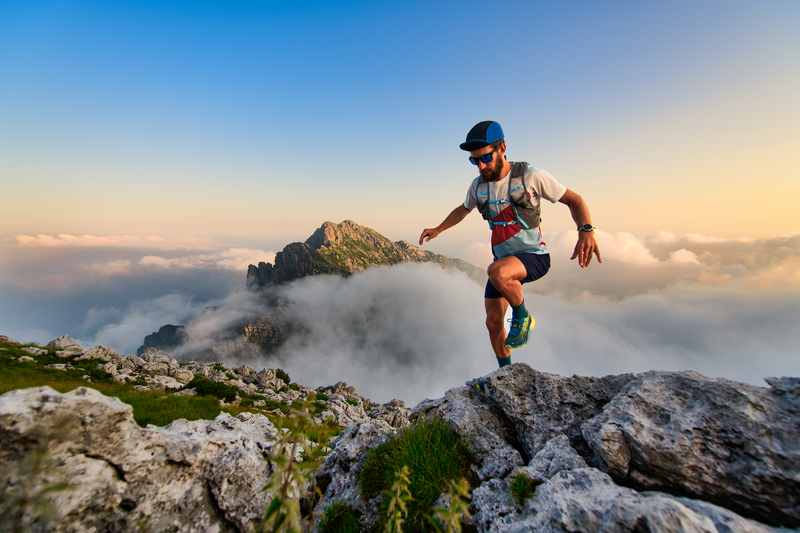
94% of researchers rate our articles as excellent or good
Learn more about the work of our research integrity team to safeguard the quality of each article we publish.
Find out more
ORIGINAL RESEARCH article
Front. Earth Sci. , 13 August 2019
Sec. Paleontology
Volume 7 - 2019 | https://doi.org/10.3389/feart.2019.00209
A marine bonebed from the Campanian (Upper Cretaceous) Bearpaw – Dinosaur Park Formation transition, containing both micro- and macrovertebrate fossils and trace fossils, was discovered in west-central Saskatchewan, Canada. The bonebed formed during transgression of the Western Interior Seaway, with the stratigraphy of the area displaying extensive interfingering of these terrestrial and marine facies. The macrovertebrate fossils occur in a layer of fine-grained, unconsolidated sand. Directly overlying this stratum is a layer of sulfur-rich, medium-grained sandstone, containing microvertebrate fossils. Most of the material in both layers is highly encrusted in gypsum. We propose that the bonebed formed in a barrier island system over three stages: original deposition of marine reptile bones in a silled basin over a period of sediment starvation, redeposition and burial of the bone material due to higher energy flow through the basin, and deposition of bony and cartilaginous fish material during a period of water stratification and subsequent euxinic (anoxic and sulfuric) conditions. Because sediment deposition rates were low when the marine reptile bones accumulated, these elements spent extended periods at the sediment-water interface, allowing them to become bioeroded. The bioerosion at this site takes two main forms: parallel-sided boreholes about one cm wide, and extensive hollowing out of the cancellous bone. Although the invertebrate tracemakers were not preserved the boreholes resemble clavate borings made by bivalves, and the hollowing could have been caused by polychaete worms or grazing gastropods. The presence of sulfur-rich deposits and chemosymbiotic Chondrites isp. traces in the layer above the bonebed suggest the development of a stratified water column in the basin, with euxinic (anoxic and sulfuric) conditions near the seafloor. Disruption of the water column culminated in a mass die-off of non-air breathing vertebrates in the surface waters and constitutes the microvertebrate layer of the bonebed. Marine reptile bonebeds are rare in the fossil record, and this site represents the first time a vertebrate assemblage has been described from a barrier island system in Saskatchewan.
The hamlet of Herschel in west-central Saskatchewan, Canada (Figure 1A) is the site of a rare multitaxic, micro- to macrovertebrate bonebed in the Upper Cretaceous marginal marine to marine strata of the Dinosaur Park – Bearpaw formation transition (Figure 1B). Bonebeds preserving the fossil remains of multiple individuals, while uncommon in terrestrial settings, are particularly rare for marine macrovertebrates (Eberth et al., 2007; Rogers and Kidwell, 2007). Bonebeds can form as biogenic and/or physical concentrations (Rogers and Kidwell, 2007). In marine settings, bonebeds are more often the result of physical processes, whether hydraulic, sedimentologic, or a combination of the two. Sedimentologic concentrations include attritional accumulations, in which bioclast input is greater that sediment input, and can result in time-averaged bonebeds with variable quality of preservation of the fossils preserved therein (Rogers and Kidwell, 2007).
Figure 1. Locality map. (A) Map of Canada with Saskatchewan shaded in black. Modified from Bamforth et al. (2014). (B) Map of Saskatchewan with location of Herschel indicated with a star. Orange shading indicates outcrops of the Bearpaw Formation. Modified from Dawson et al. (1994).
Herschel, Saskatchewan, is located approximately 150 km southwest of the city of Saskatoon. The area was primarily known for its First Nations’ history and archeological sites, including petroglyphs, effigies, and at least one buffalo jump, the study of which led to the initial discovery of the local paleontological resources. In 1990, the partial skeleton of a sub-adult polycotylid plesiosaur was discovered close to the community, turning attention toward paleontology in the area. The specimen was excavated by Tim Tokaryk of the Royal Saskatchewan Museum, and was formally described as Dolichorhynchops herschelensis Sato, 2005, a new species endemic to Saskatchewan. Further prospecting revealed the area to be moderately rich in marine fossil material. Of particular note to Tokaryk was a small knoll approximately 200 m from the D. herschelensis type locality, from which a large amount of fossil material was weathering. Excavation of this knoll, which became the Herschel marine bonebed, revealed an abundance of vertebrate fossils, trace fossils, and coalified wood in a concentrated area.
The bonebed site has been collected by the Royal Saskatchewan Museum several times in the last 30 years, with major excavations undertaken in 1997, 2006, and 2014. The Royal Saskatchewan Museum now curates thousands of fossil specimens from the Herschel bonebed. The locally run Ancient Echoes Interpretive Centre at Herschel exhibits the region’s rich archeological and paleontological history. Much of the archival material pertinent to the discovery and collection of D. herschelensis and the Herschel bonebed can be found there.
The vertebrate fauna at the Herschel marine bonebed is dominated by isolated plesiosaur skeletal elements, with elasmosaurids being more abundant than polycotylids. Mosasaur and marine turtle elements are much rarer components of the fauna. The microvertebrate component of the bonebed consists of both teeth and vertebrae of bony and cartilaginous fishes. Abundant trace fossils, mainly belonging to the ichnogenus Planolites isp., are present in the collection, as are several pieces of coalified wood.
Numerous macrovertebrate fossils recovered from the Herschel marine bonebed preserve evidence of bioerosion. In modern marine settings, whale-falls host communities of benthic bone-consuming detritivores and chemoautotrophs in both shallow- (Dahlgren et al., 2006; Braby et al., 2007; Fujiwara et al., 2007) and deep-water environments (Baco and Smith, 2003; Smith and Baco, 2003; Braby et al., 2007; Johnson et al., 2010). Such associations have also been observed in the fossil record for both Cenozoic marine mammals (Kiel et al., 2010, 2013; Higgs et al., 2012; Danise and Higgs, 2015) and Mesozoic marine reptiles (Kaim et al., 2008; Danise et al., 2014). The assemblages of invertebrates found colonizing modern whale-falls include various families of polychaete worms (Baco and Smith, 2003; Fujiwara et al., 2007), including multiple species of the bone-boring Osedax (Braby et al., 2007; Higgs et al., 2010), crustaceans including Paralomas (Johnson et al., 2010), mytilid bivalves (Fujiwara et al., 2007), and gastropods such as Rubyspira (Johnson et al., 2010). Small boreholes, pock-mark-like collapsed galleries, and more extensive bioerosion have been attributed to the presence of Osedax in the fossil record (Kiel et al., 2011, 2013; Higgs et al., 2012; Boessenecker and Fordyce, 2014; Danise and Higgs, 2015). Other fossils record evidence of scavenging by echinoderms (Danise et al., 2014) and gastropods (Kaim et al., 2008).
