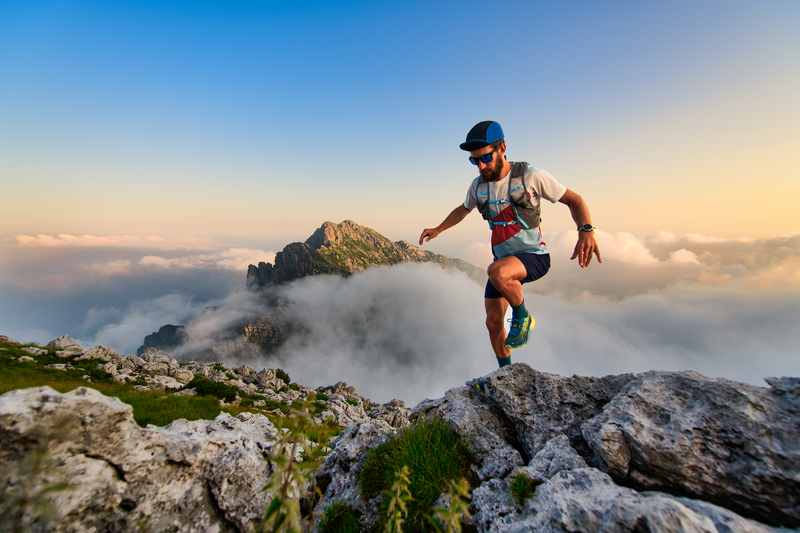
95% of researchers rate our articles as excellent or good
Learn more about the work of our research integrity team to safeguard the quality of each article we publish.
Find out more
HYPOTHESIS AND THEORY article
Front. Earth Sci. , 11 June 2019
Sec. Biogeoscience
Volume 7 - 2019 | https://doi.org/10.3389/feart.2019.00127
This article is part of the Research Topic The Role of Priming in Terrestrial and Aquatic Ecosystems View all 7 articles
Across a landscape, aquatic-terrestrial interfaces within and between ecosystems are hotspots of organic matter (OM) mineralization. These interfaces are characterized by sharp spatio-temporal changes in environmental conditions, which affect OM properties and thus control OM mineralization and other transformation processes. Consequently, the extent of OM movement at and across aquatic-terrestrial interfaces is crucial in determining OM turnover and carbon (C) cycling at the landscape scale. Here, we propose expanding current concepts in aquatic and terrestrial ecosystem sciences to comprehensively evaluate OM turnover at the landscape scale. We focus on three main concepts toward explaining OM turnover at the landscape scale: the landscape spatio-temporal context, OM turnover described by priming and ecological stoichiometry, and anthropogenic effects as a disruptor of natural OM transfer magnitudes and pathways. A conceptual framework is introduced that allows for discussing the disparities in spatial and temporal scales of OM transfer, changes in environmental conditions, ecosystem connectivity, and microbial–substrate interactions. The potential relevance of priming effects in both terrestrial and aquatic systems is addressed. For terrestrial systems, we hypothesize that the interplay between the influx of OM, its corresponding elemental composition, and the elemental demand of the microbial communities may alleviate spatial and metabolic thresholds. In comparison, substrate level OM dynamics may be substantially different in aquatic systems due to matrix effects that accentuate the role of abiotic conditions, substrate quality, and microbial community dynamics. We highlight the disproportionate impact anthropogenic activities can have on OM cycling across the landscape. This includes reversing natural OM flows through the landscape, disrupting ecosystem connectivity, and nutrient additions that cascade across the landscape. This knowledge is crucial for a better understanding of OM cycling in a landscape context, in particular since terrestrial and aquatic compartments may respond differently to the ongoing changes in climate, land use, and other anthropogenic interferences.
Our mechanistic understanding of organic matter (OM) retention and turnover has undergone a profound paradigm shift as technological advances enable measurements and visualizations at ever smaller and at the same time larger scales. Many of these advances occurred in the fields of soil and dissolved OM chemistry as well as Earth observation science. The subsequent concepts and terminologies that have developed have been adapted in nearly all Earth Science disciplines. Conceptually, OM retention is now considered a matter of microbial access, which is governed by ecosystem properties including climate – in the case of permafrost – or organo-mineral and metal interactions, among other properties (Schmidt et al., 2011). Yet, controversy and often confusion remain as concepts supporting intrinsic chemical properties, such as “lability and recalcitrance” including refractory substances such as humic and fulvic acids have persisted in the literature and society presentations. We argue that this discussion is symptomatic of an emerging paradigm that, until now, has not adequately incorporated the multiple ideas and research avenues that served as a foundation of our past understanding. This process is predicted by adaptive management research (Holling, 2001) that anticipates a period of renewal in which resources or ideas are assembled in various often unsystematic ways to serve as the building blocks of something novel. This period of change is evident in the literature as OM retention and release has been addressed from different perspectives: at the ecosystem level (Schmidt et al., 2011), the terrestrial-aquatic connectivity concept (Marin-Spiotta et al., 2014), the priming frame work (Guenet et al., 2010; Bianchi, 2011), and the linkage of carbon cycling across various spatial and temporal scales (Premke et al., 2016). Thus, our goal is to present a novel framework of OM cycling that synthesizes many of these ideas, but primarily focuses on the flows of material and energy and their interactions across landscape properties and scales. We believe that such a framework is necessary in order to advance our current understanding of OM as the Earth’s systems rapidly respond to unprecedented and rapidly increasing climate and anthropogenic impacts.
Climate and anthropogenic impacts cascade through the spatial and temporal scales that exist across the landscape and are made evident in OM changes, C transfer rates, changes to the environment. OM processing, or the landscape specific biotic and abiotic actions and properties that lead to OM turnover, is further modulated by component ecosystem properties, not the least of which is the composition of decomposer and microbial communities present in terrestrial, wetland or aquatic habitats. Thus, OM processing at the landscape scale can be understood as a function of the OM substrate and its pathway, or transit, across the landscape (Figure 1). Consequently, predicting the extent and manifold consequences of global change on Earth systems as well as their feedbacks on global climate requires a better knowledge of OM and carbon (C) cycling not only in individual ecosystems, i.e., aquatic or terrestrial, but also at the larger landscape scale (e.g., Regnier et al., 2013; Reichstein et al., 2013).
Figure 1. Organic matter pathways across a landscape with natural structures. Different flows across the landscape are depicted along with frequency histograms of turnover. The colors within the histograms refer to the relative spatio-temporal impacts on turnover (see Table 1 and Figure 4). Insets (A–C) highlight the impact of transitions between different ecosystems and how the turnover rates change as OM is transferred from one to another.
An overwhelming determining factor of landscape scale OM turnover is the anthropogenic addition of external OM, removal of internal OM, and the restructuring of OM pathways (Figure 2). There is substantial evidence of this impact on nitrogen and carbon cycling at the global scale (De Vries et al., 2013); however, anthropogenic activities that change the structure and connectivity of landscape components, affecting interfaces and land use properties, can have major effects on the rate (reactivity and mobilization) of OM exchange and transformation as well as its pathways through the landscape. Therefore, anthropogenic impacts at different landscape spatio-temporal scales are a necessary addition toward understanding OM landscape dynamics. Yet, it has been greatly neglected in current concepts of OM cycling.
Figure 2. Organic matter pathways across a landscape with anthropogenic structures. Different flows across the landscape are depicted along with frequency histograms of OM turnover. The colors within the histograms refer to the different spatio-temporal impact on turnover (see Table 1 and Figure 4). Insets (A–C) highlight the impact of flow reversals and structural changes on OM turnover.
We focus on three main concepts toward explaining OM turnover at the landscape scale: the landscape spatio-temporal context, OM turnover described by priming and ecological stoichiometry, and anthropogenic effects as a disruptor of OM transfer, transformation rates, and pathways. We introduce a spatio-temporal model as a heuristic approach to integrate the many abiotic, biotic, and anthropogenic dimensions that describe OM transformations across the landscape.
Landscapes are spatially heterogeneous entities encompassing terrestrial and aquatic domains tightly connected by flows of OM, energy, and organisms across ecosystem boundaries (Loreau et al., 2003; Premke et al., 2016). Analogous to the meta-population concept, this complex array of ecosystems has been expressed as a “meta-ecosystem” (Loreau et al., 2003), in which both biotic and abiotic factors vary among entities including OM transformation processes acting at a wide range of temporal and spatial scales, e.g., from rapid OM mineralization to long-term C stabilization at micro- to landscape scales. OM pathways through different ecosystems capture the temporal scale across the landscape, characterized by ecosystem connectivity and element transit times. The landscape’s mark on OM is a function of substrate, environmental conditions, nutrient and energy availability, and the biological metabolic requirements of heterotrophic organisms, many of which are strongly influenced by the 3D shape and topology of the landscape and its components.
