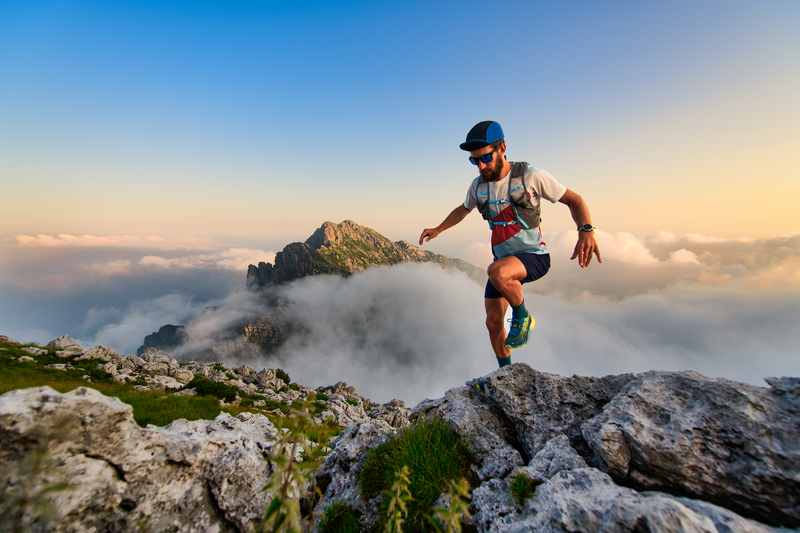
94% of researchers rate our articles as excellent or good
Learn more about the work of our research integrity team to safeguard the quality of each article we publish.
Find out more
ORIGINAL RESEARCH article
Front. Earth Sci. , 13 November 2018
Sec. Volcanology
Volume 6 - 2018 | https://doi.org/10.3389/feart.2018.00196
This article is part of the Research Topic Exploring Volcanic Paroxysmal Explosive Activity From Magma Source to Ground and Atmosphere View all 11 articles
Mt. Öræfajökull is one of the most dangerous volcanoes in Iceland with potential for a VEI6 eruption and the generation of many severe associated hazards. It is not a frequently erupting volcano with two eruptions in the last 1100 years, in 1362 and 1727–28. During the 1362 eruption 10 km3 of freshly fallen tephra was emitted, the eruption plume reached the stratosphere and was dispersed offshore toward mainland Europe. In this study we investigate the possible impact due to tephra fallout to critical infrastructures in Iceland namely – roads, airports, electrical power-lines – in case of a new eruption at Öræfajökull of similar intensity as in 1362. The analysis is done by running several times the VOL-CALPUFF dispersal model to simulate the dispersal of ash in the atmosphere and its deposition on the ground. The resulting maps show the probability of exceeding critical thickness of the tephra fall. Critical infrastructures have been added to the analysis to get a quantitative assessment of the potential impact. The results indicate that in case of an event similar to the 1362 eruption, the tephra fallout could be expected over most of the country, with higher likelihood on the eastern side. The tephra fallout is likely to have a severe impact in the proximity of the volcano, generating a deposit with a load of up to 1000 kg/m2. The likelihood of failure for more than 160 km of the electrical power-line and for critical driving conditions on about 900 km of the main ring road is between 50 and 100%. The probability that the tephra fall will affect three of the main domestic airports is higher than 50%. An eruption of this magnitude is likely to affect commuting and communication between the greater Reykjavík area, where the government resides, and the rest of the country. Our analysis also reveals the limitations of current knowledge and understanding of the Öræfajökull volcano and highlights the need for further studies on past activity to better characterize its future behavior.
There are about 30 active volcanic systems in Iceland and about half of those have featured tephra-producing eruptions (Thordarson and Höskuldsson, 2008). Volcanic eruptions are common in Iceland and have a recurrence interval of 2–5 years (Thordarson and Larsen, 2007).Basaltic eruptions are the most common volcanic events, and among them explosive within-glacier eruptions are most frequent because the most active central volcanoes are capped by glaciers (e.g., Katla, Grímsvötn, Bárðarbunga). Less frequent are explosive eruptions featuring more evolved magmas, such as dacite and rhyolite, that typify central volcanoes such as Öræfajökull and Hekla (Larsen and Eiríksson, 2008a,b). Highly active volcanic systems, as Hekla, Katla, Bárðarbunga and Grímsvötn, have explosive eruptions rates of 82, 97, 90, and 95%, respectively (CIV, 2017). Volcanogenic floods (Pagneux et al., 2015 and references herein), lava flows (Thordarson and Höskuldsson, 2008; Thordarson, 2013), tephra fallout (Larsen, 2002; Óladóttir et al., 2011; Janebo et al., 2016; Gudnason et al., 2017, 2018), lightnings (Bennett et al., 2010; Behnke et al., 2014), pyroclastic flows (Walker, 1962; Jørgensen, 1987; Thordarson and Höskuldsson, 2007; Tomlinson et al., 2010), are all phenomena associated with past eruptions in Iceland. Tephra dispersal and fallout is by far the most widespread hazard affecting local as well as distal regions. Ash clouds and tephra fallout can cause severe health issues (Baxter, 1990; Horwell and Baxter, 2006), affect important infrastructure like as electrical supply systems (Wilson et al., 2012), the national and international transportation network (Guffanti et al., 2009; Wilson et al., 2012), sensitive buildings (Spence et al., 2005), human health and life stock, vegetation and eco-system (Thorarinsson, 1979; Wilson et al., 2012; Ágústsdóttir, 2015).
