- 1Geodynamics Unit, Jawaharlal Nehru Centre for Advanced Scientific Research, Bengaluru, India
- 2Department of Crop Physiology, University of Agricultural Sciences, Bengaluru, India
In this paper, we reconstruct the climatic changes starting from the late Pleistocene to the early Holocene as recorded from a fluvio-lacustrine section located within the Kumaun Central Himalaya. The results suggest two major climatic events corresponding with the Last Glacial Maximum (LGM) and Older Dryas (OD). The values of carbon isotopes vary between -23‰ and -14‰, along with a shift in vegetation pattern. The lower part of the section shows prevalence of C3 type vegetation, indicating warm and moist conditions at around 25,000 years BP, possibly coinciding with the intensification of the Indian Summer Monsoon. The onset of cold and arid phase is evident in the gradual shift in vegetation pattern from C3 to C4 plants, which is prominently observed in the middle part of the paleolake profile. Eventually, as the value of δ13C during this time interval confirms, a prolonged phase of cold and arid climate sets in, coinciding with the strengthening of winter westerlies. This cold phase is dated at ∼19,000 years BP and the extended phase of cold interval observed at Dwarahat profile correlates well with previous results elsewhere from the Himalaya. The profile also shows that the LGM phase gradually transforms into a warm and moist climate. This transition registered at 200 cm above the base of the profile, marks the end of glacial period. The short, yet a clear warm spike could be related to the oscillation of Bølling-Allerød interstadial at ∼15,000 years BP. A significant negative excursion marked by an abrupt increase in δ13C values from -20‰ to -14‰ observed toward the top part of the profile, however, is reflective of the reduced monsoon precipitation, corresponding possibly with OD. The topmost part of the profile that registers a depleted trend in δ13C values with dominance of C3 vegetation marks the return of the warm and moist climate.
Introduction
The climate plays a significant role in the Himalaya, as much as tectonics in shaping the landscape and associated processes. The high intensity as well as the deterioration of the climate systems such as the Indian Summer Monsoon (ISM) and the Winter Westerlies has impacted the Himalayan region in the past with varying intensities, triggering major climatic regime modifications in the region. Their changing patterns over long durations of time have not only altered the vegetation pattern but had exerted influence in the formation as well as in the desertion of many lakes in this region, and also in controlling the mass balance of glaciers.
The seasonally reversing moisture laden wind from the Arabian Sea and the Bay of Bengal brings the maximum precipitation all over the mountain arc during the summer (Bookhagen et al., 2005; Anders et al., 2006; Bookhagen and Burbank, 2006). But the precipitation pattern shows spatial variation, which is fundamentally controlled by topography. The higher peaks, because of their distinctive altitude and elevation, receive moderate to heavy snowfall by the action of the mid-latitude subtropical Westerly Jet, referred as Western Disturbances (WDs) or Westerlies, and known as “The Indian Winter Monsoon” as proposed by Dimri and Niyogi (2013) and Dimri et al. (2013). The flooding in the Himalayan Rivers due to heavy rains, rapid snowmelt and outbursts of glacial lakes impact the drainage basins in the high mountain regions as well as the lower reaches. As an alternative mechanism, tectonism also contributes incrementally to altering the drainage behavior and landform architecture. These processes result in barricading the Himalayan Rivers and thus facilitating the formation of lakes and ponds in many parts of the region. The damming of rivers caused either because of climatic processes or tectonism also facilitates formation of depositional niches where long sedimentary records of past climate are likely to be preserved.
One such paleolake section is exposed near Dwarahat Village, situated in the Kumaun Central Himalaya (Figure 1). The lake was formed about 30,000–28,000 years ago, as evidenced by the charcoal date (21,197 ± 329 years BP; Figure 2) obtained from the basal part of the section. It is likely that the lake was resulted from melt water blockage either at the downstream part of the glacier-fed river channel or morphologically depressed area during the rapid snow melt phase. The sediment, which gets trapped in the lake during its active phase largely, owes its origin to the overland flow generated from precipitation that may capture the local, regional and global climatic signature to a considerable extent. These deposits have been used as significant proxies for inferring past climatic fluctuations. Several studies, across various locations and time scales, prove the enormous value of lakes as paleoclimatic archives as they are considered to reflect the precipitation-evaporation balance of their respective catchment (e.g., Cohen, 2003).
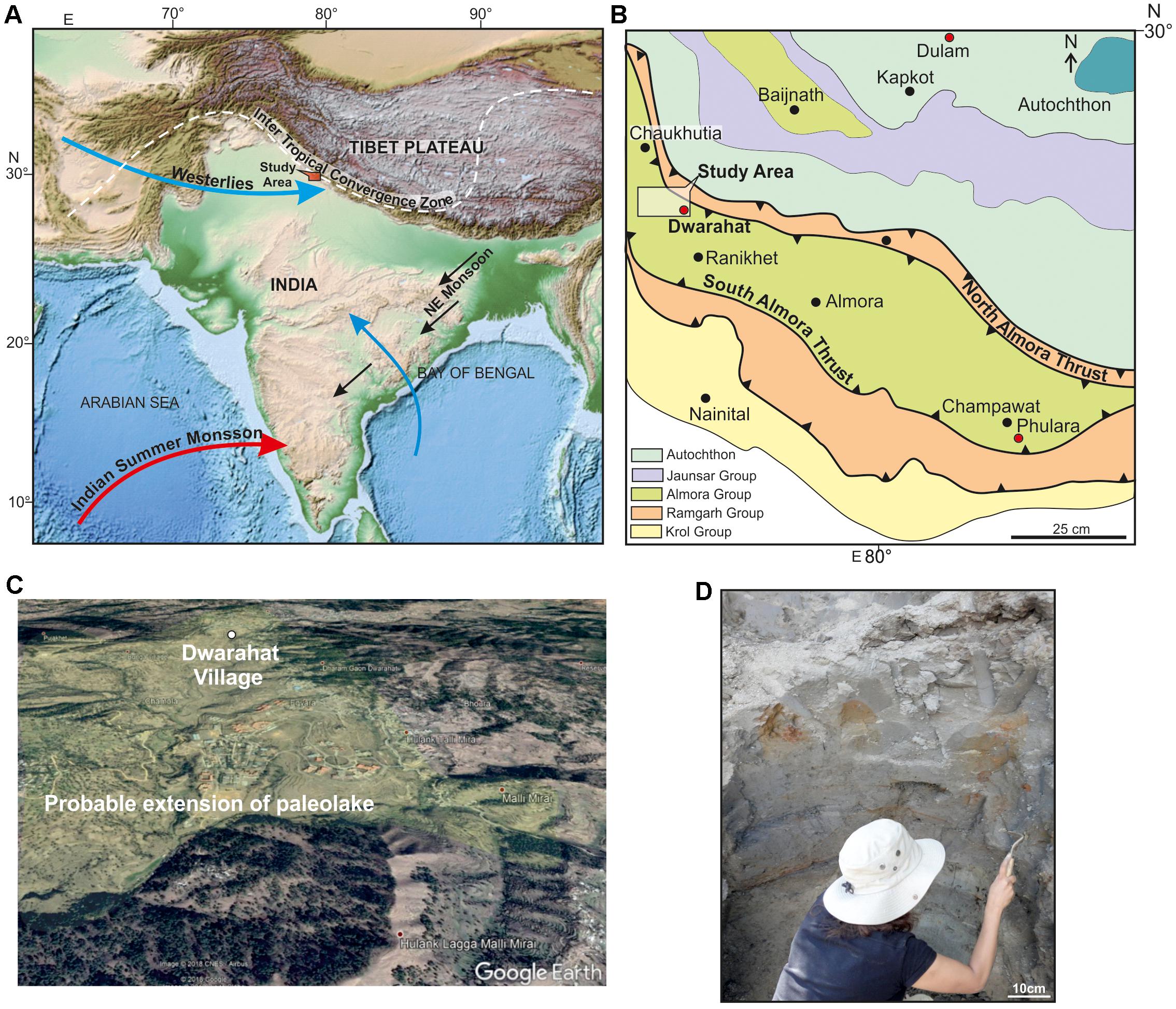
Figure 1. (A) Map shows the location of study area (Dwarahat paleolake) with trend of monsoon systems (ISM, EASM, and WDs), source of the base map: Amante and Eakins (2009), http://www.ngdc.noaa.gov/mgg/image/color_etopo1_ice_low.jpg. (B) Geology and tectonics around the study area (modified after, Joshi, 1999). (C) Google image of the Dwarahat paleolake and (D) a field exposure of paleo-lacustrine deposit.