Landscape-level OM turnover is thus not just determined by intrinsic properties of the OM per se or of individual ecosystems (i.e., landscape components), but also by variations in their spatial and temporal configuration and connectivity in the short- and long-term run. Marin-Spiotta et al. (2014) suggested that one of the primary differences between terrestrial and aquatic soils and sediments is the stability in structure and residence time of their solid matrix. Most terrestrial OM is cycled and mineralized internally (Gounand et al., 2018), an observation that forms the basis of classical analysis of OM decomposition and cycling (Olson, 1963); however, we suggest OM moving across the landscape greatly contributes to the variability in OM turnover at a site. We further contend that an integrative view of the processes and linkages between terrestrial and aquatic ecosystems from the micro- up to the landscape scale is necessary; one that emphasizes spatio-temporal changes in OM bioavailability and OM turnover along fluctuating environmental gradients, serving as a mechanistic basis behind OM and C dynamics. By taking a landscape perspective, we can extend the understanding of OM processing and turnover by considering not only unidirectional flows but also multidirectional OM transformation processes, e.g., OM deposition on land after flooding, drying of small water bodies, reciprocal OM transport by organisms (e.g., Scharnweber et al., 2014a) or anthropogenic activities such as dredging river systems.
The role of individual ecosystems and their connectivity in landscape OM turnover still remains unclear. At certain time points OM processes may be in a steady state, meaning that OM production and consumption are balanced within a system. At times when new OM does not enter the system and environmental factors remain stable for extended time periods, OM decomposition may be the dominant flux (Hofmann and Griebler, 2018), slow or even halt (Von Luetzow et al., 2006). Accelerated OM decomposition, however, often occurs during fluctuations in environmental conditions, e.g., during OM transfer into another ecosystem (i.e., terrestrial to aquatic or vice versa) or change in saturation conditions within a year as with hydroperiod, which may result in changes of redox-conditions or light-induced effects, etc. Many ecosystems are highly dynamic in space and time and thus OM properties and microbial degradation constantly vary, e.g., differences in microbial availability of humic matter differ with redox state (Lovley et al., 1996; Kluepfel et al., 2014). Given that OM turnover rates are rapid at transition zones where environmental changes are most pronounced (Krause et al., 2017), OM mobility in and between ecosystems and thus ecosystem connectivity becomes a major factor determining microbial transformations. As such, the structure and diversity of landscape elements, i.e., meta-ecosystems, and changes to meta-ecosystem properties, can be crucial drivers of OM turnover at the landscape and even global scale.
To integrate the points discussed above, we suggest that a hierarchical spatio-temporal model of different landscape processes and properties is helpful toward conceptualizing OM dynamics at the larger, landscape and global scale (Table 1). Four levels describe the model structure. (1) Non-steady state unidirectional flows: occur at the largest spatial scale and are events that transfer a large amount of OM over relatively short time periods. (2) Steady state uni- and bidirectional lateral transfers: relatively small periodic and diffuse transfers across aquatic and terrestrial domains and ecosystems and vice versa. (3) Ecosystem properties: changes in connectivity and interfaces between landscape components. Thereby, the previous scale focuses on the movement of OM, while the environment to which the OM is presented may shift the status of OM availability. Environmental properties such as redox conditions, heterotrophic (microbial) demand, and others may render OM more or less biologically available for decomposition. (4) Substrate properties: at the smallest spatial scale is the microbial processing of OM matter that occurs nearly continuously via microbial activities.
The landscape perspective is necessary to account for the spatio-temporal changes in relative conditions the OM arrives for microbial processing, as transfers from different scales will impact the role of priming and ecological stoichiometry occurring at the substrate level. In the following sections, we discuss each spatio-temporal level and provide examples of different landscape processes within the context of current ecological and biogeochemical theory. We hypothesize that the impacts of higher-level landscape transfers and rate-controlling environmental conditions need to be accounted for to fully understand OM turnover – also at the substrate level. We conclude with hypotheses to test the concepts we outline.
Mass and energy generally flow downhill (Lindeman, 1942; Gounand et al., 2018), the flow rates across landscapes, however, might differ. Events that occur within a landscape that transport large amounts of material in a relatively short period of time have important implications for OM processing. These events are disturbances that occur over a large spatial extent and fall within a regular temporal frequency in which the reoccurrence of large-magnitude events is rare. For example, an ecosystem with seasonal flooding may develop riparian areas that retain fluvially transported OM, yet these areas may be overwhelmed with a 100-year return interval flooding event. The thresholds of quasi-steady-state material flow may also be breached by events occurring in tandem such as large precipitation events following a severe wildfire. Landslides, erosion, and leaching are other events that are unidirectional transfers but they may proceed at a slower transfer rate. Depending on the magnitude of the event, the OM transported across the landscape has the potential to bypass what might be considered points of steady state processing, such as the riparian areas referenced in the previous example. In these cases, aquatic systems may receive relatively young terrestrial inputs that have not been pre-processed by resident terrestrial microbial communities presenting potential elemental and enzymatic limitations (Veen et al., 2015) or result in a feedback loop altering existing aquatic carbon cycling as shown for a sudden lake brownification event (Brothers et al., 2014). Larger events might effectively and massively remove OM through burial or through transport out of the landscape boundaries.
Transfers of OM are well recognized as important processes in landscape ecology that are facilitated by abiotic and biotic vectors (Wiens et al., 1985). Actions of abiotic vectors include windfall, leaching, and currents, while biotic vectors may include migration, foraging, and life-cycle patterns. In the case of carbon (C), these transfers are estimated to range between 10-3 and 105 gC m-2 globally (Gounand et al., 2018). A global meta-analysis of C transfers shows that highly productive ecosystems, primarily terrestrial systems, contribute to cross ecosystem transfers, although anthropogenic transfers were not accounted for (Gounand et al., 2018). Transfers across landscapes also vary in their elemental composition that may disproportionately impact the recipient ecosystem (Bartels et al., 2012). For example, the carbon, nitrogen, and phosphorus stoichiometry of detrital versus organism-derived OM can vary widely and can increase or decrease the elemental ratios of both the recipient organism and the ecosystem the material is transferred to Sitters et al. (2015). How these transfers impact OM turnover and nutrient biogeochemistry at the landscape scale, is not well known, although the aquatic-terrestrial exchanges are hypothesized to be important drivers.
The classical view of OM transport is unidirectional from vegetation to soil (Pausch and Kuzyakov, 2018), groundwater, or humans into surface water bodies (Carpenter et al., 1998, 2005; Smith and Schindler, 2009; Premke et al., 2010; Mehner et al., 2018). This view is supported by the fact that much of the OM in waters carry a terrestrial signature (Bianchi et al., 2002; Bianchi, 2011), although the use of terrestrial OM in aquatic food webs strongly depends on aquatic productivity (Mehner et al., 2016). However, so far a greatly neglected mechanism is active biological transport, e.g., aquatic insects (Reinhardt et al., 2013; Scharnweber et al., 2014b), fish/bear, birds (Bartels et al., 2012) and increasingly humans moving substantial amounts of OM, energy, and particularly nutrients from water to land (Hoekman et al., 2012; Dreyer et al., 2015). This may provide important carbon, energy, and nutrient sources for terrestrial ecosystems (Gratton et al., 2008; Vander Zanden and Gratton, 2011; Dreyer et al., 2015). The ecological relevance of OM transport across ecosystem borders has been highlighted by rapid microbial mineralization of old, terrestrial OM when channeled into aquatic ecosystems (McCallister and Del Giorgio, 2012).
The connectivity of ecosystems and structures across a landscape is paramount to OM processing (Covino, 2017). Hydrologic connectivity is multidimensional spatially (μm to km) and temporally (seconds to decades) and facilitates many of the transfers described at the larger scales. As an ecosystem property, the degree to which and how an individual ecosystem is connected will have large implications for OM turnover.
Bidirectional processes can greatly alter OM transformation and turnover by frequent changes in environmental conditions which then set the framework for further OM processing (Premke et al., 2016). The transition from one environment to another during OM movement across ecosystem boundaries greatly determines the environmental settings for OM degradation; not only due to changes in the availability of OM and nutrients, but also due to abrupt changes or gradients in many environmental features controlling microbial and decomposer community composition and their related activities. These conditions may emerge when ecosystems overlap forming zones of transition, ecotones, or areas that are different from the parent ecosystems in physical, chemical, and biotic characteristics. Many examples can be drawn from across the landscape, including areas between forests, grassland, or agricultural systems to structures such as hedgerows or other fragmented landscape structures. However, the starkest transitions occur between aquatic and terrestrial ecosystems.
Aquatic-terrestrial and ecohydrological interfaces (e.g., littoral zones, sediments, water-atmosphere, inorganic and organic particles) are hotspots of OM transfer in the landscape (McClain et al., 2003). The increase in microbial activity is generally attributed to steep gradients in physico-chemical and biological characteristics that underlie the linkages between terrestrial and aquatic processes including photo-oxidation, redox fluctuations, and surface/matrix-effects. Furthermore, as OM moves from the terrestrial to the aquatic domain and vice versa (Scharnweber et al., 2014b), availability of suitable electron acceptors (e.g., O2, , ) and light can change dramatically, greatly affecting chemical and microbial OM transformations and hence OM persistence. The impact of a fluctuating environment affects not only material transported across ecosystem boundaries but also resident OM that might encounter a shift in conditions periodically over a year. Thus, the extent of environmental gradients and the different ecosystem properties that create these gradients (e.g., hydroperiod) within the landscape will contribute to OM turnover independent of substrate composition per se.