It was during the infamous eruption at Eyjafjallajökull in 2010 when a persistent northwesterly winds carried the ash-rich plume toward Europe for more than a month (Baerbel et al., 2012; Gudmundsson et al., 2012). On that occasion a prolonged closure of the European airspace resulted in severe economic impact estimated to be € 1.3 billion in the first week of the eruption (Bolić and Sivčev, 2011). The southernmost tip of Iceland experienced heavy tephra fallout, which impacted the local residence in various ways. Situation of low visibility happened often during the eruption as well as in its aftermath because of resuspension of ash (Petersen, 2010; Karlsdóttir et al., 2012). Air quality was often poor and affected the health of population living closest to the volcano (Carlsen et al., 2012). Relocation of life stock became essential due to heavy tephra fallout (Karlsdóttir et al., 2012; Thorvaldsdóttir and Sigbjörnsson, 2015). Specific investigations were done during the Eyjafjallajökull eruption to assess the effect of ash contamination on electrical power plants (Rarik, 2010). Eruptions at Hekla volcano have also been investigated to assess their effect on the environment and eco-system (e.g., Frogner et al., 2006). Heavy tephra and lapilli fallout during the eruption at Heimaey (Vestmannaeyjar Island) in 1973 destroyed and damaged several houses (Williams, 1983; Spence et al., 2005; Gudmundsson et al., 2008), some of those were restored after extensive cleaning effort to remove the tephra fall deposit.
In the period 2013–2016 the Icelandic Government, together with the International Civil Aviation Organization (ICAO), supported several projects aimed at assessing in a quantitative manner the long-term volcanic hazard in Iceland, to be used for more in-depth risk analysis. A specific component of these projects was the investigation of the impact of tephra fallout in Iceland for both medium-size, more frequent, and large, less frequent, explosive eruptions. Öræfajökull volcano was selected as the low probability but high-impact scenario.
Here, we focus on the AD 1362 Öræfajökull event as the most extreme scenario for a regional tephra fallout hazard and a preliminary risk assessment. The sparse eruption records for Öræfajökull introduces uncertainties in the volcanological scenario considered and we address this issue by using a numerical model to investigate the sensitivity of model results to variations in the volcanological input parameters.
The results of this study are presented through probabilistic hazard maps. These type of maps have become a commonly practiced representation of volcanic hazards and helps with the visualization of the footprint of the volcanic phenomena that may impact the surroundings of a volcano (Haynes et al., 2007; Nave et al., 2012; Calder et al., 2015; Thompson et al., 2015). Different types of hazard maps exist in literature. They can be produced on the basis of geological data, historical records and/or numerical model results (Calder et al., 2015; Loughlin et al., 2017). They can refer to a past eruption, to a specific hypothetical eruptive scenario or to a distribution of scenarios. If based on numerical results they can show the results from a single specific simulation (deterministic map) or from a multitude of scenarios. In the latter case the maps are often representing the impact of a specific hazard as a spatial probability and we refer to them as “probabilistic hazard maps.” Hazard maps are often used by volcano monitoring institutions to inform their stakeholders (e.g., decision makers institutions, general public, emergency managers, land-plan managers) about areas prone to be affected by specific hazards in case of an eruption. On a map it is easy to visualize extent of borders plus location of sensitive infrastructures, roads, towns and villages and, therefore, put the hazards into a spatial context that can be perceived more effectively by the users. Volcanic hazard maps have been produced for several volcanoes using numerical models and are applied for long-term hazard and risk assessment at particular volcanoes. For example, hazard maps have been produced for pyroclastic density currents at Mt. Vesuvius and Napolitean area (Esposti Ongaro et al., 2002, 2012; Sandri et al., 2018); for lava flows at Etna (Favalli et al., 2005; Tarquini and Favalli, 2013), Nyiragongo volcano (Favalli et al., 2009) and Lanzarote (Felpeto et al., 2001); for volcanogenic floods at Öræfajökull volcano (Pagneux et al., 2015). Probabilistic hazard maps for tephra fallout have been produced for Mt. Etna (Scollo et al., 2013), Campi Flegrei (Costa et al., 2009), Tarawera volcano (Bonadonna et al., 2005), Indonesian volcanoes (Jenkins et al., 2012), Santorini volcano (Jenkins et al., 2015). Most recently probabilistic maps for hazard due to ejection of ballistic have been produced for Mt. Chihshin in North Taiwan (Nurmawati and Konstantinou, 2018).
