model of population growth in a constrained environment.
In the case P is the 135Ba concentration at given time, “K” is the maximum concentration of the 135Ba (∼ 0.2 μM), “P0” is the concentration at time zero, “r” is the rate constant and “t” is time (or depth). This equation represents biological population growth, which approaches a steady state “K” (Table 3). The application of this formulation can be justified if we think about the population of the seawater vacuoles that supply the 135Ba to the calcification site. The first vacuoles containing the spike dilute with the previous older vacuoles and with time the number of the new vacuoles increases (and the old vacuoles decrease) until all the vacuoles contain the Ba spike. The maximum concentration in the skeleton is determined by the distribution coefficient of Ba in the foraminiferal calcite.
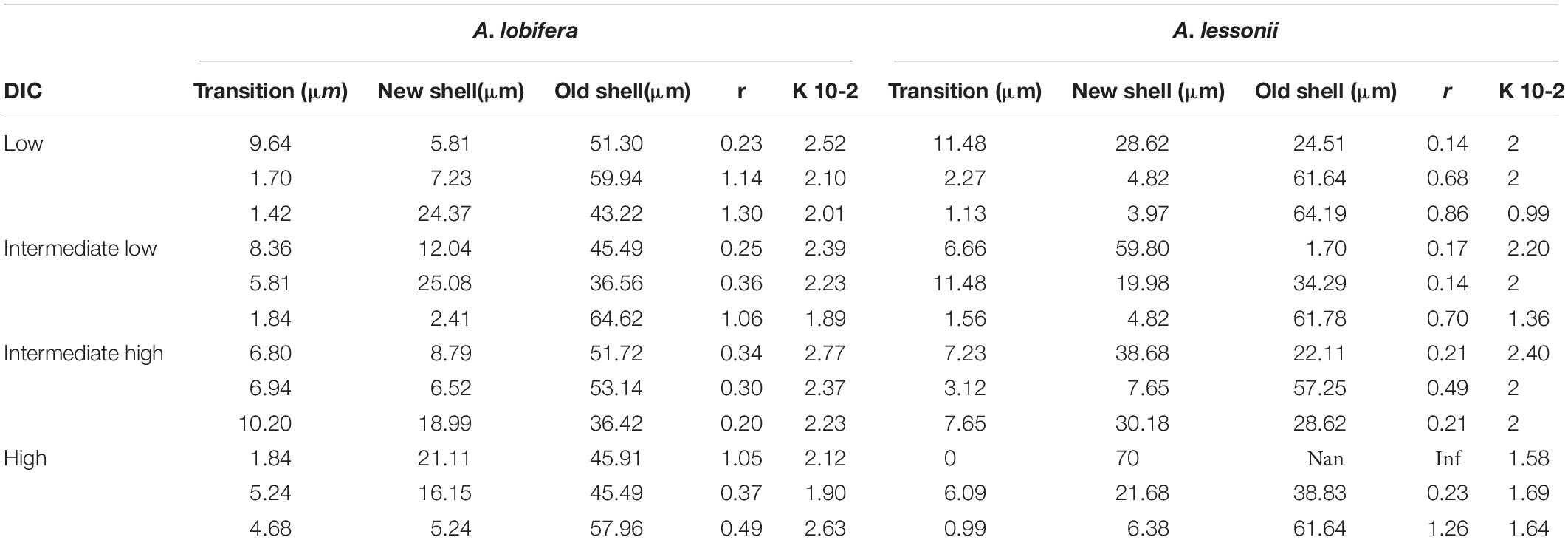
Table 3. Logistic equation coefficients for the uptake of 135Ba into the skeleton of A. lobifera and A. lessonii.
In Figures 2, 3 (red line) we show the best fit of the logistic function to the 135Ba data (black dots). The correlation coefficients are very high (all above 0.9), suggesting that the model indeed describes well the data, and this supports the seawater vacuolization mechanism proposed by Bentov et al., 2009. In this case the “t” Parameter is distance in μm between the natural 135Ba (P0) and the equilibrium values of the spike (K), obviously, this distance represents the time of saturation of the 135Ba pool, which we assume is equal to the complete replacement of the old seawater vacuoles with the new ones. If we assume that Ba is following Ca during the calcification process, this indicates a very large internal Ca pool roughly equivalent to the amount of Ca in a new chamber (and the lamination process associated with these lamellar species). Such internal Ca pools were described by Erez, 2003 for A. lobifera. Such large concentration (∼ a few Molar) of Ca in this pool is most probably a solid phase, most likely amorphous calcium carbonate (ACC).
There is no clear relationship between the DIC treatments and the thickness of the transition zone possibly because the variability between individuals in each treatment was as large as the variability between treatments. Similarly, there was no significant correlation between the thickness of the transition zone and the new skeleton thickness or the rate constant parameter “r” and the total growth (Figure 9).
