- School of Marine and Atmospheric Sciences, Stony Brook University, Stony Brook, NY, United States
Bioturbation promotes priming and total remineralization of sedimentary organic matter (Corg) in multiple ways. A primary local mode is the injection of reactive Corg from the water column, surface sediment, and mucus secretions into deposits. During feeding, burrowing, and construction activities by benthic fauna, labile substrates are brought into close association with more refractory material over a wide range of time scales, geometries, and depths, enhancing decomposition of the less reactive components (priming). One measure of these local interactions is the particle mixing coefficient, DB, which can be estimated from the averaged penetration of particle-reactive radionuclides into deposits. Patterns of DB in Long Island Sound, an estuarine system with well-defined sources of naturally occurring radionuclides, show consistent positive correlations between DB and total inventories of excess 234Th (t1/2 = 24 days) and 210Pb (t1/2 = 22 years) at local and basin scales. These correlations, maintained seasonally in the case of 234Th, demonstrate not only the penetration of plankton-derived, reactive Corg into deeper regions of deposits during bioturbation over monthly (∼5–10 cm) to decadal timescales (∼20–100 cm) but also the enhanced capture of labile substrates from the water column across basin scales into bioturbated patches as the intensity of reworking increases. In Long Island Sound, sedimentary Chl-a distributions and benthic nutrient regeneration (e.g., NH4+ fluxes) reflect these particle exchange processes. Basin and regional scale capture of labile substrates into bioturbated deposits can be generally demonstrated, for example, along the highly productive Cape Hatteras continental margin. Thus, total and net remineralization necessarily increase with the biogenic enhancement of the quantity of labile particulate substrate in deposits. This capture, intermixing, and close association of reactive and refractory substrates (reductant blending), and thus the optimization of priming potential, represent important, often overlooked, pathways by which bioturbation generates biogeochemical conditions conducive to maximum efficiency of remineralization.
Introduction
Benthic fauna influence the cycling of sedimentary organic matter in multiple ways, generally stimulating conditional remineralization rates through modification of transport – reaction conditions in deposits, direct respiration, and the formation of new labile biomass, but sometimes inhibiting decomposition through the production of refractory material or toxic compounds (Aller et al., 2001; Kristensen and Kostka, 2005). One major consequence of macro- and meiofaunal activity is the constant redistribution, packaging or fragmentation, and re-exposure of reactive and refractory sedimentary Corg within deposits. During feeding, burrowing, and construction activities by benthic fauna, relatively labile substrates are brought into close association with more refractory material over a wide range of time scales, depth scales, and geometries. For example, particles of different reactivity and origin can be intermixed randomly in a diffusion-like manner (Gerino et al., 1998; Meysman et al., 2003). In other cases, surface-derived, labile Corg can be injected into excavated subsurface pockets as highly reactive fill surrounded by relatively refractory material (Aller and Aller, 1986; Wanless et al., 1988; Smith et al., 1996). Particles of varied size and reactivity can be also be specifically segregated, coated with mucus secretions, and organized into distinct structures, for example, burrow, tube walls, or tracks and trails (Pillay and Branch, 2011; Hannides and Aller, 2016). These types of effects and their relative importance depend on population density, size distributions, and species-specific behaviors (Rhoads, 1974; François et al., 2002; Solan et al., 2008). In general, the bringing together of relatively labile and more refractory organic matter within bioturbated deposits: reductant mixing, must promote the phenomenon referred to as priming. Priming is defined by the enhanced remineralization (typically ∼10–30%) of otherwise low-reactivity organics (refractory) in association with the decomposition of relatively labile organic material in terrestrial soils, aquatic sediments, and natural waters (Löhnis, 1926; Stevenson, 1986; Graf, 1992; Hee et al., 2001; van Nugteren et al., 2009; Bianchi et al., 2015).
In addition to modification of particle interactions and reactivity distributions within deposits, benthic fauna can affect sediment mass properties by enhancing or reducing susceptibility of deposits to erosion, or by promoting accumulation of sediment through particle capture and biodeposition (Eckman et al., 1981; Rhoads and Boyer, 1982). Thus, depending on their functional group composition (e.g., feeding type, mobility, life habit) and population density, benthic communities can affect sediment resuspension, lateral transport and water column particle dynamics over basin scales for a given physical boundary layer and circulation regime. The coupling of vertical transport of particles within deposits with the horizontal exchange of particles between sedimentary facies, results in continuous interactions of organic matter having wide variations in reactivity. These interactions can vary temporally and spatially in intensity. Benthic communities and associated bioturbation are thus intimately involved in promoting the conditions necessary for extensive priming interactions at local, facies, and basin scales.
Here, we examine interactions between reactive particle pools and particle dynamics as they relate to sedimentary facies and benthic communities both locally and across basinal scales using as a primary example Long Island Sound, an estuarine lagoonal system on the Northeast coast of N. America (Figure 1). We summarize and utilize the distributions of the natural radionuclides 234Th, 210Pb, and 14C, and correlations with sedimentary reactive Chl-a and benthic nutrient fluxes, to track and reveal particle exchange behavior and age distributions, the latter correlating directly with organic C (Corg) reactivity (Middelburg, 1989). These patterns illustrate coupled biological and physical processes governing interactions between particle pools associated with organic matter having widely varying reactivity and thus priming potential. The LIS data are historical (1974–1993) and, in addition to illustrating fundamental processes, represent a reference to which subsequent studies of possible progressive changes can be compared. Examples from the Peconic Bay estuary system (Long Island) and from the Cape Hatteras continental margin off North Carolina are also used to further elucidate controls and to generalize the role of bioturbation in promoting particle dynamics and priming at local and depositional system scales.
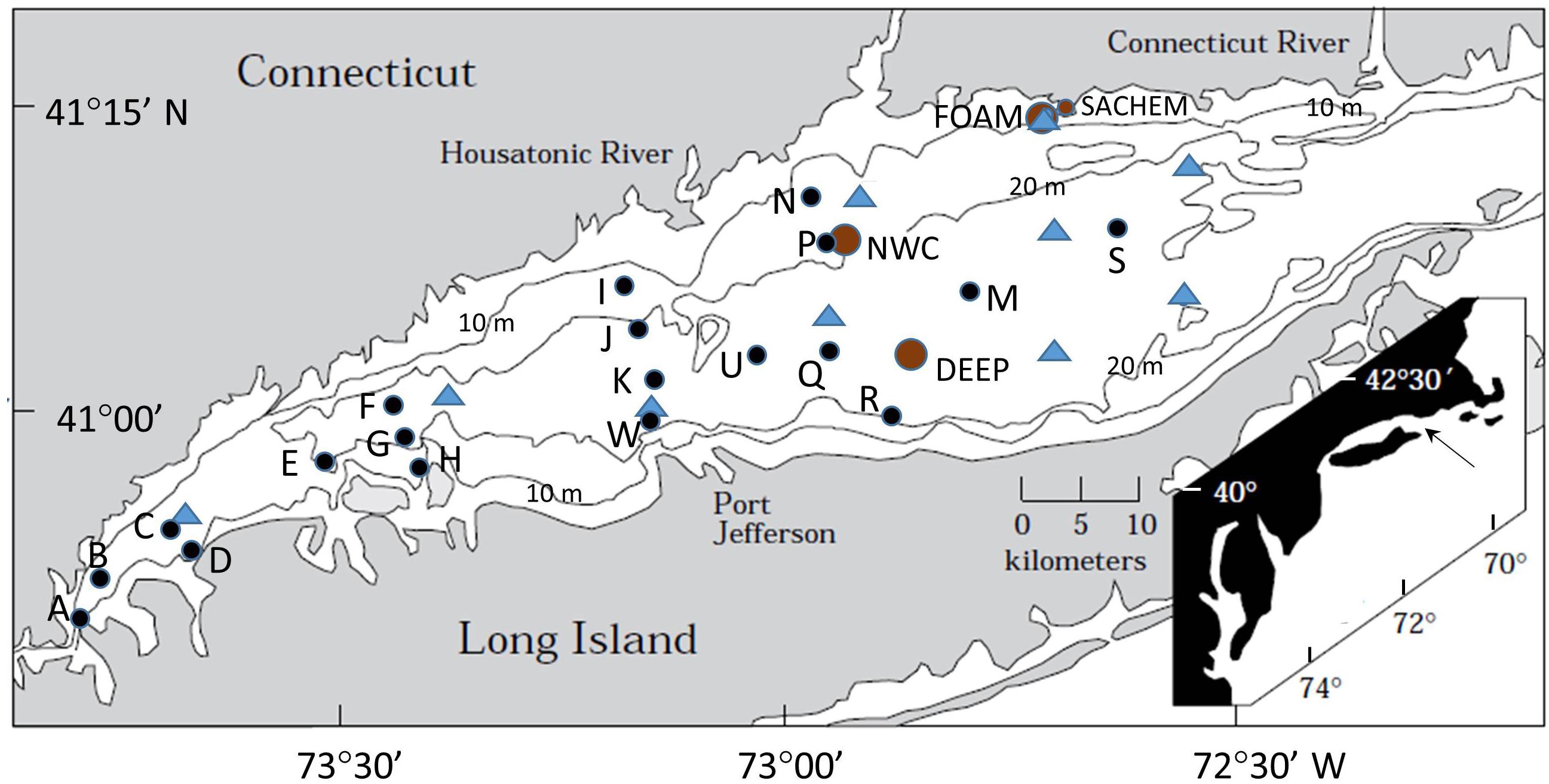
Figure 1. Map of Long Island Sound (LIS) showing locations of bottom sampling stations. Long-term study sites in the central basin: SACHEM, FOAM, NWC, and DEEP are indicated with circles (brown). August 1977 survey sites are marked by triangles (blue). The 19 Long Island Sound Study (LISS) sites are indicated by small solid circles (black) sampled seasonally for 1 year, or in the case of reference sites (A, B, G, P, R, S) over a 2 year period. The PULSE site is located near P (Gerino et al., 1998).
We do not address in this contribution, the multiple additional impacts bioturbation has on remineralization processes (e.g., Aller et al., 2001; Kristensen and Kostka, 2005). For example, Corg decomposition in bioturbated deposits is enhanced by injection of metal oxides during particle reworking, provision of O2, SO42- and local generation of additional metal oxides during irrigation, and overall increased metabolite exchange. The episodic exposure to O2, a virtually unlimited supply of additional high order oxidants, and the minimized build-up of metabolites, result in oscillating redox conditions over multiple frequencies and durations, and increased remineralization. Thus, bioturbation not only promotes intermixing and association of reactive and refractory substrates (reductant mixing) and thus priming potential, but typically generates a range of biogeochemical conditions conducive to maximum efficiency of remineralization within sedimentary deposits.
Background
Long Island Sound is an W-E trending estuarine basin along NE North America, with dimensions approximately ∼150 km long, ∼30 km maximum width, and ∼18 m average depth, the latter estimate ranging to ∼24 m depending on exactly where boundaries are drawn (e.g., Bokuniewicz and Gordon, 1980). Morphologically it is characterized by four primary regions referred to as the Narrows, Western, Central, and Eastern basins, the latter separated by relict coastal plain and glacial deposits that form ∼ N-S trending shoals (Figure 2A). The physical oceanography and hydrography have been recently summarized by O’Donnell et al. (2014): The primary source of fresh water to the Sound is the Connecticut River, located near the eastern end of the system. Thus, the Sound is an atypical estuary from the perspective of primary river input location. Tidal exchange occurs at the western end with the Hudson River through the East River channel, and at the eastern end with Block Island Sound and the Atlantic Ocean through the Race (Figure 1). Mean tidal range (semi-diurnal) increases east to west, varying from ∼0.84 to 2.2 m. Cyclonic gyres are present in the three primary basins, and there is a tendency for residual westward flow along the shallow north coast (Connecticut) and deeper water, and eastward flow along the south coast (Long Island). Water exchange is greatest in the east and decreases to the west. As a result of these circulation patterns and enhanced primary production related to anthropogenic nutrient inputs, the Western Narrows is typically hypoxic in late summer. Seasonal surface temperature ranges from ∼0 to 24°C, and salinity from ∼25 to 27 in the west and ∼29 to 31 in the east, with spatial and temporal gradients depending on vicinity to rivers and season.