The persistence of specific OM fractions, despite their potential as energy sources, is a phenomenon shared by both aquatic and terrestrial ecosystems. Recent studies point to the fact that OM persistence is an emergent system property within the context of physical, biological, and climatic ecosystem characteristics, rather than the inherent OM chemical composition or structure (Schmidt et al., 2011; Ahrens et al., 2015). This is consistent with the rubric presented in Table 1, in which OM turnover is a product of its source, age, and time in transit, as well as its environment, including gradients or transitions in electron donors, pH, or solubility. OM turnover, presented at the smallest scale in the hierarchy, is ongoing at nearly all points within the landscape afforded with substrate and microbial communities; yet, the range of OM turnover rates within an ecosystem varies widely due to the OM pathway through the landscape. Ultimately, the bottleneck of OM turnover lies in microbial functioning, requiring a review of mechanisms controlling microbial activity in both aquatic and terrestrial systems.
Priming and ecological stoichiometry are two concepts commonly used to explain alterations in the stability and degradation of OM in soils, sediments, and open water. Priming refers to the phenomenon that relates the interdependency of turnover of different OM pools (i.e., accessible versus inaccessible), with assumed contrasting microbial availability at given environmental conditions, resulting in retention of one pool and release of another (Kuzyakov et al., 2000; Guenet et al., 2010; Bianchi, 2011; Huo et al., 2017). Priming effects have also been described for aquatic ecosystems (Guenet et al., 2010; Bianchi, 2011), although their importance in those environments is still a matter of debate (Bengtsson et al., 2018) since evidence for priming in aquatic environments: has been limited to a few experiments with often little defined microbial sources and environmental settings (Kuehn et al., 2014; Ward et al., 2016), was circumstantial (Kankaala et al., 2013; Guillemette et al., 2016; Fabian et al., 2017), or was absent (van Nugteren et al., 2009; Bengtsson et al., 2014; Catalan et al., 2015; Hofmann and Griebler, 2018).
Ecological stoichiometry is the maintenance of the elemental ratio (C, N, and P) of (micro)organisms that is controlled by quality and quantity of available substrate for metabolism (Elser et al., 2007) and is also a mechanism for OM transformation applied in aquatic and terrestrial research (Glibert, 2012; Zechmeister-Boltenstern et al., 2015). At low substrate concentrations, organic carbon is primarily used in catabolic reactions providing energy to the cell but resulting in low bacterial growth efficiency (Russell and Cook, 1995) and low substrate quality (more oxidized substrate) will result in low bacterial growth efficiency (Vallino et al., 1996; Hertkorn et al., 2002; Eiler et al., 2003; Nebbioso and Piccolo, 2013). The results of (1) an increase in respiration rates at the expense of (microbial) biomass production under nutrient limitation (Manzoni et al., 2012), and (2) a mismatch in stoichiometry between bioavailable OM and organismic biomass may account for variations in OM degradation and transformation across landscape compartments leading to pronounced differences in OM elemental composition (Sardans et al., 2012).
Priming and ecological stoichiometry are not mutually exclusive but rather complementary to each other (Chen et al., 2014) and seldom are they considered in connection with each other. We suggest that the negative or positive priming effects induced by the addition of OM, is related to mechanisms of microbial growth and stoichiometry for specific microbial source communities and environmental settings. Based on the suggestions of Blagodatskaya and Kuzyakov (2008), we propose two hypotheses responsible for the priming effect (Figure 3) that are in agreement with the concept put forward by Schmidt et al. (2011).
Figure 3. Conceptual schematic of organic matter (OM) decomposition through the landscape at the substrate level including different mechanisms (A), and temporal and spatial hotspots such as extreme weather and strong biogeochemical gradients along interfaces (B). In panel (A) OM decomposition is depicted under constant environmental conditions (linear response). Different mechanisms (M1, M2) and factors (e.g., influx of material, stoichiometry, heterotrophic demand, electron availability, energy, redox changes, light etc.) have the potential to either increase or decrease OM decomposition rate. Thus, the priming effect (PE) can appear as either positive or negative. In the case where OM moves between different landscape compounds (aquatic/terrestrial), mechanisms and time scale determine OM fate and hence microbial transformation (B). Environmental changes at transition zones and specific temporal events (e.g., thunderstorm, flooding) or anthropogenic impacts into account will affect the fate of OM, for example, sediment burial will greatly reduce OM processing.
H1 A Change in stoichiometry as a result of carbon addition.
As soil microbes are often carbon limited (Demoling et al., 2007), addition of a carbon source should remove this limitation and in turn other nutrients such as nitrogen might become limiting (Dijkstra et al., 2017; Chen et al., 2019). As soil OM might contain these now limiting substances it will be degraded, N will be incorporated into the microbial biomass, and additional carbon from this source will be mineralized. Nutrient limitations, such as nitrogen, decrease microbial carbon-use-efficiency potentially leading to soil OM destabilization (Manzoni et al., 2012).
H2 An increase in microbial biomass due to the removal of carbon limitation.
The soil volume occupied by microorganisms is assumed to be less than 1% (Ekschmitt et al., 2008; Schmidt et al., 2011) and thus an increase in biomass could constitute a first trigger to greater spatial exploitation of soil OM, partially counteracting the spatial disconnect between soil carbon and decomposing microbes (Powlson et al., 2001). New technological advances in imaging mass spectrometry (Watrous and Dorrestein, 2011) allow for direct visualization or location specific assessment of biological and chemical changes within OM particles. For example, nanoscale secondary ionization mass spectrometry (NanoSIMS) as well as Raman microscopy can provide a location specific assessment of microbial processing on individual soil or sediment particles (Remusat et al., 2012; Mueller et al., 2013). Similarly matrix assisted laser desorption/ionization coupled to different mass detectors can provide high spatial resolution of metabolic shifts within the rhizosphere (Veličković and Anderton, 2017).
Because of the different matrices of soil and the water column (Marin-Spiotta et al., 2014), the discussion above focused on OM dynamics in soil. For example, mechanisms may be water column dependent. In littoral sediments, where light is available, heterotrophic microorganisms co-occur with algae resulting in a close link of phototrophic and heterotrophic processes and a large mass transfer of OM (Grossart et al., 2006; Kirchman, 2018). Thus, there is an on-going discussion of possible OM turnover mechanisms (M1 and M2 in Figure 3) in aquatic systems that are based on chemical properties of substrate and microbial demand, gross primary production, and community composition. Several studies suggest that exudates of photosynthetic metabolites stimulate, in a sense prime, the degradation of terrestrial OM (Danger et al., 2013; Kuehn et al., 2014). While, experiments that introduced refractory terrestrial particulate OM have found support for enhanced microbial activity (e.g., Halvorson et al., 2016; Ward et al., 2016) and against it (e.g., Attermeyer et al., 2013). Differences in chemical composition may also contribute to OM turnover at the substrate level. Photosynthetic exudates consist primarily of simple carbohydrates and amino acids and thus represent a readily available and rapidly metabolized source of energy and nutrients to the heterotrophic community (Espeland et al., 2001; Kuehn et al., 2014). Similarly, there is recent evidence of algal exudates stimulating terrestrial OM turnover as a result of functional shifts towards a greater contribution of bacteria, especially Bacteroidetes and Actinobacteria, along with in a shift in substrates from leaf to algal C by Proteobacteria (J. Fabian, pers. comm.). However, observed differences among bacterial groups are likely related to their distinct contribution to terrestrial OM decomposition, which depends on their catabolic capabilities.
At the substrate level, OM decomposition is predominantly driven by microbial processes and is potentially limited by the availability of terminal electron acceptors, energy supply as well as availability and ratio of essential nutrients and elements. In aquatic systems, for example, it has been shown that organic carbon accumulates when N and P are limiting (Thingstad et al., 2008). However, neither priming nor environmental stoichiometry accounts for the fact that OM degradation, even of relatively bioavailable OM in the presence of sufficient nutrients, can be limited by the availability of suitable electron acceptors (Emerson and Hedges, 2003). Moreover, OM turnover rates can be greatly affected by changes in specific environmental features, which are neither included in the concept of priming nor ecological stoichiometry. For example, microbial OM transformations may well be controlled by the presence of light and subsequent photooxidation processes in surface waters, or sorption of OM and nutrients to surfaces in soils and subsurface sediments. Neither priming nor ecological stoichiometry can fully explain current observations of OM transformation without accounting for processes and events that occur at a larger spatio-temporal scale.
H3 Intrinsic chemical recalcitrance remains a mechanism of organic matter retention.
Arguments for intrinsic recalcitrance may still exist for conditions where OM is present and the environment is static and transport potential is low (e.g., the deep water layers of permanently stratified or meromictic lakes); yet OM persists despite its availability for microbes. For instance, Kellerman et al. (2015) concluded that intrinsic molecular interactions explain recalcitrance in aquatic systems. Similarly, studies on humic substances in soils argue for chemical recalcitrance as an important mechanism of OM retention (Schnitzer and Monreal, 2011; Ghabbour et al., 2017). In this context, our understanding that some natural organic molecules can resist microbial decomposition because of their specific molecular properties forms the basis of the biogeochemical paradigm of intrinsic recalcitrance. Additional studies are needed to clarify the molecular make-up of OM that persists due to this mechanism as well as to explain the metabolic obstacles of these compounds to microbial breakdown at specific environmental conditions.