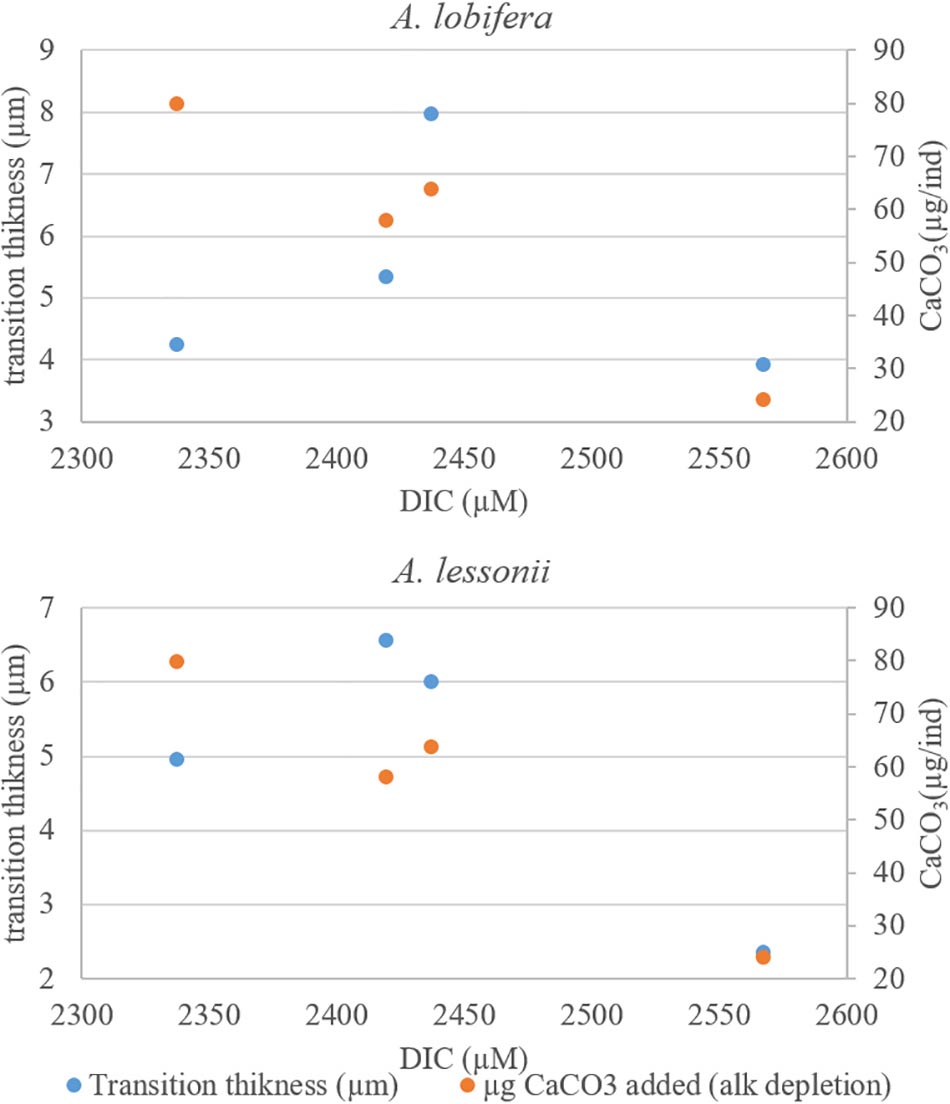
Figure 9. The transition zone thickness (based on 135Ba) as a function of DIC. The two intermediate DIC treatments yielded a thicker transition zone. There are no simple relations between the thickness of the transitions zones and the total shell growth (second Y axis) of these treatments.
Trace Element in the Knob Profiles and the Role DIC
The shell (within the knob) is composed of three parts: the natural growth (before collection) the pre experiment (short maintenance of the foraminifera before the experiment while being labeled with Calcein), and finally the part that grew during the experiment labeled with 135Ba.
The Mg banding in these species is different from that observed in the planktonic species (e.g., Eggins et al., 2003, 2004) mainly because A. lobifera and A. lessonii are involute species with a central knob (where the measurements were made) that accumulate skeleton for each new chamber (Figure 1). In addition, Ampnistegina (both species) have a long life cycle (of 6–12 months) as opposed to the short life cycle of the planktonic ones (∼30 days or less).
The Mg in this genus (as well as in other foraminifera) show growth cycles of Mg rich layers that have been described before (Eggins et al., 2003; Erez, 2003; Fehrenbacher et al., 2017; Geerken et al., 2018, 2019). The Mg cycles are observed both in the natural and in the new skeleton (Figures 2, 3, blue dots), regardless of the large variations in the DIC. Surprisingly, the elevated DIC concentrations did not have a significant effect on the patterns of the intrashell variability. This is supported by Geerken et al. (2019) showing that the high and low concentration bands are not effected by salinity and temperature. On the other hand, the averages of the measured trace elements to calcium ratios in the newly calcified shells did show some changes compared to the previous natural shells that may be related to the DIC treatment (see below, Figures 7, 8 and Table 1). Unlike the previous observations on A. lobifera (Erez, 2003), the Mg banding signal from the LA-ICPMS is less regular and the peaks are less sharp. This probably occurs because of three main reasons: (1) The growth lines in the foraminifera shells in the knob area have a topography and are only semi parallel, especially for large ablation area of 4300 μm2 used in this study. (2) The difference between the EPMA 1 μm3 interaction volume (Erez, 2003) and the LA-ICPMS ∼ 700 μm3 ablation volume per shot, which averages out and smooth the sharp peaks that seen by the EPMA. (3) The volume above the laser spot (the cup and tubing leading to the ICPMS) may causes some mixing between the layers and thus smooth out the original signal.
The element concentration between the old and new parts of the shells show trends that may be related to the elevated DIC. B/Ca, Na/Ca and Sr/Ca showing decrease with elevated DIC (Figures 2A–C) while K/Ca, Mg/Ca and showing slight increase in A. lobifera (Figures 2D,E) that are reported before for other Ampnistegina species (van Dijk et al., 2017b). In A. lessonii B/Ca, Na/Ca, and Sr/Ca also showing decrease with elevated DIC (Figures 3A–C) and in the K/Ca, Mg/Ca we not see any significant change (Figures 3D,E). The U showing increase with the elevated DIC in both Ampnistegina species but it is not significant because only three specimens per treatment was ablated and there are large variation between specimens inside each group. To address this element properly more work need to be done to gain more statistics on the influence of the DIC on U concentration.