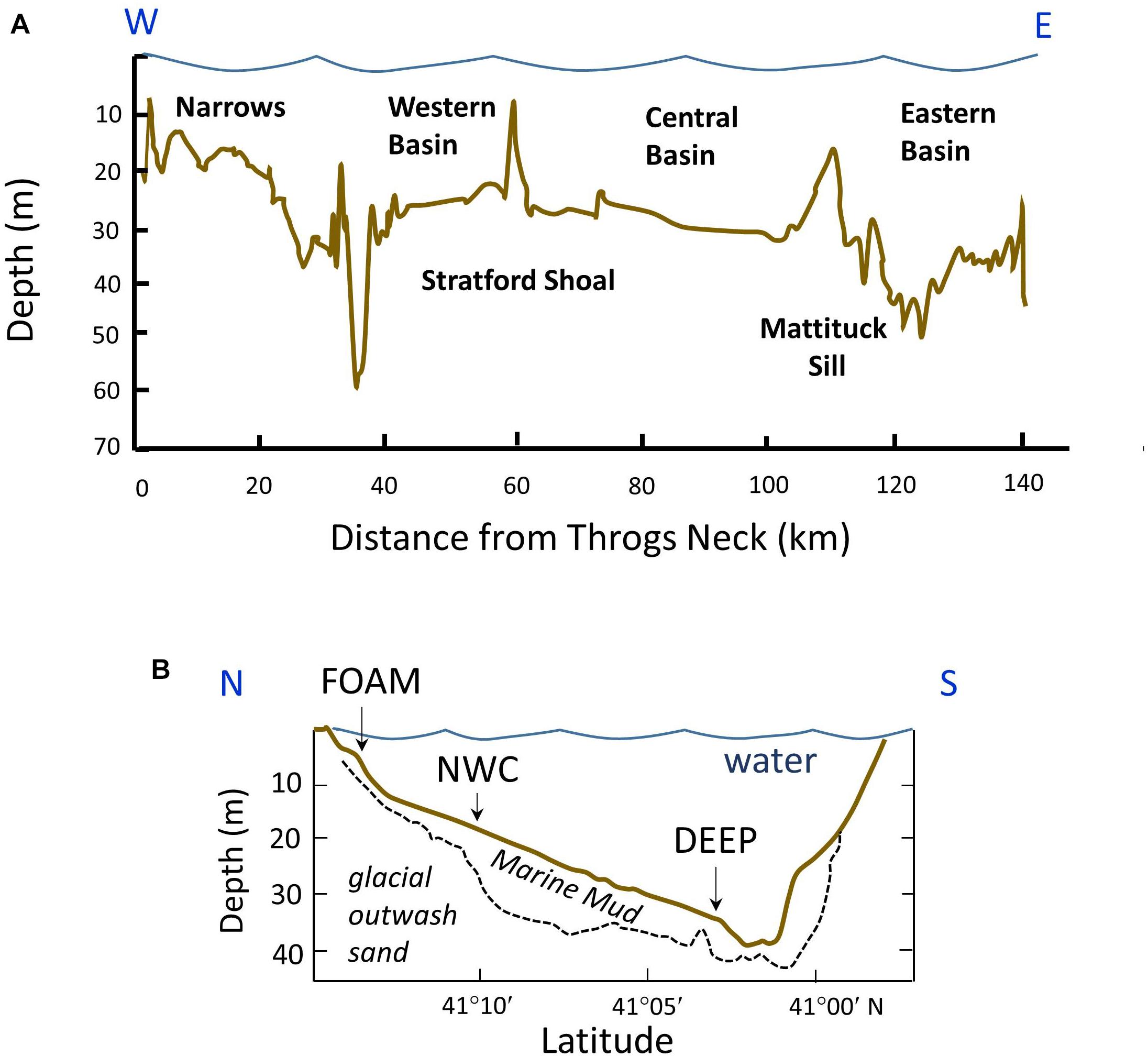
Figure 2. (A) W-E bathymetric profile along the axis of LIS indicating major shoal and basin features (modified after Auster et al., 2009). (B) N-S cross section of central basin through approximately 72° 53′ W with seismically determined thickness of marine mud outlined (post ∼13 kyr; modified after Bokuniewicz et al., 1976). The long-term sampling stations in the central basin are projected onto this plane to illustrate relative bathymetry and deposition rates (∼0.03–0.08 mm year-1).
The sediments in the Sound generally fine westward, with sands, gravel, migrating bedforms, and erosive regions characterizing the eastern boundary, and muds characterizing much of the more western basin regions (Bokuniewicz and Gordon, 1980; Knebel and Poppe, 2000; Poppe et al., 2000). There is considerable heterogeneity, however; and areas of erosion, redistribution, and deposition can be in close proximity (Knebel and Poppe, 2000). The long term sediment accumulation rates measured seismically or estimated from 14C range from ∼0.03 to 0.08 mm year-1 (Bokuniewicz and Gordon, 1980; Lewis and DiGiacomo-Cohen, 2000; Cuomo et al., 2014; Figure 2B). The modern 210Pb and long-term sediment budgets indicate that there is only minor net import of sediment from adjacent shelf regions, and that particle sources are largely from the Connecticut coastline rivers, primarily from the Connecticut River, and internally redistributed debris (Benninger, 1978; Lewis and DiGiacomo-Cohen, 2000). Although net accumulation rates of sediment are generally low, particles are continuously resuspended and refluxed by currents, waves, and episodic storm events, and small particles can move throughout the Sound. The muddy basin regions of the Sound are emphasized here.
Benthic faunal distributions and activity track the sedimentary depositional environments, intensity of physical disturbance, and, particularly in the Western Narrows, seasonal hypoxia (Sanders, 1956; McCall, 1977, 1978; Zajac et al., 2000, 2013; Cuomo et al., 2014). In general, sediments in Long Island Sound are intensely bioturbated, although nearshore shallow regions (<10 m) and eastern sands are often physically reworked by tidal currents, waves, and episodic storms (McCall, 1977, 1978; Aller et al., 1980; Bokuniewicz and Gordon, 1980; Lewis and DiGiacomo-Cohen, 2000). Muddy deposits are commonly characterized by the deposit-feeding protobranch bivalves Nucula annulata and Yoldia limatula, the opportunistic suspension feeding bivalve Mulina lateralis, and the polychaete Nephtys incisa (Sanders, 1956; Reid et al., 1979; Zajac et al., 2000, 2013), with protobranchs in particular intensively reworking the upper 0–5 cm of deposits (Rhoads and Young, 1970). Although the abundance of individual species varied, the protobranch bivalve – Nephtys community was stably present from the 1950s to at least the mid-1985s, the time of the last synoptic benthic survey (Zajac et al., 2000). In central basin sandy silts, deeper burrowing infauna such as the polychaetes Sabaco elongata, Clymenella torquata, Melinna cristata, and Pherusa affinis are also common. The mantis shrimp Squilla empusa, which burrows 1–3 m into the seabed (Myers, 1979), can be readily observed by direct observation of the seabed (e.g., diving) and its activities evidenced by sedimentary structures (Benninger et al., 1979; Aller, 1980) but it, and other deeply burrowing species (>20 cm), are unreliably reported in standard benthic surveys. Qualitative depictions of a subset of these fauna and their typical life habits at central basin sites are illustrated in Figure 3. Except in regions of migrating bedforms, immediately following current scour or storm events (<15 m water depth), or during periods of hypoxia (Narrows; methane bubbles are present during hypoxic periods), surface deposits throughout most of Long Island Sound are dominated by bioturbation activity and biogenic sedimentary structures (Figure 4).
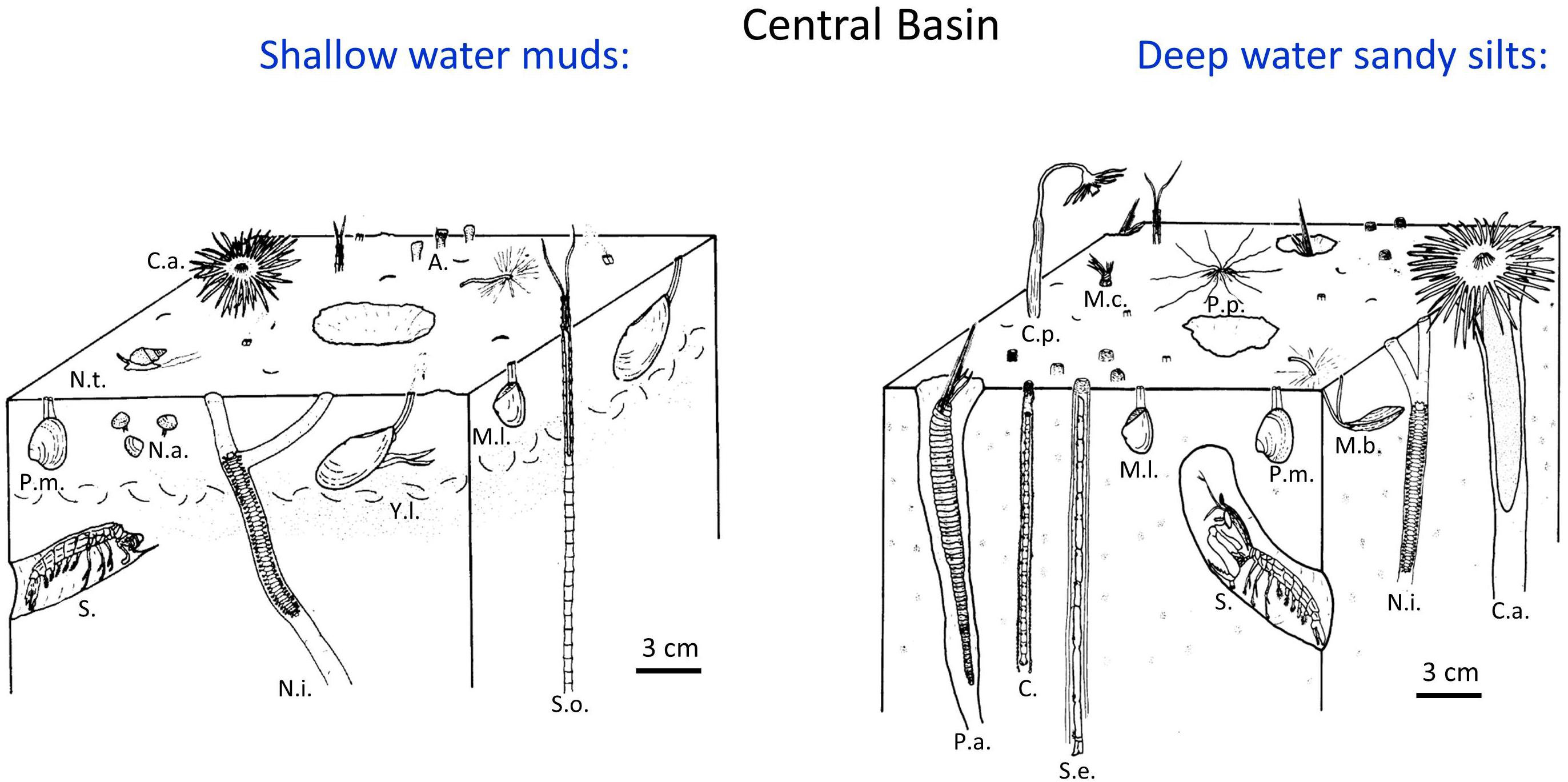
Figure 3. Schematic illustrations of major faunal components and life habits of benthic communities typically found in shallow water (<20 m) and deep water (>20 m) regions of the central basin of LIS (adapted from Aller, 1980). The deeper water regions of the central basin are often characterized by deeper feeding and deeper burrowing species. Abbreviations: Y. l., Yoldia limatula; N.a., Nucula annulata; N.i., Nephtys incisa; M.l., Mulinia lateralis; P.m., Pitar morrhuana; C.a., Ceriantheopsis americanus; N.t., Nassarius trivitatus; S.o., Spiochaetopterus oculatus; P.a., Pherusa affinis; C.p., Corymorpha pendula; M.c., Melinna cristata; C., Clymenella sp.; S.e., Sabaco elongata (drawn with some segments missing because of length); P.p., Pista palmata; S., Squilla empusa; A., Ampelisca; M.t., Macoma tenta. Biogenic structures formed by several of these species are illustrated in x-radiographs (Figure 4). A biogenic shell layer (illustrated example) or heavy mineral layer often forms as a feeding lag deposit in the presence of Yoldia (Rhoads and Young, 1970).
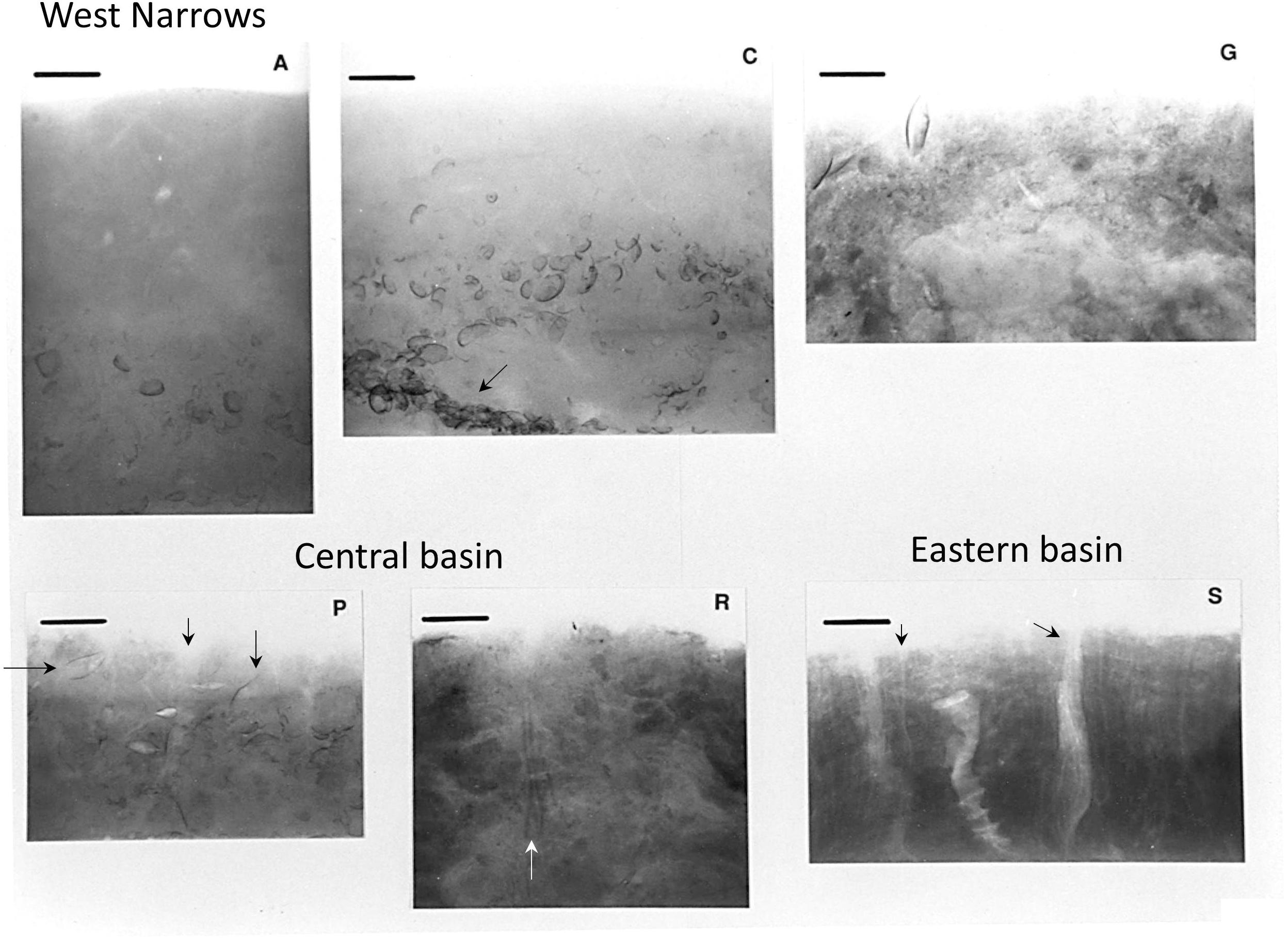
Figure 4. X-radiographs of upper 10–15 cm of sediment at LISS reference stations (Figure 1; May–June 1988). Bioturbate structure dominates at all stations. Shell layers and aggregations (stations C, G; dominantly shells of Mulinia lateralis; black arrow station C) reflect both biogenic and physical sorting processes. Tubes of the parchment worm Chaetopterus (not shown in Figure 3) and dense aggregations of Clymenella tubes are evident at station S (black arrows); Yoldia in life position and a Ceriantheopsis tube are shown at station P (black arrows); a section of a Sabaco tube is indicated at station R (white arrow). Scale bars = 3 cm.