Here, we have produced probabilistic hazard maps to investigate the potential impact at a national level of a VEI6 (Newhall and Self, 1982) eruption at Öræfajökull. The impact of tephra fallout on infrastructures is calculated via a numerical model that simulates the atmospheric dispersal of tephra and its deposition. GIS-referenced layers with information on the powerline network, roads, airports are included to assess potential disruptions to services and commuters. Possibly, these results will support the national authorities in planning and designing mitigation actions that are necessary to reduce the risks posed by a future eruption at Öræfajökull. A more complete hazard assessment should also include the investigation of smaller eruptions (VEI4) as they appear to be most frequent events at Öræfajökull as reported in Section “Geological Background.” The choice to look first at a VEI6 eruption was dictated by the need to quantify the potential damage the worst case scenario would cause to the society nowadays. The indications provided by the Icelandic Civil Protection addressed this research and supported the worst case scenario as the reference scenario for Öræfajökull.
Öræfajökull is an ice-capped stratovolcano located in South-East Iceland on the southern margin of Vatnajökull glacier (Thordarson and Larsen, 2007; Gudmundsson et al., 2008; Sharma et al., 2008; Larsen et al., 2015). It is about 20 km in diameter with a 3 by 4 km ice-filled caldera which rises to a summit of 2110 m a.s.l. (Figure 1). The volcano is part of the intraplate Öræfajökull Volcanic Belt, situated to the east of the current plate margins and possibly represents an embryonic rift (e.g., Thordarson and Larsen, 2007; Thordarson and Höskuldsson, 2008).
FIGURE 1. Map showing the locations of Öraefajökull volcano and the main sites mentioned in the text. On top of it the reconstructed deposit for the 1362 AD eruption is displayed.
The Öræfajökull central volcano has featured two explosive eruptions in historical times (e.g., Thorarinsson, 1958). The most recent was a small icelandite eruption of VEI4 in 1727–1728 CE (e.g., Larsen et al., 1999; Larsen et al., 2015). This was preceded by a much larger rhyolitic Plinian (VEI6) eruption in 1362 CE. Studies on the tephra stratigraphy in soils around the volcano have revealed five prehistoric silicic explosive eruptions at Öræfajökull and all are assumed to be smaller in magnitude and intensity than the 1362 CE event (Gudmundsson, 1998; Larsen et al., 2015).
In Iceland VEI6 events are infrequent and only three such events are known during the Holocene: the before mentioned 1362 CE event at Öræfajökull along with the two largest Holocene silicic explosive eruptions in Iceland, the 3 ka H3 and 4.2 ka H4 events at the Hekla volcanic system (e.g., Larsen and Thorarinsson, 1977; Stevenson et al., 2015).
The 1362 CE Öræfajökull eruption is the largest rhyolitic eruption in Iceland since settlement in the 9th Century, with an estimated volume of freshly fallen tephra of 10 or ∼6 km3 of compacted tephra and about 2 km3 when calculated as dense rock (Thorarinsson, 1958). Sharma et al. (2008) obtained a smaller volume of 2.3 km3 assumed to equal 1.2 km3 calculated as dense rock. Early stage pyroclastic density currents and intercalated jökulhlaups, along with the subsequent tephra fall, inundated the then prosperous farming district “Litla Hérað” causing fatalities (e.g., Thorarinsson, 1958; Jónsson and Valdimar, 2007; Thordarson and Höskuldsson, 2007). The reconstructed tephra dispersal is shown in Figure 1, where isopachs (i.e., lines of equal thickness) for the 1362 eruptions are shown as black lines, and the dashed lines indicate the inferred dispersal over the sea. About half of the country received >1 mm of ash as a consequence of the tephra fall from this eruption. Close to the volcano up to 20 cm of ash has been accumulated over an area of 1000 km2, peaks in the deposit thickness are found in Grófarlækur (40 cm) at about 10 km from the summit volcano (Thorarinsson, 1958) and between Hnappavellir and Fagurhólsmýri where the thickness reached 2 m (Jónsson and Valdimar, 2007; Sharma et al., 2008) (Figure 1). Tephra from Öræfajökull has been identified in Western Europe (e.g., Pilcher et al., 2005) and in Greenland ice-cores (e.g., Palais et al., 1991).
The magma erupted in 1362 is a medium-K alkali rhyolite that is extremely homogeneous in composition. It is thought to be extracted from the topmost portion of a compositionally stratified magma storage zone, although there is no geophysical indication of any upper crustal (<15 km depth) magma storage zone beneath Öræfajökull at present. In contrast to the East Volcanic Zone and other rift zone rhyolites, which demonstrably are generated by partial melting of hydrated metabasaltic crust (e.g., Sigmarsson et al., 1992), the Öræfajökull and other silicic magmas generated by intraplate volcanoes are inferred to have evolved from a more mafic parent via fractional crystallization (e.g., Selbekk and Tronnes, 2007; Martin and Sigmarsson, 2007; Sigmarsson et al., 2008).
From September 2017 Öræfajökull volcano has showed clear indications of unrest. The reinvigoration of the geothermal activity beneath the volcano was corroborated by elevated seismicity, gas release and the formation of a cauldron in the middle of the caldera. Consequently, the Icelandic Meteorological Office raised the color code for Öræfajökull to yellow1 in November 2017. The aviation color code was turned back to green on 4th May, 2018 when the main monitoring parameters indicated a stable situation with no immediate hazard to the aviation. However, given the persistent potential for local hazards, the Icelandic Civil Protection decided to maintain the level of Uncertainty for Öræfajökull (IMO, 2018a).