The Effect of DIC on Intra-Shell Variability
While the intrashell variability in foraminifera is widely described both in planktonic and benthic foraminifera, the source of these variations is not well understood. Erez and Bentov suggested that these variations are alternations of primary and secondary calcite (Erez, 2003; Bentov and Erez, 2005, 2006; Geerken et al., 2018) associated with the buildup of every new chamber (Figure 10). The primary calcite is associated with the organic matrix (often termed as the Primary Organic Membrane, POM or Primary Organic Sheet, POS) that forms the shape or scaffold (also called the anlage) for the newly precipitating chamber. The primary calcite is composed of very small nuclei of trace elements-rich crystals that are formed on both sides of the organic matrix (Bentov and Erez, 2005). The secondary calcite represents the well-known process of layering (or lamination) in bilamellar foraminifera (Reiss, 1957, 1959). This involves precipitation of thick low Mg layers of flat semi-hexagonal radially (c-axis) oriented calcite crystals that cover the newly precipitated primary calcite and forms the radial structure all over the exposed chambers, leaving the pores open, in the last exposed whorl of chambers. As such, the layered shell is composed of alternations of primary organic and trace element rich calcite (Erez, 2003; Bentov and Erez, 2005, 2006; Bonnin et al., 2019) and secondary calcite that comprise more than 95% of the shell mass that is lower in organic and trace element content. Note however, that in addition to the primary and secondary calcites there is a third layer termed the inner lining, which precipitates, inside every chamber (Figure 10). This layer is not represented in the knob area (investigated here) and was recently well described for planktonic foraminifera (Fehrenbacher et al., 2017). The reasons for the trace elements enrichment in the primary calcite is not known; however, we speculate that it may result from the interactions with the organic matrix, as suggested also in a by Erez (2003),Geerken et al. (2019). Additionally precipitation of Vaterite and possibly ACC precursors of the primary calcite may be rich in trace elements (Jochum et al., 2011; Jacob et al., 2017). In the planktonic foraminifera that add a new chamber every day (Spero, 1987; Eggins et al., 2004; Fehrenbacher et al., 2017) we propose that the Mg rich primary calcite is precipitated during the night as indeed well documented by these authors. In the large benthic foraminifera, each growth of new chamber with all its layering is occurring at slower rates of roughly once a week to 10 days (age dependent). In the present study we found that in addition to Mg, S, and Na (Erez, 2003; Bentov and Erez, 2005, 2006; Fehrenbacher et al., 2017), there is also enrichment in K and Sr. Another possible explanation for the multi element high bands is that they represent precipitation from a semi-closed reservoir in which Ca is depleted relative to other ions (with distribution coefficient lower than 1) thus leading to an increase in their concentration relative to Ca (Elderfield et al., 1996). It is also possible that Mg is the key variable that changes its concentrations in the calcifying fluid and other elements are readily incorporated into the lattice due to the distortion of the crystal lattice (Mucci and Morse, 1983; Reeder et al., 1999). We cannot resolve with our data set the causes for these multi elemental cycles, but it should be mentioned that such cycles are also observed in other biomineralizing systems: clams (e.g,. Warter et al., 2018), corals (e.g., Vielzeuf et al., 2018) and others. Our observation that DIC did not affect the pattern of elemental cycles within the shells suggest that these variations are indeed an essential part of the calcification process that may be strongly biologically controlled and therefore are not influenced by the DIC. Recently Geerken et al. (2019) provided similar observations with respect to salinity and temperature. While the patterns of intrashell variability did not change, the average ratios of elemets to calcium did change in response to DIC (Figures 7, 8 and Table 1). Because our observations are based on very few individuals, we do feel that further mechanistic interpretation of these data is beyond the scope of this paper.
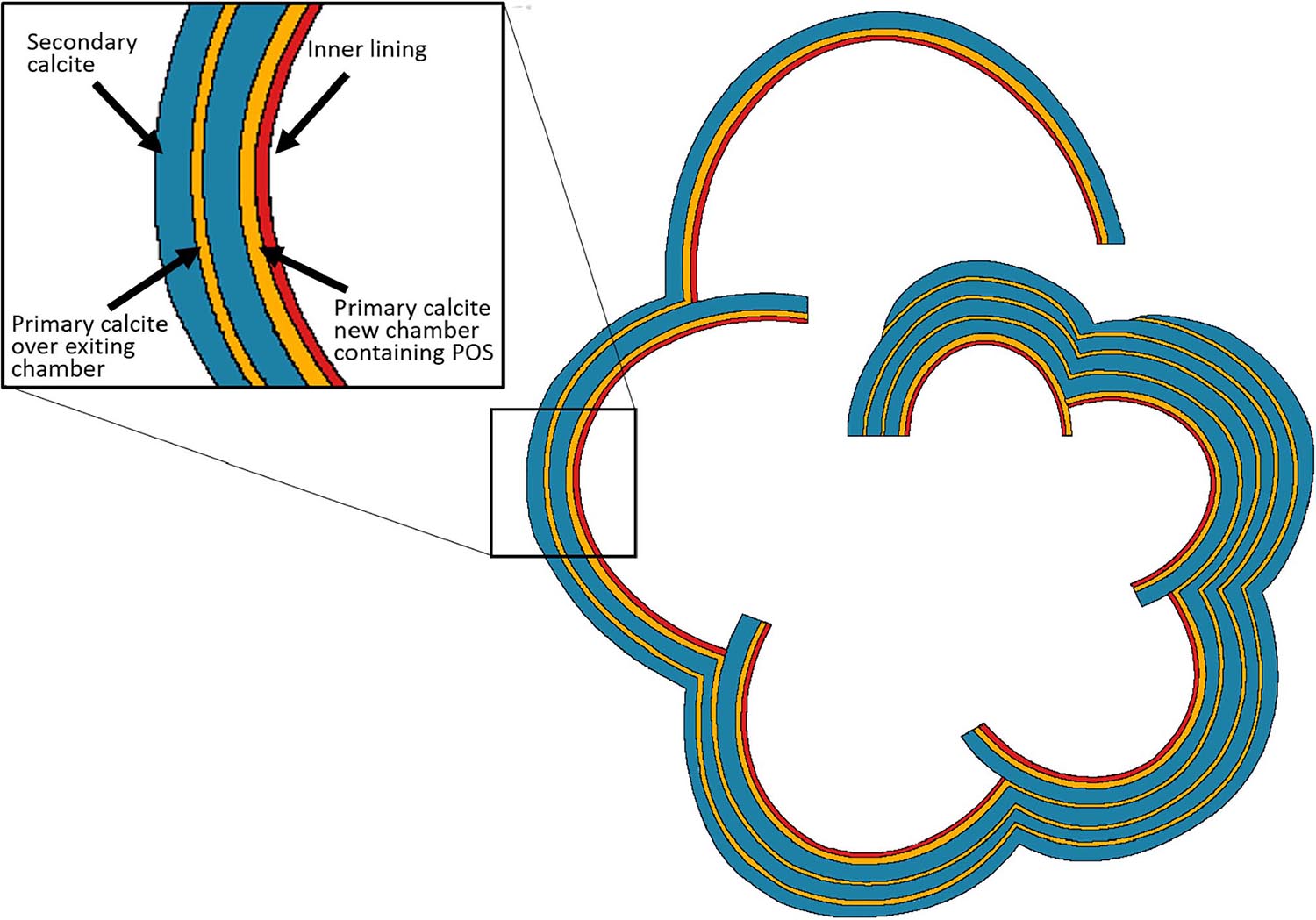