Sampling and Analytical Methods
The data in this synthesis come from multiple studies and were acquired primarily from sites throughout Long Island Sound but with an emphasis on the Central Basin and stations along the W-E axis (Figures 1, 2). Sediment box cores or pipe cores were retrieved by divers (1974–1979; 1992–1993; Aller and Cochran, 1976; Aller et al., 1980; Gerino et al., 1998), or obtained remotely using either a Soutar box corer for surface sediment sampling or a gravity corer for deeper radiochemical measurements [1989–1991 previously unpublished; Long Island Sound Study (LISS)]. These techniques ensured retention of an undisturbed sediment surface, confirmed by inspection. Many locations were sampled seasonally (Figure 1: NWC, P, LISS sites). Surface water samples discussed here in order to illustrate benthic inputs of organic matter during the spring bloom period, are from a single site in the central basin (P; Figure 1) and were obtained during 1992–1993 (Gerino et al., 1998).
Sediment analyses summarized here include the radionuclides 234Th (t1/2= 24.1 days), 210Pb (t1/2= 22 years), 14C (t1/2= 5730 years), Chl-a, and x-radiography. 234Th and 238U measurements on samples taken in 1974–1979 and 1989–1991 were done in the same manner, namely leaching dried ground sediment in hot 8N HCl and then separating the leachate from the residual sediment by centrifugation. An aliquot (∼80% of the leach solution) was spiked with a 232U/228Th yield tracer. The remaining solution was left unspiked for determination of the natural 228Th/232Th activity ratio in the sample. The leachates were processed by ion exchange chromatography to separate the U and Th fractions, which were then electroplated onto stainless steel disks. The Th planchet was immediately counted on a gas flow proportional counter to determine the beta activity (234Th) and subsequently counted on silicon surface barrier detectors to assay the alpha activities of 232Th and 228Th. All excess 234Th (234Thxs) activities (= measured 234Th – measured 238U) were corrected to the time of collection. Uncertainties are based on 1σ counting errors. For the 1992–1993 samples, cores were sectioned and 234Th was determined on most samples by gamma spectrometry, using the 63 keV 234Th peak. Similarly, 210Pb and 226Ra measurements were made by gamma spectrometry at all sampling times. The 46.3 keV 210Pb and 352 keV 214Pb gamma peaks were used. Self-absorption corrections were made for 210Pb and 234Th using a calibration that relates the cpm/dpm of a standard to effective sample “density,” as determined by the attenuation of a gamma source counted through the sample vs. an empty sample container. Additional analytical techniques and details of sediment processing are given in the references cited in association with each data set.
Results and Discussion
Average Age of Sedimentary Corg
14C ages of bulk sedimentary Corg in the central basin of LIS demonstrate a typical surface sediment value of ∼2900 years, with a range from ∼2000 to 4000 and excursions to >6000 years, the latter presumably representing episodic deposition of more deeply eroded material (Figure 5; Benoit et al., 1979; Krishnaswami et al., 1984; Krom and Bennett, 1985). These bulk ages represent mixtures of modern planktonic and sewage inputs, moderately aged soil debris, and recycled fossil carbon (e.g., Trumbore et al., 1989; Plante et al., 2013). In 1975, the Δ14C value of LIS plankton was ∼281 (corrected for sediment contribution by Krom and Bennett, 1985) and of sewage sludge ∼429 (Benoit et al., 1979). Age of Corg is a direct indicator of lability, and in this case an apparent bulk Corg decomposition rate constant can be estimated as ∼0.8 × 10-4 year-1 (pseudo-first order coefficient) (Middelburg, 1989; Middelburg et al., 1993). Thus, LIS deposits represent a bulk Corg reservoir containing a spectrum of relatively refractory Corg fractions with average 14C age ∼3000 years into which younger and more labile material is constantly introduced by sedimentation and bioturbation processes. The vertical gradients in 14C activity are likely minimized by deep bioturbation (1–3 m; e.g., Squilla) but are nevertheless consistent with sediment accumulation rates in the range of the long-term seismically determined values (Benninger et al., 1979; Benoit et al., 1979; Krishnaswami et al., 1984).
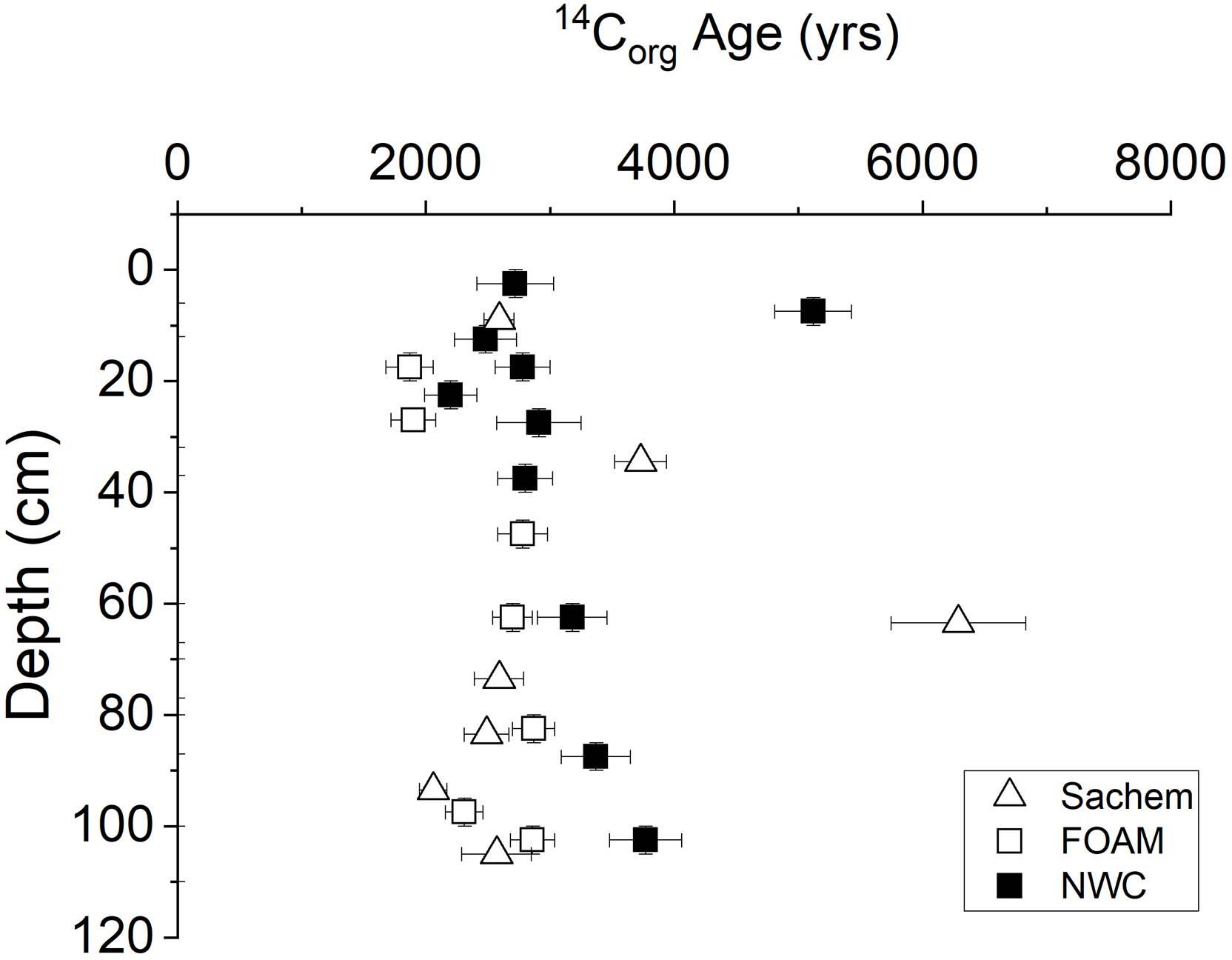
Figure 5. 14C age distributions in cores from Sachem, FOAM, and NWC sites in central basin (Benoit et al., 1979; Krishnaswami et al., 1984; Krom and Bennett, 1985).
Local Input of Reactive Organic Matter
The rapid penetration of labile, planktonically derived Corg into the background of more refractory sedimentary substrates in LIS deposits can be demonstrated by tracking the fate of Chl-a supplied to the seabed during the spring bloom period. Sedimentary Chl-a decomposition follows pseudo-first order kinetics with rate coefficients ∼0.02–0.04 days-1, similar to the decay constant of the natural radionuclide 234Th (λ = 0.0287 days-1) (Sun et al., 1991). The exact timing of the spring bloom in LIS can vary substantially from year to year, ranging from February to April. In 1993, the bloom occurred in late March–early April in central LIS (Figure 6). Chl-a derived from the bloom was deposited on the seabed (Station P; 15 m depth) and rapidly penetrated to depths >10 cm by diffusive, advective, and nonlocal biogenic transport modes (Gerino et al., 1998). In the absence of bioturbation, bloom inputs would otherwise be restricted to the upper ∼1 mm based on sediment accumulation rates, resuspension depths, and lack of deep reworking by storms during the study period. Prior to spring bloom deposition, sedimentary Chl-a was at near background levels for the preceding late fall and winter months, consistent with degradation timescales and lowered primary production. The Chl-a profiles illustrate the dynamic response of the seabed to production of labile Corg in the water column and the role of bioturbation in promoting interactions between Corg reductants of varied reactivity. Although the scaling of these examples documents interactions over ∼10 cm, infilling of burrow structures (e.g., Squilla) with surface material can take place to depths >1 m in LIS (Benninger et al., 1979).
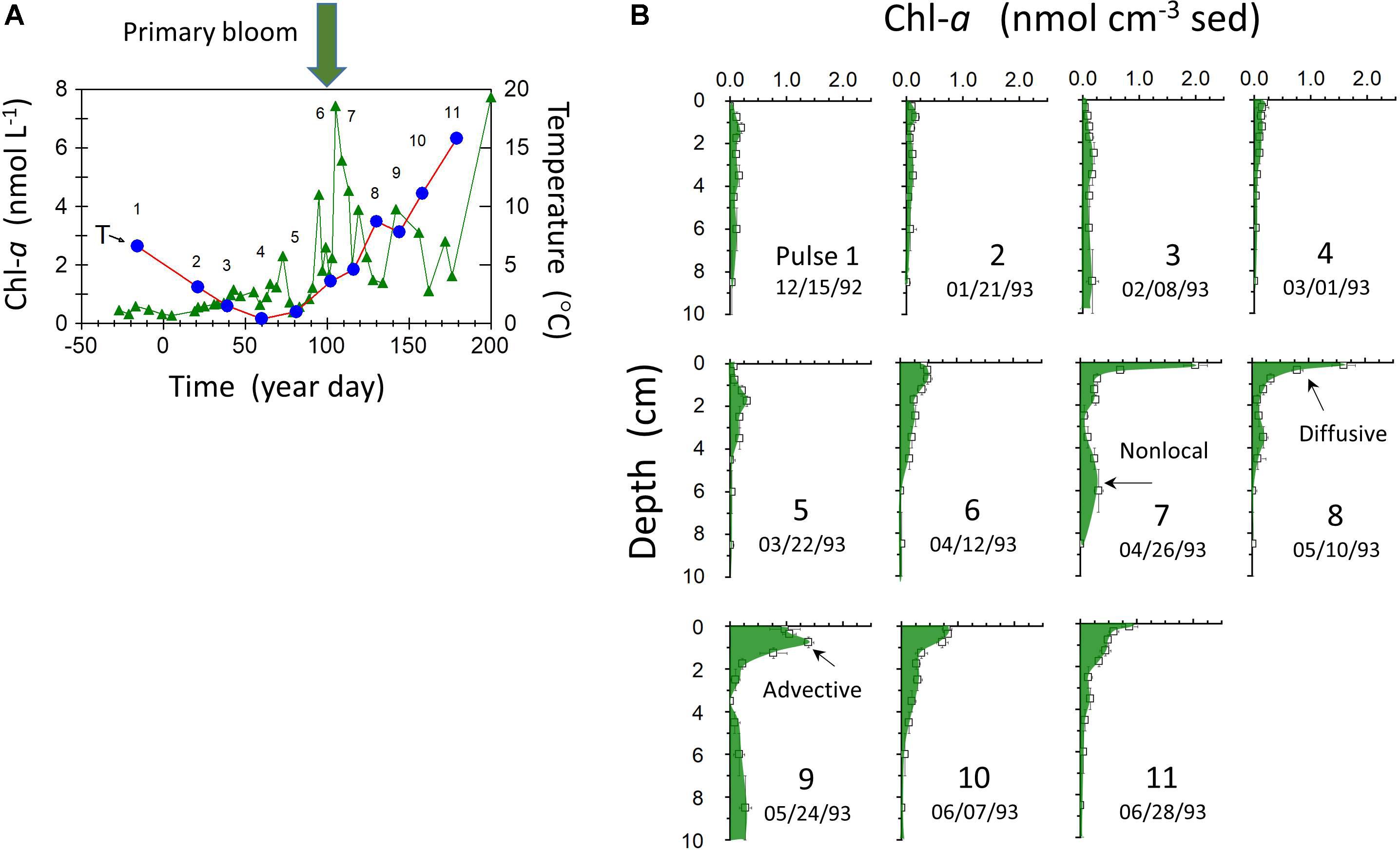
Figure 6. (A) Water column Chl-a (triangles) and temperature (circles) at station P (Figure 1) from 12/15/1992 to 6/28 1993 (year day indicated relative to January 1, 1993). (B) Sediment profiles of total Chl-a at successive sampling dates [sampling number labels also indicated in panel (A)]. Distribution features indicative of diffusive, advective, and nonlocal transport mechanisms on the time scale of Chl-a decay are indicated.