The purpose of this study is to describe the formation of this highly unique bonebed, deposited during the transition between the terrestrial Dinosaur Park Formation and the marine Bearpaw Formation. The ichnology associated with the vertebrate material in this bonebed and the unusual bioerosion of the fossils are described. The unique stratigraphy, lithology, and array of trace fossils allow for interpretations about the ecology and depositional setting to be made regarding this region of the Western Interior Sea during the Late Campanian age of the Cretaceous.
The Upper Cretaceous Bearpaw Formation was deposited in the Western Canadian Sedimentary Basin during the Campanian (∼76–70 Ma). Orogeny in the Canadian Cordillera was active, flexing the North American craton to create accommodation space. This, combined with high global sea levels, resulted in the formation of the Western Interior Seaway, effectively bisecting North America from the Gulf of Mexico to the Arctic (Dawson et al., 1994). Regional subsidence controlled by tectonics resulted in transgressive-regressive cycles, with clastic sedimentation provided by erosion of western mountain sediments. The Bearpaw Formation represents one of the last transgressive pulses of the Western Interior Seaway across the Canadian plains (Kauffman and Caldwell, 1993). The Bearpaw Formation gradationally to abruptly overlies the Dinosaur Park Formation, the uppermost formation of the Belly River Group (and Judith River Formation equivalents of Montana) (McLean, 1971; Dawson et al., 1994; Gilbert et al., 2019).
The Dinosaur Park Formation of Alberta and Saskatchewan is comprised of alluvial, floodplain, estuarine, and lagoon facies bounded at the base by a regional disconformity, and is gradationally to abruptly overlain by marine deposits of the Bearpaw Formation (Eberth and Hamblin, 1993; Gilbert et al., 2018, 2019). The Bearpaw Formation of central Canada and the north-central United States records marine deposition in a shallow, epicontinental seaway, with coarse-grained sedimentation controlled by wave, storm, and fluvial processes. As sea level was in continuous flux, the deposits in the Herschel area are characterized by an interfingering of marine and terrestrial units. Interfingering is commonly seen across the eastern exposures, recording the onset of transgression (e.g., Woodhawk Member of the Judith River Formation) and has been observed in the Cypress Hills region of southwestern Saskatchewan (McLean, 1971; Rogers et al., 2016; Gilbert et al., 2019).
The regional geology at Herschel is represented by marginal marine facies of the Dinosaur Park Formation abruptly overlain by fully marine shales of the Bearpaw Formation. Transitional facies are characteristic of a marginal marine setting, as sediments were deposited along a dynamic paleocoastline. A composite stratigraphic section of the area (Figure 2) suggests the Dinosaur Park Formation at Herschel is a tongue of terrestrial sediment bound between marine strata of the Bearpaw Formation, during the initial transgressive phase of the 2nd order Bearpaw Cycle (Kauffman and Caldwell, 1993; Tsujita, 1995; Gilbert et al., 2019). Palynological analysis of sediments from the site constrain it stratigraphically to within the uppermost Dinosaur Park Formation and the lowermost Bearpaw Formation (Gilbert et al., 2019), dating to late, but not the latest, Campanian age (Baadsgaard et al., 1992). Placing the marginal marine deposits within the Dinosaur Park Formation, instead of the Bearpaw Formation, is consistent with Gilbert et al. (2019) treatment of the formation in the Cypress Hills, where estuary, lagoon, and barrier island sandstones immediately overlying coastal plain deposits were considered marginal marine expressions of the Dinosaur Park Formation.
Figure 2. Composite section of strata and facies associations in Coal Miners’ Ravine, Herschel, Saskatchewan. Symbols are as indicated in the legend. Stratigraphic position of the bonebed-bearing knoll indicated with BB, shown in detail in Figure 3. Modified from Gilbert et al. (2019).
The Herschel marine bonebed is located on the southeast side of Coal Miners’ Ravine, a tributary of Eagle Creek, a large, shallow glacial meltwater valley that runs through west-central Saskatchewan. Rotational-slump faulting due to modern channel migration of Coal Miners’ Creek has offset the stratigraphy on the two sides of the ravine, such that the strata containing the bonebed sit topographically lower in section than the corresponding strata on the northwest side. Lowermost exposures in Coal Miners’ Ravine represent wave-dominated shallow marine deposits of the Bearpaw Formation and are overlain by barrier island sandstones, lagoon heterolithics, and coastal plain mudstones of the Dinosaur Park Formation (Gilbert et al., 2019). The upper Dinosaur Park – Bearpaw Formation contact in the area is marked by a marine flooding surface and a transition from cross-bedded sandstone to shale (Figure 2).
The site was deposited in a transitional zone and records interfingering marginal marine and terrestrial deposits of the Dinosaur Park Formation and marine sediments of the Bearpaw Formation. The interface between the interfingering terrestrial and marine facies is characterized by a wave-dominated, tidally influenced, fluvially affected coastline dominated by barrier island, lagoon, and estuary deposits (Gilbert et al., 2018, 2019). This, combined with regional slump faulting, has resulted in complex stratigraphy with little lateral continuity. The projection of Dinosaur Park facies represents a progradational wedge in an overall transgressive cycle, suggesting the site formed during a time when the rate of sedimentation exceeded the rate at which accommodation space was being created. In regressive barrier island systems of this nature, estuary and tidal flat facies on the paleocoastline would have remained relatively stable as lagoon and barrier island deposits migrated seaward (Timmons et al., 2010). This is analogous to modern barrier island systems at Morgan Peninsula, Alabama (Rodriguez and Meyer, 2006).
Fossil specimens from the Herschel marine bonebed were obtained by the Royal Saskatchewan Museum in a number of collection events between 1990 and 2019. Fossil positions were mapped using a one-meter grid prior to removal from the quarry, and the specimens were prepared, identified, and cataloged at the T. rex Discovery Centre, Eastend, SK, Canada or the Royal Saskatchewan Museum, Regina, SK, Canada. Specimens from the Herschel collection are currently curated at the T. rex Discovery Centre. Each collection event was designated a specific division number, under which all specimens collected during that event were cataloged. The following division numbers were designated for the Herschel marine bonebed collection: RSKM P2311, RSKM P2625, RSKM P2975, RSKM P3182, RSKM P3197, RSKM P3198, and RSKM P3199. Within each division number, cataloge numbers separated by a period (e.g., RSKM P2975.1) were assigned to individual specimens. The rose diagram used to plot the fossil alignment along fossil’s long axis was generated using the “rose.diag” function in the “Circular” package (Agostinelli and Lund, 2017) of R Statistical Software, version 3.4.4 (R Core Team, 2018). The fossil orientations that were used in this diagram were measured from the quarry map.
The images of the bioeroded specimens illustrated in this study were generated using a Z-Stack (focal stacking) method. Specimens that are tall, rounded, or have convoluted shapes are often difficult to photograph with the entire fossil in focus due to the large depth of field. Z-Stacking is a method whereby multiple images are taken of an object at different focal lengths, and the images are then combined into a single composite image in which the whole specimen is in focus. The specimen photographs were taken at the Royal Saskatchewan Museum with a Visionary Digital photography station using a Canon EOS 5D DSLR camera with Canon EF65 mm lens and Tamron SP AF teleconverter. Specimens were photographed at 10 mm focal intervals, and the subsequent images were combined (stacked) using Helicon Focus 5.4.14 software.
In order to determine the elemental composition of unusual nodules found in the bonebed layer, X-ray florescence analysis (XRF) was employed. XRF is a technique that classifies elements based on the amount of energy released when electrons are displaced from their atomic orbital positions by an X-ray beam. In our analysis, we used a portable Brucker Handheld XRF Analyzer for multi-elemental analyses. The relative elemental composition of the sample was interpreted using a spectral overlay of elemental concentrations, generated using the software provided by the manufacturer. XRF was used to analyze the elemental composition of the Herschel fossil material, bonebed matrix, shale above the microsite layer, and the nodules, though only the composition of the nodules is discussed herein.
The Herschel bonebed is located on a small knoll of outcrop, approximately 1.5 m high, on the southeastern edge of Coal Miners’ Ravine. The strata overlying the bonebed, and forming the majority of the outcrop, consist of massive, medium to dark gray, and dominantly structureless marine shale (Figure 3A). Localized silt, sand, and sulfur stringers, with rare oscillatory ripples, 3–5 mm in thickness, are pervasive throughout this unit.