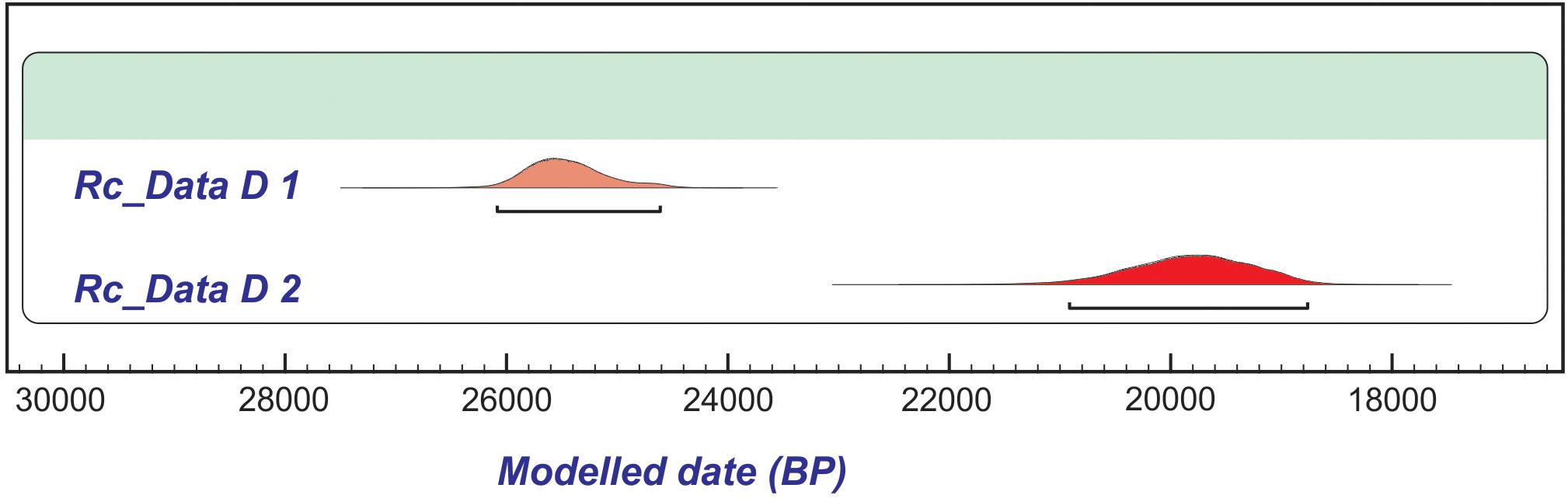
Figure 2. Age model obtained for AMS radiocarbon ages using Oxcal version 4.2.4 (Bronk Ramsey, 2009).
The present study is an attempt toward deciphering the late Quaternary climatic history of the central Kumaun Himalaya using lake deposits as an archive. Although it is known that the climatic patterns in the Himalayan region are mostly controlled by the variations in the ISM and WDs, their long-term variations are not easily resolvable from the geological proxies. Frequently overprinted by the influence of winter westerlies, the interpretation of these proxy records of precipitation often pose challenges in isolating the individual roles of ISM and WDs (Enzel et al., 1999; Prasad and Enzel, 2006; Sinha et al., 2006; Dixit et al., 2014a). However, the earlier studies were able to document the Quaternary climatic variations from the central and western Himalaya (e.g., Chauhan et al., 1997; Kotlia et al., 1997b, 2000, 2010, 2016; Pant et al., 1998, 2005; Sekar, 2000; Chakraborty et al., 2006; Ranhotra et al., 2007, 2017; Trivedi and Chauhan, 2008, 2009; Demske et al., 2009; Juyal et al., 2009; Bali et al., 2013, 2015; Sanwal et al., 2013; Rawat et al., 2015).
Regional Setting
Geology, Geomorphology, Climate, and Vegetation of the Study Area
The study area is located at an altitude of ∼1,100 to 1,200 m (MSL) near Dwarahat Township in the district of Bageshwar of Kumaun Central Himalaya (Figure 1). The lateral extent of the exposed part of fluvio-lacustrine deposit varies from 70 to 80 m, and the maximum thickness of the sediment measures up to 6 m. This section is exposed along a small stream (Shirogarh), and our current work focuses on the exposed part of the deposit adjacent to the Ranikhet-Dwarahat motor road, northwest of Dwarahat Township. The country rocks in the study site consist of gneisses of Almora group and, the area is situated in the zone of ‘North Almora Thrust’ (Figure 1B). The landscape is expressed as a complex mosaic of mountain rises and deeply dissected valleys including a large part of flat land. The elevation from valleys to hills varies from 1,100 to 2,400 m (MSL). As mentioned earlier, such undulated and dissected landscape is prone to a range of micro-climatic regimes, marked by variation in temperature and precipitation within relatively short distances. The river valleys at the lower elevation in general are dominated by sub-tropical climate whereas the elevated and high gradient areas host temperate climatic conditions.
Presently, this region receives moderate precipitation (∼60%) from the ISM during June to September and about 20% from Westerlies during the winter season. The δ13C values of the soil samples collected from the study site vary between -23.5 and -21.5%, which indicates that this region falls under the sub-tropical climate with warm and wet conditions. The mean annual precipitation of this area varies from 104.0 to 129.0 cm. The variation of climatic conditions is also expressed in the type of vegetation. The higher elevations are dominated by Spruce (Picea), Fir (Abies), Birch (Betula), and Juniper (Juniperus) while the lower elevations are covered with the forests of Cypress, pine (Pinus), Oak (quercus) and Rhododendron.
Lithology of Paleolake Sequence
The base of the Dwarahat lacustrine profile mainly comprises by coarse-grained (coarse sand to boulder size particles) and non-cohesive sediments. These deposits could have been generated by debris flows that are most often triggered by extreme precipitation following a period of depleted precipitation, or by rapid snow melt. The geomorphological and sedimentological evidence also implies that the high pore-water pressures might have caused the soil and weathered rock to rapidly lose strength and flow downslope toward relatively depressed/flat land where it gets accumulated within ponds or lakes. The morphology around the study area indicates that the climate had played a foremost role in the development of this basin and the formation of the lake. Thus, the paleolake at Dwarahat (29°45’ N: 79°25’ E) in the Kumaun Himalaya, was most probably resulted from damming of meltwater.
The lake profile consists of 4-m-thick mud, silt and sand; the base of the lacustrine profile is dominated by coarse-grained sediment (Figure 3A). The entire profile can be divided into six different units, the basal unit (unit 1) is homogeneously made of black to gray carbonaceous mud demonstrating low energy deposition; whereas the second unit (unit 2) from the bottom mostly consists of gritty sand and silt that reveals comparatively moderate energy conditions during the deposition. The overlying unit 3 comprises mainly silt representing moderate energy condition, which is capped by a band of black to brown mud (unit 4) indicating low energy conditions during their deposition. The fifth unit (unit 5) is consists of cross-bedded channel sand and is topped by the thinly laminated alternative layers of upward grading silt and sand (unit 6). This top stratigraphic unit is overlain by a well sorted cross-bedded upward coarsening fluvial succession, demarcating the transition from a lacustrine to fluvial phase.