Figure 10. Schematic drawing of a horizontal section showing the layering structure in laminated, perforate (calcitic radial) foraminiferal shell. These layers are represented also in the knob area of the two involute species of the genus Amphistegina in this study. Every new chamber contains thin layer of primary calcite (yellow) which precipitates over the primary organic sheet (POS) and is rich in Mg and other elements. The primary calcite is followed by a thick layer of secondary calcite (blue). The secondary calcite has much lower concentrations of Mg and other trace elements, which show radial crystal arrangement, described as “calcitic radial.” While the mechanism of formation of the primary calcite is not well known for this genus, the secondary calcite is precipitated from alkaline seawater vacuoles (Bentov et al., 2009). Each chamber contains another thin layer of calcite, the inner lining (red) which may also have laminations (Fehrenbacher et al., 2017). This scheme was originally suggested by Reiss, 1957, 1959 and here was modified from that of Erez (2003).
Conclusion
Intra-shell chemical variability of the large benthic foraminifera A. lobifera and A. lessonii that were cultured under four high DIC concentrations (2340–2570 μM) was measured in the knob area using LA-ICPMS. The LA profiles (in three specimens for each treatment per species) represent the growth history of last whorl of chambers (∼10) in these two species. The knob displays alterations of Mg-rich and Mg-poor layers. The high Mg layers are also rich in Na, U and K while Sr shows similar variability but out of phase with the Mg. The high Mg layers are associated with the primary calcite while the low-Mg layers of the secondary calcite have lower concentrations of minor and trace elements. The alternations between the high and low element bands in Amphistegina represent new chamber growth at a rate of ∼ once a week for these larger specimens. Furthermore, there is no significant influence of the DIC (pCO2) changes on the alternation bands. On the other hand, the averages of some element to calcium ratios (B, Na, and Sr) are lower than the original shell at higher DIC while Mg and K are similar or slightly higher than the natural shell. 135Ba was added to the culture media to mark the newly precipitated calcite. The dynamics of 135Ba incorporation into the knob area is well describes using the logistic population growth function. We propose that this may represent the population of seawater vacuoles that bring the ions to the calcification site and are inherent to the biomineralization mechanism of these foraminifera. As for the intrashell cycles, we did not observe a correlation between the 135Ba dynamics and the DIC treatments.
Author Contributions
JE designed and directed the study. AL performed the growth experiments and analyzed the data. WM performed LA-ICPMS analysis and processing. AL, JE, and WM wrote the manuscript.
Funding
This work was supported by Israel Science Foundation grants ISF grants 551/10 and 790/16 to JE.
Conflict of Interest
The authors declare that the research was conducted in the absence of any commercial or financial relationships that could be construed as a potential conflict of interest.
Acknowledgments
We thank Dr. David Evans who helped with the analysis of the foraminifera using the LA-ICPMS. We thank the HUJI students Matan Yona, Hagar Hauzer, and Elana Palaria for their help in the field collections and the laboratory experiments.
Supplementary Material
The Supplementary Material for this article can be found online at: https://www.frontiersin.org/articles/10.3389/feart.2019.00247/full#supplementary-material
References
Allen, K. A., Hönisch, B., Eggins, S. M., Haynes, L. L., Rosenthal, Y., and Yu, J. (2016). Trace element proxies for surface ocean conditions: a synthesis of culture calibrations with planktic foraminifera. Geochim. Cosmochim. Acta 193, 197–221. doi: 10.1016/j.gca.2016.08.015
Barker, S., and Elderfield, H. (2002). Foraminiferal calcification response to glacial-interglacial changes in atmospheric Co2. Science 297, 833–836. doi: 10.1126/science.1072815
Bentov, S., Brownlee, C., and Erez, J. (2009). The role of seawater endocytosis in the biomineralization process in calcareous foraminifera. Proc. Natl. Acad. Sci. U.S.A. 106, 21500–21504. doi: 10.1073/pnas.0906636106
Bentov, S., and Erez, J. (2005). Novel observations on biomineralization processes in foraminifera and implications for Mg/Ca ratio in the shells. Geology 33, 841–844. doi: 10.1130/G21800.1
Bentov, S., and Erez, J. (2006). Impact of biomineralization processes on the Mg content of foraminiferal shells: a biological perspective. Geochem. Geophys. Geosyst. 7, 1010–1029. doi: 10.1029/2005GC001015