Figure 3. Lithology of the Herschel bonebed fossil site. Scale bars are as indicated. (A) General view of outcrop. White rectangle represents the strata shown in (B). (B) Detailed lithology of fossil- and ichnofossil-bearing layers. (C,D) Chondrites isp. (E) Detail of sulfur-rich microvertebrate bearing layer (note black carbonized plant material and white gypsum crystals). (F) Fish vertebra found within microvertebrate layer (note crust of gypsum encapsulating each fossil). (G) RSKM P3229, in situ macrovertebrate fossils from bonebed (from left, plesiosaur propodial and two vertebrae with neural arches attached; note directional alignment of specimens and gypsum encrustation). (H) Manganese and iron-rich nodule. (I) Amber granules found in association with carbonized wood. (J) RSKM P3197.197, ghost crab burrow (Spongeliomorpha isp.). (K) RSKM P3197.206, invertebrate feeding trace (Planolites isp.). CL, Chondrites isp. layer; MvL, microvertebrate layer; BbL, bonebed layer.
The bonebed layer is approximately 15–20 cm thick. In contrast to the massive shale unit that overlies it, the strata above and below the bonebed comprise multiple different lithologies (Figure 3B). Underlying the bonebed is a thin layer of finely laminated shale, gray to yellow-gray (Munsell Soil Color Charts, 2010; 5YR 6/4) in color, and rich in fine black carbonaceous plant debris. Overlying this is the marine macrovertebrate bonebed layer (Figure 3B), approximately 10–15 cm thick, consisting of a fine-grained olive-colored (10Y 6/2), unconsolidated sandstone. The stratum containing the macrovertebrate bonebed is dominantly structureless, but does record small parallel laminae consisting of interbedded mudstone, shale and siltstone. The vast majority of the large vertebrate bones are encrusted with gypsum (Figure 3G), with large gypsum crystals found isolated among the skeletal elements. Coalified wood and amber granules (Figure 3I) are also common throughout. Occasional fish vertebrae and shark teeth were recovered among the macrovertebrate fossils. The bonebed layer is associated with ghost crab burrows (c.f. Spongeliomorpha isp.) (Figure 3J), as well as Planolites isp., (Figure 3K) cylindrical, straight to jointed, and sand-filled casts of invertebrate feeding traces. The bonebed also contained two enigmatic clusters of egg-shaped nodules (Figure 3H). Analysis by X-ray fluorescence revealed these nodules to consist almost entirely of iron and manganese.
Overlying the macrovertebrate bonebed is a laterally continuous 2–7 cm thick, yellow-orange (5YR 8/14) deposit consisting of sulfur, medium-grained sand, pieces of carbonized wood and amber (Figure 3E), and abundant gypsum-encrusted fish and shark isolated elements (Figure 3F). This distinct sulfur-rich layer is referred to as the “microvertebrate layer,” in reference to the vertebrate material it contains. Within the microvertebrate layer, the medium-grained sand deposits occasionally appear to be finely laminated with organic material. This carbon-rich, sulfur deposit reoccurs in patches farther up in the section in the strata overlying the bonebed. Directly above the bonebed layer, the deposits are heavily bioturbated with a monospecific Chondrites isp. assemblage (Figures 3C,D). This same trace fossil is found inside some of the hollowed out marine reptile bones. Above the Chondrites isp. layer is the medium to dark gray, dominantly structureless marine shale that comprises most of the outcrop. Mottled strata, consisting of small sulfur-rich patches is observed close to the base of this stratum. This type of strata is often seen as the result of sediment reworking in other Saskatchewan marine deposits. The presence of a small number of fossils approximately one meter above the bonebed layer to the northwest of the main bonebed (Figure 4A) is consistent this observation.
Figure 4. Quarry map. (A) Detailed map of the location of the fossils recovered from the bonebed. Orange shaded fossils were found one meter above the rest of the bonebed. Original quarry map created by D. Stoffregen and W. Long. (B) Rose diagram of the orientation fossils in the bonebed demonstrating current alignment. F, fish vertebra; G, gastrolith; S, shark tooth.
Spatially, the macrovertebrate fossils in the bonebed layer are not evenly distributed. There are two distinct concentrations of fossils (Figure 4A), each of which contains bones primarily aligned in one direction. The northern arm of the bonebed was uncovered first when excavation began on the north face of the bonebed-hosting knoll. In this northern arm of the bonebed, the fossils are somewhat more dispersed, and long-bones and ribs lie primarily in an east-west orientation (Figure 4B). As the excavation progressed, the denser southern arm of the bonebed was revealed. Ribs in this part of the bonebed are oriented in a northeast-southwest direction (Figure 4B).
Over 3,100 fossil specimens have been recovered from the Herschel Marine bonebed. These fossils include both body and trace fossils, primarily of marine species but also including rare terrestrial taxa. Large marine reptile fossils are the most abundant, but there are also numerous teeth and vertebrae from both bony and cartilaginous fishes. A detailed investigation of the fish diversity preserved in the bonebed is beyond the scope of the present study, but preserved teeth indicate the presence of ichthyodectids, Enchodus sp., Protosphyraena sp., Ischyodus rayhaasi, hybodontids, odontaspidids, and other lamniforms. Most of the marine reptile fossils are referable to polycotylid or elasmosaurid plesiosaurs, though there are also numerous mosasaur remains, representing both Mosasaurinae and Plioplatecarpinae. Finally one identifiable marine turtle costal has also been found. There is a relatively high abundance of juvenile plesiosaur fossils, as evidenced by small, incompletely ossified propodials (humeri and femora) and numerous unfused vertebral centra and neural arches (Brown, 1981). Molluscan fossils are vanishingly rare in the bonebed, with just one ammonite chamber infill recovered; no shelly molluscan material has been found. Trace fossils include both burrows and feeding traces such as Chondrites isp., Planolites isp., and Spongeliomorpha isp. Terrestrial fossils include layers of plant hash below the macrovertebrate bonebed layers, pieces of coalified wood, up to ∼305 mm long with amber inclusions, and champsosaur ribs.
In addition to the isolated burrows and feeding traces (Figures 3J,K), trace fossils from the marine bonebed include evidence of bioerosion of the preserved macrovertebrate skeletal material. The two primary forms of bioerosion are large (mean ∼ 13 mm diameter) boreholes (Figures 5A–C) and extensive hollowing out of bones (Figures 5B–H). Of these two types of trace fossils, the hollowed bones are more common than the boreholes: hollowing was observed on 27 specimens, boreholes were observed on 5 specimens, and one small plesiosaur propodial was both bored and hollowed (Figures 5B,C). The numerous partial specimens and bone fragments, for which the source of damage could not be determined, were not included in this tally, nor were the microvertebrate fossils. We did not observe evidence of macrovertebrate bioerosion in the form of scavenging scrape marks.
Figure 5. Bioeroded plesiosaur fossils. (A) RSKM P3199.21, vertebra in ventral view exhibiting borehole along path of nutritive foramina. (B) RSKM P3199.152, propodial in post-axial view exhibiting borehole. (C) RSKM P3199.152, same propodial in dorsal or ventral view, also exhibiting hollowing. (D) RSKM P2975.377, distal end of propodial exhibiting hollowing. (E) RSKM P2975.557, vertebra in dorsal view exhibiting hollowing. (F) RSKM P2975.1469, rib exhibiting hollowing. (G) RSKM P2975.1722, distal end of propodial in pre- or post-axial view exhibiting hollowing. (H) RSKM P2975.377, same propodial in proximal view exhibiting hollowing and infilling by Chondrites isp. Black box indicates area magnified in (I). (I) RSKM P2975.377, close-up of Chondrites isp. from (H). Scale bars A-H = 5 cm. Scale bar I = 5 mm.
The boreholes form tubes that pierce through the cortical bone into the cancellous bone (Figures 5A–C). These ichnofossils differ from Gastrochaenolites isp. (produced by bivalves through mechanical boring) in that they are parallel-sided throughout their length, rather than exhibiting a narrower neck and expanded chamber, known as clavate traces (Belaústegui et al., 2012). Additionally, some of the boreholes penetrate all the way through both sides of the element (Figure 5A), unlike the parallel-sided borings reported by Tapanila et al. (2004); whether this morphology reflects the original trace of the boring or is the result of secondary taphonomic processes is unclear. The width of these borings, and the individual rather than grouped morphology, distinguish them from Trypanites isp. (Muñiz et al., 2010). No tracemaker was found in situ in any of these borings, but while the traces are atypical for clavate traces, it is still possible that a bivalve is the responsible tracemaker. In some cases the boreholes appear to have followed the nutritive foramina that characteristically perforate the ventral surface of plesiosaur vertebral centra (Figure 5A).