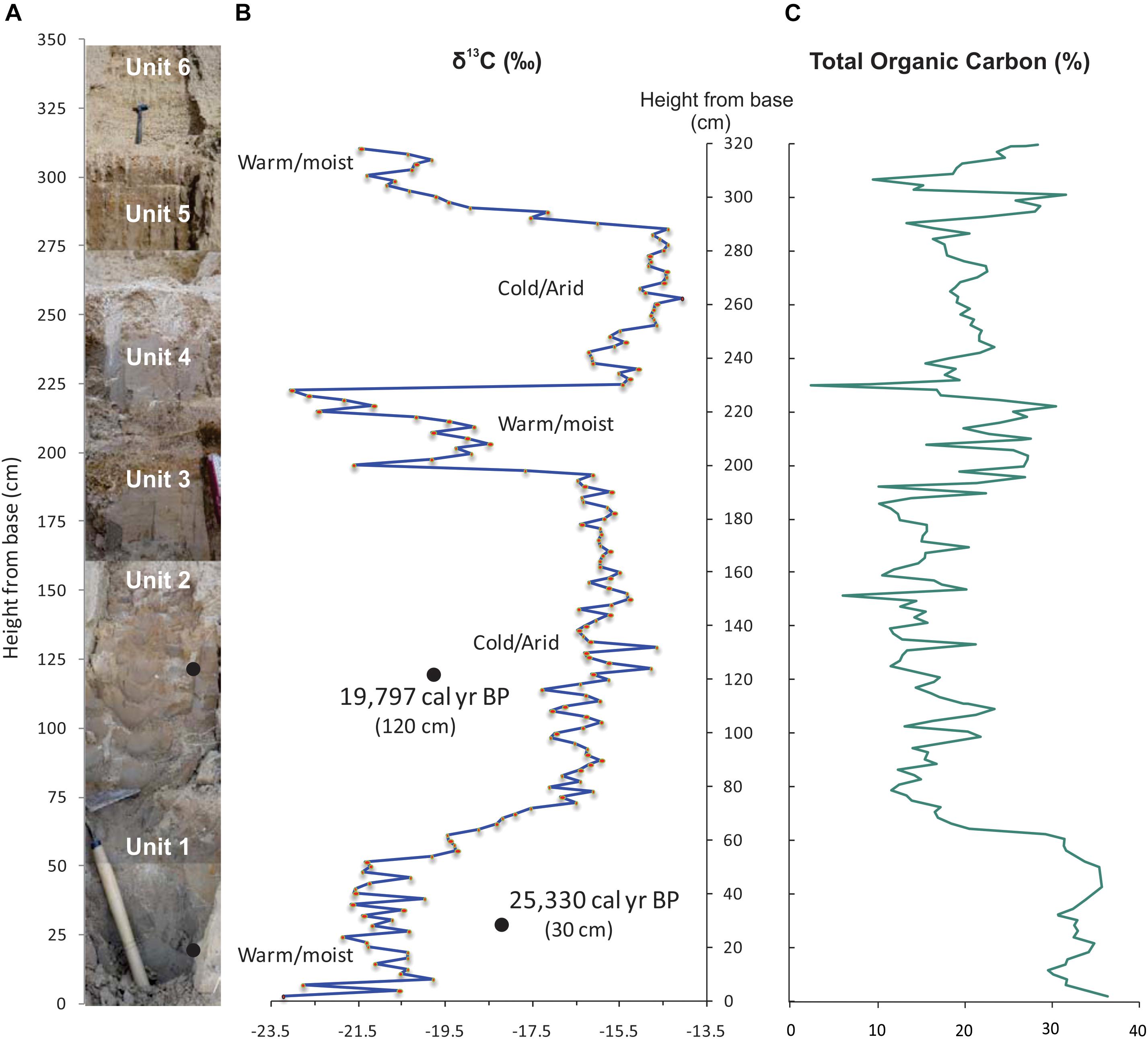
Figure 3. (A) Lithostratigraphy of Dwarahat paleolake, (B) carbon isotope variation (δ13C‰), and (C) total organic carbon (TOC%).
Materials and Methods
Chronology
Radiocarbon dates (Acceleration Mass Spectrometry; AMS) were obtained from the bulk organic sediment samples as no charcoal material was available from the section. The age data was calibrated using CALIB 6.0 program (Stuiver and Reimer, 1993), in calibrated years before present (cal years BP). The oldest radiocarbon date: 21,197 ± 329 years BP is obtained from the lowermost unit (at ∼30 cm from the base) of the profile is calibrated as 25,330 cal years BP (Table 1 and Figure 2). A younger age obtained at ∼120 cm from the base corresponds to 16,380 ± 453 years BP and is calibrated as 19,797 cal years BP (Table 1). The interpolated ages for the section discussed in the text are estimated from the above cited calibrated radiocarbon dates.
We have further refined chronological constraints by applying OxCal V 4.2.4 (Bronk Ramsey, 2009), a Bayesian statistical program that incorporates sequential stratigraphic relationships that can filter the probability distributions of each sample age. This age model developed through an iterative process narrows the probability distribution functions (PDFs) for the ages of each of the calibrated samples and identifies outliers (Figure 2). With only two dates, our chronological constraints obtained from the Dwarahat section are too limited. We believe that there is scope for refining the chronology and better age resolution in future studies, which will help in finer interpretation of climatic fluctuations.
Organic Carbon
In a lake system, the primary production of organic matter depends on the plants within the lake and around it, but its alteration varies geographically and temporally. The maximum organic substance in the lake can be divided into two categories on the basis of their biochemical compositions: (a) the non-vascular plants and phytoplanktons that contain limited or no carbon-rich cellulose and lignin, and (b) terrestrial plants like grasses, herbaceous plants and macrophytes (Last and Smol, 2001). These herbs and/or macrophytes that are photoautotrophic utilize CO2 for photosynthesis with different efficiencies. Water availability and other environmental factors like temperature can also alter carbon assimilation. Hence, assessing the carbon isotope signatures could be a powerful tool in estimating the photosynthetic efficiency of the planktons of a lake. For this study the carbon isotope ratios were measured using Isotope Ratio Mass Spectrometer (IRMS) working on a continuous flow basis.
Stable Carbon Isotope (δ13C)
Stable carbon isotopic analysis of organic matter is a proven tool for deciphering the past climatic history. The results are expressed here as δ13C with respect to VPDB standard using the standard δ (‰) notation. In general, based on the carbon isotope abundance (δ13C values), the photosynthetic plants can be separated into two categories: C3 and C4 plants (Smith and Epstein, 1971). The δ13C values of C3 plants range between -35‰ and -22‰, averaging around -27‰, while those of the C4 plants range between -20‰ and -9‰ with an average of -13‰ (Osmond and Ziegler, 1975; Cerling, 1984). The lake sediments contain inorganic as well as organic carbon along with organic matter. Isotopic signature of organic carbon distinguishes between C3 and C4 photosynthetic mechanisms. Hence, careful separation of organic matter from the bulk sediment is essential. We powdered 2 g of samples and treated with dilute HCL (1:4) for 10 to 15 min for the removal of inorganic carbon and carbonate followed by centrifuging at ∼3,000 rpm with Milli-Q water and same process was repeated until the inorganic carbon and carbonate were completely removed following the method by Sanwal (2004, Unpublished) and Kotlia et al. (2010). Thereafter residue power was dried at 60°C and the resultant samples were stored in sealed containers.
Stable carbon isotope composition (δ13C) was measured using an IRMS (DeltaV Adv., Thermo Fischer Scientific, Bremen, Germany) interfaced with an elemental analyzer (NA1112, Carlo-Erba, Italy) with a continuous flow device (Conflo-III, Thermo Fischer Scientific) installed in the Department of Crop Physiology, University of Agricultural Sciences (UAS), Bengaluru. About 1.5–3 mg of samples was packed in silver capsules and combusted at ∼1,060°C in an oxygenated environment of the Elemental Analyzer. Resultant CO2 was flushed along a helium carrier flow after scrubbing excess oxygen and moisture. The helium flow carried the gases through a “reduction” furnace filled with reduced copper heated at 680oC to reduce the nitrogen oxides to nitrogen (N2). The CO2 gas was effectively separated from nitrogen by passing the gases through a Gas Chromatography column in the elemental analyzer. Mass to charge ratio (m/z) for mass 44, and 45 were determined by the Continuous Flow IRMS. The internal precision of the system was determined by sequencing injection of reference CO2 gas and was found to be better than 0.06‰. External precision to include variability caused due to sample combustion was determined by using two standards. Potato starch (Sigma) as a C3 standard and ANU Sucrose, as a C4 standard. The analytical uncertainty was found to be less than 0.15‰. The results are expressed as δ13C with respect to VPDB standard using the δ (‰) notation as:
In the aforementioned equation, Rsample and Rstandard express the ratio of heavy to light isotopes (13C/12C) in sample as well in the standard. We analyzed 163 samples at the interval of every 2 cm out of 320 cm long profile for δ13C values (Figure 3).