Bonnin, E. A., Zhu, Z., Fehrenbacher, J. S., Russell, A. D., Hönisch, B., Spero, H. J., et al. (2019). Submicron sodium banding in cultured planktic foraminifera shells. Geochim. Cosmochim. Acta 253, 127–141. doi: 10.1016/j.gca.2019.03.024
Branson, O., Kaczmarek, K., Redfern, S. A. T., Misra, S., Langer, G., Tyliszczak, T., et al. (2015). The coordination and distribution of B in foraminiferal calcite. Earth Planet. Sci. Lett. 416, 67–72. doi: 10.1016/j.epsl.2015.02.006
Davis, C. V., Fehrenbacher, J. S., Benitez-Nelson, C., and Thunell, R. C. (2020). Trace element heterogeneity across individual planktic foraminifera from the modern Cariaco basin. J. Foraminifer. Res. 50, 204–218. doi: 10.2113/gsjfr.50.2.204
de Nooijer, L. J., Spero, H. J., Erez, J., Bijma, J., and Reichart, G. J. (2014). Biomineralization in perforate foraminifera. Earth Sci. Rev. 135, 48–58. doi: 10.1016/j.earscirev.2014.03.013
de Nooijer, L. J., Toyofuku, T., and Kitazato, H. (2009). Foraminifera promote calcification by elevating their intracellular pH. Proc. Natl. Acad. Sci. U.S.A. 106, 15374–15378. doi: 10.1073/pnas.0904306106
Dias, B. B., Hart, M. B., Smart, C. W., and Hall-Spencer, J. M. (2010). Modern seawater acidification: the response of foraminifera to high-CO2 conditions in the Mediterranean Sea. J. Geol. Soc. Lond. 167, 843–846. doi: 10.1144/0016-76492010-050
Dissard, D., Nehrke, G., Reichart, G. J., and Bijma, J. (2010). The impact of salinity on the Mg/Ca and Sr/Ca ratio in the benthic foraminifera Ammonia tepida: results from culture experiments. Geochim. Cosmochim. Acta 74, 928–940. doi: 10.1016/J.GCA.2009.10.040
Eggins, S., De Deckker, P., and Marshall, J. (2003). Mg/Ca variation in planktonic foraminifera tests: implications for reconstructing -palaeo-seawater temperature and habitat migration. Earth Planet. Sci. Lett. 212, 291–306. doi: 10.1016/S0012-821X(03)00283-8
Eggins, S. M., Sadekov, A., and De Deckker, P. (2004). Modulation and daily banding of Mg/Ca in Orbulina universa tests by symbiont photosynthesis and respiration: a complication for seawater thermometry? Earth Planet. Sci. Lett. 225, 411–419. doi: 10.1016/j.epsl.2004.06.019
Elderfield, H., Bertram, C. J., and Erez, J. (1996). A biomineralization model for the incorporation of trace elements into foraminiferal calciumcarbonate. Earth Planet. Sci. Lett. 142, 409–423. doi: 10.1016/0012-821x(96)00105-7
Elderfield, H., Vautravers, M., and Cooper, M. (2002). The relationship between shell size and Mg/Ca, Sr/Ca, δ 18 O, and δ 13 C of species of planktonic foraminifera. Geochem. Geophys. Geosyst. 3, 1–13. doi: 10.1029/2001GC000194
Emiliani, C., and Shackleton, N. J. (1974). The Brunhes epoch: isotopic paleotemperatures and geochronology. Science 183, 511–514. doi: 10.1126/science.183.4124.511
Erez, J. (1978). Vital effect on stable-isotope composition seen in foraminifera and coral skeletons. Nature 273, 199–202. doi: 10.1038/273199a0
Erez, J. (2003). The source of ions for biomineralization in Foraminifera and their implications for paleoceanographic proxies. Rev. Mineral. Geochem. 54, 115–149. doi: 10.2113/0540115
Evans, D., Erez, J., Oron, S., and Müller, W. (2015). Mg/Ca-temperature and seawater-test chemistry relationships in the shallow-dwelling large benthic foraminifera Operculina ammonoides. Geochim. Cosmochim. Acta 148, 325–342. doi: 10.1016/j.gca.2014.09.039
Evans, D., Müller, W., and Erez, J. (2018). Assessing foraminifera biomineralisation models through trace element data of cultures under variable seawater chemistry. Geochim. Cosmochim. Acta 236, 198–217. doi: 10.1016/j.gca.2018.02.048
Evans, D., Wade, B. S., Henehan, M., Erez, J., and Müller, W. (2016). Revisiting carbonate chemistry controls on planktic foraminifera Mg/Ca: implications for sea surface temperature and hydrology shifts over the Paleocene-Eocene Thermal Maximum and Eocene-Oligocene transition. Clim. Past 12, 819–835. doi: 10.5194/cp-12-819-2016
Evans, D., and Müller, W. (2018). Automated extraction of a five-year LA-ICP-MS trace element data set of ten common glass and carbonate reference materials: long-term data quality, optimisation and laser cell homogeneity. Geostand. Geoanal. Res. 42, 159–188. doi: 10.1111/ggr.12204
Fehrenbacher, J. S., Russell, A. D., Davis, C. V., Gagnon, A. C., Spero, H. J., Cliff, J. B., et al. (2017). Link between light-triggered Mg-banding and chamber formation in the planktic foraminifera Neogloboquadrina dutertrei. Nat. Commun. 8:15441. doi: 10.1038/ncomms15441