While various skeletal elements in the bonebed exhibit the second form of bioerosion, it is best exemplified by the propodials that have been hollowed out from their distal extremities (Figures 5B,G). In these cases, the cancellous bone has been consumed from the articular facets, leaving only thin laminae of the dorsal and ventral surfaces of the distal ends of the fossils. This process can also erode the proximal ends of propodials but usually to a lesser degree. Hollowing bioerosion of vertebral centra in the bonebed occurred at various angles, sometimes leaving one surface of the element intact (Figure 5E) and other times leaving just a core of cancellous bone. This lack of directional pattern likely reflects the orientation in which the vertebrae were sitting on the seafloor and the surfaces that were most available to the bioeroding invertebrates. Some ribs appear to have been consumed along their lengths (Figure 5F). It is unclear whether incomplete ribs broke due to other taphonomic processes or whether their ends were completely consumed.
The hollowed fossils differ from the morphology attributed to the ichnospecies Osspecus tuscia Higgs et al., 2012, which is characterized by 0.5 – 0.7 mm holes perforating the bone surface and connecting to short canals and globular chambers up to 5 mm in diameter (Higgs et al., 2012). Some of the vertebrate material from the Herschel bonebed might have exhibited similar patterns of bioerosion at one time, but the surface preservation of most of the fossils is too poor to allow for confident identification of this ichnospecies.
Based on our observations of the site, we suggest that the Herschel bonebed formed in three stages, reflective of shifts in the paleoenvironment. The initial deposition of the bonebed material took place in a barrier island system at the western edge of the Western Interior Sea (Figure 6A). This silled basin would have been protected from the open ocean, but would not have been completely restricted from it. The macrovertebrate assemblage includes an unusually high proportion of juvenile plesiosaur fossils; during this stage, we suggest that plesiosaurs inhabited the basin, congregating in the area to give birth and raise their young (Wiffen et al., 1995; Kear, 2006; Martin et al., 2007). The bones of individuals who died in this environment were deposited across the sea floor during a period of sediment starvation, resulting in an accumulation of bone material over time. It is notable that despite a hypothesized close proximity to land, there are no terrestrial vertebrate fossils in the bonebed other than three champsosaur ribs. However, terrestrial plant fossils are common, including large pieces of wood containing grains of amber, abundant fragments of coalified plant material throughout the bonebed, and a layer of plant hash directly under the macrovertebrate marine bone layer. It is possible that a coastal marsh acted as a sediment trap, thus preventing the majority of terrestrial organic remains or much terrestrial sediment from reaching the basin as is seen in modern coastal systems (Stumpf, 1983; Neubauer et al., 2002). While a macrovertebrate bonebed could form as a similar attritional accumulation in deeper water, we consider the prevalence of juvenile marine reptile fossils and terrestrial plant material to be shallow water indicators. Additionally, the coarser grain size of the sediments comprising the bonebed horizons differ from the fine-grained shales associated with deposition in the open marine Bearpaw Sea that are seen in the section above and below the tongue of Dinosaur Park Formation (Figure 2).
Figure 6. Environmental interpretation. (A) Region at time of original deposition of marine reptile bones within a barrier island system; a time of sediment starvation and bioerosion of the bones. (B) A new inlet to the basin opens through the barrier islands changing the regional hydrodynamics; the unburied bones are transported into a newly formed channel and are aligned with the current. (C) Rising sea level, as a result of a marine transgression floods the barrier islands and the coastal marshes; the water column becomes stratified, leading to euxinia of the bottom waters, and the subsequent deposition of the microvertebrate layer. Sedimentary fill swatches from U.S. Geological Survey, (2006).
This interval of low sediment input would have allowed for invertebrates such as polychaetes and gastropods to colonize and consume bones exposed at the sediment-water interface. The extensive bioerosion exhibited by some of the macrovertebrate fossils (Figure 5) provides insights about both the biotic and aboitic factors of the environment in which the bones were deposited. In modern whale-falls, species that colonize buried bones are less common and represent late phases of community succession (Braby et al., 2007; Lundsten et al., 2010). Therefore, the extent of bioerosion could indicate low levels of disturbance and sedimentation. These bioeroded specimens may have spent multiple years at the sediment-water interface before burial, facilitated by the sediment starvation attributable to a coastal marsh, resulting in an attritional accumulation. The degree of bioerosion resembles that seen on whale bones that had lain on the seafloor for four to seven years (Braby et al., 2007; Johnson et al., 2010). Even many of the elements lacking bioerosion show evidence of physical weathering (abrasion, fragmentation) further supporting the hypothesis of delayed burial. The number of specimens reported to exhibit hollowing and boreholes likely underrepresents the actual frequency of bioerosion. It is possible that some of the numerous fragmentary fossils recovered from the bonebed are the remnants of heavily bioeroded bones.
While we were unable to recognize published examples of trace fossils identical to the hollowing of skeletal elements preserved in the Herschel marine bonebed, preferential destruction of long-bone ends due to grazing activity of gastropods has been observed in modern human forensic studies (Haglund and Sorg, 2002), and consumption of cancellous bone has been observed in the marine mammal fossil record (Boessenecker et al., 2014). Additionally, this state of preservation somewhat resembles that displayed by an early Oligocene whale rib interpreted to have been colonized by the bone-eating worm Osedax (Kiel et al., 2010), however, no shallow, sub-millimeter sized bore-holes have been observed on the fossils from the Herschel marine bonebed. Extended time at the sediment-water interface could have allowed traces, such as chambers attributable to O. tuscia, to merge, or for secondary processes – whether scavenging by larger invertebrates or chemical dissolution – to enlarge the original chambers, thereby obliterating identifiable ichnofossils.
If Osedax spp. preferentially consume denser cortical bone (Higgs et al., 2010) and damage to cancellous bone is due to mollusks or crustaceans grazing on exposed Osedax spp. palps and microbial mats, it is at first surprising that no fossils of these invertebrates are preserved in the bonebed. Numerous gastropod shells have been found at the sites of both modern whale-falls (Johnson et al., 2010) and marine reptile-falls (Kaim et al., 2008). The lack of calcareous shell material from the Herschel marine bonebed is more likely a taphonomic than biologic signal, whereby calcareous material was dissolved through diagenetic processes. The single fragment of ammonite that has been recovered from the site is preserved as an internal mold, retaining none of the original aragonitic shell. Additionally, the invertebrate community (crustaceans and gastropods) responsible for consuming cancellous bone has previously been reported from modern deep-water environments, so the discovery of that kind of bioerosion in a shallow-water setting was unanticipated (S. Johnson, pers. comm.). It would imply a range extension for gastropods similar to Rubyspira spp. or fossil provannids from the Upper Cretaceous of Japan. However, different species of extant Osedax have been reported to inhabit different water depths (Braby et al., 2007) and numerous mollusks were observed to colonize whale-falls in shallow marine settings (Fujiwara et al., 2007).
Interestingly, despite the apparent abundance of invertebrate scavengers, there is no indication of macrovertebrate scavenging. The bones bear no evidence of the surficial grooves or scratches attributed to scavenging by sharks (Schwimmer et al., 1997; Pobiner, 2008). While it is possible that a combination of generally poor surface preservation and removal of the encrusting gypsum crystals could have obliterated some grooves or scratches, the complete absence of such marks suggests that macrovertebrate scavengers were less abundant than their invertebrate counterparts. Additionally, despite recovering hundreds of shark teeth from the bonebed, none of the teeth is referable to Squalicorax species, which are considered to be some of the primary vertebrate scavengers of the Late Cretaceous Western Interior Sea (Schwimmer et al., 1997). Even though Squalicorax spp. teeth can be locally less abundant than those attributable to contemporaneous genera such as Cretalamna (Schwimmer et al., 1997), their complete absence from this site is surprising.