Total Organic Carbon (TOC)
Pure potato starch and elemental analyzer standards such as cyclohexanone were used to develop a calibration curve to determine the total organic carbon (TOC) content. Area under the chromatogram for all masses of CO2 was used to compute the TOC content using the calibration curve developed using elemental analyzer standards. The results of TOC are demonstrated as the percentage of the dry weight of the samples
Results and Interpretations
Climatic Reconstruction
We have used the stable isotopic data (δ13C) obtained from a fluvio-lacustrine sedimentary profile along with the available chronological constraints for the reconstruction of the Late Quaternary climatic changes. The AMS date obtained from the base of the profile, (∼25,330 cal years BP), suggests that the lake might have been in existence between 30,000 and 28,000 years BP (Table 1 and Figure 2). We have identified five prominent climatic phases by mainly tracking the carbon isotope trends. Correspondingly, the lower, middle and topmost parts of the profile also display a large variation in the distribution of C3 and C4 type of vegetation (Figure 3). As mentioned earlier, the benchmark is provided by the present-day δ13C values that vary between -23.5 and -21.5‰ corresponding with the sub-tropical climate with warm and wet conditions.
The lowermost part of the profile (0–60 cm) that consists of black carbonaceous mud and marked by the abundance of C3 kind of vegetation, is characterized by depleting trend in δ13C values (up to -23‰). These features suggest an interval of warm and moist climate between 30,000 and 25,000 years BP marked by a long spell of intensified monsoon. The isotope curve indicates a gradual rise in δ13C values (up to -14‰) at the height of 55–60 cm from the base and this trend suggests a prolonged phase of cold and arid conditions with dominance of C4 kind of vegetation with the peak aridity at the height of 140 cm from the base. This extended cold and arid interval must have started around 19,000 years BP.
The carbon isotope results reveal that the LGM interval of cold and arid climate gradually emerges into a phase of climatic amelioration marked by warm and moist climate, and this transition makes its appearance on the profile at 200 cm above the base. The gradually increasing dominance of C3 vegetation with a prominent depletion in δ13C values (from ∼-17 to 22.5‰) indicates escalating monsoon during this period, i.e., after ∼15,000 years BP. This climatic transformation is marked by the intensification of the Indian Summer Monsoon. This short, yet prominent warm spike is probably related to the oscillation of Bølling-Allerød interstadial around 15,000 years BP. Although we lack dates from this part of the profile, the isotope values obtained by us mimic the vertical trends reported from the paleo-lacustrine sections elsewhere in the central Himalaya that are chronologically much better constrained (see Kotlia et al., 2010).
The aforesaid phase of climatic amelioration is followed by a conspicuous negative excursion as evidenced by the abrupt increase in δ13C values from -20‰ up to -14‰. The dominance of C4 type vegetation reflects the weakening of monsoon precipitation. This phase of declined monsoon may overlap the Older Dryas (OD), which is largely been documented around ∼14,000–13,000 years BP in the Asian continent. The carbon isotope data toward the top of the profile shows domination of C3 vegetation as reflected in the gradual depletion of δ13C values. The values of δ13C varying from -15 to -21‰ are indicative of warm and moist climate with increasing summer precipitation.
The data obtained from TOC range between ∼2.0 and 38%. The lowermost part of the profile dominated by carbonaceous mud exhibits high values of TOC that varies between 30 and 36.5% and are indicative of high precipitation. A gradual decreasing trend of TOC (5–25%) is obtained from the middle part of the profile (∼22,000–18,000 years BP) suggesting arid conditions. An increasing trend of TOC varying from 15 to 30% is registered around 15,000 years BP and is an indicator of enhanced precipitation. A decreasing trend of TOC is also noticed toward the top of the profile with some distinct fluctuations (Figure 4B).
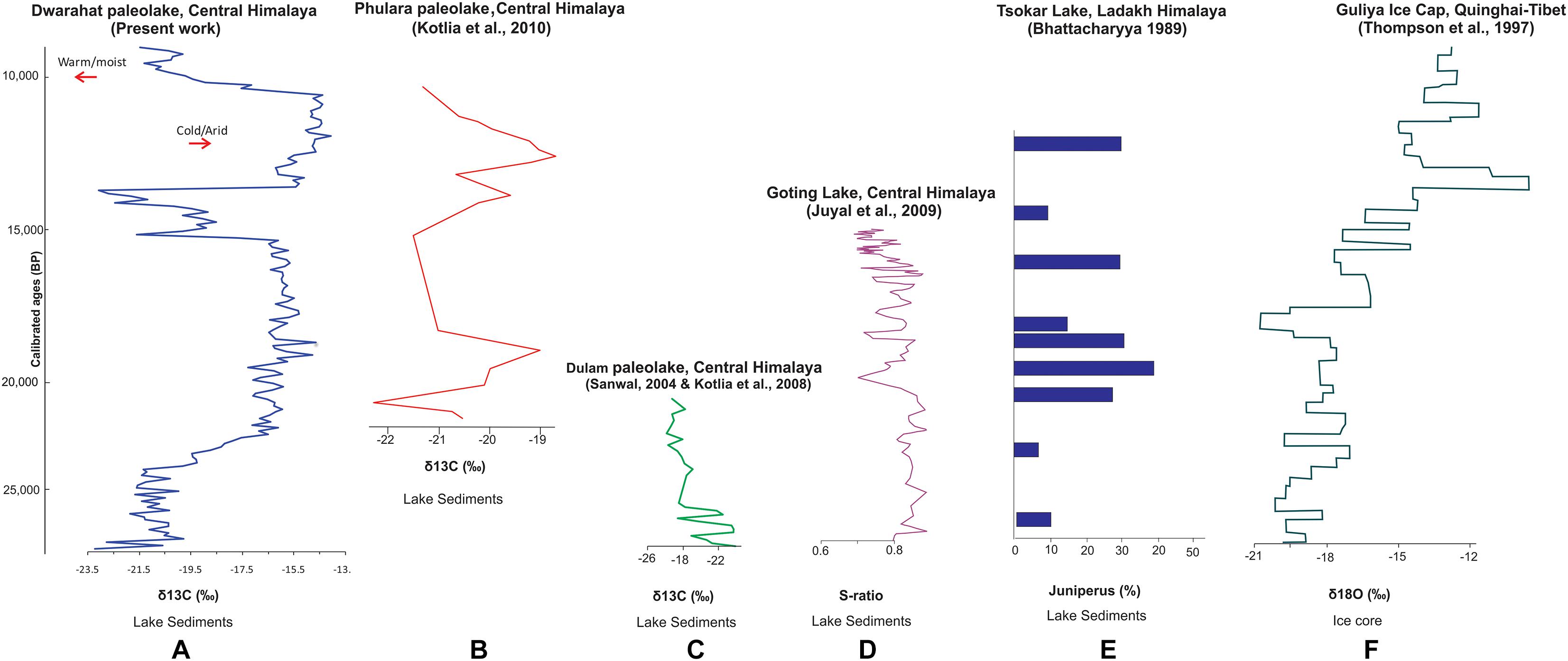
Figure 4. A few proxy records of paleoclimate covering the last glacial-interglacial transition, reconstructed from the Central Himalaya (A–D) Ladakh Himalaya (E) and Guliya Ice Cap, Qinghai-Tibet (F).
The isotope signature is an indicator of the efficiency of carbon metabolism and it tracks the record of climatic conditions that influence the growth of vegetation, whereas TOC trends reflect absorption or assimilation of carbon. But there are cases when TOC may not correspond well with the isotope ratios, as may be applicable to our present study also. Therefore, we have exclusively used the variation in δ13C for climatic reconstruction presented here.