An alternative example of the rapid penetration, utilization, and dissipation of spring bloom inputs of labile substrates in a background of more refractory Corg is provided by exoenzyme activity seasonally at a mud site in central Great Peconic Bay (Figure 7). This site (8 m depth) has very similar sediment and environmental properties to the central LIS station P (Waugh and Aller, 2017). The average 14C age of Corg in the Peconics is ∼1800 ± 290 years (upper ∼50 cm; Cochran et al., 2000). In this case, the seasonal activity of the exoenzyme leucine aminopeptidase shows the presence of disseminated, low activity in the upper ∼8 cm during July, minor activity during Nov, deposition of labile spring bloom debris at the surface in March (with physical disturbance from a storm), and penetration of reactive substrate throughout the upper 6–7 cm by May (Cao et al., 2013). This exoenzyme activity is not dependent on oxygen availability, and thus reflects a range of sediment redox conditions. The bottom water temperature in May is comparable to that in November (∼12–13°C), illustrating the critical importance of labile substrate availability and inputs rather than temperature per se in determining remineralization rates (priming). The rapid dissemination of bloom debris into the upper ∼10 cm, results from intense bioturbation largely by maldanid polychaetes (e.g., Sabaco, Clymenella) and infaunal ophiuroids (Amphioplus), as reflected in profiles of the cosmogenic nuclide7Be (t1/2 = 53 days) that show penetration to >12 cm (Aller et al., 2019).
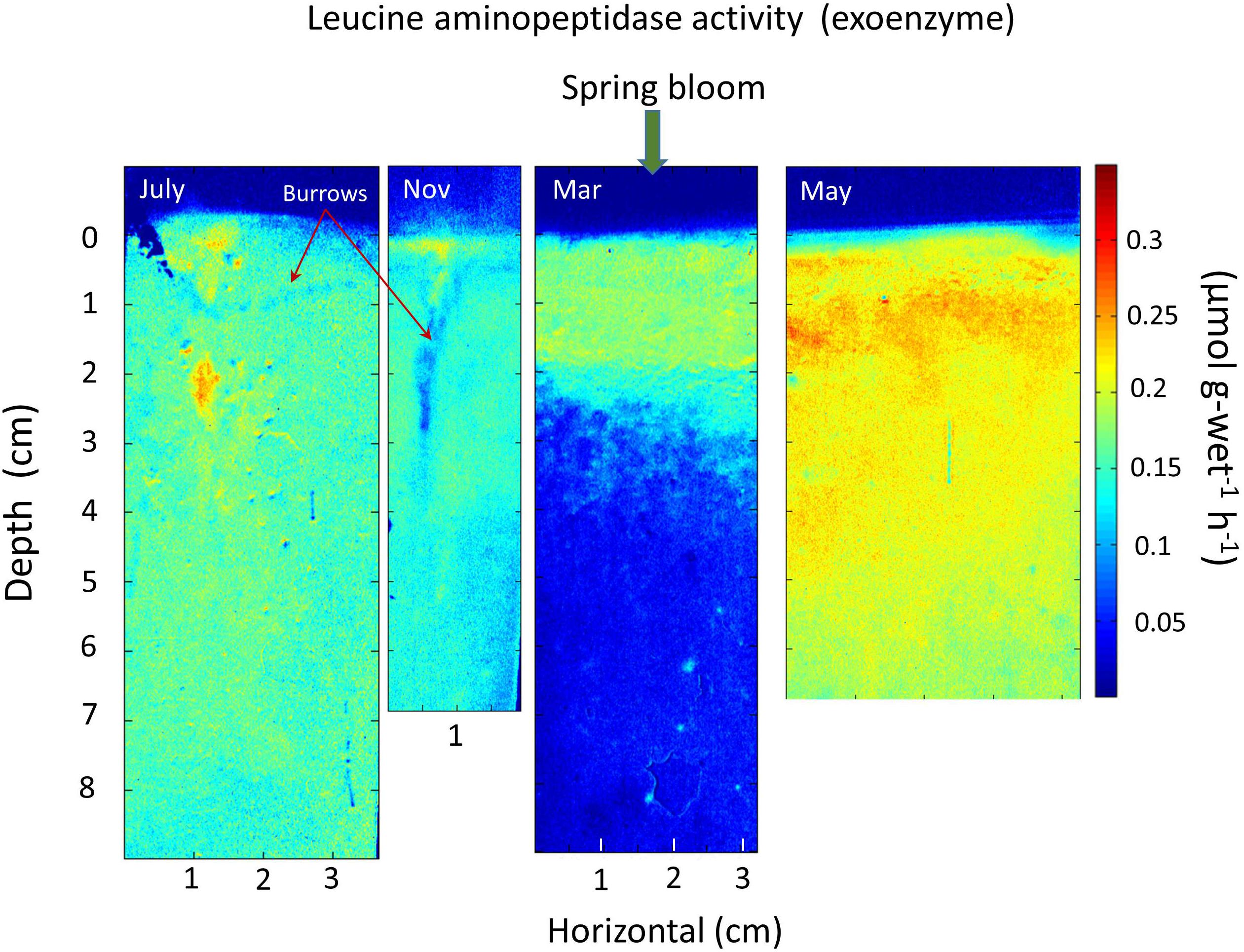
Figure 7. Seasonal exoenzyme leucine aminopeptidase activity in central Great Peconic Bay muds (Station 1; 8 m depth; Cao et al., 2013). The spring bloom input is evident during March (layer resuspended and emplaced during a storm event). Labile, reactive substrate is mixed into the deposit by intense deposit-feeding activity, penetrating at least 7 cm by May. May and November have similar temperatures (∼12–13°C), so that comparison of May, July, and November patterns (assuming periodic behavior) demonstrates the progressive remineralization and depletion of reactive substrates during spring–summer to fall periods, and the critical role of substrate availability introduced during particle reworking. Priming processes are presumably maximized during April–May in this system and in Long Island Sound.
Basin Wide Patterns of Labile Corg Supply
Profiles of sedimentary Chl-a in LIS sediments were determined seasonally at the 19 LISS sites (Figure 1; Sun et al., 1994). Late winter–spring period Chl-a inventories in the upper 10 cm of deposits show a regular pattern versus bathymetric depth, with higher inventories in shallower regions of LIS (Figure 8). The western Narrows sites show extremely high deposition of Chl-a during late winter–spring consistent with the elevated input of sewage derived nutrients and relatively low water exchange in that region. Although the water column in the Narrows is well-oxygenated during the winter, spring and early summer, it typically becomes hypoxic during late August (Cuomo et al., 2014). During the hypoxic period, bottom deposits in this region show greatly reduced infaunal activity, and, as revealed by x-radiographs, methane bubbles are present in deposits a few centimeters below the sediment surface (data not shown). At other times of the year, these deposits are colonized by macrobenthos and bioturbated (e.g., Figure 4). The sediment Chl-a inventory patterns are consistent with satellite image estimates of water column Chl-a showing relatively enhanced concentrations in the western Sound and Narrows at all times of the year (seasonal images1). Although elevated surface water Chl-a is also evident along the shorelines, in general there are minor N-S gradients compared to W-E gradients. Direct measurements of primary production in Long Island Sound are relatively limited, with estimates ranging from ∼400 to 1000 gC m-2 years-1 (90–230 mmol m-2 days-1), declining moderately from west to east, and averages in the central and western areas of ∼400 ± 80 gC m-2 years-1 (Riley, 1941, 1956; Goebel et al., 2006).
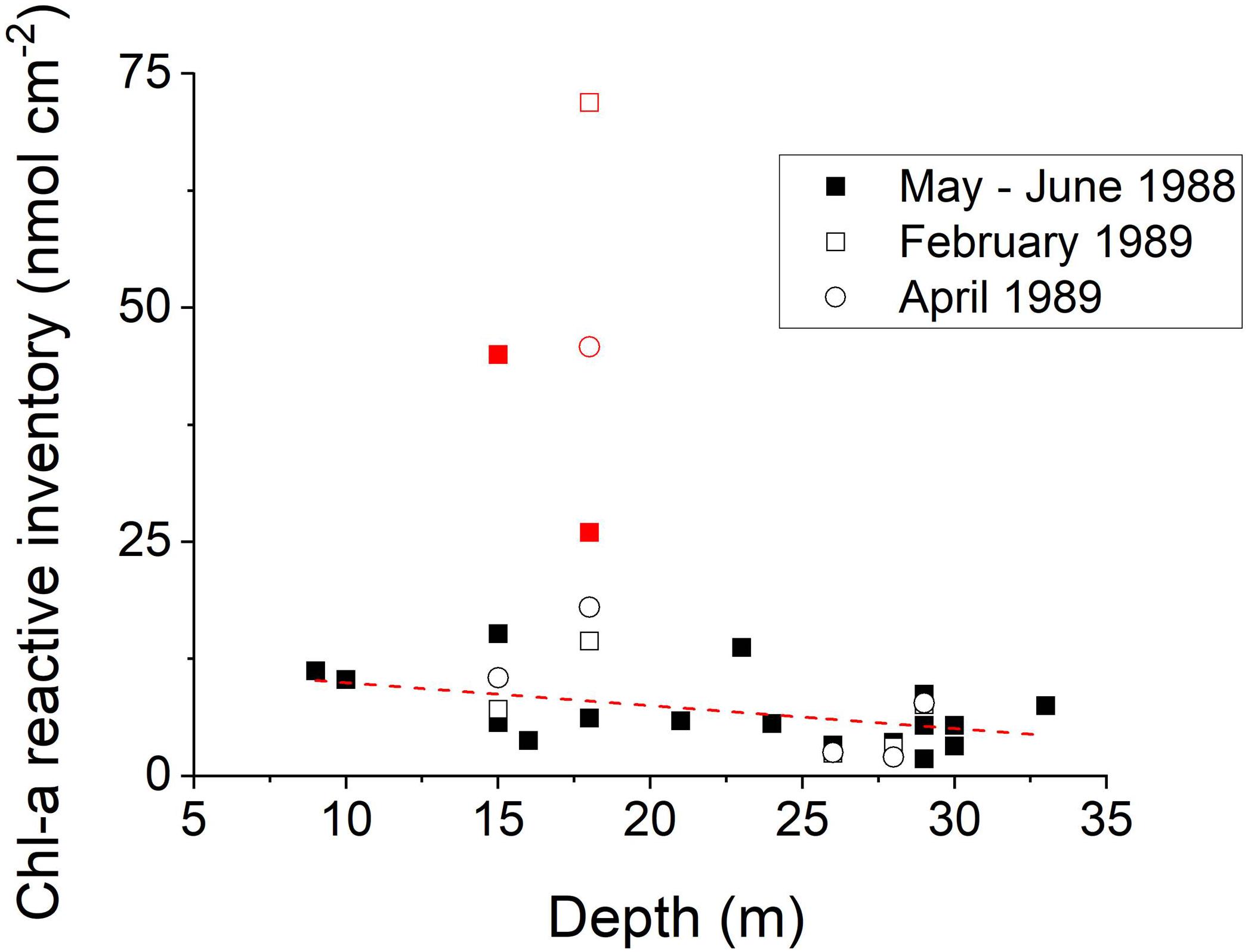
Figure 8. The inventory of Chl-a in the upper 10 cm at LISS stations during bloom or post-bloom periods in 1988 and 1989. Ignoring the four samples from the western Narrows (red; inserted graph), the regression (dotted line; y = –0.24x + 12.4) is statistically significant (r2 = 0.23; p < 0.05). There is a tendency to focus labile planktonic Corg into the shallower regions (Sun et al., 1994).