The fossil distribution in the bonebed indicates that some environmental process occurred to concentrate and channelize the deposit. It is possible that the fossils settled into existing depressions on the seafloor, but that would not account for the differential alignment of the bones in the two arms of the bonebed (Figure 4B). We propose that current-producing forces – whether geologically instantaneous, such as a storm that opened a new inlet in the barrier island, or prolonged, such as a change in prevailing wind directions – altered or increased the currents flowing through the system (Figure 6B). The Dinosaur Park – Bearpaw Formation transition has been previously described as being dominated by wave processes, tidal currents, and occasional storm events (Gilbert et al., 2019). The formation of a new inlet would effectively change the hydrodynamics of the immediate area, allowing tide, wave, and storm currents to erode the inlet and lagoon sediments. This would generate localized turbulence and scouring of the substrate, allowing for the bones to be secondarily concentrated into a bonebed (Figure 4). This transport and increase in water energy would account for the fragmentation and abrasion observed on many of the fossils. Stronger currents would have carried a higher sediment load, eventually burying the bones.
The sedimentology and ichnology of the Herschel site suggest that the region underwent a period of euxinia (a form of anoxia combinded with sulfuric conditions) after the redeposition of the macrovertebrate fossil material. Anoxia has been found in modern lagoonal environments, and is attributed to increased environmental temperatures, high organic content, and low water turbidity, often due to decreased wind speed (Cioffi et al., 1995; Harzallah and Chapelle, 2002). Euxinia occurs when anoxia is coupled with the presence of organic matter, sulfate ions, and sulfur-reducing bacteria. This scenario usually occurs in near-shore settings including silled basins (i.e., barrier island bays) and restricted saltwater bodies with a freshwater influence from rivers or streams (Nägler et al., 2011). In silled basin environments that lack regular upwellings that mixes waters of different densities that occurs in the open ocean, euxinia can also develop when the water column becomes strongly stratified, with an oxic, highly productive surface layer and an anoxic, sulfidic bottom layer. In silled basins, rising sea-level can exacerbate euxinia by enhancing stratification of the water with the introduction of denser saltwater (Middelburg et al., 1991). Euxinia has been noted in restricted saltwater bodies such as the Black Sea, which is fed by several freshwater rivers (Nägler et al., 2011).
The fossil plant material in the bonebed implies that the site was deposited relatively near to shore, indicating the silled basin had some freshwater influence. The presence of some trace fossils, bioerosion, and bioturbation in the bonebed layer suggests that the water column at the time of initial deposition was not strongly stratified. It is possible that there may have been periodic stratification events however, as is suggested by the presence of Chondrities isp., a trace fossil known to frequent deoxygenated to anoxic bottom water in marine to marginal marine environments (Seilacher, 1990) and the occurrence of manganese nodules in the bonebed. Manganese nodules (also called polymetallic nodules) are rock concretions composed of iron and manganese hydroxides that form around a core, which are found globally on the seafloor. Fossil manganese nodules date back as far as 137 Mya (Ito and Komuro, 2006), and tended to form in oxic, deep-water conditions where the sedimentation rate was low (Usui and Ito, 1994). Today these nodules are typically restricted to deep-water settings such as abyssal plains, but there are a few rare exceptions. In the Baltic Sea, rivers introduce high concentrations of iron and manganese. Ferromanganese nodules form as a result of summer anoxia near the seafloor with the diagenetic mobilization of manganese (Kuhn et al., 2017). As there was likely a freshwater influence at Herschel, it is possible that the clusters of manganese-rich nodules may have formed in a similar way. If this were the case, such periodic stratification of the water column would not have had a long-term detrimental effect on the bottom-dwelling communities. However, the fully marine shales that overlie the bonebed indicate a marine transgression (Figure 6C); a rise in sea-level may have initiated the development of a strongly stratified water column. This stratification would have led to the establishment of a pycnocline, separating waters of different densities, and a chemocline, separating the oxic water above from the anoxic, hydrogen sulfide-rich waters below.
The microvertebrate layer that overlies the bonebed is found in the distinct sulfur-rich layer, approximately 5 cm thick. Hydrogen sulfide is the by-product of anaerobic respiration of sulfur-reducing bacteria, and is found in association with lagoons and restricted basins in the modern world (Howarth and Teal, 1979; Goodman et al., 1995). The long period of sediment starvation during which the bonebed material was initially deposited would have left abundant sources of organic matter on the seafloor. Additionally, studies of modern whale-falls have found that one of the final phases of decay is a sulphophilic stage in which lipid deposits in the bones undergo anaerobic decomposition (Smith and Baco, 2003). Furthermore, the sulfides released during this process can accumulate and persist in the surrounding sediments (Fujiwara et al., 2007). If the waters in the silled basin became strongly stratified and the bottom waters anoxic, sulfur-reducing bacteria could have colonized the sea floor at this time.
The one cm-thick fine-grained siltstone that directly overlies the microvertebrate layer contains monospecific occurrences of the trace fossil Chondrites isp. (Figures 3B–D). Chondrites isp. burrows are also found inside the hollowed out cavities of some of the marine reptile fossils (Figures 5H,I), implying that these trace fossils formed after the bioerosion and subsequent redeposition of the main bonebed. This branching, dendritic ichnotaxon is often associated with deeper water settings, but can also be found in poorly oxygenated environments of any depth. These Chondrites isp. traces are produced by chemosymbionts that utilize the ecospace provided by oxygen poor environments (Seilacher, 1990; Bhattacharya and Banerjee, 2014). These chemosymbionts may have colonized the seafloor with the sulfur-reducing bacteria, dominating an environment where little else could live.
The microvertebrate layer is composed almost entirely of fish and shark material. This is unexpected, as the majority of the bonebed is dominated by marine reptiles. If the microvertebrate layer were deposited during a period of euxinia, it would primarily affect non-air-breathing animals. Like the Black Sea, the Framvaren Fjord in Norway has a stratified water column (Meyer and Kump, 2008). In 1942, a strong marine incursion into the fjord disrupted the chemocline, mixing the anoxic, hydrogen-sulfide rich waters into the oxic surface waters. The result was a basin-wide die-off of fish (Pakhomova et al., 2014). A similar scenario has been proposed to explain the formation of a Cenomanian-aged, fish-dominated bonebed from Western Interior Sea in eastern Saskatchewan (Schröder-Adams et al., 2001). The microvertebrate layer at Herschel may have formed in this manner, with rising sea level making the basin prone to a massive marine intrusion that disrupted the chemocline and lead to a massive die off of non-air-breathing animals.
As a final line of evidence to support this hypothesis of euxinia, relative to other Upper Cretaceous deposits across southern Saskatchewan, the Herschel marine bonebed is unusually enriched in the evaporite mineral gypsum. Gypsum (CaSO4⋅2H2O) has formed extensive evaporative beds as far back as the Archean (Cockell and Raven, 2007), and is the most common sulfur mineral (Deer et al., 1966). The gypsum deposits at Herschel are found as isolated crystals and also encrust most of the fossil material. The gypsum is most likely diagenetic, forming as a result of groundwater percolating through the sediments and precipitating crystals on the surface or forming crusts around porous material such a vertebrate bone (Murray, 1964). Gypsum is very common in Upper Cretaceous shallow marine and costal sediments in Saskatchewan, though the frequency of gypsum-encrusted fossils at Herschel is unusual. Its prevalence may suggest that the deposits are richer in sulfur compounds than elsewhere, possibly related to this euxinic event. These observations and interpretations of Dinosaur Park Formation central basin deposits are in line with those reported by Gilbert et al. (2019).
Out of a database of 383 publications on fossil bonebeds, totaling over 1,000 sites, only 30 studies discussed marine bonebeds (Eberth et al., 2007). Out of these publications on marine bonebeds, half focused solely on fishes, and only eight addressed Mesozoic marine reptiles. Since the publication of that database, additional studies have described marine reptile bonebeds (e.g., Strganac et al., 2014), but it remains clear that marine macrovertebrate bonebeds are rare in the fossil record. Any marine bonebed is therefore significant, but the site near Herschel appears to be one of the few bonebeds in the world in which plesiosaur fossils are the most abundant. Not only are plesiosaur fossils the most common in the Herschel marine bonebed, but the assemblage includes specimens across a wide range of developmental stages (e.g., Figures 5C,D). Other marine reptile fossil assemblages have been reported to contain both juveniles and adults (Wiffen et al., 1995; Kear, 2006) but not in such a dense deposit. Interestingly, other locations where abundant juvenile plesiosaur material has been found were at paleolatitudes similar or higher than that of the Herschel marine bonebed (∼57°N) (Wiffen et al., 1995; Kear, 2006; van Hinsbergen et al., 2015). The prevalence of juvenile plesiosaur fossils at this site will be the subject of future research. Additionally, to our knowledge, this is the first report of invertebrate bioerosion from a marine reptile bonebed. The bioerosion of the macrofossils at the Herschel marine bonebed is distinctive, and provides evidence that some components of the seafloor detritivore community have changed very little over the past 76 million years. As fossilization generally requires that bones be buried rapidly, out of the reach of surface-scavengers, such evidence of detritivore activity is a rare occurrence.