Discussion
Studies of climate variability during the Quaternary in the Himalayan region are of great interest as they help in furthering our understanding of the climatic evolution of a mountainous terrain that is also currently a severely impacted ecosystem. These studies could open a way to understand more about how the climate system diversify under the natural conditions in a unique mountainous environment during an interval that is known for abrupt as well as gradual climatic transitions. In this context, the ancient lakes sedimentary sections are considered excellent storehouses that embed not only local but regional and global climatic signals, as well.
The Dwarahat paleolake was possibly formed because of damming of meltwater about 30,000 years ago. Presently, the area falls under moderately warm and moist conditions, receiving about 70% of the ISM during summers and experiences cool winters with 20% of rains with low to moderate snow. The results obtained using organic carbon isotopes (δ13C) reveal that the initial stage of deposition (∼30,000–25,000 years BP) was dominated by organic carbon-rich sediments and the vegetation represented by C3 plants. These observations are suggestive of warm and moist climate with strong monsoon precipitation. The trend in general agrees with the results obtained elsewhere in the Himalaya (Figure 4).
The earlier studies from paleolake and peat deposits from the central Himalaya indicate that the climatic amelioration with the humid phase began around 30,000 years, before present (Pant et al., 1998; Kotlia et al., 2000, 2008). The humid conditions have also been documented at around 30,000 years BP from some other parts like Tso Kar Lake located in the Ladakh Himalaya. The palynological signals indicate abundance of biogenic deposits and increasing trend in Juniperus (Bhattacharyya, 1989) (Figure 4), along with the chemical signatures marked by lower concentration of Na, K, and Mg (Sekar, 2000). The ice core analyses reported from Tibet (Dunde Ice Cap) suggest relatively warm conditions around 30,000 years BP (Thompson et al., 1989, 1990). The studies on Chinese loess and lake deposits from Western China also suggest similar patterns (Kukla and An, 1989; Jelinowska et al., 1995).
A prominent phase of warm/humid climate from 28,900 to 27,400 years BP and 26,800 to 25,300 years BP has been identified from a paleolake section at Dulam in the Central Himalaya (Sanwal, 2004, Unpublished; Kotlia et al., 2008) (Figures 1, 4). The signatures include declining levels of Ca/Mg and Fe/Mn ratios and clay minerals like chlorite and muscovite, corresponding with domination of C3 type vegetation (Sanwal, 2004, Unpublished; Kotlia et al., 2010). The magnetic susceptibility studies of peat sediments from Garhwal Himalaya also indicate warm moist climate during this interval (Pant et al., 1998). Corollary evidence is obtained from Qaidam basin in the northern margin of the Tibetan Plateau where the lake levels continued to be high from 40,000 to 25,000 years BP, which is linked to increased precipitation (Chen and Bowler, 1986). Comparable results of warm and moist climate at around 24,000 years BP is documented from the southeastern part of China, as well (Zheng and Li, 2000).
The dominance of C4 type of vegetation, with a gradual rise in δ13C values (up to -14‰), marking the onset of prolonged phase of cold and arid conditions can be traced at the Dwarahat section around 140 cm from the base. The peak of this extended period of cold and aridity about 19,000 years BP corresponds with the Last Glacial Maximum (LGM). Comparable signals are available from the terrestrial and marine records from India and Tibet. It is worth mentioning here that a study from Dulam paleolake located in the Central Himalaya (Figures 1, 4), not far from Dwarahat also indicates the dominance of C3 vegetation (Sanwal, 2004, Unpublished; Kotlia et al., 2010). Thus, the central Himalaya displays an abrupt rise in δ13C and a drop in marshy taxa, mesic herbs, the dominance of Pinus, and a depleted trend in warmer minerals and reduced magnetic susceptibility (Kotlia et al., 1997a; Basavaiah et al., 2004; Juyal et al., 2009; Kotlia et al., 2010) (Figure 4). In the same interval (23,000 years BP), a spell of accelerated erosion has also been identified in the Garhwal Himalaya (Pant et al., 1998). An arid cold period also existed in the Nepal Himalaya between 25,000 and 18,000 years BP (Richards et al., 2001). The dominance of steppe vegetation and a declining trend in Juniperus have been observed at 21,000 years BP in the Ladakh region (Bhattacharyya, 1989) (Figure 4), reiterating the validity of an arid phase during this period. These signatures of onset of arid conditions starting from 22,000 years BP, therefore, appear to be regional in nature. These results also should be seen in the context of an expanded distribution of Himalayan glaciers that is registered at ∼18,000 years BP (Benn and Owen, 1998; Owen et al., 2002). The records of δ18O and δ13C from Guliya ice core from Qinghai-Tibetan plateau, suggest the timing of the LGM in the tropics and subtropics to be around 18,000 years BP (Thompson et al., 1997) (Figure 4), and the peak aridity at ∼18,000 years BP, as reported from the northeastern Tibetan Plateau (Yan et al., 1999).
The records between 20,000 and 13,000 years BP from the continental areas of India, as exemplified by the Thar Desert, show hyper saline conditions that overlap with a decline in the summer monsoon with increased winter precipitation (Wasson et al., 1983; Singh et al., 1990). These records of prolonged arid phase in the western India between 20,000 and 15,000 years BP are also well supported by the findings from southern India (Sukumar et al., 1993; Bera et al., 1996; Rajagopalan et al., 1997; Sukhija et al., 1998), as well as from the central India (Singh et al., 1974, 1990; Andrews et al., 1998; Srivastava et al., 2003).
The marine proxy data for the same period (∼20,000 to 15,000 years BP) from the Arabian Sea and the Bay of Bengal suggest lowered of sea levels (Duplessy, 1982; Van Campo et al., 1982; Van Campo, 1986; Sarkar et al., 1990; Chauhan and Suneethi, 2001). Further, this interval (18,000 years BP) is also defined by weak upwelling in the Arabian Sea (Van Campo et al., 1982; Prell and Van Campo, 1986; Prell and Kutzbach, 1987; Sirocko et al., 1991, 1993).
The Dwarahat section reveals that the prolonged phase of cold climate ends in short spell of climatic amelioration marked by warm and moist conditions (observed at 200 cm above the base). This transition marks the end of the glacial period. Although we lack chronological controls on this phase our interpretations are based on the cues provided by the increasing appearance of C3 vegetation. Showing a prominent depleting trend in δ13C this phase indicates an escalating monsoon during this period. Furthermore, these patterns identified at Dwarahat also agree with the signals identified from other sites in the Himalaya at the corresponding stratigraphic levels. For instance, the speleothem records of δ18O from the Central Himalaya reveal intensified monsoon during ∼15,200 to 11,700 years BP and this strong monsoon phase has been correlated with Bølling-Allerød interstadial (Sinha et al., 2005). A similar phase of climatic amelioration is reported from the distant Kashmir (Singh and Agrawal, 1976) and the Ladakh Himalaya at ∼15,000 years BP (Bhattacharyya, 1989). Correspondingly, a post-glacial temperature rise is recorded from the Tibetan Plateau around 15,000 years BP (Yan et al., 1999). This observation is further supported by the climatic amelioration identified from Northwestern China around this time (Feng et al., 2007).
A sharp spike of negative excursion at Dwarahat (230 cm above the base) is marked by an abrupt increase in δ13C values with dominance of C4 vegetation. This may be interpreted to be the result of weak summer monsoon precipitation; this phase of declined monsoon spans the OD cooling event, a global event that occurred between 14,000 and 13,000 years BP. This cooling event is evidenced by an abrupt increase in δ13C and magnetic susceptibility, as reported in an earlier study (Kotlia et al., 2010). Similar records of declined monsoon precipitation are also documented from the Ganga Plains (Sharma et al., 2004) and the Bay of Bengal (Kudrass et al., 2001; Chauhan, 2003). The declining monsoon is reported to be evident in the continent as well the marine deposits in mid-latitude regions of China (Zhou et al., 1991; Li, 1993).
The Dwarahat profile toward the top (∼300 cm above the base) shows depleting δ13C values, concomitant with the domination of C3 vegetation. Marking the transition to the Holocene, this trend reflecting the warm and moist conditions might have been triggered by the intensification of the southwest monsoon.