Foster, G. L., and Rae, J. W. B. (2016). Reconstructing ocean pH with boron isotopes in foraminifera. Annu. Rev. Earth Planet. Sci. 44, 207–237. doi: 10.1146/annurev-earth-060115-012226
Friedrich, O., Schiebel, R., Wilson, P. A., Weldeab, S., Beer, C. J., Cooper, M. J., et al. (2012). Influence of test size, water depth, and ecology on Mg/Ca, Sr/Ca, δ 18O and δ 13C in nine modern species of planktic foraminifers. Earth Planet. Sci. Lett. 319-320, 133–145. doi: 10.1016/j.epsl.2011.12.002
Fujita, K., Hikami, M., Suzuki, A., Kuroyanagi, A., Sakai, K., Kawahata, H., et al. (2011). Effects of ocean acidification on calcification of symbiont-bearing reef foraminifers. Biogeosciences 8, 2089–2098. doi: 10.5194/bg-8-2089-2011
Geerken, E., de Nooijer, L. J., Roepert, A., Polerecky, L., King, H. E., and Reichart, G. J. (2019). Element banding and organic linings within chamber walls of two benthic foraminifera. Sci. Rep. 9:3598. doi: 10.1038/s41598-019-40298-y
Geerken, E., Jan de Nooijer, L., van DIjk, I., and Reichart, G. J. (2018). Impact of salinity on element incorporation in two benthic foraminiferal species with contrasting magnesium contents. Biogeosciences 15, 2205–2218. doi: 10.5194/bg-15-2205-18
Gray, W. R., and Evans, D. (2019). Nonthermal influences on Mg/Ca in planktonic foraminifera: a review of culture studies and application to the last glacial maximum. Paleoceanogr. Paleoclimatol. 34, 306–315. doi: 10.1029/2018PA003517
Griffiths, N., Müller, W., Johnson, K. G., and Aguilera, O. A. (2013). Evaluation of the effect of diagenetic cements on element/Ca ratios in aragonitic Early Miocene (~16Ma) Caribbean corals: implications for “deep-time” palaeo-environmental reconstructions. Palaeogeogr. Palaeoclimatol. Palaeoecol. 369, 185–200. doi: 10.1016/j.palaeo.2012.10.018
Gussone, N., Filipsson, H. L., and Kuhnert, H. (2016). Mg/Ca, Sr/Ca and Ca isotope ratios in benthonic foraminifers related to test structure, mineralogy and environmental controls. Geochim. Cosmochim. Acta 173, 142–159. doi: 10.1016/j.gca.2015.10.018
Hall, J. M., and Chan, L. H. (2004). Li/Ca in multiple species of benthic and planktonic foraminifera: thermocline, latitudinal, and glacial-interglacial variation. Geochim. Cosmochim. Acta 68, 529–545. doi: 10.1016/S0016-7037(00)00451-4
Hathorne, E. C., James, R. H., and Lampitt, R. S. (2009). Environmental versus biomineralization controls on the intratest variation in the trace element composition of the planktonic foraminifera G. inflata and G. scitula. Paleoceanography 24, 1–14. doi: 10.1029/2009PA001742
Hauzer, H., Evans, D., Müller, W., Rosenthal, Y., and Erez, J. (2018). Calibration of Na partitioning in the calcitic foraminifer Operculina ammonoides under variable Ca concentration: toward reconstructing past seawater composition. Earth Planet. Sci. Lett. 497, 80–91. doi: 10.1016/j.epsl.2018.06.004
Holland, K., Eggins, S. M., Hönisch, B., Haynes, L. L., and Branson, O. (2017). Calcification rate and shell chemistry response of the planktic foraminifer Orbulina universa to changes in microenvironment seawater carbonate chemistry. Earth Planet. Sci. Lett. 464, 124–134. doi: 10.1016/j.epsl.2017.02.018
Hori, M., Shirai, K., Kimoto, K., Kurasawa, A., Takagi, H., Ishida, A., et al. (2018). Chamber formation and trace element distribution in the calcite walls of laboratory cultured planktonic foraminifera (Globigerina bulloides and Globigerinoides ruber). Mar. Micropaleontol. 140, 46–55. doi: 10.1016/j.marmicro.2017.12.004
Jacob, D. E., Wirth, R., Agbaje, O. B. A., Branson, O., and Eggins, S. M. (2017). Planktic foraminifera form their shells via metastable carbonate phases. Nat. Commun. 8, 1–8. doi: 10.1038/s41467-017-00955-0
Jochum, K. P., Weis, U., Stoll, B., Kuzmin, D., Yang, Q., Raczek, I., et al. (2011). Determination of reference values for NIST SRM 610-617 glasses following ISO guidelines. Geostand. Geoanal. Res. 35, 397–429. doi: 10.1111/j.1751-908X.2011.00120.x
Jonkers, L., Buse, B., Brummer, G. J. A., and Hall, I. R. (2016). Chamber formation leads to Mg/Ca banding in the planktonic foraminifer Neogloboquadrina pachyderma. Earth Planet. Sci. Lett. 451, 177–184. doi: 10.1016/j.epsl.2016.07.030
Katz, M. E., Cramer, B. S., Franzese, A., Hönisch, B., Miller, K. G., Rosenthal, Y., et al. (2010). Traditional and emerging geochemical proxies in foraminifera. J. Foraminifer. Res. 40, 165–192. doi: 10.2113/gsjfr.40.2.165