The Herschel marine bonebed differs from bonebeds found in other Dinosaur Park Formation/Judith River Formation – Bearpaw Formation transitions both in mode of formation and in fossil components. A marine bonebed from the Lethbridge Coal Zone predominantly contains elasmobranchs, and the only marine reptile fossils recovered are teeth, which are extremely rare from the site (Beavan and Russell, 1999). Bonebeds from the Judith River Formation predominantly contain microvertebrates, and most of these are preserved in sediments deposited in freshwater systems (Rogers and Brady, 2010). Marine fossil accumulations, which include rare large marine reptile bones (Rogers et al., 2016, 8H) have been recovered from the Woodhawk Member of the Judith River Formation. The Woodhawk Member is characterized as wave-dominated shoreface deposits, and has not been reported to contain barrier island development (Rogers and Brady, 2010; Rogers et al., 2016). This indicates variable coastal morphology along the Western Interior Seaway during the Campanian, and would directly impact marine reptile habitat potential. The localized bonebeds deposits in the Woodhawk Member also contain shell fragments, which highlights another preservational difference from the Herschel marine bonebed (Rogers et al., 2016). Despite the close contemporaneity of the Woodhawk Member and the Lethbridge Coal Zone with the Hershel marine bonebed, the disparities in lithology and fossil assemblages suggest localized variations in depositional environments across the Western Canadian Sedimentary Basin and Montana during the Late Cretaceous.
In addition to preserving a rare example of a marine macrovertebrate bonebed, the Herschel marine bonebed provides a new spatial perspective of the Dinosaur Park Formation – Bearpaw Formation transition. Significantly, these deposits near Herschel record an easternmost extension of the Dinosaur Park Formation in North America. While this transition is marked in Alberta by the Lethbridge Coal Zone, the paleobiology of which is well-studied (i.e., Beavan and Russell, 1999), and in Montana by the Woodhawk Member of the Judith River Formation (Rogers and Brady, 2010; Rogers et al., 2016), little attention has hitherto been given to understanding the transgression in Saskatchewan. The Herschel marine bonebed differs from these other sites in lithology, fossil diversity, and taphonomy. This site may aid in understanding how the transition unfolded over large spatial scales and what factors may have been driving the biodiversity at the time. This study also demonstrates that barrier island basins in the Late Cretaceous were subject to periods of water stratification and euxinia. As these factors can lead to the formation of bonebeds, it can therefore be suggested that the Dinosaur Park – Bearpaw transition may be a favorable place to look for similarly rich fossil deposits across southwestern Saskatchewan.
HS initiated the line of inquiry, investigated the bioerosion, and contributed to the paleoenvironmental interpretations. HS and EB curated the Herschel fossil collection at the RSM’s T. rex Discovery Centre. EB was involved with the 2014 excavation, identified the presence of ichnofossils at the site, described the local lithology, and contributed to the paleoenvironmental interpretations. MG measured the stratigraphic section, identified and described the trace fossil and facies associations, and contributed to the geologic and paleoenvironmental interpretations. All authors contributed to the writing of the manuscript and creation of the figures.
MG received a Dean’s Scholarship from the University of Saskatchewan.
The authors declare that the research was conducted in the absence of any commercial or financial relationships that could be construed as a potential conflict of interest.
Without the long-standing and dedicated work of Tim Tokaryk (RSM), this research would not have been possible. We wish to thank the landowners, IB and MB, for providing access to the Herschel bonebed site. Gratitude is extended to the community of Herschel, especially D and S Neufeld, and to the RM of Mountain View, for their hospitality. Thanks to the Ancient Echoes Interpretive Centre staff, especially M. Carlson and J. Cooper. Credit is also given to many members of the excavation crews, especially to D. Stoffregen, W. Long, M. Vovchuk, M. Carlson, I. Fendley, D. Bast, S. Popov, A. Kilmury, the Neufeld family, and many local volunteers. Thanks to T. Tokaryk and summer student D. Bast for cataloging the collection, and to many lab volunteers for fossil preparation. Thanks to W. Long and D. Stoffregen for the detailed quarry map. Thanks to R. Boessenecker for discussion about marine vertebrate taphonomy. Thanks to S. Johnson for sharing photographs and discussion about gastropods at modern whale-falls. Thanks finally to the four reviewers who contributed their time and suggestions to the improvement of this manuscript.
Baadsgaard, H., Lerbekmo, J. F., Wijbrans, J. R., Swisher, C. C. III, and Fanning, M. (1992). Multimethod radiometric age for a bentonite near the top of the Baculites reesidei zone of southwestern Saskatchewan (Campanian- Maastrichtian stage boundary?). Can. J. Earth Sci. 30, 769–775. doi: 10.1139/e93-063
Baco, A. R., and Smith, C. R. (2003). High species richness in deep-sea chemoautotrophic whale skeleton communities. Mar. Ecol. Prog. Ser. 260, 109–114. doi: 10.3354/meps260109
Bamforth, E. L., Button, C. L., and Larsson, H. C. E. (2014). Paleoclimate estimates and fire ecology immediately prior to the end-Cretaceous mass extinction in the Frenchman Formation (66Ma), Saskatchewan, Canada. Palaeogeogr. Palaeoclimatol. Palaeoecol. 401, 96–110. doi: 10.1016/j.palaeo.2014.02.020
Beavan, N. R., and Russell, A. P. (1999). An elasmobranch assemblage from the terrestrial-marine transitional Lethbridge Coal Zone (Dinosaur Park Formation: upper Campanian), Alberta, Canada. J. Paleontol. 73, 494–503. doi: 10.1017/S0022336000028006
Belaústegui, Z., de Gibert, J. M., Domènech, R., Muñiz, F., and Martinell, J. (2012). Clavate borings in a Miocene cetacean skeleton from Tarragona (NE Spain) and the fossil record of marine bone bioerosion. Palaeogeogr. Palaeoclimatol. Palaeoecol. 32, 68–74. doi: 10.1016/j.palaeo.2012.01.031
Bhattacharya, B., and Banerjee, S. (2014). Chondrites isp. indicating late paleozoic atmospheric anoxia in eastern peninsular India. Sci. World J. 2014, 434672. doi: 10.1155/2014/434672
Boessenecker, R. W., and Fordyce, R. E. (2014). Trace fossil evidence of predation upon bone-eating worms on a baleen whale skeleton from the Oligocene of New Zealand. Lethaia 48, 326–331. doi: 10.1111/let.12108
Boessenecker, R. W., Perry, F. A., and Schmitt, J. G. (2014). Comparative taphonomy, taphofacies, and bonebeds of the Mio-Pliocene Purisima Formation, Central California: strong physical control on marine vertebrate preservation in shallow marine settings. PLoS One 9:e91419. doi: 10.1371/journal.pone.0091419
Braby, C. E., Rouse, G. W., Johnson, S. B., Jones, W. J., and Vrijenhoek, R. C. (2007). Bathymetric and temporal variation among Osedax boneworms and associated megafauna on whale-falls in Monterey Bay, California. Deep. Res. Part I Oceanogr. Res. Pap. 54, 1773–1791. doi: 10.1016/j.dsr.2007.05.014
Brown, D. S. (1981). The English Upper Jurassic Plesiosauroidea (Reptilia) and a review of the phylogeny and classification of the Plesiosauria. Bull. Br. Museum Nat. Hist. 35, 253–347.