Conclusion
Based on the carbon isotopic (δ13C) variations of the sedimentary profile exposed at Dwarahat in the Kumaun central Himalaya we attempt to construct the climatic changes in the region during the interval ranging from 28,000 to 12,000 years BP that forms the later part of the Quaternary Period. Our results demonstrate five distinct alternating phases of warm and cold conditions. The profile begins with the warm and moist climate with intensified monsoon with high vegetation growth of C3 type. This phase gradually gives way to prolonged cold spell with abundance of C4 plants. This interval of aridity corresponding with the LGM gradually transits into a short spell of climatic amelioration with warm and moist climate. We suggest that this initial onset of climate change is marked by the intensification of summer monsoon around 15,000 years BP – a timing that agrees well with the results elsewhere from the Himalaya. This phase is followed by depleted precipitation curve, which is evident by the dominance of C4 vegetation, a marker for the OD (14,000–13,000 years BP). A prominent warm spike at the top end of the profile probably corresponds with the Allerød oscillation, around ∼12,000 years BP. The factors including the shifting of the Intratropical Convergence Zone (ITCZ) and southwest airflow, along with the changing patterns of summer monsoon and westerlies play important determining roles in the changing dynamics of the climatic conditions in the Himalayan region. A weak southwest airflow, southward shift in the ITCZ, depletion in the summer monsoon and stronger westerlies characterize the alternating cycles of cold and arid periods, whereas strengthening of the southwestern monsoon during the summers facilitates the onset of warm and moist climate.
Author Contributions
The manuscript was planned and written by JS with inputs from CPR and MS. The fieldwork and sample collection were done by JS and CPR. The isotopic measurements were conducted by MS and JS.
Funding
The study was funded by the Department of Science and Technology (Government of India) under the Science and Engineering Research Council (SERC) Fast Track Scheme (No. SR/FTP/ES-74/2014) awarded to JS.
Conflict of Interest Statement
The authors declare that the research was conducted in the absence of any commercial or financial relationships that could be construed as a potential conflict of interest.
Acknowledgments
We are thankful to Abhishek and Arpita Kandpal for providing botanical and recent sediment samples of the present vegetation. JS acknowledges Vineet Kotiyal for his extended help during the field work. We thank the faculty and students of the Government Polytechnic at Dwarahat for extending their co-operation at the study site. We thank the reviewers for their thoughtful comments on the manuscript. The dating of samples was conducted in Accelerator Mass Spectrometry (AMS) Laboratory, University of Arizona, United States.
References
Amante, C., and Eakins, B. W. (2009). ETOPO 1 Arc-Minute Global Relief Model: Procedures, Data Sources and Analysis. Silver Spring, MD: NOAA.
Anders, A. M., Roe, G. H., Hallet, B., Montgomery, D. R., Finnegan, N. J., and Putkonen, J. (2006). “Spatial patterns of precipitation and topography in the Himalaya,” in Tectonics, Climate, and Landscape Evolution, Geological Society of America, Special Paper, Vol. 398, ed. D. Willett (Boulder, CO: Geological Society of America), 39–53.
Andrews, J. E., Singhvi, A. K., Kailath, A. J., Kuhn, R., Dennis, P. F., Tandon, S. K., et al. (1998). Do calcrete stable isotopes record the late Pleistocene monsoonal climate variation in the Thar Desert of India? Quat. Res. 50, 240–251. doi: 10.1006/qres.1998.2002
Bali, R., Agarwal, K. K., Nawaz Ali, S., Rastogi, S. K., Krishna, K., and Srivastava, P. (2013). Chronology of late quaternary glaciation in the pindar valley, alaknanda basin, central himalaya (India). J. Asian Earth Sci. 66, 224–233. doi: 10.1016/j.jseaes.2013.01.011
Bali, R., Nawaz, A. S., Bera, S. K., Patil, S. K., Agarwal, K. K., and Nautiyal, C. M. (2015). “Impact of anthropocene vis-a-vis holocene climatic changes on central indian himalayan glaciers,” in Engineering Geology for Society and Territory, Vol. 1, ed. G. Lollino (Switzerland: Springer International Publishing), 467–471.
Basavaiah, N., Juyal, N., Pant, R. K., Yadava, M. G., Singhvi, A. K., and Appel, A. (2004). Late Quaternary climatic changes reconstructed from mineral magnetic studies from pro-glacial lake deposits of Higher Central Himalaya. J. Indian Geophys. Union 8, 27–37.
Benn, D. I., and Owen, L. A. (1998). The role of the Indian summer monsoon and the mid latitude westerlies in Himalayan glaciation: review and speculative discussion. J. Geol. Soc. 155, 353–363. doi: 10.1144/gsjgs.155.2.0353
Bera, S. K., Gupta, H. P., and Farooqui, A. (1996). Berijam lake: 20,000 years sequence of palaeofloristics and palaeoenvironment in Palni Hills, south India. Geophytology 26, 99–104.
Bhattacharyya, A. (1989). Vegetation and climate during the last 30,000 years in Ladakh. Palaeogeogr. Palaeoclimatol. Palaeoecol. 30, 25–38. doi: 10.1016/0031-0182(89)90042-4
Bookhagen, B., and Burbank, D. W. (2006). Topography, relief, and TRMM-derived rainfall variations along the Himalaya. Geophys. Res. Lett. 33,
Bookhagen, B., Thiede, R. C., and Strecker, M. R. (2005). Abnormal monsoon years and their control on erosion and sediment flux in high, arid northwest Himalaya. Earth Planet. Sci. Lett. 231, 131–146. doi: 10.1016/j.epsl.2004.11.014
Bronk Ramsey, C. (2009). Bayesian analysis of radiocarbon dates. Radiocarbon 51, 337–360. doi: 10.1017/S0033822200033865
Cerling, T. E. (1984). The stable isotopic composition of modern soil carbonate and its relationship to climate. Earth Planet. Sci. Lett. 71, 229–240. doi: 10.1016/0012-821X(84)90089-X
Chakraborty, S., Bhattacharya, S. K., Ranhotra, P. S., Bhattacharyya, A., and Bhushan, R. (2006). Palaeoclimatic scenario during Holocene around Sangla Valley, Kinnaur northwest Himalaya based on multi proxy records. Curr. Sci. 91, 777–782.
Chauhan, M. S., Sharma, C., and Rajagopalan, G. (1997). Vegetation and climate during Late-Holocene in Garhwal Himalaya. Palaeobotanist 46, 211–216.
Chauhan, O. S. (2003). Past 20,000-year history of Himalaya aridity: evidence fromoxygen isotope records in the Bay of Bengal. Curr. Sci. 84, 90–93.
Chauhan, O. S., and Suneethi, J. (2001). 18 ka BP records of climatic changes, Bay of Bengal: isotopic and sedimentological evidences. Curr. Sci. 8, 1231–1234.
Chen, K. Z., and Bowler, J. M. (1986). Late Pleistocene evolution of salt lakes in the Qaidam Basin, Qinghai Province, China. Palaeogeogr. Palaeoclimatol. Palaeoecol. 54, 87–104. doi: 10.1016/0031-0182(86)90119-7
Cohen, A. S. (2003). Paleolimnology: The History and Evolution of Lake Systems. New York, NY: Oxford University Press.