Keul, N., Langer, G., De Nooijer, L. J., Nehrke, G., Reichart, G. J., and Bijma, J. (2013). Incorporation of uranium in benthic foraminiferal calcite reflects seawater carbonate ion concentration. Geochem. Geophys. Geosyst. 14, 102–111. doi: 10.1029/2012GC004330
Keul, N., Langer, G., Thoms, S., de Nooijer, L. J., Reichart, G. J., and Bijma, J. (2017). Exploring foraminiferal Sr/Ca as a new carbonate system proxy. Geochim. Cosmochim. Acta 202, 374–386. doi: 10.1016/j.gca.2016.11.022
Kisakürek, B., Eisenhauer, A., Böhm, F., Garbe-Schönberg, D., and Erez, J. (2008). Controls on shell Mg/Ca and Sr/Ca in cultured planktonic foraminiferan, Globigerinoides ruber (white). Earth Planet. Sci. Lett. 273, 260–269. doi: 10.1016/j.epsl.2008.06.026
Kuroyanagi, A., Kawahata, H., Suzuki, A., Fujita, K., and Irie, T. (2009). Impacts of ocean acidification on large benthic foraminifers: results from laboratory experiments. Mar. Micropaleontol. 73, 190–195. doi: 10.1016/j.marmicro.2009.09.003
Lea, D. W. (1999). “Trace elements in foraminiferal calcite,” in Modern Foraminifera, (Dordrecht: Springer Netherlands), 259–277. doi: 10.1007/0-306-48104-9_15
Lea, D., and Boyle, E. (1989). Barium content of benthic foraminifera controlled by bottom-water composition. Nature 338, 751–753. doi: 10.1038/338751a0
Lear, C. H., Rosenthal, Y., and Slowey, N. (2002). Benthic foraminiferal Mg/Ca-paleothermometry: a revised core-top calibration. Geochim. Cosmochim. Acta 66, 3375–3387. doi: 10.1016/S0016-7037(02)00941-9
Lear, C. H., Rosenthal, Y., and Wright, J. D. (2003). The closing of a seaway: ocean water masses and global climate change. Earth Planet. Sci. Lett. 210, 425–436. doi: 10.1016/S0012-821X(03)00164-X
McIntyre-Wressnig, A., Bernhard, J. M., McCorkle, D. C., and Hallock, P. (2013). Non-lethal effects of ocean acidification on the symbiont-bearing benthic foraminifer Amphistegina gibbosa. Mar. Ecol. Prog. Ser. 472, 45–60. doi: 10.3354/meps09918
Mucci, A., and Morse, J. W. (1983). The incorporation of Mg2+ and Sr2+ into calcite overgrowths: influences of growth rate and solution composition. Geochim. Cosmochim. Acta 47, 217–233. doi: 10.1016/0016-7037(83)90135-7
Müller, W., Shelley, M., Miller, P., and Broude, S. (2009). Initial performance metrics of a new custom-designed ArF excimer LA-ICPMS system coupled to a two-volume laser-ablation cell. J. Anal. At. Spectrom. 24, 209–214. doi: 10.1039/b805995k
Nehrke, G., Keul, N., Langer, G., de Nooijer, L. J., Bijma, J., and Meibom, A. (2013). A new model for biomineralization and trace-element signatures of Foraminifera tests. Biogeosciences 10, 6759–6767. doi: 10.5194/bg-10-6759-2013
Nürnberg, D., Bijma, J., and Hemleben, C. (1996). Assessing the reliability of magnesium in foraminiferal calcite as a proxy for water mass temperatures. Geochim. Cosmochim. Acta 60, 803–814. doi: 10.1016/0016-7037(95)00446-7
Nürnberg, D. (2000). Taking the temperature of past ocean surfaces. Science 289, 1698–1699. doi: 10.1126/science.289.5485.1698
Paton, C., Hellstrom, J., Paul, B., Woodhead, J., and Hergt, J. (2011). Iolite: freeware for the visualisation and processing of mass spectrometric data. J. Anal. At. Spectrom. 26, 2508–2518. doi: 10.1039/C1JA10172B
Raitzsch, M., Hathorne, E. C., Kuhnert, H., Groeneveld, J., and Bickert, T. (2011a). Modern and late pleistocene B/Ca ratios of the benthic foraminifer Planulina wuellerstorfidetermined with laser ablation ICP-MS. Geology 39, 1039–1042. doi: 10.1130/G32009.1
Raitzsch, M., Kuhnert, H., Hathorne, E. C., Groeneveld, J., and Bickert, T. (2011b). U/Ca in benthic foraminifers: a proxy for the deep-sea carbonate saturation. Geochem. Geophys. Geosys. 12, 1–12. doi: 10.1029/2010GC003344
Rathmann, S., Hess, S., Kuhnert, H., and Mulitza, S. (2004). Mg/Ca ratios of the benthic foraminifera Oridorsalis umbonatus obtained by laser ablation from core top sediments: relationship to bottom water temperature. Geochem. Geophys. Geosyst. 5, 1–10. doi: 10.1029/2004GC000808
Reeder, R. J., Lamble, G. M., and Northrup, P. A. (1999). XAFS study of the coordination and local relaxation around Co2+, Zn2+, Pb2+, and Ba2+trace elements in calcite. Am. Mineral. 84, 1049–1060. doi: 10.2138/am-1999-7-807
Reichart, G. J., Jorissen, F., Anschutz, P., and Mason, P. R. D. (2003). Single foraminiferal test chemistry records the marine environment. Geology 31, 355–358.
Reiss, Z. (1957). The bilamellidea, nov. superfamily, and remarks on cretaceous globorotaliids. Contrib. Cushman Found. Foraminifer. Res. 8, 127–145.
Reiss, Z. (1959). The wall-structure of Cibicides, Planulina, Gyroidinoides, and Globorotalites. Micropaleontology 5, 355–357.