Radionuclide Tracers of Reactive Corg and Particle Dynamics
Monthly and Seasonal Timescales
Particle-reactive 234Th is produced continually in the water column by the decay of dissolved 238U, and provides a natural tracer of particle dynamics and the fate of labile Corg in LIS (Aller and Cochran, 1976; Aller et al., 1980). The production of 234Th varies with 238U activity, and thus directly with salinity (238U = 2.45 × (S/35) dpm L-1; where S = salinity). Once formed by decay, 234Th is scavenged from solution within <1 day onto abundant suspended particles in LIS, resulting in excess activity of234Th above the particulate 238U background (234Thxs). This excess activity decays with a half-life of 24.1 days (decay constant λ = 0.0288 day-1). As in the case of Chl-a produced in the water column, the 234Thxs can be deposited on the seabed and reworked into deposits by bioturbation or physical reworking. Fine, organic rich particles are particularly efficient at scavenging 234Th, as illustrated by the direct correlation of 234Thxs in surface sediment with indicators of particle size or Corg (Figure 9A and Supplementary Tables 1–5). This association and its further correspondence with labile Corg are confirmed by the direct correlation between summer period 234Thxs inventories and measurements of fluxes of remineralized NH4+ from bottom sediments (Figure 9B).
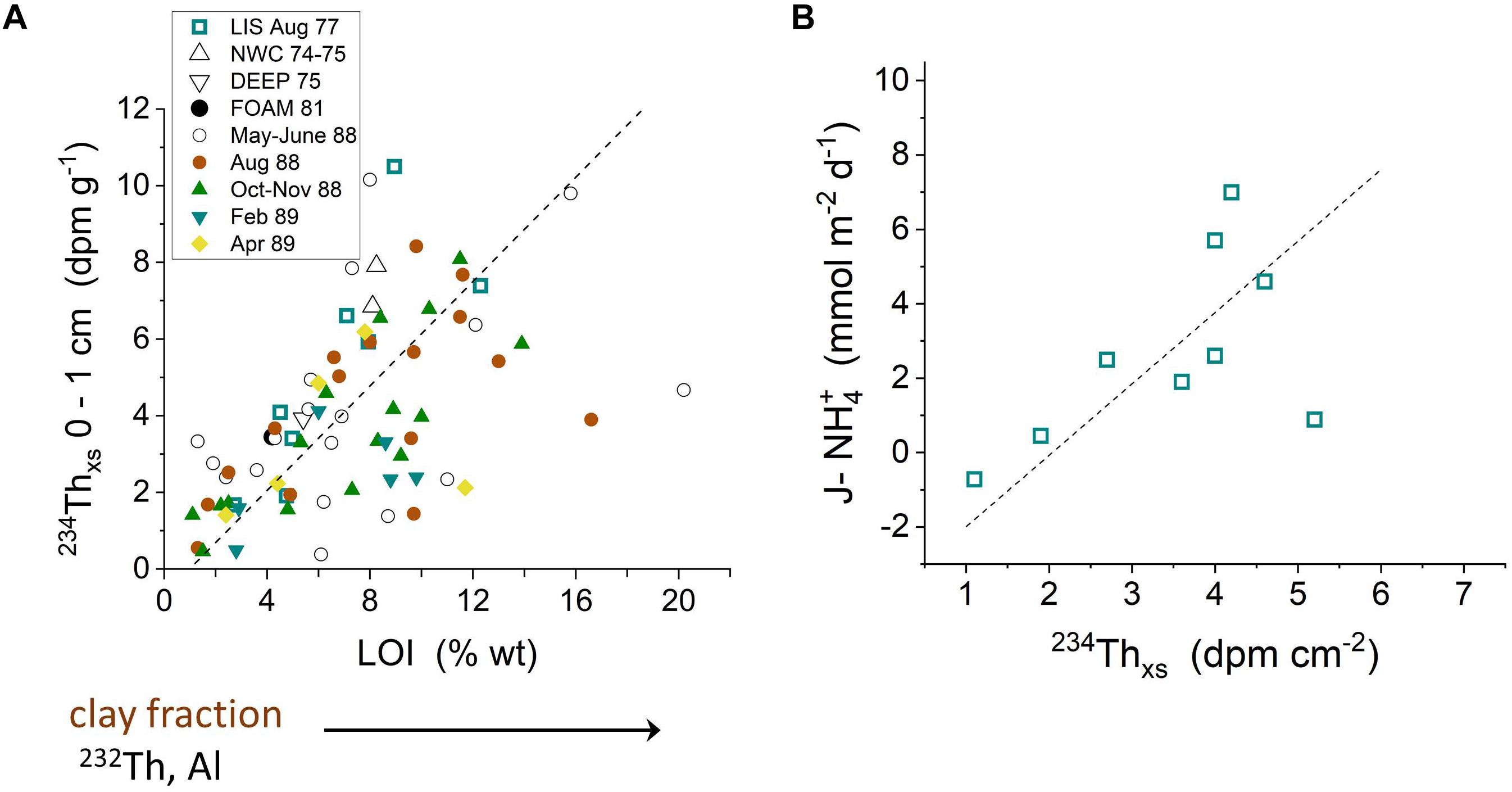
Figure 9. (A) 234Thxs activities in the upper 0–1 cm correlate directly with % weight loss on ignition (LOI), indicative of organic matter content (Aller et al., 1980; Krishnaswami et al., 1984). 234Thxs activities also correlate directly with 232Th (not shown), an indicator of small, clay mineral-rich particles. A geometric mean regression for all data is plotted (dashed line: y = 0.68x – 0.67; correlation coefficient r2 = 0.31; p < 0.001). Correlations can vary between seasonal data sets. (B) 234Thxs inventories (0–5 cm) correlate directly with the benthic flux of NH4+ from sediment at the same sampling location and time, demonstrating the coherence between 234Thxs and labile organic matter inventories during at least the summer periods when planktonic production is relatively high (August) (Aller and Benninger, 1981).
Vertical gradients of 234Thxs in deposits can be used to derive particle mixing coefficients, DB, and to estimate other modes of particle transport (e.g., nonlocal; Figure 6) (Aller and Cochran, 1976). In the present cases, DB was estimated assuming exponential decreases of 234Thxs with depth, and optimal fits to the integrated activities measured over finite sampling intervals (per Aller et al., 1980). Because the quantity of 234Thxs is fixed by 238U concentrations in the water column at secular equilibrium, the inventory of 234Thxs activity in LIS sediments must equal the source 238U activity. If, for example, particles simply settled out of the water column vertically to the bottom, the inventory of 234Thxs, ITh, would equal the integrated 238U activity in the immediate overlying water column: IThxs = z × (2.45 × S/35)/10 dpm cm-2; where z = water depth in meters. In LIS, salinity varies from 24 to 29, averaging ∼27, and average depth is 18–20 m, suggesting that 234Thxs inventories should vary directly with depth and salinity but average 3.4–3.8 dpm cm-2.
A summary of sedimentary 234Thxs inventories in LIS shows values scattered around the expected overall mean, implying that all 234Thxs produced in the LIS water column is found in bottom deposits (Figure 10A and Supplementary Tables 1–5). The lack of direct correlation between inventories and depth demonstrates that distributions do not result from simple 1-D vertical settling and that fine particles are cycled laterally through much of LIS on 234Th decay time scales (∼1 month). When annual average inventories are normalized to the theoretical inventory expected from production in the immediately overlying water column, it is clear that activity is differentially focused into relatively shallow water regions <25 m (Figure 10B). The coherence between the Chl-a inventory and 234Thxs inventory spatial patterns shows that water-column derived labile Corg behaves similarly, at least during periods when Chl-a is generated (Figures 8, 10B).
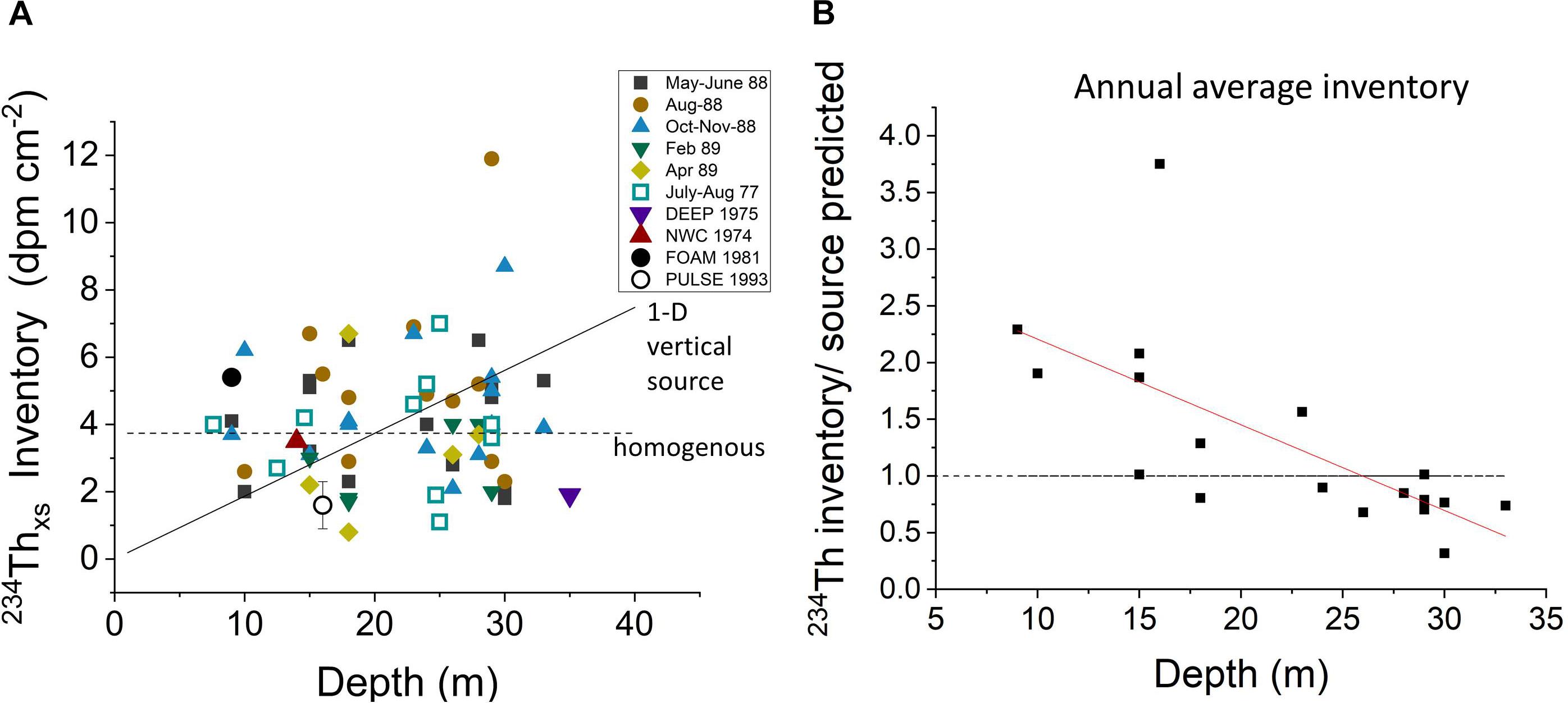
Figure 10. (A) The relationship between 234Thxs inventories in sediments and water depth. Inventories do not show the increase with depth expected for simple vertical settling of particles (solid line) but tend to be homogenized by lateral transport, scattering about the mean value expected for 234Thxs at the average depth of LIS (horizontal dotted line). (B) The average annual inventories at LISS sites normalized to the theoretical value at the same sites (238U source in immediate overlying water). The trend shows the tendency to focus 234Thxs into shallow regions (<20 m) at the expense of deeper ones on 234Th decay timescales (∼month), consistent with Chl-a inventory patterns (Figure 8).
The critical role of bioturbation in mediating the capture of labile Corg in this sedimentary system is demonstrated quantitatively by the relationship between biological reworking rates, DB, estimated from 234Thxs profiles at each site and the corresponding 234Thxs inventory (Figure 11; DB calculated per Aller et al., 1980). A positive correlation exists between DB and 234Thxs inventory throughout LIS (except during February 1989, see below), with regions of more rapidly mixed sediment having higher 234Thxs, and by inference, labile Corg.