We can infer from the discussion above that OM properties within the landscape are described by two dominant properties that capture OM turnover potential: the substrate potential for microbial processing and the environment the OM is exposed to Figure 4. Within this construct we can conceptualize how the aforementioned landscape properties can impact the status of OM availability as a function of its pathway across the landscape. In general, we expect processing rates to be largest at the substrate scale and the largest impact on the environment to arise from the largest disturbance or unidirectional flow. The maximum rates are constrained by the physical landscape, such as lithology or geomorphology, in the case of processing rates, and disturbance regime in the case of impacts on the local environment. The response trajectory following these constraints are determined by different landscape properties and processes, summarized in the table embedded in Figure 4, and discussed in detail below.
Figure 4. Conceptual model of different spatio-temporal level impacts on potential OM processing and the environment in a hypothetical landscape. The responses are expected to shift for each landscape determined by the relative impact of the spatio-temporal levels listed along the x-axis. Examples of different impacts are provided in the accompanying table of slope shape forming factors. The spatio-temporal hierarchy forms the basis for the various colors represented in the histograms of Figures 1, 2.
Impact on the immediate processing of OM is expected to be low and not vary turnover rates beyond physical or chemical transformation capacities. For example, a landslide might physically break up aggregates or a wildfire will oxidize OM, chemically transforming what remains. Additionally, given a high unidirectional flow of material the impact on processing could be large since OM is anticipated to have bypassed many of the pre-processing that occurs within a resident ecosystem. The impact of non-steady-state unidirectional flows and disturbances on the environmental control of OM transformation is expected to be very large, including potentially changing the light regimes resulting in growth release, or shifting climate that leads to the melting of permafrost and the release of previously climate stabilized OM. Anthropogenic inputs resulting from dredging or irrigation also play a role that presents a reverse flow that may destabilize OM cycling (Van Cappellen and Maavara, 2016).
This level is distinguished from the higher level by the assumption that the decomposer community anticipates or has evolved to expect the chemical and physical characteristics of the substrate transferred. The processing rates may increase or decrease with elemental composition of the OM transferred depending on the microbial community composition and status. The transfer magnitude is productivity dependent, demonstrated previously by the transfer of C from highly productive terrestrial ecosystems to relatively low productive aquatic systems. Different species traits will also impact these transfers as well, such as native versus exotic species, migration distance, or traits that feedback to higher spatio-temporal level, such as shifting a disturbance regime. While transfers will not directly impact the environment, indirect cases may exist, for example, management activities might introduce concentrated grazing to a landscape the impact of which may cause interrupted hydrologic flow paths or changes in vegetation status.
Landscapes characterized by transition zones, distinct hydroperiods or hydrologic regimes that feature punctuated events such as overland flow can accelerate OM turnover. Ecosystem connectivity facilitates OM imports and exports and nutrient exchange in the critical zone, hyporheic zone, and between the surface and groundwater (Julian et al., 2016; Covino, 2017). The number and variation of these features within the landscape will dictate the overall impact on OM processing. Ecosystem properties dictate the immediate environment (i.e., temperature, light or moisture) OM is exposed to, thus having an important impact on OM turnover. Biota can potentially impact the environmental conditions by shading, root penetration, or evaporative cooling. In aquatic systems, primary producers can significantly affect light penetration and oxygen supply (e.g., Brothers et al., 2014).
Priming accounts for the influx quantity or transfer of OM to a site while ecological stoichiometry unites the substrate composition to the microbial demand. Thus, how OM travels across the landscape spatio-temporal continuum and how the chemical status changes during its transit influences the potential for OM turnover. For example, OM that has been exposed to a suite of environmental conditions and microbial communities as it travels through a landscape will be conditioned for further processing very differently than OM with a much faster transit. Given the small scale of substrate, we expect minimal impact of substrate on the environment, although OM content within soils is known to impact decomposition through moisture holding capacity, pH, and nutrient retention. Benthic algae, fungi and bacteria contained within biofilms can affect particle deposition and transport (Dodds and Biggs, 2002; Salant, 2011; Wilkes et al., 2018) and have been suggested to have interactive effects that can influence channel morphology (Atkinson et al., 2018). Particulate OM presents important interfaces where microbial transformation of OM is increased by increased nutrient availability and often intense interactions at the microscale (e.g., Grossart, 2010).
H4 Pathways through the landscape will be imprinted on OM molecular composition.
In the terrestrial domain, OM and matrix characteristics are used to understand the sources of OM, the means of transport (e.g., baseline versus event flow in the case of sediments), and the age of the material. OM chemical characteristics are regularly achieved through stable isotope methods, fallout radionuclides, and elemental composition to identify sources of material transported by erosion (Laceby et al., 2017). Particle size, distribution, and mineral-metal composition can provide complementary information regarding transport velocities, weathering, and hydrologic conditions (e.g., exposure to fluctuating redox conditions). In addition to fingerprint methods, direct measurements OM age, through radiocarbon measurements (Marwick et al., 2015) or metabolic availability indicated by O/C content versus H/C content (Kim et al., 2003; Williams et al., 2018) can be achieved. When the OM chemical composition and matrix properties are used in combination then a composite picture is assembled similar to the turnover frequency histograms depicted in Figures 1, 2. Similarly, dissolved chemical mixtures from watershed draining streams and potentially waterbodies within a watershed are hypothesized to capture element sources, changes in land-use, and the impact of anthropogenic changes within a watershed (Kaushal et al., 2018).
In addition to empirical estimates of OM turnover and the spatio-temporal elements that influence these, modeling of landscapes and watersheds can provide estimates at the systems level and simultaneously point to areas of uncertainty. Probabilistic modeling of elemental transit time and age have been shown to diverge strongly due to the complex flow paths of atoms through interconnected pools across a landscape (Metzler et al., 2018). Mechanistic modeling of stream channels have provided insight into the complexity of hydrologic exchanges with the terrestrial-aquatic interface (Dwivedi et al., 2018) or with changes in stage flow due to dam regulation (Song et al., 2018). The combination of empirical and analytic approaches will help in constraining the age, turnover, and transit of OM through a landscape.
The conceptual model we present is not comprehensive in explaining the different spatio-temporal levels impact on processing and the environmental conditions leaving several open questions. In Figure 4, we suggest that the bar width of each spatio-temporal level conveys the impact potential of each level on the OM processing potential and environmental control responses. For example, a landscape with fewer transition zones or experiencing an infrequency of large disturbance events may be smaller (length along the x-axis) than what is depicted in the graph, which then corresponds to a smaller impact on both response curves. The model allows for synergy between levels, illustrated by overlap of the different levels, shorter overlaps may indicate a younger landscape while a larger degree of overlap indicates transitions that may have come to steady state or possible legacy effects developed from previous disturbances or land-use. Furthermore, the model does not concisely represent feedbacks between different levels, for example, the breakdown of substrate from a particulate to soluble phase, which then affects transfer rates. It is likely that most attempts to compartmentalize a continuous process will be fraught with inaccuracies, but such models help constrain the large number of dimensions involved in understanding OM turnover at the landscape scale.
Current global changes in conjunction with increasing anthropogenic activities (i.e., global climate change and land use practices) have an enormous effect on OM transformation in a landscape leading to new steady states or even a destabilization of OM cycling. Anthropogenic activities, such as agriculture, have the potential to greatly exacerbate carbon inputs to inland waters with major environmental consequences (Carpenter et al., 1998). The increase of nitrogen inputs into terrestrial systems cascade through the landscapes resulting in not only eutrophication downstream but also the acceleration of carbon and phosphorus cycling (Gruber and Galloway, 2008). Unidirectional flows from upland and urban ecosystems also have indirect effects including toxins released downstream affecting decomposer communities and the environment (Schulz et al., 2015).
Many of the transfers previously discussed stem from what might be called natural or management independent events, but anthropogenic activities have the potential to reciprocate the transfer of OM. Although anthropogenic activities have greatly increased the flux of OM from terrestrial to aquatic ecosystems via increasing erosion, recent anthropogenic activities also increase result in an accelerated return of “aquatic” OM (e.g., dams, dredging ditches, flooding, irrigation) or decrease (e.g., channelization of rivers) the backflow of OM from aquatic to terrestrial ecosystems. For example, dredging and sediment removal for port development and maintenance increase OM fluxes (Eyre et al., 1998). In addition, the anthropogenically increased backflow of dissolved and particulate OM from aquatic to soil ecosystems via irrigation (Schmitter et al., 2012) – the major anthropogenic water consumption, has yet to be robustly quantified. Furthermore, increasing the simultaneous backflow of OM and nutrients from aquatic to terrestrial systems presumably intensifies microbial activities and hence OM cycling in the landscape.