Demske, D., Tarasov, P. E., Wünnemann, B., and Riedel, F. (2009). Late glacial and Holocene vegetation, Indian monsoon and westerly circulation dynamics in the Trans-Himalaya recorded in the pollen profile from high-altitude TsoKar Lake, Ladakh, NW India. Palaeogeogr. Palaeoclimatol. Palaeoecol. 279, 172–185. doi: 10.1016/j.palaeo.2009.05.008
Dimri, A. P., and Niyogi, D. (2013). Regional climate model application at subgrid scale on Indian winter monsoon over the western Himalayas. Inte. J. Climatol. 33, 2185–2205. doi: 10.1002/joc.3584
Dimri, A. P., Yasunari, T., Wiltshire, A., Kumar, P., Mathison, C., Ridley, J., et al. (2013). Application of regional climate models to the Indian winter monsoon over the western Himalayas. Sci. Total Environ. 468-469, S36–S47. doi: 10.1016/j.scitotenv.2013.01.040
Dixit, Y., Hodell, D. A., and Petrie, C. A. (2014a). Abrupt weakening of the summer monsoon in northwest India ∼ 4100 yr ago. Geology 42, 339–342. doi: 10.1130/G35236.1
Duplessy, J. C. (1982). Glacial to interglacial contrast in the northern Indian Ocean. Nature 295, 494–498. doi: 10.1038/295494a0
Enzel, Y., Ely, L. L., Mishra, S., Ramesh, R., Amit, R., Lazar, B., et al. (1999). High-resolution holocene environmental changes in the Thar Desert, northwestern India. Science 284, 125–128. doi: 10.1126/science.284.5411.125
Feng, D. Z., Zhai, X. W., Ma, Y. Z., Huang, C. Q., Wang, W. G., Zhang, H. C., et al. (2007). Eolian environmental change in the northern Mongolian plateau during the past ∼35,000 yr. Palaeogeogr. Palaeoclimatol. Palaeoecol. 245, 505–517. doi: 10.1016/j.palaeo.2006.09.009
Jelinowska, A., Tucholka, P., Gasse, F., and Fontes, J. C. (1995). Mineral magnetic record of environment in the late pleistocene and holocene sediments, Lake Manas, Xinjiang, China. Geophys. Res. Lett. 22, 953–956. doi: 10.1029/95GL00708
Joshi, M. (1999). Evolution of the basal shear zone of the almora nappe, kumaun himalaya. Gondwana Res. Group Memoir 6, 69–80.
Juyal, N., Pant, R. K., Basavaiah, N., Bhushan, R., Jain, M., Saini, N. K., et al. (2009). Reconstruction of late glacial to early holocene monsoon variability from relict lake sediments of the Higher Central Himalaya, Uttrakhand, India. Journal of Asian Earth Science 34, 437–449. doi: 10.1016/j.jseaes.2008.07.007
Kotlia, B. S., Bhalla, M. S., Sharma, C., Rajagopalan, G., Ramesh, R., Chauhan, M. S., et al. (1997a). Palaeoclimatic conditions in the upper Pleistocene and Holocene Bhimtal-Naukuchiatal lake basin in south-central Kumaun, North India. Palaeogeogr. Palaeoclimatol. Palaeoecol. 130, 307–322. doi: 10.1016/S0031-0182(96)00126-5
Kotlia, B. S., Shukla, U. K., Bhalla, M. S., Mathur, P. D., and Pant, C. C. (1997b). Quaternary fluvio-lacustrine deposits of the Lamayuru basin, Ladakh Himalaya: preliminary multidisciplinary investigations. Geol. Mag. 134, 807–815. doi: 10.1017/S0016756897007826
Kotlia, B. S., Sanwal, J., and Bhattacharya, S. K. (2008). Climatic record between ca. 31 and 22 ka BP in east-central Uttarakhand Himalaya, India. Himalayan Geol. 29, 25–33.
Kotlia, B. S., Sanwal, J., Phartiyal, B., Joshi, L. M., Trivedi, A., and Sharma, C. (2010). Late Quaternary climatic changes in the eastern Kumaun Himalaya, India, as deduced from multi-proxy studies. Quat. Int. 213, 44–55. doi: 10.1016/j.quaint.2009.09.002
Kotlia, B. S., Sharma, C., Bhalla, M. S., Rajagopalan, G., Subrahmanyam, K., Bhattacharyya, A., et al. (2000). Palaeoclimatic conditions in the late pleistocene Wadda Lake, eastern Kumaun Himalaya, India. Palaeogeogr. Palaeoclimatol. Palaeoecol. 162, 105–118. doi: 10.1016/S0031-0182(00)00107-3
Kotlia, B. S., Singh, A. K., Sanwal, J., Raza, W., Ahmad, S. M., Joshi, L. M., et al. (2016). Stalagmite inferred high resolution climatic changes through pleistocene-holocene transition in Northwest Indian Himalaya. J. Earth Sci. Clim. Change 7:338.
Kudrass, H. R., Hofmann, A., Doose, H., Emeis, K., and Erlenkeuser, H. (2001). Modulation and amplification of climatic change in the northern Hemisphere by the Indian summer monsoon during the past 80 k.y. Geology 29, 63–66. doi: 10.1130/0091-7613(2001)029<0063:MAAOCC>2.0.CO;2
Kukla, G., and An, Z. S. (1989). Loess stratigraphy in central China. Paleogeogr. Paleoclimatol. aleoecol. 72, 203–225. doi: 10.1016/0031-0182(89)90143-0
Last, W. M., and Smol, J. P. (eds) (2001). Tracking Environmental Change Using Lake Sediments. Volume 2: Physical and Geochemical Methods. Dordrecht: Kluwer Academic Publishers. doi: 10.1007/0-306-47669-X
Osmond, C. B., and Ziegler, H. (1975). Schwerepflanzen and leichtepfanzen: stable isotope in photosynthesis toffwechelun in der biochemischeno kaklogie. Narturwissenschaft Rundschau 28, 1–323.
Owen, L. A., Finkel, R. C., and Caffee, M. W. (2002). A note on the extent of glaciation throughout the Himalaya during the global Last Glacial Maximum. Quat. Sci. Rev. 21, 147–157. doi: 10.1016/S0277-3791(01)00104-4
Pant, R. K., Basavaiah, N., Juyal, N., Saini, N. K., Yadava, M. G., Appel, E., et al. (2005). A 20-ka climate record from Central Himalayan loess deposits. J. Quat. Sci. 20, 485–492. doi: 10.1002/jqs.938
Pant, R. K., Juyal, N., Rautela, P., Yadava, M. G., and Sangode, S. (1998). Climate instability during last glacial stage: evidence from varve deposits at goting, district Chamoli, Garhwal Himalaya, India. Curr. Sci. 75, 850–855.
Prasad, S., and Enzel, Y. (2006). Holocene paleoclimates of India. Quat. Res. 66, 442–453. doi: 10.1038/s41598-018-23606-w
Prell, W. L., and Kutzbach, J. E. (1987). Monsoon variability over the past 150,000 years. J. Geophys. Res. 92, 8411–8425. doi: 10.1038/srep24374
Prell, W. L., and Van Campo, E. (1986). Coherent response of arabian sea upwelling and pollen transport to late quaternary monsoonal winds. Nature 323, 526–528. doi: 10.1038/323526a0
Rajagopalan, G., Sukumar, R., Ramesh, R., Pant, R. K., and Rajagopalan, G. (1997). Late Quaternary vegetational and climatic changes from tropical peats in southern India - an extended record up to 40,000 yr. B.P. Curr. Sci. 73, 60–63.
Ranhotra, P. S., Bhattacharyya, A., and Kotlia, B. S. (2007). Vegetation and climatic changes around Lamayuru, Trans-Himalaya during the last 35 kyr B.P. Palaeobotanist 56, 117–126.
Ranhotra, P. S., Sharma, J., Bhattacharyya, A., Basavaiah, N., and Dutta, K. (2017). Late pleistocene - holocene vegetation and climate from the palaeolake sediments, rukti valley, kinnaur, himachal himalaya. Quat. Int. 479, 79–89. doi: 10.1016/j.quaint.2017.08.025
Rawat, S., Gupta, A. K., Sangode, S. J., Srivastava, P., and Nainwal, H. C. (2015). Late pleistocene-holocene vegetation and indian summer monsoon record from the Lahaul, Northwest Himalaya, India. Quat. Sci. Rev. 114, 167–181. doi: 10.1016/j.quascirev.2015.01.032
Richards, B. W. M., Benn, D. I., Owen, L. A., Rhodes, E. J., and Spencer, J. Q. (2001). Timing of late quaternary glaciations south of mount everest in the Khumbu Himal, Nepal. Geol. Soc. Am. Bull. 112, 1621–1632. doi: 10.1130/0016-7606(2000)112<1621:TOLQGS>2.0.CO;2
Sanwal, J., Kotlia, B. S., Rajendran, C. P., Ahmad, S. M., Rajendran, K., and Sandiford, M. (2013). Climatic variability in Central Indian Himalaya during the last ∼1,800 years: evidence from high resolution speleothem record. Quat. Int. 304, 183–192. doi: 10.1016/j.quaint.2013.03.029
Sarkar, A., Ramesh, R., Bhattacharya, S. K., and Rajagopalan, G. (1990). Oxygen isotopic evidence for a stronger winter monsoon current during the last Glaciation. Nature 343, 549–551. doi: 10.1038/343549a0
Sekar, B. (2000). Interpretation of past climatic changes around Tsokar lake, Ladakh for the last 3 ka on the basis of chemical data. Palaeobotanist 49, 519–527.