Cioffi, F., Di Eugenio, A., and Gallerano, F. (1995). A new representation of anoxic crises in hypertrophic lagoons. Appl. Math. Model. 19, 685–695. doi: 10.1016/0307-904X(95)00075-U
Cockell, C. S., and Raven, J. A. (2007). Ozone and life on the Archaean Earth. Philos. Trans. R. Soc. A Math. Phys. Eng. Sci. 365, 1889–1901. doi: 10.1098/rsta.2007.2049
Dahlgren, T. G., Wiklund, H., Källström, B., Lundälv, T., Smith, C. R., and Glover, A. G. (2006). A shallow-water whale-fall experiment in the north Atlantic. Cah. Biol. Mar. 47, 385–389.
Danise, S., and Higgs, N. D. (2015). Bone-eating Osedax worms lived on Mesozoic marine reptile deadfalls. Biol. Lett. 11, 1–5. doi: 10.1098/rsbl.2015.0072
Danise, S., Twitchett, R. J., and Matts, K. (2014). Ecological succession of a Jurassic shallow-water ichthyosaur fall. Nat. Commun. 5, 1–8. doi: 10.1038/ncomms5789
Dawson, F. M., Evans, C. G., Marsh, R., and Richardson, R. (1994). “Uppermost Creataceous and Tertiary strata of the Western Canada sedimentary basin,” in Geological Atlas of the Western Canada Sedimentary Basin, eds G. Mossop, and I. Shetsen, (Edmonton, AB: Canadian Society of Petroleum Geologists and the Alberta Research Council), 387–407.
Deer, W. A., Howie, R. A., and Zussman, J. (1966). An Introduction to the Rock-Forming Minerals. London: Longman.
Eberth, D. A., and Hamblin, A. P. (1993). Tectonic, stratigraphic, and sedimentologic significance of a regional discontinuity in the upper Judith River Group (Belly River wedge) of southern Alberta, Saskatchewan, and northern Montana. Can. J. Earth Sci. 30, 174–200. doi: 10.1139/e93-016
Eberth, D. A., Shannon, M., and Norland, B. G. (2007). “A bonebeds database: classification, biases, and atterns of occurrence,” in Bonebeds: Genesis, Analysis, and Paleobiological Significance, eds R. R. Rogers, D. A. Eberth, and A. R. Fiorillo, (Chicago, IL: Chicago University Press), 499.
Fujiwara, Y., Kawato, M., Yamamoto, T., Yamanaka, T., Sato-Okoshi, W., Noda, C., et al. (2007). Three-year investigations into sperm whale-fall ecosystems in Japan. Mar. Ecol. 28, 219–232. doi: 10.1111/j.1439-0485.2007.00150.x
Gilbert, M., Buatois, L., and Renaut, R. (2019). Ichnology and depositional environments of the Upper Cretaceous Dinosaur Park - Bearpaw formation transition in the Cypress Hills region of Southwestern Saskatchewan. Cretac. Res. 98, 189–210. doi: 10.1016/j.cretres.2018.12.017
Gilbert, M. M., Bamforth, E. L., Buatois, L. A., and Renaut, R. W. (2018). Paleoecology and sedimentology of a vertebrate microfossil assemblage from the easternmost Dinosaur Park Formation (Late Cretaceous, Upper Campanian) Saskatchewan, Canada: reconstructing diversity in a coastal ecosystem. Palaeogeogr. Palaeoclimatol. Palaeoecol. 495, 227–244. doi: 10.1016/j.palaeo.2018.01.016
Goodman, J. L., Moore, K. A., and Dennison, W. C. (1995). Photosynthetic responses of eelgrass (Zostera marina L.) to light and sediment sulfide in a shallow barrier island lagoon. Aquat. Bot. 50, 37–47. doi: 10.1016/0304-3770(94)00444-Q
Haglund, W. D., and Sorg, M. H. (2002). “Human remains in water environments,” in Advances in Forensic Taphonomy: Method, Theory, and Archaeological Perspectives, eds W. D. Haglund, and M. H. Sorg, (Boca Raton: CRC Press), 201–218. doi: 10.1201/9781420058352-13
Harzallah, A., and Chapelle, A. (2002). Contribution of climate variability to occurrences of anoxic crises “malaïgues” in the Thau lagoon (southern France). Oceanol. Acta 25, 79–86. doi: 10.1016/S0399-1784(02)01184-2
Higgs, N. D., Glover, A. G., Dahlgren, T. G., and Little, C. T. S. (2010). Using computed-tomography to document borings by Osedax mucofloris in whale bone. Cah. Biol. Mar. 51, 401–405.
Higgs, N. D., Little, C. T. S., Glover, A. G., Dahlgren, T. G., Smith, C. R., and Dominici, S. (2012). Evidence of Osedax worm borings in Pliocene (~3 Ma) whale bone from the Mediterranean. Hist. Biol. An Int. J. Paleobiol. 24, 269–277. doi: 10.1080/08912963.2011.621167
Howarth, R. W., and Teal, J. M. (1979). Sulfate reduction in a New England salt marsh. Limnol. Oceanogr. 24, 999–1013. doi: 10.4319/lo.1979.24.6.0999
Ito, T., and Komuro, K. (2006). Paleoceanographic setting and reservation of buried manganese deposits in DSDP/ODP Cores. Resour. Geol. 56, 457–470. doi: 10.1111/j.1751-3928.2006.tb00298.x
Johnson, S. B., Warén, A., Lee, R. W., Kano, Y., Kaim, A., Davis, A., et al. (2010). Rubyspira, new genus and two new species of bone-eating deep-sea snails with ancient habits. Biol. Bull. 219, 166–177. doi: 10.1086/bblv219n2p166
Kaim, A., Kobayashi, Y., Echizenya, H., Jenkins, R. G., and Tanabe, K. (2008). Chemosynthesis-based associations on Cretaceous plesiosaurid carcasses. Acta Palaeontol. Pol. 53, 97–104. doi: 10.4202/app.2008.0106
Kauffman, E. G., and Caldwell, W. G. E. (1993). “The Western interior basin in space and time,” in Evolution of the Western Interior Basin, eds W. G. E. Caldwell, and E. G. Kauffman, (St. John’s: Geological Association of Canada), 1–30.
Kear, B. P. (2006). Marine reptiles from the Lower Cretaceous of South Australia: elements of a high-latitude cold-water assemblage. Palaeontology 49, 837–856. doi: 10.1111/j.1475-4983.2006.00569.x
Kiel, S., Goedert, J. L., Kahl, W.-A., and Rouse, G. W. (2010). Fossil traces of the bone-eating worm Osedax in early Oligocene whale bones. Proc. Natl. Acad. Sci. U.S.A. 107, 8656–8659. doi: 10.1073/pnas.1002014107
Kiel, S., Kahl, W., and Goedert, J. L. (2011). Osedax borings in fossil marine bird bones. Naturwissenschaften 98, 51–55. doi: 10.1007/s00114-010-0740-5
Kiel, S., Kahl, W.-A., and Goedert, J. L. (2013). Traces of the bone-eating annelid Osedax in Oligocene whale teeth and fish bones. Paläontol. Z. 87, 161–167. doi: 10.1007/s12542-012-0158-9
Kuhn, T., Wegorzewski, A. V., Rühlemann, C., and Vink, A. (2017). “Composition, formation, and occurrence of polymetallic nodules,” in Deep-Sea Mining: Resource Potential, Technical and Environmental Considerations, ed. R. Sharma, (Basel: Springer International Publishing), 23–63. doi: 10.1007/978-3-319-52557-0_2
Lundsten, L., Schlining, K. L., Frasier, K., Johnson, S. B., Kuhnz, L. A., Harvey, J. B. J., et al. (2010). Time-series analysis of six whale-fall communities in Monterey Canyon, California, USA. Deep. Res. Part I Oceanogr. Res. Pap. 57, 1573–1584. doi: 10.1016/j.dsr.2010.09.003
Martin, J. E., Sawyer, J. F., Reguero, M., and Case, J. A. (2007). “Occurrence of a young elasmosaurid plesiosaur skeleton from the late Cretaceous (Maastrichtian) of Antarctica,” in Proceedimgs of the 10th international Symposium on Antartic earth sciences. Antarctica: A Keystone a Changing World. USGS Open-File Report 2007-1047 Short Research Paper. 066, (Santa Barbara, CA), 1–4. doi: 10.3133/of2007-1047.srp066
McLean, J. R. (1971). Stratigraphy of the Upper Cretaceous Judith River Formation in the Canadian Great Plains. Saskatoon, SK: Saskatchewan Research Council.