Anthropogenic effects on OM cycling also occur on the level of connectivity between landscape components. Draining of wetlands and regulation of fluvial ecosystems are examples for activities that decrease the residence time of water and thus the wetness of the terrestrial-aquatic interfaces. The wetness of the landscape is strongly linked to the potential to mobilize matter from soils to aquatic systems (Musolff et al., 2018). Channelization of streams and rivers disconnects the aquatic and terrestrial ecosystems in the landscape, reducing nutrients and OM inputs, and altering soil moisture and temperature regimes. In addition, the sharply increasing number of recently built and projected dams has huge implications (Zarfl et al., 2015), but little recognized impacts on OM flows. Furthermore, channelization, damming, and other landscape modifications lead to the simplification of the landscape, disrupting water and OM flow that have substantial feedbacks to biota, the environment, and OM turnover (Peipoch et al., 2015). Thus, OM flow across the landscape is increasingly determined by anthropogenic activities and greatly changes spatio-temporal patterns of OM transformation across landscape boundaries.
Finally, both priming and nutrient-to-carbon stoichiometry are heavily affected by human alterations. Nutrient levels are strongly elevated above natural background levels with different trajectories for N and P loading over the last decades (Dupas et al., 2018). Concentrations of organic carbon and thus the potential for priming can strongly increase either by sewage import or by eutrophication due to enhanced nutrient concentrations and corresponding exudation of labile organic carbon.
As Figure 2 illustrates, anthropogenic changes have the potential to cause large shifts in OM turnover by altering landscape structures and OM flow paths. Many of the impacts have been recognized, but robust evaluations are lacking for most. Assessment of in situ OM (i.e., natural OM) will most likely be similar to the techniques described previously. In the case of OM flow reversals, it is expected that a unique chemical signature will be left on the remaining OM, likewise with OM transfers (e.g., OM input from grazing animals). Anthropogenic imprints may be readily apparent, particularly when derivative structures are present that indicate synthetic chemicals in the form of microplastics (Strungaru et al., 2018), pharmaceuticals, pesticides, and herbicides (Kaushal et al., 2018). Already, these forms, such as plastics, are found in microaggregates (De Souza Machado et al., 2018) and pharmaceuticals and other water-soluble chemicals can be traced through watersheds (Kaushal et al., 2018). The dichotomy presented between Figures 1, 2, may already be superfluous as anthropogenic changes have altered global conditions through reactive nitrogen additions accelerating global cycles (Gruber and Galloway, 2008) or through CO2 fertilization changing biomass C:N ratios and subsequent OM decomposition rates (Fernández-Martínez et al., 2014), among others.
To fully appreciate OM turnover at the landscape scale, one needs to be a traveler following a path in two worlds, one world describes the elemental and microbial relationships involved in OM processing and the other the environmental conditions the OM is placed in. In effect, OM carries a legacy of its path across the landscape that ultimately reveals its turnover potential. OM age and transit times are a powerful means to accomplish this (Spohn and Sierra, 2018), but age does not convey the scars, bumps, and bruises the OM has accumulated along the way, and should also include the degree of processing, measured isotopically or spectroscopically, to tell its story. In other words, the question is: what are the state variables to fully describe the transient behavior of OM? As we suggest, the synthesis of priming and ecological stoichiometry may be one strategy to take this into consideration. Finally, the role humans have on directions of OM flows through the landscape cannot be ignored. The impact goes beyond reversing the traditional paradigm of mass flow from terrestrial to aquatic environments, but now includes restructuring OM pathways and processes, destabilizing mechanisms built over centuries, and greatly altering global OM cycling. Our spatio-temporal hierarchical model helps in specifying the human alterations of OM cycling on different levels in the landscape. Going forward, integrative approaches are needed to assess the landscape properties that alter OM transfers, environmental conditions, and substrate properties to achieve a better understanding of the universal mechanisms underlying OM cycling.
KP and ZK led the writing of the manuscript with particular support by H-PG, AG, SH, MW, and MG. All authors initially conceived the manuscript during a round-table discussion on landscape-scale carbon dynamics and contributed either to the development of the research question and discussion and/or the writing of the manuscript.
This work was supported by the German Research Foundation (DFG, grant number 60154). ZK was supported by the Idaho Water Resources Research Institute and from the USGS 104b program. The publication of this article was funded by the Open Access Fund of the Leibniz Association.
The authors declare that the research was conducted in the absence of any commercial or financial relationships that could be construed as a potential conflict of interest.
This manuscript originated from a round-table discussion on “Mechanistic understanding of carbon dynamics in coupled aquatic-terrestrial ecosystems under rapid environmental change” initiated by Klement Tockner.
Ahrens, B., Braakhekke, M. C., Guggenberger, G., Schrumpf, M., and Reichstein, M. (2015). Contribution of sorption, DOC transport and microbial interactions to the C-14 age of a soil organic carbon profile: insights from a calibrated process model. Soil Biol. Biochem. 88, 390–402. doi: 10.1016/j.soilbio.2015.06.008
Atkinson, C. L., Allen, D. C., Davis, L., and Nickerson, Z. L. (2018). Incorporating ecogeomorphic feedbacks to better understand resiliency in streams: a review and directions forward. Geomorphology 305, 123–140. doi: 10.1016/j.geomorph.2017.07.016
Attermeyer, K., Premke, K., Hornick, T., Hilt, S., and Grossart, H.-P. (2013). Ecosystem-level studies of terrestrial carbon reveal contrasting bacterial metabolism in different aquatic habitats. Ecology 94, 2754–2766. doi: 10.1890/13-0420.1
Bartels, P., Cucherousset, J., Steger, K., Eklov, P., Tranvik, L. J., and Hillebrand, H. (2012). Reciprocal subsidies between freshwater and terrestrial ecosystems structure consumer resource dynamics. Ecology 93, 1173–1182. doi: 10.1890/11-1210.1
Bengtsson, M. M., Attermeyer, K., and Catalán, N. (2018). Interactive effects on organic matter processing from soils to the ocean: are priming effects relevant in aquatic ecosystems? Hydrobiologia 822, 1–17. doi: 10.1007/s10750-018-3672-2
Bengtsson, M. M., Wagner, K., Burns, N. R., Herberg, E. R., Wanek, W., Kaplan, L. A., et al. (2014). No evidence of aquatic priming effects in hyporheic zone microcosms. Sci. Rep. 4:5187. doi: 10.1038/srep05187
Bianchi, T. S. (2011). The role of terrestrially derived organic carbon in the coastal ocean: a changing paradigm and the priming effect. Proc. Natl. Acad. Sci. U.S.A. 108, 19473–19481. doi: 10.1073/pnas.1017982108
Bianchi, T. S., Mitra, S., and Mckee, B. A. (2002). Sources of terrestrially-derived organic carbon in lower Mississippi River and Louisiana shelf sediments: implications for differential sedimentation and transport at the coastal margin. Mar. Chem. 77, 211–223. doi: 10.1016/s0304-4203(01)00088-3
Blagodatskaya, E., and Kuzyakov, Y. (2008). Mechanisms of real and apparent priming effects and their dependence on soil microbial biomass and community structure: critical review. Biol. Fertil. Soils 45, 115–131. doi: 10.1007/s00374-008-0334-y
Brothers, S., Koehler, J., Attermeyer, K., Grossart, H. P., Mehner, T., Meyer, N., et al. (2014). A feedback loop links brownification and anoxia in a temperate, shallow lake. Limnol. Oceanogr. 59, 1388–1398. doi: 10.4319/lo.2014.59.4.1388
Carpenter, S. R., Caraco, N. F., Correll, D. L., Howarth, R. W., Sharpley, A. N., and Smith, V. H. (1998). Nonpoint pollution of surface waters with phosphorus and nitrogen. Ecol. Appl. 8, 559–568. doi: 10.1890/1051-0761(1998)008