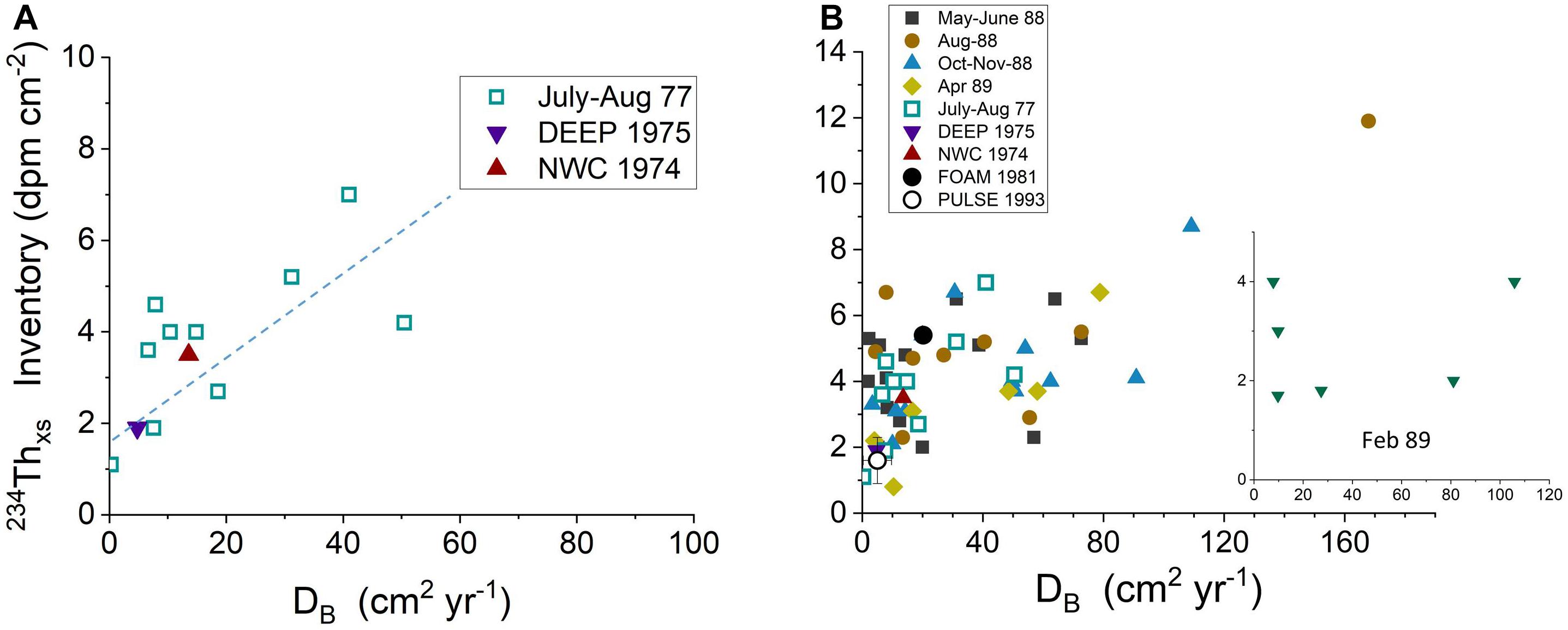
Figure 11. (A) Relationship of 234Thxs inventories with bioturbation rates measured as DB, diffusive mixing coefficients, in diver-collected cores during summer periods (1974–1977) (Aller et al., 1980). (B) Relationship of 234Thxs inventories with bioturbation rates estimated as DB, diffusive mixing coefficients, in diver-collected and remotely collected cores (LISS) during all seasons (1974–1991). Except during February 1989 (insert), there is a direct correlation between 234Thxs inventories and bioturbation rates (data from Aller et al., 1980; Cochran et al., 1991).
Annual and Decadal Timescales
The distributions of the particle reactive radionuclide 210Pb (t1/2 = 22 years; λ = 0.0315 year-1) in LIS reveal similarities and differences with the 234Thxs patterns. The sources of 210Pbxs to LIS are dominated by atmospheric deposition and detrital inputs from the Connecticut River with relatively minor contributions from the open shelf (Benninger, 1978). Thus, as in the case of 234Thxs, LIS behaves as a largely closed system with respect to 210Pbxs, and its distribution reflects particle cycling, reworking, and net deposition processes internal to LIS but over longer timescales than 234Thxs. By analogy, 210Pbxs should track the behavior of particulate Corg of moderate lability that is commonly present in sedimentary deposits (Westrich and Berner, 1984; Middelburg et al., 1993; Sayles et al., 2001). 210Pbxs inventories in LIS deposits show a direct correlation with biogenic vertical transport (Figure 12A and Supplementary Table 6), as calculated from either apparent sediment accumulation rates (biogenic advection model) or equivalent magnitudes of DB (biogenic diffusion model) (end-member transport calculations per Benninger et al., 1979; Gerino et al., 1998). Regardless of the exact transport mechanisms, the penetration rates of 210Pbxs into LIS deposits far exceed those predicted from net accumulation of sediment (0.03–0.08 cm year-1). These relationships show that surface-derived sedimentary material of moderate lability is, like the more labile fraction tracked by 234Thxs, captured and subducted into deposits during biogenic reworking. The additional correlation between 210Pbxs penetration depth and inventory shows that locations that are more deeply mixed accumulate greater quantities of moderately reactive material (Figure 12B and Supplementary Table 6). Thus, the more physically stable, often deeper regions of LIS, such as at stations DEEP and R in the central basin which are inhabited by abundant deep burrowing species of macrobenthos (Figure 3), tend to accumulate more 210Pbxs, and by inference, moderately reactive Corg into the bulk refractory Corg background.
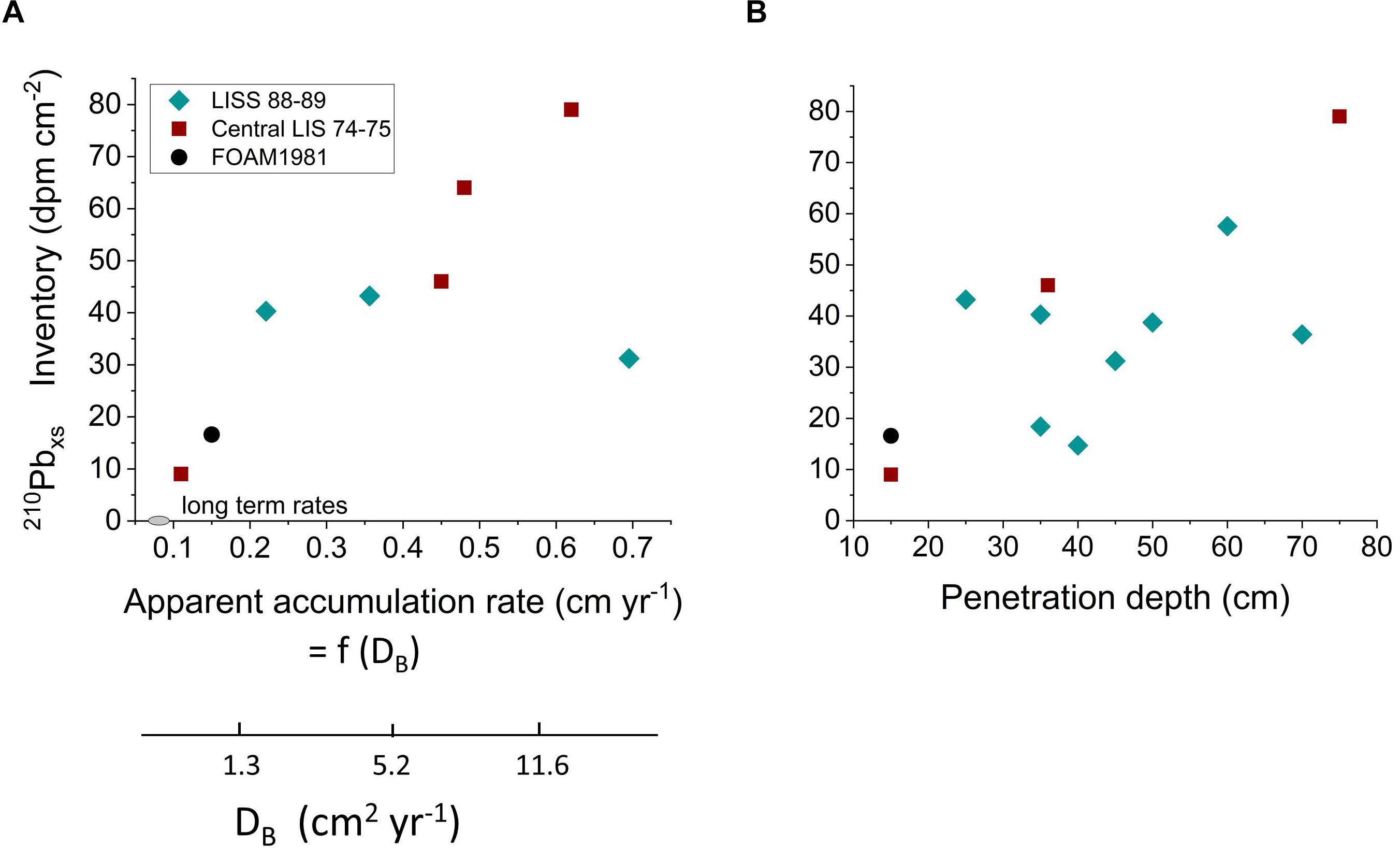
Figure 12. (A) 210Pbxs inventories in the central basin of LIS correlate with biogenic transport of particles estimated as either apparent accumulation rate (advection) or equivalent transport by diffusive mixing (DB). 234Thxs advection rates greatly exceed the long term accumulation rate estimated in the basin either from 14C gradients or seismically (range plotted as gray ellipse on x-axis). (B) 210Pbxs inventories correlate with 210Pbxs penetration depth throughout LIS. Deep mixing results in greater exchange of nonreactive for reactive particles over 210Pbxs decay timescales (decades).
Coupling of Physical and Biological Sediment Reworking
The supplies of labile planktonic material (Chl-a), 234Thxs, and 210Pbxs in LIS are fixed by primary production, 238U concentrations, and atmospheric/river inputs, respectively. The radiochemical sources are relatively constant, so that the differential accumulation of reactive material in deposits of one region is at the expense of another, that is, overall quantities cannot be changed only redistributed. Primary production is more episodic or periodic (seasonal) than are radiochemical supplies but organic particles are subject to the same redistribution processes. The mechanism by which reactive particle capture occurs into specific regions reflects the exchange of nonreactive for reactive material vertically in deposits during bioturbation (excavation), resuspension at the sediment-water interface (injection into water column), and lateral mixing – homogenization by physical transport and particle diffusion through the basin (Aller et al., 1980; Aller, 1982). Even in the absence of net accumulation of sediment, the exchange of reactive material by the coupling of vertical (biological) and horizontal (physical) transport will result in the capture and accumulation of reactive material into biologically mixed patches (Figure 13).
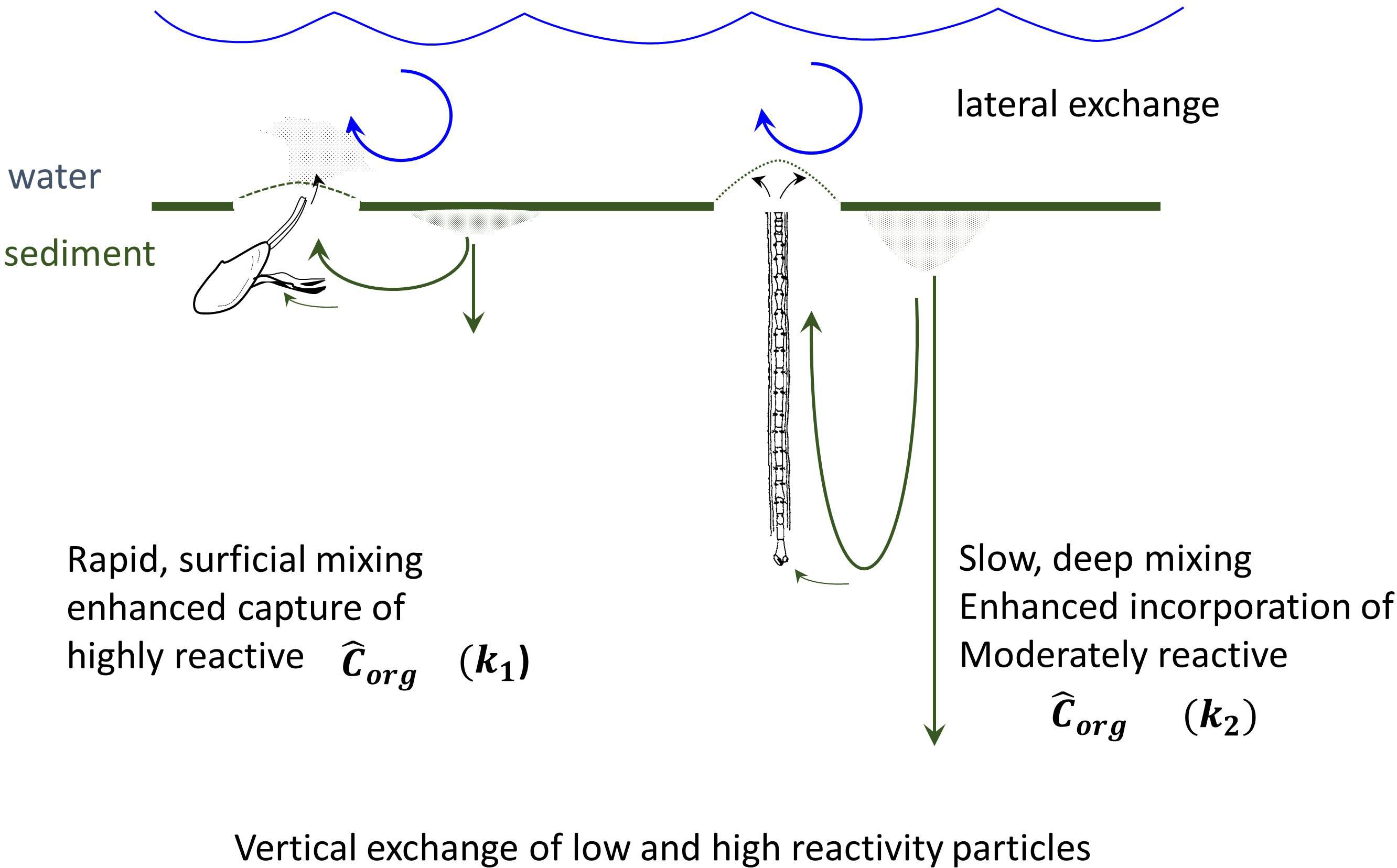
Figure 13. Schematic depiction of coupling of reactive organic matter capture and particle exchange with the scaling of particle bioturbation. Pseudo-first order rate coefficients for organic matter, k1 and k2, represent highly labile and moderately labile Corg pools, respectively. Highly labile substrates are focused into the intensely mixed regions having high resuspension and exchange. The direct injection of pseudofeces and feces into the water column, and fluidization of the seabed, by protobranch bivalves enhances turbidity and promotes physically driven lateral transport – exchange (Rhoads and Young, 1970). Reactive (labile) particles are captured by vertical exchange into intensely bioturbated patches. Over longer timescales, moderately reactive material migrates and exchanges into deeper regions of more slowly mixed deposits.