In the period November 2017 to May 2018, a priority has been placed on improving the real-time monitoring around Öræfajökull. At the same time, hazard, and risk assessments performed for glacial outbursts originating from this volcano (Pagneux et al., 2015) were provided to the Department of Civil Protection and Emergency Management of the National Commissioner of the Icelandic Police to finalize the evacuation plans for the Öræfi district (NCIP, 2017). The preliminary study on tephra fallout and its potential impact on infrastructures at a national level, here presented, was also finalized.
At the time of writing the seismicity is elevated and higher than in November 2017. The deformation data (from both cGPS and InSAR analysis) reveal clear indication of an on going inflation process likely due to injection of new magma at depth (IMO, 2018b).
The methodology used for the hazard assessment in this study is shown in Figure 2. It consists of four main steps: (1) identification of the scenario of interest (this can be defined on the basis of an Event Tree outcome or from literature); (2) initialization of VOL-CALPUFF model (Barsotti et al., 2008) by using a synthetic scenario (selected eruption source parameters to be used as a model input) as well as a range of meteorological scenarios); (3) execution of several numerical runs by using different starting times; (4) statistical processing of the results from multiple runs. The obtained probabilities, as visualized in the final maps, are those called “conditional probabilities,” i.e., conditioned to the occurrence of that specific eruptive scenario.
FIGURE 2. The four main steps at the base of this study. It starts with the identification of the scenario of interest (and its volcanological parameters), the synthetic scenario is designed to fulfill the need of input data required to run the numerical model, several simulations are executed by using different meteorological conditions in input and finally the multitude of results are integrated in one single probabilistic map.
Available publications (Thorarinsson, 1958; Jónsson and Valdimar, 2007; Thordarson and Höskuldsson, 2007; Sharma et al., 2008), provide the general framework for the eruption source parameters used in the dispersal simulations. Calculating the total mass emitted during the Plinian phase of the eruption is a first order estimate because large portionof tephra fell onto the sea (see Figure 1). Using the values given in Thorarinsson (1958) the total mass is estimated to be 4.8 × 1012 kg (Table 1).
TABLE 1. Volcanological parameters characterizing the 1362 eruption at Öræfajökull as reported in Thorarinsson, 1958.
A GIS referenced reconstruction of the original map by Thorarinsson (1958) is used to recalculate the erupted tephra mass by (1) assuming a constant thickness between the different isopachs and (2) by interpolating between two successive isopachs assuming a linear trend. These two estimates give an erupted tephra mass of 4.29 × 1012 and 6.96 × 1012 kg, where the latter is about factor of 1.5 larger than that obtained from Thorarinsson (1958) data (see Table 2).
TABLE 2. Estimation of total mass emitted during the 1362 eruption by using different ways to interpolate the isopachs drawn by Thorarinsson (1958).
In order to run a dispersal model several input parameters need to be defined. VOL-CALPUFF model requires initial values of parameters like the vertical exit velocity of the volcanic mixture, the vent radius, the total grain size distribution (TGSD). As we currently have no information on the values for these variables, they are obtained via iteration by running VOL-CALPUFF model with a range of input values for the above mentioned variables. The output values selected are those that produced the best fit between the modeled and the observed deposit distribution as well as the eruption column heights. In an attempt to reproduce the scenario reported in Table 1 and to match the isopachs as depicted in Thorarinsson (1958), the VOL-CALPUFF dispersal model was run multiple times using a vent radius from 150 to 300 m; a gas mass fraction from 1 to 5% and three different TGSDs (one peaked at phi = -3, one peaked at phi = 0 and a bi-modal distribution with two peaks at phi = -3 and phi = 2, respectively). By a comparison of the model results and the original isopach map and by constraining the top plume height between 24 and 34 km, the best fit was obtained by using the following input parameters:
1. Vertical velocity: 300 m/s
2. Vent radius: 300 m
3. Gas mass fraction: 3%
4. Total grain size distribution: bi-modal (see Figure 3).
5. Mass flow rate: 4.24 × 108 kg/s
6. Duration of the emission: 18 h
FIGURE 3. Reconstructed Total Grain Size Distribution (TGSD) for a typical rhyolitic Plinian fall deposit (adapted from Stevenson et al., 2015) used for the synthetic scenario. Important to note that no accretionary lapilli have been identified in the AD 1362 fall deposit (Thorvaldur Thordarson pers. com. 2018).
A forward run of the plume model with these input parameters produced the following values for plume height and tephra emitted mass (to compare with values reported in Table 1):
1. Plume height: 23.5–37 km a.s.l.
2. Total erupted tephra mass: 2.75 × 1013 kg
This synthetic scenarios is in a reasonable agreement with anticipated duration of the Plinian phase and plume height. However, there is a larger discrepancy in terms of mass. The new numerical simulation results suggest that in order to match the original isopachs, the DRE volume is 5.5 km3. This volume is 2.75 times larger than that provided by Thorarinsson, and 1.9 times larger than the value calculated with the GIS interpolation (Table 2). Considering the huge uncertainites affecting the meteorological conditions during the eruption, the real extension of the deposit and the few observational data available, we considered valuable in this study to use those input parameters obtained through the matching procedure to perform the probabilistic assessment.