Carpenter, S. R., Cole, J. J., Pace, M. L., Van De Bogert, M., Bade, D. L., Bastviken, D., et al. (2005). Ecosystem subsidies: terrestrial support of aquatic food webs from C-13 addition to contrasting lakes. Ecology 86, 2737–2750. doi: 10.1890/04-1282
Catalan, N., Kellerman, A. M., Peter, H., Carmona, F., and Tranvik, L. J. (2015). Absence of a priming effect on dissolved organic carbon degradation in lake water. Limnol. Oceanogr. 60, 159–168. doi: 10.1007/s00248-017-0976-0
Chen, J., Seven, J., Zilla, T., Dippold, M. A., Blagodatskaya, E., and Kuzyakov, Y. (2019). Microbial C: N: P stoichiometry and turnover depend on nutrients availability in soil: A 14C, 15N and 33P triple labelling study. Soil Biol. Biochem. 131, 206–216. doi: 10.1016/j.soilbio.2019.01.017
Chen, R., Senbayram, M., Blagodatsky, S., Myachina, O., Dittert, K., Lin, X., et al. (2014). Soil C and N availability determine the priming effect: microbial N mining and stoichiometric decomposition theories. Glob. Change Biol. 20, 2356–2367. doi: 10.1111/gcb.12475
Covino, T. (2017). Hydrologic connectivity as a framework for understanding biogeochemical flux through watersheds and along fluvial networks. Geomorphology 277, 133–144. doi: 10.1016/j.geomorph.2016.09.030
Danger, M., Cornut, J., Chauvet, E., Chavez, P., Elger, A., and Lecerf, A. (2013). Benthic algae stimulate leaf litter decomposition in detritus-based headwater streams: a case of aquatic priming effect? Ecology 94, 1604–1613. doi: 10.1890/12-0606.1
De Souza Machado, A. A., Lau, C. W., Till, J., Kloas, W., Lehmann, A., Becker, R., et al. (2018). Impacts of microplastics on the soil biophysical environment. Environ. Sci. Technol. 52, 9656–9665. doi: 10.1021/acs.est.8b02212
De Vries, W., Kros, J., Kroeze, C., and Seitzinger, S. P. (2013). Assessing planetary and regional nitrogen boundaries related to food security and adverse environmental impacts. Curr. Opin. Environ. Sustainabil. 5, 392–402. doi: 10.1016/j.cosust.2013.07.004
Demoling, F., Figueroa, D., and Bååth, E. (2007). Comparison of factors limiting bacterial growth in different soils. Soil Biol. Biochem. 39, 2485–2495. doi: 10.1016/j.soilbio.2007.05.002
Dijkstra, F. A., Jenkins, M., De Rémy De Courcelles, V., Keitel, C., Barbour, M. M., Kayler, Z. E., et al. (2017). Enhanced decomposition and nitrogen mineralization sustain rapid growth of eucalyptus regnans after wildfire. J. Ecol. 105, 229–236. doi: 10.1111/1365-2745.12663
Dodds, W. K., and Biggs, B. J. (2002). Water velocity attenuation by stream periphyton and macrophytes in relation to growth form and architecture. J. North Am. Benthol. Soc. 21, 2–15. doi: 10.2307/1468295
Dreyer, J., Townsend, P. A., Hook, J. C. III, Hoekman, D., Vander Zanden, M. J., and Gratton, C. (2015). Quantifying aquatic insect deposition from lake to land. Ecology 96, 499–509. doi: 10.1890/14-0704.1
Dupas, R., Minaudo, C., Gruau, G., Ruiz, L., and Gascuel-Odoux, C. (2018). Multidecadal trajectory of riverine nitrogen and phosphorus dynamics in rural catchments. Water Res. Res. 54, 5327–5340. doi: 10.1029/2018wr022905
Dwivedi, D., Steefel, C. I., Arora, B., Newcomer, M., Moulton, J. D., Dafflon, B., et al. (2018). Geochemical exports to river from the intrameander hyporheic zone under transient hydrologic conditions: East River Mountainous Watershed, Colorado. Water Res. Res. 54, 8456–8477. doi: 10.1029/2018wr023377
Eiler, A., Langenheder, S., Bertilsson, S., and Tranvik, L. J. (2003). Heterotrophic bacterial growth efficiency and community structure at different natural organic carbon concentrations. Appl. Environ. Microbiol. 69, 3701–3709. doi: 10.1128/aem.69.7.3701-3709.2003
Ekschmitt, K., Kandeler, E., Poll, C., Brune, A., Buscot, F., Friedrich, M., et al. (2008). Soil-carbon preservation through habitat constraints and biological limitations on decomposer activity. J. Plant Nutr. Soil Sci. 171, 27–35. doi: 10.1002/jpln.200700051
Elser, J. J., Bracken, M. E. S., Cleland, E. E., Gruner, D. S., Harpole, W. S., Hillebrand, H., et al. (2007). Global analysis of nitrogen and phosphorus limitation of primary producers in freshwater, marine and terrestrial ecosystems. Ecol. Lett. 10, 1135–1142. doi: 10.1111/j.1461-0248.2007.01113.x
Emerson, S., and Hedges, J. (2003). “Sedimentary diagenesis and benthic flux,” in Treatise on Geochemistry, eds H. Elderfield, H. D. Holland, and K. K. Turekian (Seattle: University of Washington), 293–319. doi: 10.1016/b0-08-043751-6/06112-0
Espeland, E. M., Francoeur, S. N., and Wetzel, R. G. (2001). Influence of algal photosynthesis on biofilm bacterialproduction and associated glucosidase and xylosidaseactivities. Microb. Ecol. 42, 524–530. doi: 10.1007/s00248-001-1022-8
Eyre, B., Hossain, S., and Mckee, L. (1998). A suspended sediment budget for the modified subtropical Brisbane River estuary, Australia. Estuar. Coast. Shelf Sci. 47, 513–522. doi: 10.1006/ecss.1998.0371
Fabian, J., Zlatanovic, S., Mutz, M., and Premke, K. (2017). Fungal-bacterial dynamics and their contribution to terrigenous carbon turnover in relation to organic matter quality. ISME J. 11, 415–425. doi: 10.1038/ismej.2016.131
Fernández-Martínez, M., Vicca, S., Janssens, I. A., Sardans, J., Luyssaert, S., Campioli, M., et al. (2014). Nutrient availability as the key regulator of global forest carbon balance. Nat. Clim. Change 4:471. doi: 10.1016/j.scitotenv.2018.06.014
Ghabbour, E. A., Davies, G., Misiewicz, T., Alami, R. A., Askounis, E. M., Cuozzo, N. P., et al. (2017). National comparison of the total and sequestered organic matter contents of conventional and organic farm soils. Adv. Agron. 146, 1–35. doi: 10.1016/bs.agron.2017.07.003
Glibert, P. M. (2012). Ecological stoichiometry and its implications for aquatic ecosystem sustainability. Curr. Opin. Environ. Sustainabil. 4, 272–277. doi: 10.1016/j.cosust.2012.05.009
Gounand, I., Little, C. J., Harvey, E., and Altermatt, F. (2018). Cross-ecosystem carbon flows connecting ecosystems worldwide. Nat. Commun. 9:4825. doi: 10.1038/s41467-018-07238-2
Gratton, C., Donaldson, J., and Vander Zanden, M. J. (2008). Ecosystem linkages between lakes and the surrounding terrestrial landscape in northeast Iceland. Ecosystems 11, 764–774. doi: 10.1007/s10021-008-9158-8
Grossart, H. P. (2010). Ecological consequences of bacterioplankton lifestyles: changes in concepts are needed. Environ. Microbiol. Rep. 2, 706–714. doi: 10.1111/j.1758-2229.2010.00179.x
Grossart, H. P., Czub, G., and Simon, M. (2006). Algae–bacteria interactions and their effects on aggregation and organic matter flux in the sea. Environ. Microbiol. 8, 1074–1084. doi: 10.1111/j.1462-2920.2006.00999.x
Gruber, N., and Galloway, J. N. (2008). An Earth-system perspective of the global nitrogen cycle. Nature 451, 293–296. doi: 10.1038/nature06592
Guenet, B., Danger, M., Abbadie, L., and Lacroix, G. (2010). Priming effect: bridging the gap between terrestrial and aquatic ecology. Ecology 91, 2850–2861. doi: 10.1890/09-1968.1
Guillemette, F., Mccallister, S. L., and Del Giorgio, P. A. (2016). Selective consumption and metabolic allocation of terrestrial and algal carbon determine allochthony in lake bacteria. ISME J. 10, 1373–1382. doi: 10.1038/ismej.2015.215
Halvorson, H. M., Scott, E. E., Entrekin, S. A., Evans-White, M. A., and Scott, J. T. (2016). Light and dissolved phosphorus interactively affect microbial metabolism, stoichiometry and decomposition of leaf litter. Freshwater Biol. 61, 1006–1019. doi: 10.1111/fwb.12763
Hertkorn, N., Claus, H., Schmitt-Kopplin, P. H., Perdue, E. M., and Filip, Z. (2002). Utilization and transformation of aquatic humic substances by autochthonous microorganisms. Environ. Sci. Technol. 36, 4334–4345. doi: 10.1021/es010336o
Hoekman, D., Bartrons, M., and Gratton, C. (2012). Ecosystem linkages revealed by experimental lake-derived isotope signal in heathland food webs. Oecologia 170, 735–743. doi: 10.1007/s00442-012-2329-5
Hofmann, R., and Griebler, C. (2018). DOM and bacterial growth efficiency in oligotrophic groundwater: absence of priming and co-limitation by organic carbon and phosphorus. Aquat. Microb. Ecol. 81, 55–71. doi: 10.3354/ame01862
Holling, C. S. (2001). Understanding the complexity of economic, ecological, and social systems. Ecosystems 4, 390–405. doi: 10.1007/s10021-001-0101-5