The time and spatial scales of biological reworking are controlled by benthic community compositions, which in turn are strongly influenced by physical conditions and interact with them, for example, by altering sediment mass properties and susceptibility to resuspension – erosion (Rhoads and Boyer, 1982). As mentioned earlier, in LIS, the shallow water muds (<20 m) are often inhabited by deposit-feeding protobranch bivalves which intensely rework the upper 0–5 cm of deposits (Sanders, 1956; Zajac et al., 2000). Their feeding and burrowing activities increase sediment water contents, enhance erodibility, and substantially elevate overlying water turbidity (Rhoads and Young, 1970). These biogenic impacts vary seasonally so that during warmer periods of high activity, the turbidity of bottom waters in LIS can be greatly increased (Rhoads et al., 1984). The less turbid water during winter periods allows benthic photosynthesis to depths of at least 15 m in the central basin (unpublished O2 production measurements) alters LIS particle recycling patterns, and may be a cause of the lack of correlation between 234Thxs inventories and DB during that time (Figure 11B). The LIS basin scale patterns of reactive particle capture are otherwise reflective of faunal communities and their coupling to the physical sedimentation regime (Figure 13). Any future shifts in these faunal distributions related, for example, to progressive environmental changes, will likely alter spatial patterns of particle exchange and remineralization.
Generality of Relationships
The relationships between reactive particle capture into deposits (inventories) and bioturbation (particle mixing) can be demonstrated in other systems, and in particular, in open margin environments. The highly productive margin region off Cape Hatteras has been particularly well studied in this regard (e.g., Blake and Hilbig, 1994; Blair et al., 1996; Levin et al., 1999; Green et al., 2002). Alperin et al. (2002) examined 210Pbxs and 239,240Pu distributions in a series of cores at 12 sites spanning depths of 212–1004 m, with an average of 568 m (median 538). 239,240Pu (t1/2= 24,110; 6573 years for 239Pu, 240Pu, respectively) was introduced into the marine system during atmospheric testing of nuclear weapons in the 1950s to ∼1963, and is a transient tracer of surface-derived reactive particles. The background bulk Corg pool in deposits in this region is typically 1000–2000 years old (DeMaster et al., 2002). Their data show that the inventories of both 239,240Pu and 210Pbxs along the margin correlate directly with DB, the latter calculated from an optimized mixing model of both 210Pb and 239,240Pu penetration patterns (Figure 14). As in the case of the LIS examples,239,240Pu and 210Pb inventories in this region do not generally correlate with net sedimentation (Alperin et al., 2002). Reactive reductant mixing mediated by bioturbation is clearly focused into bioturbated patches by the coupling of vertical (biogenic) and horizontal (physical) exchange.
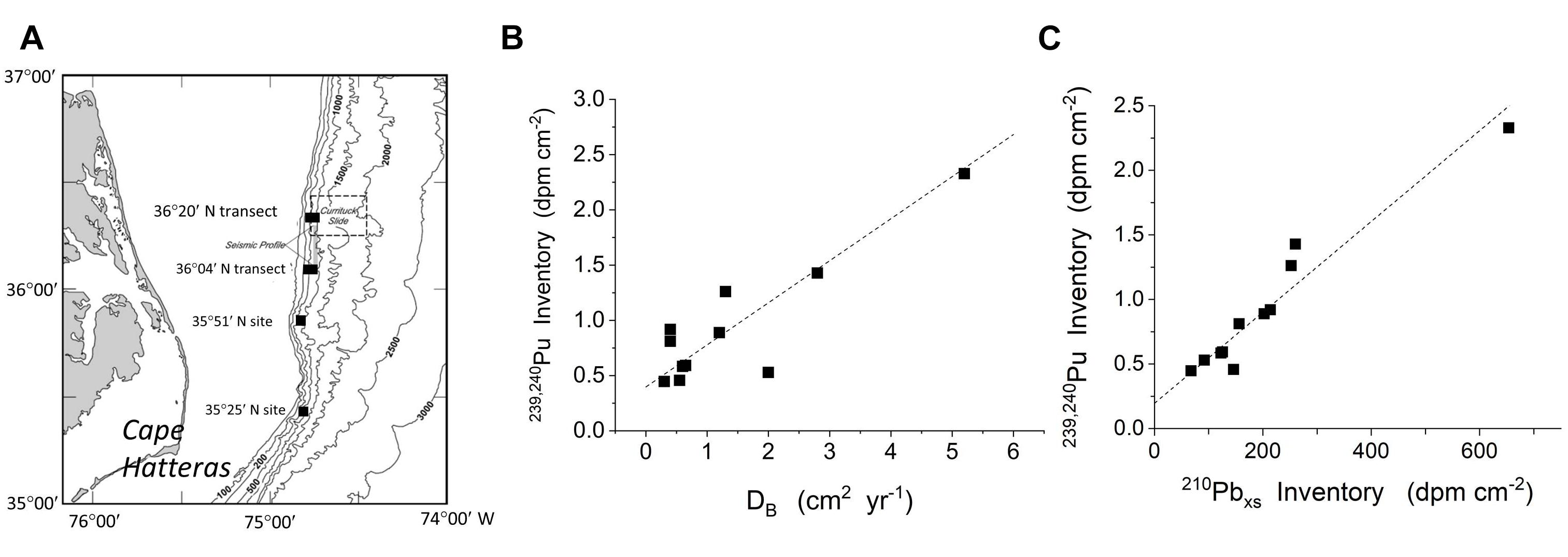
Figure 14. Data from the Cape Hatteras margin demonstrate that the coupling of local bioturbation with physical transport processes to produce large scale patterns of reactive particle distribution is a general phenomenon in sedimentary environments. (A) Location of sites sampled by Alperin et al. (2002) along the highly productive continental margin off Cape Hatteras. (B) The inventory of 239,240Pu varies directly with DB estimated at the same sites (data from Alperin et al., 2002). (C) The close correlation between 239,240Pu and 210Pbxs demonstrates that the tendency for reactive particle inventories to increase with biological mixing holds for multiple reactive components in this region (data from Alperin et al., 2002).
Summary and Conclusion
Priming of Corg remineralization in marine deposits depends on the mixing together and juxtaposition of relatively labile and refractory substrates. The biological reworking of sedimentary deposits inherently promotes priming through local redistribution of relatively reactive particles introduced at the sediment–water interface or direct injection of reactive secretions (mucus) by fauna into otherwise refractory deposits.
The coupling of resuspension of surface sediment and lateral transport of particles with bioturbation activity exchanges sediment and increases the flux, inventory, and penetration depths of reactive Corg into otherwise more refractory sedimentary debris.
Total remineralization (aerobic, anaerobic) in bioturbated deposits increases due to the increased inventory of reactive Corg as a function of reworking rate. Thus, when coupled to physical sedimentation processes, bioturbation is self-stimulating and priming interactions are enhanced at the community level.
Bioturbation affects remineralization patterns and priming patterns across basin scales, with the scaling of reactive reductant mixing depending directly on scaling of particle bioturbation.
These relations further suggest competition for food resources between spatially separated bioturbated regions of the seafloor, that is, bioturbation patch wars.
Although the local impacts of bioturbation on priming in marine sediments must be similar to those occurring in terrestrial soils, the coupling of water column sediment transport with local bioturbation processes in marine systems results in distinctive basin-scale patterns of remineralization and potential priming interactions not common in terrestrial environments.
Data Availability
All datasets generated for this study are included in the manuscript and/or the Supplementary Files.
Author Contributions
RA and JC conceptualized the project, and participated in the field and laboratory work. RA wrote the initial version of the manuscript.
Funding
This synthesis utilizes data that were collected with funding from a variety of sources, including the NSF, DOE, EPA, and NOAA. This work was supported by the NSF OCE 1737749 (to RA) and the NSF OCE 1736591 (to JC).
Conflict of Interest Statement
The authors declare that the research was conducted in the absence of any commercial or financial relationships that could be construed as a potential conflict of interest.
Acknowledgments
A large number of colleagues aided with the multiple field campaigns and analyses utilized here, in particular D. Hirschberg and C. Heilbrun. J. Y. Aller aided with manuscript preparation. We thank the late K. K. Turekian for the intellectual catalysis, and also multiple additional colleagues for discussion.
Supplementary Material
The Supplementary Material for this article can be found online at: https://www.frontiersin.org/articles/10.3389/feart.2019.00157/full#supplementary-material
Footnotes
References
Aller, J. Y., and Aller, R. C. (1986). Evidence for localized enhancement of biological-activity associated with tube and burrow structures in deep-sea sediments at the Hebble Site. West. North Atlantic Deep-Sea Res. 33, 755–790. doi: 10.1016/0198-0149(86)90088-9
Aller, R. C. (1980). “Diagenetic processes near the sediment-water interface of Long Island Sound. I. Decomposition and nutrient element geochemistry (S,N,P),” in Advances in Geophysics 22, ed. B. Saltzman (New York, NY: Elsevier), 235–348.
Aller, R. C. (1982). “The effects of macrobenthos on the chemical properties of marine sediments and overlying water,” in Animal-Sediment Relations :The biogenic alterations of sediments, eds P. L. McCall and M. J. S. Tevez (Berlin: Springer), 53–102. doi: 10.1007/978-1-4757-1317-6_2
Aller, R. C., Aller, J. Y., and Kemp, P. F. (2001). “Effects of particle and solute transport on rates and extents of remineralization in bioturbated sediments,” in Organism-Sediment Interactions, eds J. Y. Aller, R. C. Aller, and S. Woodin (Columbia SC: University So. Carolina Press), 315–333.
Aller, R. C., Aller, J. Y., Zhu, Q.-Z., Heilbrun, C., Klingensmith, I., and Kaushik, A. (2019). Worm tubes as conduits for the electrogenic microbial grid in marine sediments. Sci. Adv. 5, (in press).
Aller, R. C., and Benninger, L. K. (1981). Spatial and temporal patterns of dissolved ammonium, manganese, and silica fluxes from bottom sediments of Long Island Sound. U.S.A. J. Mar. Res. 39, 295–314.
Aller, R. C., Benninger, L. K., and Cochran, J. K. (1980). Tracking particle associated processes in the coastal marine environment by use of 234-Th / 238-U disequilibrium. Earth Planet. Sci. Lett. 47, 161–175. doi: 10.1016/0012-821x(80)90034-5
Aller, R. C., and Cochran, J. K. (1976). 234-Th / 238-U disequilibrium in near-shore sediment - particle reworking and diagenetic time scales. Earth Planet. Sci. Lett. 29, 37–50. doi: 10.1016/0012-821x(76)90024-8
Alperin, M. J., Suayah, I. B., Benninger, L. K., and Martens, C. S. (2002). Modern organic carbon burial fluxes, recent sedimentation rates, and particle mixing rates from the upper continental slope near Cape Hatteras. North Carolina (USA). Deep-Sea Res. Part II 49, 4645–4665. doi: 10.1016/s0967-0645(02)00133-9
Auster, P. J., Heinonen, K. B., Witharana, C., and McKee, M. (2009). A Habitat Classification Scheme for the Long Island Sound Region. Stamford: EPA Long Island Sound Office, 83.
Benninger, L. K. (1978). Pb-210 balance in Long-Island Sound. Geochim. Cosmochim. Acta 42, 1165–1174. doi: 10.1016/0016-7037(78)90111-4
Benninger, L. K., Aller, R. C., Cochran, J. K., and Turekian, K. K. (1979). Effects of biological sediment mixing on the Pb-210 chronology and trace-metal distribution in a Long Island Sound sediment core. Earth Planet. Sci. Lett. 43, 241–259. doi: 10.1016/0012-821x(79)90208-5
Benoit, G. J., Turekian, K. K., and Benninger, L. K. (1979). Radiocarbon dating of a core from Long-Island Sound. Est. Coast. Mar. Sci. 9, 171–180. doi: 10.1016/0302-3524(79)90112-9
Bianchi, T. S., Thornton, D. C. O., Yvon-Lewis, S. A., King, G. M., Eglinton, T. L., Shields, M. R., et al. (2015). Positive priming of terrestrially derived dissolved organic matter in a freshwater microcosm system. Geophy.res. Lett. 42, 5460–5467. doi: 10.1002/2015GL064765
Blair, N. E., Levin, L. A., DeMaster, D. J., and Plaia, G. (1996). The short-term fate of fresh algal carbon in continental slope sediments. Limnol. Oceanogr. 41, 1208–1219. doi: 10.4319/lo.1996.41.6.1208
Blake, J. A., and Hilbig, B. (1994). Dense infaunal assemblages on the continental-slope off Cape-Hatteras. North-Carolina. Deep-Sea Res. II 41, 875–899. doi: 10.1016/0967-0645(94)90052-3
Bokuniewicz, H., Gebert, J., and Gordon, R. B. (1976). Sediment mass balance of a large estuary. Long Island Sound. Est. Coast. Mar. Sci. 4, 523–536. doi: 10.1016/j.jenvrad.2014.08.014
Bokuniewicz, H., and Gordon, R. B. (1980). “Sediment transport and deposition in Long Island Sound,” in Advances in Geophysics, 22, ed. B. Saltzman (New York, NY: Elsevier), 69–106. doi: 10.1016/s0065-2687(08)60063-1
Cao, Z., Zhu, Q., Aller, R. C., Aller, J. Y., and Waugh, S. (2013). Seasonal, 2-D sedimentary extracellular enzyme activities and controlling processes in Great Peconic Bay, Long Island. J. Mar. Res. 71, 399–423. doi: 10.1357/002224013812587573
Cochran, J. K., Aller, R. C., Aller, J. Y., Hirschberg, D. J., and Mackin, J. E. (1991). Long Island Sound Study Sediment Geochemistry and Biology. Stony Brook, NY: Marine Sciences Research Center.