Sharma, S., Joachimski, M., Sharma, M., Tobschall, H. J., Singh, I. B., Sharma, C., et al. (2004). Late glacial and Holocene environmental changes in Ganga plain, northern India. Quat. Sci. Rev. 23, 145–159. doi: 10.1016/j.quascirev.2003.10.005
Singh, G., and Agrawal, D. P. (1976). Radiocarbon evidence for deglaciation in north-western Himalaya, India. Nature 260:232. doi: 10.1038/260232a0
Singh, G., Joshi, R. A., Chopra, R. A., and Singh, A. D. (1974). Late Quaternary history of vegetation and climate of the Rajasthan desert, India. Phillos. Trans. R. Soc. Lond. 267, 467–501. doi: 10.1098/rstb.1974.0006
Singh, G., Wasson, R. J., and Agarwal, D. P. (1990). Vegetational and seasonal climatic changes since the last full glacial in the Thar Desert, northwestern India. Rev. Palaeobot. Palynol. 64, 351–358. doi: 10.1016/0034-6667(90)90151-8
Sinha, A., Cannariato, K. G., Stott, L. D., Li, H. C., You, C. F., Cheng, H., et al. (2005). Variability of southwest Indian summer monsoon during the Bølling–Ållerød. Geol. Soc. Am. 33, 813–816. doi: 10.1130/G21498.1
Sinha, R., Smykatz-Kloss, W., Stueben, D., Harrison, S. P., Berner, Z., and Kramar, U. (2006). Late Quaternary palaeoclimatic reconstruction from the lacustrine sediments of the Sambhar playa core, Thar Desert margin, India. Palaeogeogr. Palaeoclimatol. Palaeoecol. 233, 252–270. doi: 10.1016/j.palaeo.2005.09.012
Sirocko, F., Sarnthein, M., Lange, H., and Erlenkeuser, H. (1991). Atmospheric summer circulation and coastal upwelling in the Arabian Sea during the Holocene and the last glaciation. Quat. Res. 36, 72–93. doi: 10.1016/0033-5894(91)90018-Z
Sirocko, F., Sarnthein, M., Erlenkeuser, H., Lange, H., Arnold, M., and Duplessy, J. C. (1993). Century scale events in monsoonal climatic over past 24,000 years. Nature 354, 322–324. doi: 10.1038/364322a0
Smith, B. N., and Epstein, S. (1971). Two categories of 13C and 12C ratio for higher plants. Plant Physiol. 47, 380–384. doi: 10.1104/pp.47.3.380
Srivastava, P., Singh, I. B., Sharma, S., Shukla, U. K., and Singhvi, A. K. (2003). Late pleistocene-holocene hydrological changes in the interfluve areas of Central Ganga plain, India. Geomorphology 54, 279–292. doi: 10.1016/S0169-555X(02)00361-6
Stuiver, M., and Reimer, P. J. (1993). Extended 14C database and revised CALIB radiocarbon calibration program. Radiocarbon 35, 215–230. doi: 10.1017/S0033822200013904
Sukhija, B. S., Reddy, V. R., and Nagabhushanam, P. (1998). Isotopic Fingerprints of paleoclimates during the last 30,000 years in deep confined ground waters of southern India. Quat. Res. 50, 252–260. doi: 10.1006/qres.1998.2001
Sukumar, R., Ramesh, R., Pant, R. K., and Rajagopalan, G. (1993). A IJC record of the late quaternary climate change from tropical peats in southern India. Nature 364, 703–706. doi: 10.1038/364703a0
Thompson, L. G., Mosley-Thompson, E., Davis, M. E., Bolzan, J., Dai, J., Klein, L., et al. (1990). Glacial stage ice-core records from the subtropical Dunde ice cap, China. Ann. Glaciol. 14, 288–297. doi: 10.1017/S0260305500008776
Thompson, L. G., Mosley-Thompson, E., Davis, M. E., Bolzan, J. F., Dai, J., Yao, T., et al. (1989). Holocene–late pleistocene climatic ice core records from qinghai–tibetan plateau. Science 246, 474–477. doi: 10.1126/science.246.4929.474
Thompson, L. G., Yao, T., Davis, M. E., Handerson, K. A., Mosley-Thompson, E., Lin, P. N., et al. (1997). Tropical climate instability: the last glacial cycle from a Qinghai-Tibetan ice core. Science 276, 1821–1825. doi: 10.1126/science.276.5320.1821
Trivedi, A., and Chauhan, M. S. (2008). Pollen proxy records of holocene vegetation and climate change from Mansar Lake, Jammu region, India. Curr. Sci. 95, 1347–1354.
Trivedi, A., and Chauhan, M. S. (2009). Holocene vegetation and climate fluctuations in Northwest Himalaya based on pollen evidence from Surinsar Lake, Jammu region, India. J. Geol. Soc. India 74, 402–412. doi: 10.1007/s12594-009-0142-5
Van Campo, E. (1986). Monsoon fluctuations in two 20,000 yrs B.P. oxygen-isotope/pollen records off southwest India. Quat. Res. 26, 376–388. doi: 10.1016/0033-5894(86)90097-9
Van Campo, E., Duplessy, J. C., and Rossignl-Strick, M. (1982). Climatic conditions deduced from a 150 kyr oxygen isotope-pollen record from the Arabian Sea. Nature 296, 56–59. doi: 10.1038/296056a0
Wasson, R. J., Rajaguru, S. N., Misra, V. N., Agrawal, D. P., Dhir, R. P., Singhvi, A. K., et al. (1983). Late quaternary stratigraphy and palaeoclimatology of the Thar dune field. Z. Geomorphol. 45, 117–151.
Yan, G., Wang, F. B., Shi, G. R., and Li, S. F. (1999). Palynological and stable isotopic study of palaeoenvironmental change on the northeastern Tibetan plateau in the last 30,000 years. Palaeogeogr. Palaeoclimatol. Palaeoecol. 153, 147–159. doi: 10.1016/S0031-0182(99)00064-4
Zheng, Z., and Li, Q. (2000). Vegetation, climate and sea level in the past 50,000 years, Hanjiang delta, southeastern China. Quat. Res. 53, 330–340. doi: 10.1006/qres.1999.2126
Keywords: Central Himalaya, paleoclimate, stable carbon isotopes, fluvio-lacustrine profile, Last Glacial Maximum, Older Dryas
Citation: Sanwal J, Rajendran CP and Sheshshayee MS (2019) Reconstruction of Late Quaternary Climate From a Paleo-Lacustrine Profile in the Central (Kumaun) Himalaya: Viewing the Results in a Regional Context. Front. Earth Sci. 7:2. doi: 10.3389/feart.2019.00002
Received: 23 April 2018; Accepted: 07 January 2019;
Published: 22 January 2019.
Edited by:
Simon Allen, University of Zurich, SwitzerlandReviewed by:
Selvaraj Kandasamy, Xiamen University, ChinaParminder Singh Ranhotra, Birbal Sahni Institute of Palaeobotany, India
Copyright © 2019 Sanwal, Rajendran and Sheshshayee. This is an open-access article distributed under the terms of the Creative Commons Attribution License (CC BY). The use, distribution or reproduction in other forums is permitted, provided the original author(s) and the copyright owner(s) are credited and that the original publication in this journal is cited, in accordance with accepted academic practice. No use, distribution or reproduction is permitted which does not comply with these terms.
*Correspondence: Jaishri Sanwal, amFpc2hyaWdlb2xvZ3lAZ21haWwuY29t; amFpc2hyaUBqbmNhc3IuYWMuaW4=