Huo, C. F., Luo, Y. Q., and Cheng, W. X. (2017). Rhizosphere priming effect: a meta-analysis. Soil Biol. Biochem. 111, 78–84. doi: 10.1111/gcb.12816
Julian, J., Podolak, C., Meitzen, K., Doyle, M., Manners, R., Hester, E., et al. (2016). “Shaping the physical template: Biological, hydrological, and geomorphic connections in stream channels,” in Stream Ecosystems in a Changing Environment, eds J. B. Jones and E. H. Stanley (Amsterdam: Elsevier Academic Press), 85–133. doi: 10.1016/b978-0-12-405890-3.00002-6
Kankaala, P., Bellido, J. L., Ojala, A., Tulonen, T., and Jones, R. I. (2013). Variable production by different pelagic energy mobilizers in Boreal Lakes. Ecosystems 16, 1152–1164. doi: 10.1007/s10021-013-9674-z
Kaushal, S. S., Gold, A. J., Bernal, S., Johnson, T. A. N., Addy, K., Burgin, A., et al. (2018). Watershed ‘chemical cocktails’: forming novel elemental combinations in Anthropocene fresh waters. Biogeochemistry 141, 281–305. doi: 10.1007/s10533-018-0502-6
Kellerman, A. M., Kothawala, D. N., Dittmar, T., and Tranvik, L. J. (2015). Persistence of dissolved organic matter in lakes related to its molecular characteristics. Nat. Geosci. 8, 454–457. doi: 10.1038/ngeo2440
Kim, S., Kramer, R. W., and Hatcher, P. G. (2003). Graphical method for analysis of ultrahigh-resolution broadband mass spectra of natural organic matter, the van Krevelen diagram. Anal. Chem. 75, 5336–5344. doi: 10.1021/ac034415p
Kirchman, D. L. (2018). Microbial proteins for organic material degradation in the deep ocean. Proc. Natl. Acad. Sci. 115, 445–447. doi: 10.1073/pnas.1720765115
Kluepfel, L., Piepenbrock, A., Kappler, A., and Sander, M. (2014). Humic substances as fully regenerable electron acceptors in recurrently anoxic environments. Nat. Geosci. 7, 195–200. doi: 10.1038/ngeo2084
Krause, S., Lewandowski, J., Grimm, N. B., Hannah, D. M., Pinay, G., Mcdonald, K., et al. (2017). Ecohydrological interfaces as hot spots of ecosystem processes. Water Resour. Res. 53, 6359–6376. doi: 10.1002/2016WR019516
Kuehn, K. A., Francoeur, S. N., Findlay, R. H., and Neely, R. K. (2014). Priming in the microbial landscape: periphytic algal stimulation of litter-associated microbial decomposers. Ecology 95, 749–762. doi: 10.1890/13-0430.1
Kuzyakov, Y., Friedel, J. K., and Stahr, K. (2000). Review of mechanisms and quantification of priming effects. Soil Biol. Biochem. 32, 1485–1498. doi: 10.1016/s0038-0717(00)00084-5
Laceby, J. P., Evrard, O., Smith, H. G., Blake, W. H., Olley, J. M., Minella, J. P. G., et al. (2017). The challenges and opportunities of addressing particle size effects in sediment source fingerprinting: a review. Earth Sci. Rev. 169, 85–103. doi: 10.1016/j.earscirev.2017.04.009
Lindeman, R. L. (1942). The trophic-dynamic aspect of ecology. Ecology 23, 399–417. doi: 10.2307/1930126
Loreau, M., Mouquet, N., and Holt, R. D. (2003). Meta-ecosystems: a theoretical framework for a spatial ecosystem ecology. Ecol. Lett. 6, 673–679. doi: 10.1046/j.1461-0248.2003.00483.x
Lovley, D. R., Coates, J. D., Bluntharris, E. L., Phillips, E. J. P., and Woodward, J. C. (1996). Humic substances as electron acceptors for microbial respiration. Nature 382, 445–448. doi: 10.1038/382445a0
Manzoni, S., Taylor, P., Richter, A., Porporato, A., and Ågren, G. I. (2012). Environmental and stoichiometric controls on microbial carbon-use efficiency in soils. New Phytol. 196, 79–91. doi: 10.1111/j.1469-8137.2012.04225.x
Marin-Spiotta, E., Gruley, K. E., Crawford, J., Atkinson, E. E., Miesel, J. R., Greene, S., et al. (2014). Paradigm shifts in soil organic matter research affect interpretations of aquatic carbon cycling: transcending disciplinary and ecosystem boundaries. Biogeochemistry 117, 279–297. doi: 10.1007/s10533-013-9949-7
Marwick, T. R., Tamooh, F., Teodoru, C. R., Borges, A. V., Darchambeau, F., and Bouillon, S. (2015). The age of river-transported carbon: a global perspective. Glob. Biogeochem. Cycles 29, 122–137. doi: 10.1002/2014gb004911
McCallister, S. L., and Del Giorgio, P. A. (2012). Evidence for the respiration of ancient terrestrial organic C in northern temperate lakes and streams. Proc. Natl. Acad. Sci. U.S.A. 109, 16963–16968. doi: 10.1073/pnas.1207305109
McClain, M. E., Boyer, E. W., Dent, C. L., Gergel, S. E., Grimm, N. B., Groffman, P. M., et al. (2003). Biogeochemical hot spots and hot moments at the interface of terrestrial and aquatic ecosystems. Ecosystems 6, 301–312. doi: 10.1007/s10021-003-0161-9
Mehner, T., Attermeyer, K., Brauns, M., Brothers, S., Diekmann, J., Gaedke, U., et al. (2016). Weak response of animal allochthony and production to enhanced supply of terrestrial leaf litter in nutrient-rich lakes. Ecosystems 19, 311–325. doi: 10.1007/s10021-015-9933-2
Mehner, T., Rapp, T., Monk, C. T., Beck, M. E., Trudeau, A., Kiljunen, M., et al. (2018). Feeding aquatic ecosystems: whole-lake experimental addition of angler’s ground bait strongly affects omnivorous fish despite low contribution to lake carbon budget. Ecosystems 22, 346–362. doi: 10.1007/s10021-018-0273-x
Metzler, H., Müller, M., and Sierra, C. A. (2018). Transit-time and age distributions for nonlinear time-dependent compartmental systems. Proc. Natl. Acad. Sci. U.S.A. 115, 1150–1155. doi: 10.1073/pnas.1705296115
Mueller, C. W., Weber, P. K., Kilburn, M. R., Hoeschen, C., Kleber, M., and Pett-Ridge, J. (2013). Advances in the analysis of biogeochemical interfaces: nanoSIMS to investigate soil microenvironments. Adv. Agron. 121, 1–46. doi: 10.1016/b978-0-12-407685-3.00001-3
Musolff, A., Fleckenstein, J., Opitz, M., Büttner, O., Kumar, R., and Tittel, J. (2018). Spatio-temporal controls of dissolved organic carbon stream water concentrations. J. Hydrol. 566, 205–215. doi: 10.1016/j.scitotenv.2017.08.322
Nebbioso, A., and Piccolo, A. (2013). Molecular characterization of dissolved organic matter (DOM): a critical review. Anal. Bioanal. Chem. 405, 109–124. doi: 10.1007/s00216-012-6363-2
Olson, J. S. (1963). Energy storage and the balance of producers and decomposers in ecological systems. Ecology 44, 322–331. doi: 10.2307/1932179
Pausch, J., and Kuzyakov, Y. (2018). Carbon input by roots into the soil: quantification of rhizodeposition from root to ecosystem scale. Glob. Change Biol. 24, 1–12. doi: 10.1111/gcb.13850
Peipoch, M., Brauns, M., Hauer, F. R., Weitere, M., and Valett, H. M. (2015). Ecological simplification: human influences on riverscape complexity. BioScience 65, 1057–1065. doi: 10.1093/biosci/biv120
Powlson, D. S., Hirsch, P. R., and Brookes, P. C. (2001). The role of soil microorganisms in soil organic matter conservation in the tropics. Nutr. Cycl. Agroecosyst. 61, 41–51. doi: 10.1007/978-94-017-2172-1_5
Premke, K., Attermeyer, K., Augustin, J., Cabezas, A., Casper, P., Deumlich, D., et al. (2016). The importance of landscape diversity for carbon fluxes on the landscape level: small-scale heterogeneity matters. WIRES Water 3, 601–617. doi: 10.1002/wat2.1147
Premke, K., Karlsson, J., Steger, K., Gudasz, C., Von Wachenfeldt, E., and Tranvik, L. J. (2010). Stable isotope analysis of benthic fauna and their food sources in boreal lakes. J. North Am. Benthol. Soc. 29, 1339–1348. doi: 10.1899/10-002.1
Regnier, P., Friedlingstein, P., Ciais, P., Mackenzie, F. T., Gruber, N., Janssens, I. A., et al. (2013). Anthropogenic perturbation of the carbon fluxes from land to ocean. Nat. Geosci. 6, 597–607.
Reichstein, M., Bahn, M., Ciais, P., Frank, D., Mahecha, M. D., Seneviratne, S. I., et al. (2013). Climate extremes and the carbon cycle. Nature 500, 287–295. doi: 10.1038/nature12350
Reinhardt, T., Steinfartz, S., Paetzold, A., and Weitere, M. (2013). Linking the evolution of habitat choice to ecosystem functioning: direct and indirect effects of pond-reproducing fire salamanders on aquatic-terrestrial subsidies. Oecologia 173, 281–291. doi: 10.1007/s00442-013-2592-0
Remusat, L., Hatton, P.-J., Nico, P. S., Zeller, B., Kleber, M., and Derrien, D. (2012). NanoSIMS study of organic matter associated with soil aggregates: advantages, limitations, and combination with STXM. Environ. Sci. Technol. 46, 3943–3949. doi: 10.1021/es203745k
Russell, J. B., and Cook, G. M. (1995). Energetics of bacterial-growth - balance of anabolic and catabolic reactions. Microbiol. Rev. 59, 48–62.