The tephra dispersal from the synthetic scenario is compared with the isopachs reconstructed for the real event in Figure 4. The gray filled contours are the model results whereas the solid black lines are the deposit isopachs as reconstructed by Thorarinsson (1958). A westerly wind has been selected to run the dispersal and match the general feature of the deposit pattern. For the same simulation (i.e., by assuming the same input condition) the model reproduced a super-buoyant plume with height of 30.6 km above the vent.
FIGURE 4. Model results are overlayed with the deposit isopach for the 1362 eruption. The simulation has been run by using a wind field characterized by a westerly wind to match the prevailing direction of the deposit.
Plume ascent is described solving plume theory equations (Bursik, 2001) to compute column height as a function of volcanological source input data and wind field parameters. The latter are relevant for simulating weak plumes that are strongly affected by wind shearing. During plume ascent the heaviest particles fall from the column and a lighter mixture continues its upward motion, entraining air up to a null-vertical velocity altitude where only lateral dispersion takes place. The plume initially decelerates due to higher density compared with surrounding atmosphere, but due to heating of entrained air (mixed by turbulent motions) the mixture can eventually become lighter than air. Buoyancy effect can cause the mixture to accelerate upward until an equilibrium is achieved.
The dispersal code VOL-CALPUFF originates from the CALPUFF model and it has been modified to reproduce some processes specific of a volcanic eruption, e.g., plume rise phase and the dispersal of a distribution of solid particles. It is a hybrid model in which the plume rise phase is described with a Eulerian approach, whereas the ash cloud transport is solved in a Lagrangian framework. Along the plume and at its top the material is released as a series of diffusing packets (puffs) containing an initially assigned amount of particulate matter which varies during the transport due to gravitational fallout. Since its development the VOL-CALPUFF model has been applied mostly at Mt. Etna to reconstruct past explosive events (Barsotti and Neri, 2008; Barsotti et al., 2008), as an ash dispersal forecasting tool (Barsotti et al., 2008) and to estimate potential hazards posed by volcanic ash to human health and ground infrastructures (Barsotti et al., 2010). In the past years VOL-CALPUFF has been also applied to other active volcanoes to produce forecasting maps of ash dispersal during eruptive crises at Redoubt Volcano (Alaska) in 2009, Eyjafjallajökull (Iceland) and Mount Merapi (Indonesia) in 2010 (Barsotti et al., 2011; Spinetti et al., 2013) and Grímsvötn (Iceland) in 2011.
Numerical models can be used to investigate the behavior of a specific process process (Kavanagh et al., 2018) as for example dispersal of ash (Bonadonna et al., 2011; Folch, 2012), lava flow invasion (Del Negro et al., 2005; Favalli et al., 2005, 2009), maximum distances of pyroclastic flow (Esposti Ongaro et al., 2012; Dufek, 2016). Each simulation needs specific input conditions to characterize the volcanological scenarios to be investigated. For volcanic ash dispersal simulation the eruptive source parameters (ESP) as plume height, particle size distribution, mass flow rate need to be quantified (Mastin et al., 2009). As we do not know in advance about the next eruptive conditions, like the weather and the ESP, a way to treat this uncertainty is to reflect this into a probabilistic analysis. Looking into a range of eruptive scenarios it is possible to get a statistics that would investigate and reflect the uncertainty in the assumption made analyzing a single scenario. By running the model with several sets of starting conditions enables us to estimate the probability that a specific area will be affected by a certain type of hazard. A method widely used to achieve such a result is called Monte Carlo approach and is based exactly on the assumption that a model could be executed multiple times for as many initial conditions as required for producing an ensamble modeling (Sparks and Aspinall, 2013). The application of this approach for a probabilistic analysis of a simulated volcanic process can be found in Cioni et al. (2003), Hurst and Smith (2004), Bonadonna et al. (2005), Macedonio et al. (2008), Costa et al. (2009), Barsotti et al. (2010), Scaini et al. (2012, 2014), Scollo et al. (2013), Biass et al. (2014), Bonasia et al. (2014).
A Monte Carlo simulation is performed for this study by running several times the dispersal model VOL-CALPUFF for a fixed volcanological scenario (the 1362 AD reference eruption) and by using several years of meteorological data. Each simulation is performed using the same input data given in Section “Scenario Definition.” The simulations are initiated with different starting date and times of the day, over a period of 10 years. This is done to ensure the randomness of the analysis and to avoid bias in the results due to the daily variations of the atmospheric parameters as we only investigate the effect of the wind field statistics on the tephra dispersal. The potential variability associated with the uncertainty in the volcanological scenario is not considered. A total of 500 simulations were performed in order to obtain a convergence for the modeled tephra dispersal distribution.