Reiss, Z., and Hottinger, L. (1984). The Gulf of Aqaba: Ecological Micropaleontology. Berlin: Springer Science & Business Media. doi: 10.1007/978-3-642-69787-6
Rollion-Bard, C., Erez, J., and Zilberman, T. (2008). Intra-shell oxygen isotope ratios in the benthic foraminifera genus Amphistegina and the influence of seawater carbonate chemistry and temperature on this ratio. Geochim. Cosmochim. Acta 72, 6006–6014. doi: 10.1016/J.GCA.2008.09.013
Rosenthal, Y., Boyle, E. A., and Slowey, N. (1997). Temperature control on the incorporation of magnesium, strontium, fluorine, and cadmium into benthic foraminiferal shells from little bahama bank: prospects for thermocline paleoceanography. Geochim. Cosmochim. Acta 61, 3633–3643. doi: 10.1016/S0016-7037(97)00181-6
Rosenthal, Y., Lear, C. H., Oppo, D. W., and Linsley, B. K. (2006). Temperature and carbonate ion effects on Mg/Ca and Sr/Ca ratios in benthic foraminifera: Aragonitic species Hoeglundina elegans. Paleoceanography 21:A1007. doi: 10.1029/2005PA001158
Russell, A. D., Hönisch, B., Spero, H. J., and Lea, D. W. (2004). Effects of seawater carbonate ion concentration and temperature on shell U, Mg, and Sr in cultured planktonic foraminifera. Geochim. Cosmochim. Acta 68, 4347–4361. doi: 10.1016/j.gca.2004.03.013
Sanyal, A., Hemming, N. G., Broecker, W. S., Lea, D. W., Spero, H. J., and Hanson, G. N. (1996). Oceanic pH control on the boron isotopic composition of foraminifera: evidence from culture experiments. Paleoceanography 11, 513–517. doi: 10.1029/96PA01858
Spero, H. J. (1987). Symbiosis in the Planktonic Foraminifer, orbulina universa, and the isolation of its symbiotic Dinoflagellate, Gymnodinium Béii Sp. Nov. J. Phycol. 23, 307–317. doi: 10.1111/j.1529-8817.1987.tb04139.x
Spero, H. J., Bijma, J., Lea, D. W., Bemis, B. E., and Bernis, B. E. (1997). Effect of seawater carbonate concentration on foraminiferal carbon and oxygen isotopes. Nature 390, 497–500. doi: 10.1038/37333
Spero, H. J., Eggins, S. M., Russell, A. D., Vetter, L., Kilburn, M. R., and Hönisch, B. (2015). Timing and mechanism for intratest Mg/Ca variability in a living planktic foraminifer. Earth Planet. Sci. Lett. 409, 32–42. doi: 10.1016/j.epsl.2014.10.030
ter Kuile, B., Erez, J., and Padan, E. (1989). Mechanisms for the uptake of inorganic carbon by two species of symbiont-bearing foraminifera. Mar. Biol. 103, 241–251. doi: 10.1007/BF00543354
van Dijk, I., de Nooijer, L. J., Boer, W., and Reichart, G. J. (2017a). Sulfur in foraminiferal calcite as a potential proxy for seawater carbonate ion concentration. Earth Planet. Sci. Lett. 470, 64–72. doi: 10.1016/j.epsl.2017.04.031
van Dijk, I., Nooijer de, L. J., and Reichart, G. J. (2017b). Trends in element incorporation in hyaline and porcelaneous foraminifera as a function of pCO2. Biogeosciences 14, 497–510. doi: 10.5194/bg-14-497-2017
van Dijk, I., Mouret, A., Cotte, M., Le Houedec, S., Oron, S., Reichart, G. J., et al. (2019). Chemical Heterogeneity of Mg, Mn, Na, S, and Sr in Benthic Foraminiferal Calcite. Front. Earth Sci. 7:281. doi: 10.3389/feart.2019.00281
Verhulst, P. J. (1938). Notice sur la lois Que la population Suit Dans sons accroissenment. Corresp. Mathématique Phys. 10, 113–121.
Vielzeuf, D., Gagnon, A., Ricolleau, A., Devidal, J.-L., Balme-Heuze, C., Yahiaoui, N., et al. (2018). Growth kinetics and distribution of trace elements in precious corals. Front. Earth Sci. 6:167. doi: 10.3389/feart.2018.00167
Vogel, N., and Uthicke, S. (2012). Calcification and photobiology in symbiontbearing benthic foraminifera and responses to a high CO2 environment. J. Exp. Mar. Biol. Ecol. 424, 15–24. doi: 10.1016/j.jembe.2012.05.008
Warter, V., Erez, J., and Müller, W. (2018). Environmental and physiological controls on daily trace element incorporation in Tridacna crocea from combined laboratory culturing and ultra-high resolution LA-ICP-MS analysis. Palaeogeogr. Palaeoclimatol. Palaeoecol. 496, 32–47. doi: 10.1016/j.palaeo.2017.12.038
Wit, J. C., de Nooijer, L. J., Wolthers, M., and Reichart, G. J. (2013). A novel salinity proxy based on na incorporation into foraminiferal calcite. Biogeosciences 10, 6375–6387. doi: 10.5194/bg-10-6375-2013
Woodhead, J. D., Hellstrom, J., Hergt, J. M., Greig, A., and Maas, R. (2007). Isotopic and elemental imaging of geological materials by laser ablation inductively coupled plasma-mass spectrometry. Geostand. Geoanal. Res. 31, 331–343. doi: 10.1111/j.1751-908X.2007.00104.x
Yu, J., and Elderfield, H. (2007). Benthic foraminiferal B/Ca ratios reflect deep water carbonate saturation state. Earth Planet. Sci. Lett. 258, 73–86. doi: 10.1016/j.epsl.2007.03.025
Yu, J., and Elderfield, H. (2008). Mg/Ca in the benthic foraminifera Cibicidoides wuellerstorfi and Cibicidoides mundulus: temperature versus carbonate ion saturation. Earth Planet. Sci. Lett. 276, 129–139. doi: 10.1016/j.epsl.2008.09.015
Yu, J., Elderfield, H., Jin, Z., Tomascak, P., and Rohling, E. J. (2014). Controls on Sr/Ca in benthic foraminifera and implications for seawater Sr/Ca during the late Pleistocene. Quat. Sci. Rev. 98, 1–6. doi: 10.1016/J.QUASCIREV.2014.05.018
Keywords: biomineralization, LA-ICPMS, foraminifera, Amphistegina, trace elements, Mg banding, DIC, primary calcite
Citation: Levi A, Müller W and Erez J (2021) Intrashell Variability of Trace Elements in Benthic Foraminifera Grown Under High CO2 Levels. Front. Earth Sci. 7:247. doi: 10.3389/feart.2019.00247
Received: 06 February 2019; Accepted: 04 September 2019;
Published: 04 October 2019.
Edited by:
Alberto Perez-Huerta, The University of Alabama, United StatesReviewed by:
Alexey Kamyshny, Ben-Gurion University of the Negev, IsraelWilliam Patrick Gilhooly III, Purdue University Indianapolis, United States
Copyright © 2021 Levi, Müller and Erez. This is an open-access article distributed under the terms of the Creative Commons Attribution License (CC BY). The use, distribution or reproduction in other forums is permitted, provided the original author(s) and the copyright owner(s) are credited and that the original publication in this journal is cited, in accordance with accepted academic practice. No use, distribution or reproduction is permitted which does not comply with these terms.
*Correspondence: Adam Levi, YWRhbS5sZXZpQG1haWwuaHVqaS5hYy5pbA==