Cochran, J. K., Hirschberg, D., and Amiel, D. (2000). Particle Mixing and Sediment Accumulation Rates of Peconic Estuary Sediments: A Sediment Accretion Study in Support of the Peconic Estuary Program. Stony Brook, NY: Marine Sciences Research Center.
Cuomo, C., Cochran, J. K., and Turekian, K. K. (2014). “Geochemistry of the Long Island Sound Estuary,” in Long Island Sound, eds J. S. Latimer, M. A. Tedesco, R. L. Swanson, C. Yarish, P. E. Stacey, and C. Garza (Berlin: Springer), 159–201. doi: 10.1007/978-1-4614-6126-5_4
DeMaster, D. J., Thomas, C. J., Blair, N. E., Fornes, W. L., Plaia, G., and Levin, L. A. (2002). Deposition of bomb (14)C in continental slope sediments of the Mid-Atlantic Bight: assessing organic matter sources and burial rates. Deep-Sea Res. II. 49, 4667–4685. doi: 10.1016/s0967-0645(02)00134-0
Eckman, J. E., Nowell, A. R. M., and Jumars, P. A. (1981). Sediment destabilization by animal tubes. J. Mar. Res. 39, 361–374.
François, F., Gérino, M., Stora, G., Durbec, J.-P., and Poggiale, J.-C. (2002). Functional approach to sediment reworking by gallery-forming macrobenthic organisms: modeling and application with the polychaete Nereis diversicolor. Mar. Ecol. Prog. Ser. 229, 127–136. doi: 10.3354/meps229127
Gerino, M., Aller, R. C., Lee, C., Cochran, J. K., Aller, J. Y., Green, M. A., et al. (1998). Comparison of different tracers and methods used to quantify bioturbation during a spring bloom: 234-Thorium, luminophores and chlorophyll a. Est. Coast. Shelf Sci. 46, 531–547. doi: 10.1006/ecss.1997.0298
Goebel, N. L., Kremer, J. N., and Edwards, C. A. (2006). Primary production in Long Island Sound. Est. Coast. 29, 232–245. doi: 10.1007/bf02781992
Graf, G. (1992). Benthic-pelagic coupling: a benthic view. Oceanogr. Mar. Biol. Annu. Rev. 30, 149–190. doi: 10.1007/s11356-015-4997-2
Green, M. A., Aller, R. C., Cochran, J. K., Lee, C., and Aller, J. Y. (2002). Bioturbation in shelf/slope sediments off Cape Hatteras, North Carolina: the use of Th-234, Ch1-a, and Br- to evaluate rates of particle and solute transport. Deep-Sea Res. II. 49, 4627–4644. doi: 10.1016/s0967-0645(02)00132-7
Hannides, A. K., and Aller, R. C. (2016). Priming effect of benthic gastropod mucus on sedimentary organic matter remineralization. Limnol. Oceanogr. 61, 1640–1650. doi: 10.1002/lno.10325
Hee, C. A., Pease, T. K., Alperin, M. J., and Martens, C. S. (2001). Dissolved organic carbon production and consumption in anoxic marine sediments: a pulsed-tracer experiment. Limnol. Oceanogr. 46, 1908–1920. doi: 10.4319/lo.2001.46.8.1908
Knebel, H. J., and Poppe, L. J. (2000). Sea-floor environments within Long Island Sound: a regional overview. J. Coast. Res. 16, 533–550.
Krishnaswami, S., Monaghan, M. C., Westrich, J. T., Bennett, J. T., and Turekian, K. K. (1984). chronologies of sedimentary processes in sediments of the Foam Site. Long-Island Sound Connecticut. Am. J. Sci. 284, 706–733. doi: 10.2475/ajs.284.6.706
Kristensen, E., and Kostka, J. E. (2005). Macrofaunal burrows and irrigation in marine sediment: microbiological and biogeochemical interactions. AGU Coas. Estuar. Stud. 60, 125–157. doi: 10.1029/ce060p0125
Krom, M. D., and Bennett, J. T. (1985). Sources, deposition rates and decomposition of organic-carbon in recent sediment of Sachem Head Harbor. Long-Island Sound. Est. Coast. Shelf Sci. 21, 325–336. doi: 10.1016/0272-7714(85)90014-9
Levin, L. A., Blair, N. E., Martin, C. M., DeMaster, D. J., Plaia, G., and Thomas, C. J. (1999). Macrofaunal processing of phytodetritus at two sites on the Carolina margin: in situ experiments using C-13-labeled diatoms. Mar. Ecol. Prog. Ser. 182, 37–54. doi: 10.3354/meps182037
Lewis, R. S., and DiGiacomo-Cohen, M. (2000). A review of the geologic framework of the Long Island Sound basin, with some observations relating to postglacial sedimentation. J. Coast. Res. 16, 522–532.
McCall, P. L. (1977). Community patterns and adaptive strategies of infaunal benthos of Long Island Sound. J. Mar. Res. 35, 221–266.
McCall, P. L. (1978). “Spatial-temporal distributions of Long Island Sound infauna: the role of bottom disturbance in a nearshore habitat,” in Estuarine interactions, ed. M. L. Wiley (New York, NY: Academic Press), 191–219. doi: 10.1016/b978-0-12-751850-3.50019-4
Meysman, F. J. R., Boudreau, B. P., and Middelburg, J. J. (2003). Relations between local, nonlocal, discrete and continuous models of bioturbation. J. Mar. Res. 61, 391–410. doi: 10.1357/002224003322201241
Middelburg, J. J. (1989). A simple rate model for organic-matter decomposition in marine-sediments. Geochim. Cosmochim. Acta. 53, 1577–1581. doi: 10.1016/0016-7037(89)90239-1
Middelburg, J. J., Vlug, T., and Vandernat, F. (1993). Organic-matter mineralization in marine systems. Glob. Planet. Change 8, 47–58. doi: 10.1016/0921-8181(93)90062-s
Myers, A. C. (1979). Summer and winter burrows of a mantis shrimp, Squilla empusa, in Narragansett Bay, Rhode-Island (USA). Est. Coast. Mar. Sci. 8, 87–98. doi: 10.1016/0302-3524(79)90107-5
O’Donnell, J., Wilson, R. E., Lwiza, K., Whitney, M., Bohlen, W. F., Codiga, D., et al. (2014). “The physical oceanography of Long Island Sound,” in Long Island Sound, eds J. S. Latimer, M. A. Tedesco, R. L. Swanson, C. Yarish, P. E. Stacey, and C. Garza (Berlin: Springer), 79–158. doi: 10.1007/978-1-4614-6126-5_3
Pillay, D., and Branch, G. M. (2011). Bioengineering effects of burrowing thalassinidean shrimps on marine soft-bottom ecosystems. Oceanogr. Mar. Biol. 49, 137–191.
Plante, A. F., Beaupre, S. R., Roberts, M. L., and Baisden, T. (2013). Distribution of radiocarbon ages in soil organic matter by thermal fractionation. Radiocarbon 55, 1077–1083. doi: 10.1017/s0033822200058215
Poppe, L. J., Knebel, H. J., Mlodzinska, Z. J., Hastings, M. E., and Seekins, B. A. (2000). Distribution of surficial sediment in Long Island Sound and adjacent waters: texture and total organic carbon. J. Coast. Res. 16, 567–574.
Reid, R. N., Radosh, D. J., Frame, A. B., and Fromm, S. A. (1979). Benthic macrofauna of the New York Bight, 1979-89. NOAA Technical Rep. 738, 1–50.
Rhoads, D. C. (1974). Organism-sediment relations on the muddy sea floor. Oceanogr. Mar. Biol. Ann. Rev. 12, 263–300.
Rhoads, D. C., and Boyer, L. F. (1982). “The effects of marine benthos on physical properties of sediments: asuccessional perspective,” in Animal-Sediment Relations: The Biogenic Alterations of Sediments, eds P. L. McCall and M. J. S. Tevesz (Berlin: Springer), 3–52. doi: 10.1007/978-1-4757-1317-6_1
Rhoads, D. C., Boyer, L. F., Welsh, B. L., and Hampson, G. R. (1984). Seasonal dynamics of detritus in the benthic turbidity zone (btz) - implications for bottom-rack molluscan mariculture. Bull. Mar. Sci. 35, 536–549.
Rhoads, D. C., and Young, D. K. (1970). Influence of deposit-feeding organisms on sediment stability and community trophic structure. J. Mar. Res. 28, 150–178.
Riley, G. A. (1941). Plankton studies. III. Long Island Sound. Bull. Bingham Oceanogr. Coll. 7, 1–93.
Riley, G. A. (1956). Oceanography of Long Island Sound, 1952–1954. IX. Production and utilization of organic matter. Bull. Bingham Oceanogr. Coll. 15, 324–344.
Sanders, H. L. (1956). “The Biology of Marine Bottom Communities,” in Oceanography of Long Island Sound, 1952–1954, eds G. A. Riley, S. A. M. Conover, G. B. Deevey, R. J. Conover, S. B. Wheatland, E. Harris, et al. (New Haven, CT: Peabody Museum of Natural History, Yale University), 345–414.
Sayles, F. L., Martin, W. R., Chase, Z., and Anderson, R. F. (2001). Benthic remineralization and burial of biogenic SiO2, CaCO3, organic carbon, and detrital material in the Southern Ocean along a transect at 170. West. Deep-Sea Res. II. 48, 4323–4383. doi: 10.1016/s0967-0645(01)00091-1
Smith, C. R., Hoover, D. J., Doan, S. E., Pope, R. H., Demaster, D. J., Dobbs, F. C., et al. (1996). Phytodetritus at the abyssal seafloor across 10 degrees of latitude in the central equatorial Pacific. Deep-Sea Res. II. 43, 1309–1338. doi: 10.1016/0967-0645(96)00015-x
Solan, M., Batty, P., Bulling, M. T., and Godbold, J. A. (2008). How biodiversity affects ecosystem processes: implications for ecological revolutions and benthic ecosystem function. Aq. Biol. 2, 289–301. doi: 10.3354/ab00058
Sun, M. Y., Aller, R. C., and Lee, C. (1991). Early diagenesis of chlorophyll-a in Long Island Sound sediments: a measure of carbon flux and particle reworking. J. Mar. Res. 49, 379–401. doi: 10.1357/002224091784995927
Sun, M. Y., Aller, R. C., and Lee, C. (1994). Spatial and temporal distributions of sedimentary chloropigments as indicators of benthic processes in Long-Island Sound. J. Mar. Res. 52, 149–176. doi: 10.1357/0022240943076768
Trumbore, S. E., Vogel, J. S., and Southon, J. R. (1989). AMS C-14 measurements of fractionated soil organic-matter - an approach to deciphering the soil carbon-cycle. Radiocarb. 31, 644–654. doi: 10.1017/s0033822200012248
van Nugteren, P., Moodley, L., Brummer, G. J., Heip, C. H. R., Herman, P. M. J., and Middelburg, J. J. (2009). Seafloor ecosystem functioning: the importance of organic matter priming. Mar. Biol. 156, 2277–2287. doi: 10.1007/s00227-009-1255-5
Wanless, H. R., Tedesco, L. P., and Tyrrell, K. M. (1988). Production of subtidal tubular and surficial tempestites by hurricane Kate. Caicos platform, British-West-Indies. J. Sed. Pet. 58, 739–750.
Waugh, S., and Aller, R. C. (2017). N-2 production and fixation in deep-tier burrows of Squilla empusa in muddy sediments of Great Peconic Bay. J. Sea Res. 129, 36–41. doi: 10.1016/j.seares.2017.08.012
Westrich, J. T., and Berner, R. A. (1984). The role of sedimentary organic-matter in bacterial sulfate reduction - the G model tested. Limnol. Oceanogr. 29, 236–249. doi: 10.4319/lo.1984.29.2.0236
Zajac, R. N., Lewis, R. S., Poppe, L. J., Twichell, D. C., Vozarik, J., and DiGiacomo-Cohen, M. L. (2000). Relationships among sea-floor structure and benthic communities in Long Island Sound at regional and benthoscape scales. J. Coast. Res. 16, 627–640.
Keywords: bioturbation, priming, sediment diagenesis, carbon remineralization, reductant mixing, particle mixing, natural radionuclides
Citation: Aller RC and Cochran JK (2019) The Critical Role of Bioturbation for Particle Dynamics, Priming Potential, and Organic C Remineralization in Marine Sediments: Local and Basin Scales. Front. Earth Sci. 7:157. doi: 10.3389/feart.2019.00157
Received: 13 March 2019; Accepted: 05 June 2019;
Published: 25 June 2019.
Edited by:
Nicholas David Ward, Pacific Northwest National Laboratory (DOE), United StatesReviewed by:
Erik Kristensen, University of Southern Denmark, DenmarkAndrew W. Dale, GEOMAR Helmholtz Centre for Ocean Research Kiel, Germany
Copyright © 2019 Aller and Cochran. This is an open-access article distributed under the terms of the Creative Commons Attribution License (CC BY). The use, distribution or reproduction in other forums is permitted, provided the original author(s) and the copyright owner(s) are credited and that the original publication in this journal is cited, in accordance with accepted academic practice. No use, distribution or reproduction is permitted which does not comply with these terms.
*Correspondence: Robert C. Aller, cm9iZXJ0LmFsbGVyQHN0b255YnJvb2suZWR1