Forecast data produced by the Europen Centre of Medium-range Weather Forecast is used to run the VOL-CALPUFF model. The meteorological data for the probabilistic maps have been downloaded from the ERA-INTERIM archive and cover a period of 10 years, from 1980 to 1991. This dataset is produced by re-analyzing the forecast, i.e., by assimilating the observational data into the original weather forecast (Dee et al., 2011). This means that this dataset provides a quite complete and verified description of the 3D atmospheric fields over the period of interest. On the other side the horizontal resolution of the meteorological data is of 0.7° (i.e., about 35 and 77 km in the longitude and latitude respectively), making the spatial resolution of this dataset a bit coarse for the domain considered in this project. The statistics for the investigated area around Öræfajökull produced by using the ECMWF data over this time period is shown in Figure 5.
FIGURE 5. The nine windroses show the wind direction (the sectors correspond to the direction from which the wind blows) and speed (different colors) at different altitudes over Vatnajökull glacier. 1000 mb corresponding roughly to the ground level up to 20 mb corresponding about to 24000 m asl. Ten years of ECMWF data (1980–1990) have been processed to produce these windroses.
In Figure 5 each windrose shows the direction of provenance for the wind field, identified by the sector, and the wind velocity, identified by the color. Each windrose is produced for a specific altitude expressed in pressure from 1000 mb (surface level) to 20 mb (∼25 km). At low levels (1000 mb, a in Figure 5) the wind is weak, with wind velocity between 5 and 10 m/s. The prevailing direction at this altitude is location dependent, and has a NE component over Vatnajökull. Higher up in the atmosphere the wind field is more uniform and less affected by the surface topography. The velocity tends to increase moving higher up to 300 mb (9–10 km, f in Figure 5). Further up the wind velocity decreases to increase again at 20 mb (i in Figure 5) where there is a clear W-E directionality, with Easterly winds weaker than Westerly ones.
Tephra can have wide type of impacts either nearby and far away the volcano. As reported in literature, at ground level volcanic tephra can cause:
1. health issues (Baxter, 1990; Horwell and Baxter, 2006);
2. roofs/building collapse (Spence et al., 2005);
3. poor visibility conditions (Blong, 1996);
4. dangerous road conditions (Wilson et al., 2012; Blake et al., 2017);
5. contamination of water reservoirs and vegetation (Wilson et al., 2012; Ágústsdóttir, 2015);
6. damages to electrical infrastructures (Bebbington et al., 2008; Wardman et al., 2012; Wilson et al., 2012);
7. transportation system disruptions (Casadevall, 1994; Guffanti et al., 2009; Wilson et al., 2012);
8. impact on telecommunication networks (Wilson et al., 2012).
Composition of the tephra, its grain-size distribution and presence of precipitation might enhance some of these hazards, as for example roof collapse conditions, damages to electrical infrastructure and contamination of water and vegetation. Wet tephra can reach higher load due to the contribution of rain that remains trapped in the deposit (Macedonio and Costa, 2012). This means that tephra fallout might have a different impact on buildings if it rains during or shortly after the eruption. Similarly, wet conditions might affect the conduction properties of tephra enhancing its effect in flashover events (Wilson et al., 2012). Finally silicic tephra can be strongly toxic for humans, pollute water supplies and poison grazing animals (Thorarinsson, 1979; Cronin et al., 2003).
Here, we investigate the potential impact of tephra fall on three key infrastructures, because if damaged, it can result in prolonged disruptions to the local population. The primary/direct hazards due to tephra fall onto airports, roads and power-lines are here considered.
Tephra on roads is an issue for traffic safety. It can result in the reduction of tire friction, obscure road markings, cause blockage of engine air intake filters and reduce the visibility for drivers. Few studies have properly investigated the thresholds of tephra fall capable to trigger critical driving conditions. Recently, Blake et al. (2017) presented results of laboratory experiments on how the properties of tephra fall (thickness, particle composition, particle size) along with precipitation can compromise skid resistance. These results showed that for a 1 mm-thick fallout the skid resistance is below the “difficult site” safety levels. In case of thicker deposit (>5 mm) the conditions are more favorable, with skid resistance above the “safe” value. The study concludes that tephra fall thickness of 5 mm is the critical limit for which mitigation actions need to be taken to guarantee safe driving conditions on roads. At the same time Blake et al. (2017) presents a list of road disruptions occurred at volcanoes worldwide and shows how in several cases deposit several-10s-of-mm-thick have also been causing difficulties to the ground transportation. In this study we use a threshold value of 3 mm to represent disruptive conditions on the roads.
As reported in Guffanti et al. (2009) and Prata and Tupper (2009) the primary hazard to airports is ashfall, which can cause loss of visibility, create slippery runways, infiltrate communication and electrical systems, interrupt ground services, and damage buildings and parked airplanes. The skid resistance analysis perfomed by Blake et al. (2017) can be partly applied to airfield and runways, even though no clear thresholds exist for this environment with each airport operating authorities responsible for mantaining the runways functional and secure. Some critical conditions described for roads can be also applied to airfield and runways with few-mm ash deposit- to be considered a condition that can be critical for safe operations. Here, we use a threshold value of 1 mm to investigate the impact on airports.