Salant, N. L. (2011). ‘Sticky business’: the influence of streambed periphyton on particle deposition and infiltration. Geomorphology 126, 350–363. doi: 10.1016/j.geomorph.2010.08.015
Sardans, J., Rivas-Ubach, A., and Penuelas, J. (2012). The elemental stoichiometry of aquatic and terrestrial ecosystems and its relationships with organismic lifestyle and ecosystem structure and function: a review and perspectives. Biogeochemistry 111, 1–39. doi: 10.1007/s10533-011-9640-9
Scharnweber, K., Syvaeranta, J., Hilt, S., Brauns, M., Vanni, M. J., Brothers, S., et al. (2014a). Whole-lake experiments reveal the fate of terrestrial particulate organic carbon in benthic food webs of shallow lakes. Ecology 95, 1496–1505. doi: 10.1890/13-0390.1
Scharnweber, K., Vanni, M. J., Hilt, S., Syväranta, J., and Mehner, T. (2014b). Boomerang ecosystem fluxes: organic carbon inputs from land to lakes are returned to terrestrial food webs via aquatic insects. Oikos 123, 1439–1448. doi: 10.1111/oik.01524
Schmidt, M. W. I., Torn, M. S., Abiven, S., Dittmar, T., Guggenberger, G., Janssens, I. A., et al. (2011). Persistence of soil organic matter as an ecosystem property. Nature 478, 49–56. doi: 10.1038/nature10386
Schmitter, P., Fröhlich, H., Dercon, G., Hilger, T., Thanh, N. H., Lam, N., et al. (2012). Redistribution of carbon and nitrogen through irrigation in intensively cultivated tropical mountainous watersheds. Biogeochemistry 109, 133–150. doi: 10.1007/s10533-011-9615-x
Schnitzer, M., and Monreal, C. M. (2011). Quo vadis soil organic matter research? A biological link to the chemistry of humification. Adv. Agron. 113, 143–217. doi: 10.1016/b978-0-12-386473-4.00003-8
Schulz, R., Bundschuh, M., Gergs, R., Brühl, C. A., Diehl, D., Entling, M. H., et al. (2015). Review on environmental alterations propagating from aquatic to terrestrial ecosystems. Sci. Total Environ. 538, 246–261. doi: 10.1016/j.scitotenv.2015.08.038
Sitters, J., Atkinson, C. L., Guelzow, N., Kelly, P., and Sullivan, L. L. (2015). Spatial stoichiometry: cross-ecosystem material flows and their impact on recipient ecosystems and organisms. Oikos 124, 920–930. doi: 10.1111/oik.02392
Smith, V. H., and Schindler, D. W. (2009). Eutrophication science: where do we go from here? Trends Ecol. Evol. 24, 201–207. doi: 10.1016/j.tree.2008.11.009
Song, X., Chen, X., Stegen, J., Hammond, G., Song, H. S., Dai, H., et al. (2018). Drought conditions maximize the impact of high-frequency flow variations on thermal regimes and biogeochemical function in the hyporheic zone. Water Res. Res. 54, 7361–7382. doi: 10.1029/2018wr022586
Spohn, M., and Sierra, C. A. (2018). How long do elements cycle in terrestrial ecosystems? Biogeochemistry 139, 69–83. doi: 10.1007/s10533-018-0452-z
Strungaru, S.-A., Jijie, R., Nicoara, M., Plavan, G., and Faggio, C. (2018). Micro-(nano) plastics in freshwater ecosystems: abundance, toxicological impact and quantification methodology. TrAC Trends Anal. Chem. 110, 116–128. doi: 10.1016/j.trac.2018.10.025
Thingstad, T. F., Bellerby, R. G. J., Bratbak, G., Borsheim, K. Y., Egge, J. K., Heldal, M., et al. (2008). Counterintuitive carbon-to-nutrient coupling in an Arctic pelagic ecosystem. Nature 455, 387–437. doi: 10.1038/nature07235
Vallino, J. J., Hopkinson, C. S., and Hobbie, J. E. (1996). Modeling bacterial utilization of dissolved organic matter: optimization replaces Monod growth kinetics. Limnol. Oceanogr. 41, 1591–1609. doi: 10.4319/lo.1996.41.8.1591
Van Cappellen, P., and Maavara, T. (2016). Rivers in the Anthropocene: global scale modifications of riverine nutrient fluxes by damming. Ecohydrol. Hydrobiol. 16, 106–111. doi: 10.1016/j.ecohyd.2016.04.001
van Nugteren, P., Moodley, L., Brummer, G. J., Heip, C. H. R., Herman, P. M. J., and Middelburg, J. J. (2009). Seafloor ecosystem functioning: the importance of organic matter priming. Mar. Biol. 156, 2277–2287. doi: 10.1007/s00227-009-1255-5
Vander Zanden, M. J., and Gratton, C. (2011). Blowin’ in the wind: reciprocal airborne carbon fluxes between lakes and land. Can. J. Fish. Aquat. Sci. 68, 170–182. doi: 10.1139/f10-157
Veen, G., Freschet, G. T., Ordonez, A., and Wardle, D. A. (2015). Litter quality and environmental controls of home-field advantage effects on litter decomposition. Oikos 124, 187–195. doi: 10.1111/oik.01374
Veličković, D., and Anderton, C. R. (2017). Mass spectrometry imaging: towards mapping the elemental and molecular composition of the rhizosphere. Rhizosphere 3, 254–258. doi: 10.1016/j.rhisph.2017.03.003
Von Luetzow, M., Koegel-Knabner, I., Ekschmitt, K., Matzner, E., Guggenberger, G., Marschner, B., et al. (2006). Stabilization of organic matter in temperate soils: mechanisms and their relevance under different soil conditions - a review. Eur. J. Soil Sci. 57, 426–445. doi: 10.1111/j.1365-2389.2006.00809.x
Ward, N. D., Bianchi, T. S., Sawakuchi, H. O., Gagne-Maynard, W., Cunha, A. C., Brito, D. C., et al. (2016). The reactivity of plant-derived organic matter and the potential importance of priming effects along the lower Amazon River. J. Geophys. Res. Biogeosci. 121, 1522–1539. doi: 10.1002/2016jg003342
Watrous, J. D., and Dorrestein, P. C. (2011). Imaging mass spectrometry in microbiology. Nat. Rev. Microbiol. 9:683. doi: 10.1038/nrmicro2634
Wiens, J. A., Crawford, C. S., and Gosz, J. R. (1985). Boundary dynamics: a conceptual framework for studying landscape ecosystems. Oikos 45, 421–427.
Wilkes, M. A., Gittins, J. R., Mathers, K. L., Mason, R., Casas-Mulet, R., Vanzo, D., et al. (2018). Physical and biological controls on fine sediment transport and storage in rivers. Wiley Interdiscipl. Rev. Water 6:e1331. doi: 10.1002/wat2.1331
Williams, E. K., Fogel, M. L., Berhe, A. A., and Plante, A. F. (2018). Distinct bioenergetic signatures in particulate versus mineral-associated soil organic matter. Geoderma 330, 107–116. doi: 10.1016/j.geoderma.2018.05.024
Zarfl, C., Lumsdon, A. E., Berlekamp, J., Tydecks, L., and Tockner, K. (2015). A global boom in hydropower dam construction. Aquat. Sci. 77, 161–170. doi: 10.1007/s00027-014-0377-0
Keywords: landscape connectivity, organic matter mineralization, priming effects, ecological stoichiometry, aquatic-terrestrial interfaces, anthropogenic interferences
Citation: Kayler ZE, Premke K, Gessler A, Gessner MO, Griebler C, Hilt S, Klemedtsson L, Kuzyakov Y, Reichstein M, Siemens J, Totsche K-U, Tranvik L, Wagner A, Weitere M and Grossart H-P (2019) Integrating Aquatic and Terrestrial Perspectives to Improve Insights Into Organic Matter Cycling at the Landscape Scale. Front. Earth Sci. 7:127. doi: 10.3389/feart.2019.00127
Received: 02 February 2019; Accepted: 10 May 2019;
Published: 11 June 2019.
Edited by:
Thomas S. Bianchi, University of Florida, United StatesReviewed by:
Bruce A. Hungate, Northern Arizona University, United StatesCopyright © 2019 Kayler, Premke, Gessler, Gessner, Griebler, Hilt, Klemedtsson, Kuzyakov, Reichstein, Siemens, Totsche, Tranvik, Wagner, Weitere and Grossart. This is an open-access article distributed under the terms of the Creative Commons Attribution License (CC BY). The use, distribution or reproduction in other forums is permitted, provided the original author(s) and the copyright owner(s) are credited and that the original publication in this journal is cited, in accordance with accepted academic practice. No use, distribution or reproduction is permitted which does not comply with these terms.
*Correspondence: Zachary E. Kayler, emtheWxlckB1aWRhaG8uZWR1; Katrin Premke, cHJlbWtlQGlnYi1iZXJsaW4uZGU=
†Co-first authors
Disclaimer: All claims expressed in this article are solely those of the authors and do not necessarily represent those of their affiliated organizations, or those of the publisher, the editors and the reviewers. Any product that may be evaluated in this article or claim that may be made by its manufacturer is not guaranteed or endorsed by the publisher.
Research integrity at Frontiers
Learn more about the work of our research integrity team to safeguard the quality of each article we publish.