Meyer, K. M., and Kump, L. R. (2008). Oceanic euxinia in earth history: causes and consequences. Annu. Rev. Earth Planet. Sci. 36, 251–288. doi: 10.1130/0091-761319910192.3.CO;2
Middelburg, J. J., Calvert, S. E., and Karlin, R. (1991). Organic-rich transitional facies in silled basins: response to sea-level change. Geology 19, 679–682.
Muñiz, F., de Gibert, J. M., and Esperante, R. (2010). First trace-fossil evidence of bone-eating worms in whale carcasses. Palaios 25, 269–273. doi: 10.2110/palo.2009.p09-112r
Murray, R. C. (1964). Origin and diagenesis of gypsum and anhydrite. J. Sediment. Petrol. 34, 512–523. doi: 10.1306/74D710D2-2B21-11D7-8648000102C1865D
Nägler, T. F., Neubert, N., Böttcher, M. E., Dellwig, O., and Schnetger, B. (2011). Molybdenum isotope fractionation in pelagic euxinia: evidence from the modern Black and Baltic Seas. Chem. Geol. 289, 1–11. doi: 10.1016/j.chemgeo.2011.07.001
Neubauer, S. C., Anderson, I. C., Constantine, J. A., and Kuehl, S. A. (2002). Sediment deposition and accretion in a mid-Atlantic (U.S.A.) tidal freshwater marsh. Estuar. Coast. Shelf Sci. 54, 713–727. doi: 10.1006/ecss.2001.0854
Pakhomova, S., Braaten, H. F., Yakushev, E., and Skei, J. (2014). Biogeochemical consequences of an oxygenated intrusion into an anoxic fjord. Geochem. Trans. 15:5. doi: 10.1186/1467-4866-15-5
Pobiner, B. L. (2008). Paleoecological information in predator tooth marks. J. Taphon. 6, 373–397. doi: 10.1093/clinids/23.3.454
R Core Team, (2018). R A Language Environment Statistical Computing. Vienna: R Foundation for Statistical Computing.
Rodriguez, A. B., and Meyer, C. T. (2006). Sea-level variation during the Holocene deduced from the morphologic and stratigraphic evolution of Morgan Peninsula, Alabama, USA. J. Sediment. Res. 76, 257–269. doi: 10.2110/jsr.2006.018
Rogers, R. R., and Brady, M. E. (2010). Origins of microfossil bonebeds: insights from the upper Cretaceous Judith River formation of north-central Montana. Paleobiology 36, 80–112. doi: 10.1666/0094-8373-36.1.80
Rogers, R. R., and Kidwell, S. M. (2007). “A conceptual framework for the genesis and analysis of vertebrate skeletal concentrations,” in Bonebeds: Genesis, Analysis, and Paleobiological Significance, eds R. R. Rogers, D. A. Eberth, and A. R. Fiorillo, (Chicago, IL: Chicago University Press), 499.
Rogers, R. R., Kidwell, S. M., Deino, A. L., Mitchell, J. P., Nelson, K., and Thole, J. T. (2016). Age, correlation, and lithostratigraphic revision of the Upper Cretaceous (Campanian) Judith River Formation in its type area (north-central Montana), with a comparison of low- and high-accommodation alluvial records. J. Geol. 124, 99–135. doi: 10.1086/684289
Sato, T. (2005). A new polycotylid plesiosaur (Reptilia: Sauropterygia) from the upper Cretaceous Bearpaw formation in Saskatchewan, Canada. J. Paleontol. 79, 969–980. doi: 10.1666/0022-3360(2005)079
Schröder-Adams, C. J., Cumbaa, S. L., Bloch, J., Leckie, D. A., Craig, J., Seif El-Dein, S. A., et al. (2001). Late Cretaceous (Cenomanian to Campanian) paleoenvironmental history of the Eastern Canadian margin of the Western Interior Seaway: bonebeds and anoxic events. Palaeogeogr. Palaeoclimatol. Palaeoecol. 170, 261–289. doi: 10.1016/S0031-0182(01)00259-0
Schwimmer, D. R., Stewart, J. D., and Dent Williams, G. (1997). Scavenging by sharks of the genus Squalicorax in the late Cretaceous of North America. Palaios 12, 71–83. doi: 10.2307/3515295
Seilacher, A. (1990). Aberrations in bivalve evolution related to photo- and chemosymbiosis. Hist. Biol. 3, 289–311. doi: 10.1080/08912969009386528
Smith, C. R., and Baco, A. R. (2003). Ecology of whale falls at the deep-sea floor. Oceanogr. Mar. Biol. 41, 311–354. doi: 10.1136/thx.2004.039818
Strganac, C., Jacobs, L. L., Polcyn, M. J., Mateus, O., Myers, T. S., Salminen, J., et al. (2014). Geological setting and paleoecology of the upper Cretaceous Bench 19 marine vertebrate bonebed at Bentiaba, Angola. Netherlands J. Geosci. 94, 121–136. doi: 10.1017/njg.2014.32
Stumpf, R. P. (1983). The process of sedimentation on the surface of a salt marsh. Estuar. Coast. Shelf Sci. 17, 495–508. doi: 10.1016/0272-7714(83)90002-1
Tapanila, L., Roberts, E. M., Bouaré, M. L., Sissoko, F., and O’Leary, M. A. (2004). Bivalve borings in phosphatic coprolites and bone, Cretaceous – Paleogene, northeastern Mali. Palaios 19, 565–573. doi: 10.1669/0883-13512004019<0565
Timmons, E. A., Rodriguez, A. B., Mattheus, C. R., and DeWitt, R. (2010). Transition of a regressive to a transgressive barrier island due to back-barrier erosion, increased storminess, and low sediment supply: Bogue Banks, North Carolina, USA. Mar. Geol. 278, 100–114. doi: 10.1016/j.margeo.2010.09.006
Tsujita, C. J. (1995). Origin of concretion-hosted shell clusters in the late Cretaceous Bearpaw formation, southern Alberta, Canada. Palaios 10, 408–423.
U.S. Geological Survey, (2006). FGDC Digital Cartographic Standard for Geologic Map Symbolization (PostScript Implementation). Reston, VA: U.S. Geological Survey.
Usui, A., and Ito, T. (1994). Fossil manganese deposits buried within DSDP/ODP cores, Legs 1–126. Mar. Geol. 119, 111–136. doi: 10.1016/0025-3227(94)90144-9
van Hinsbergen, D. J. J., de Groot, L. V., van Schaik, S. J., Spakman, W., Bijl, P. K., Sluijs, A., et al. (2015). A paleolatitude calculator for paleoclimate studies. PLoS One 10:e0126946. doi: 10.1371/journal.pone.0126946
Keywords: anoxia, bioerosion, bonebed, Campanian, ichnofossil, marine reptile, marine transgression, Western Interior Sea
Citation: Street HP, Bamforth EL and Gilbert MM (2019) The Formation of a Marine Bonebed at the Upper Cretaceous Dinosaur Park - Bearpaw Transition of West - Central Saskatchewan, Canada. Front. Earth Sci. 7:209. doi: 10.3389/feart.2019.00209
Received: 16 November 2018; Accepted: 29 July 2019;
Published: 13 August 2019.
Edited by:
K. Christopher Beard, The University of Kansas, United StatesReviewed by:
Spencer G. Lucas, New Mexico Museum of Natural History and Science, United StatesCopyright © 2019 Street, Bamforth and Gilbert. This is an open-access article distributed under the terms of the Creative Commons Attribution License (CC BY). The use, distribution or reproduction in other forums is permitted, provided the original author(s) and the copyright owner(s) are credited and that the original publication in this journal is cited, in accordance with accepted academic practice. No use, distribution or reproduction is permitted which does not comply with these terms.
*Correspondence: Hallie P. Street, aGFsbGllLnN0cmVldEBnb3Yuc2suY2E=; aHN0cmVldEB1YWxiZXJ0YS5jYQ==
Disclaimer: All claims expressed in this article are solely those of the authors and do not necessarily represent those of their affiliated organizations, or those of the publisher, the editors and the reviewers. Any product that may be evaluated in this article or claim that may be made by its manufacturer is not guaranteed or endorsed by the publisher.
Research integrity at Frontiers
Learn more about the work of our research integrity team to safeguard the quality of each article we publish.