Several papers discuss the vulnerability of electrical instrustructure to volcanic ash (Bebbington et al., 2008; Wardman et al., 2012; Wilson et al., 2012) and are used to identify correlation between specific thresholds of ash thickness and the level of impact (low, medium, and high) it would have on electrical infrastructure (Wilson et al., 2012). Critical infrastructure as power-lines have been investigated for damages due to:
insulator flashover;
electrical tower and pole damage;
electrical line damage.
The effect of tephra fall on this type of infrastructure depends on three main factors: the grain-size, the tephra load and presence of precipitation (wet deposition).
In this study we only look into those critical conditions associated with fine tephra because, as shown in Figure 3, this is the assumption we use for an eruption at Öræfajökull. Table 3 summarizes the results as reported in the mentioned paper for fine tephra. For a deposit larger than 5 mm in wet conditions there is a high likelihood of insulator flashover. Damages to tower, poles and lines are highly likely for thicknesses larger than 100 mm in dry conditions and 5 mm in wet conditions. In the following analysis we have been considering the likelihood to get deposit larger than 10 and 100 mm in dry conditions. The assumption is that these limits can be considered as of danger in wet condition for a fully wet deposit (i.e., all the voids in the deposit are full of water).
TABLE 3. Tephra fallout conditions investigated to cause insulator flashover and pole and line damages for wet and dry ash (as reported in Wilson et al., 2012).
We performed numerical simulations of volcanic tephra dispersal by using VOL-CALPUFF code. The simulations yielded tephra thicknesses and concentrations data points over Iceland. We then generated probabilistic hazard maps for tephra loading at given thresholds by adopting a Monte Carlo approach. Here, we present three probabilistic hazard maps (Figures 6–8) for tephra loading thresholds of 1.0 kg/m2 (equivalent to about 0.1 cm under the assumption of a deposit of 1000 kg/m3), 100 kg/m2 (equivalent to about 10 cm) and 1000 kg/m2 (equivalent to about 1 m).
FIGURE 6. Probabilistic hazard map for tephra loading higher than 1.0 kg/m2 given an eruption at Öræfajökull as 1362 AD. The isopachs reconstructed by Thorarinsson (1958) are reported as a reference with black lines.
FIGURE 7. Probabilistic hazard map for tephra loading higher than 100 kg/m2 given an eruption at Öræfajökull as 1362 AD. The isopachs reconstructed by Thorarinsson (1958) are reported as a reference with black lines.
FIGURE 8. Probabilistic hazard map for tephra loading higher than 1000 kg/m2 given an eruption at Öræfajökull as 1362 AD. The isopachs reconstructed by Thorarinsson (1958) are reported as a reference with black lines.
Comparison of these three maps reveals that the higher deposit load threshold investigated, the smaller is the area likely interested by this load. This is because in each simulation the thick deposit impacts a proximal area around the volcano, whereas thinner deposit can spread over a larger domain. In addition, in all maps there is a general eastward trend that reflects the meteorological statistics, as shown in Figure 5. At high altitudes the stronger westerly wind dominates the distribution of tephra toward the East.
In Figure 6 the isoline of 0.1 cm thickness produced by Thorarinsson (1958) falls entirely within the 25–50% probability of reaching this deposit thickness. All the country, apart from the northern part of the Westfjords, has at least a 5% probability of exceeding a ground load threshold of 1 kg/m2.
In Figure 7 the isoline of 10 cm produced by Thorarinsson (1958) falls entirely within the 75–100% probability of reaching this deposit thickness. About half of the country has at least a probability of 1% of exceeding a ground load of 100 kg/m2. This area includes eastern and South-Eastern Iceland, most of the highlands and parts of the North–East.
In Figure 8 the likelihood of exceeding a threshold of 1000 kg/m2 is confined to a small area closest to the volcano edifice. Part of the proximal area enclosed within the 20 cm isoline falls within the 5–25% probability of reaching a deposit thickness of 1 m.
The potential impact of tephra fallout can also be investigated at specific locations by performing an analysis site by site. For example Table 4 shows the likelihood of exceeding specific tephra ground loads at five key population centers in Iceland (Figure 1). All of these population centers have a likelihood higher than 5% of experiencing tephra fallout deposit exceeding a thickness of 1 mm. Höfn (97.8%) and Egilsstaðir (61.4%) are the most vulnerable centers as they are closest to the volcano. Höfn can experience very high loads (10 and 100 kg/m2) and the likelihood is about 95 and 73%, respectively.
TABLE 4. Likelihood of receiving specific amounts of tephra is calculated for five main towns in the country.
Reykjavík is the less exposed town due to its distance from the volcano and mainly because it is located upwind the dominant wind direction. Akureyri (in the North), despite its long distance, is showing intermediate values.
An analysis to investigate how these results translate into an impact assessment has been done by quantifying the level of disruption due to tephra fallout on roads, airports, and powerline network. The type of disruption has been defined with regards to: (1) kilometers of roads potentially exposed to critical driving conditions, (2) number of airports potentially affected by tephra fallout on the runways, and (3) kilometers of powerline potentially affected by tephra fallout that can cause either flashover and physical damage to the lines. For each of this infrastructure a map has been created and the results are shown in Figures 9–11.
FIGURE 9. Map showing potential impact of tephra fallout to roads in case of a 1362-like eruption at Öræfajökull.
FIGURE 10. Map showing potential tephra fallout impact to airport in case of a 1362-like eruption at Öræfajökull.
FIGURE 11. Map showing potential tephra fallout impact to power-lines in case of a 1362-like eruption at Öræfajökull.
When looking at the possible disruption to road traffic (Figure 9) we can see that up to 268 km of the main road system (either paved and unpaved according to the National Land Survey IS50V database v3.4, 2012) could be affected by tephra fallout thicker than 3 mm with a likelihood between 75 and 100% (red road sector). There is a 25% likelihood that more than 2000 km of the road network can be affected by this condition (the yellow, orange, and red road sector). The main road extending from Vík, in the South, to Húsavík, in the North, passing through the East part of the country will be impassable (see Figure 1 for site locations).
The analysis performed for the airports network includes all the main airports in the country for which flights are regularly scheduled (as reported from the National Land Survey IS50V database v3.4, 2012). The analysis reveals that the airport in Hornafjörður will be affected by a deposit thicker than 1 mm with a probability between 75 and 100%. The likelihood for two more airports (Egilsstaðir and Vopnafjörður) to receive this amount of tephra is between 50 and 75%. These three airports are located in the Eastern sector of the country. The international airport of Akureyri shows a likelihood between 25 and 50%, whereas the domestic airport in Reykjavík is calculated to have a likelihood between 5 and 25%. The International airport of Keflavík has potential to be affected by tephra fallout from Öræfajökull volcano with a likelihood between 1 and 5%. All the main airports of Iceland (14 in total) have >0% likelihood to be affected by tephra fallout capable to create disruption to the operations of landing and taking off.
Figure 11 shows a zoomed domain around Öræfajökull, as the main impact on the power line network is assessed to be quite proximal to the volcano. Only the network owned by the principal Icelandic provider Landsnet, which operates >33 kV powerage line, has been considered here. For this investigation a threshold of 100 mm of ash has been adopted, as given in Tables 3, 4. We assume this condition, corresponding to a load of about 100 kg/m2, to correspond to critical conditions for powerlines. The results show that up to 115 km of powerline network will be exposed to such a load with likelihood of 75 to 100%. This is the part of the powerline passing nearby the volcano at a minimum distance of about 9.5 km (red line sector). Additional 45 km will be exposed to such load with a likelihood between 50 and 75%. So that overall more than 160 km of the powerline network can be damaged by an eruption at Öræfajökull and the likelihood for such an occurrence is greater than 50%. Almost the entire powerline network feeding the Öræfi district is vulnerable to these effects.
looseness1 An eruption at Öræfajökull is likely to have a significant impact in Iceland, its nearby Nordic countries and, Europe. As evidence shows, such an eruption can produce pyroclastic density currents that will inundate the communities at the base of the volcano, glacial outbursts can also cover a substantial area (possibly >300 km2) around the volcano. On top of that a heavy tephra fallout is expected to occur over a period of about 1 day (e.g., Thorarinsson, 1958; Jónsson and Valdimar, 2007; Thordarson and Höskuldsson, 2007; Gudmundsson et al., 2008).
The volcanological scenario selected for this study represents the worst case scenario known to have happened at Öræfajökull, the 1362 AD eruption. The model results and the probabilistic hazard maps clearly indicate how, in case of a similar event, the associated tephra fallout might have effects all over the country. However, the location of the volcano, in the southernmost tip of the island, together with the prevailing westerly wind at high altitude favor the tephra fallout and ash dispersal to occur mainly toward the East. This will possibly represent a serious issue for the air traffic over the European air space, as occurred in 2010 when Eyjafjallajökull volcano erupted (Reichardt et al., 2018).
This study highlights two main issues: firstly, there are no places in the country completely safe from receiving ash generated from an eruption like 1362 at Öræfajökull (Figure 6); second, the tephra fallout can have a very severe impact in the proximity of the volcano with up to 1000 kg/m2 of ash expected up to a distance of only 25 km the vent (Figure 9). Most of the main towns in Iceland have likelihood higher than 1% to receive an amount of ash larger than 10 kg/m2 (Table 4).
The impact analysis performed for three different types of infrastructures (roads, airports, and power lines) reveals the vulnerability of the country in case of such an eruption. Figure 9, which shows the kilometeres of road network potentially affected by critical driving conditions, suggests that the entire Eastern part of the country will be hardly reached by car either through the Southern and the Northern ring road section. The main town of Egilsstaðir and the very popular localities of Höfn, Skaftafell and Jökulsarlón will be cut off the main viable connections, with important implications for either inhabitants and tourists potentially trapped in the area due to very low visibility conditions and unsafe driving conditions of paved roads.
The results show also that the likelihood for the airports in Hornafjörður (next to Höfn) and Egilsstadir to be disrupted by the tephra fallout is >50% (Figure 10). Consequently, connection with the Eastern part of the country via air or road will be very difficult and dangerous during and shortly after the eruption. An eruption like 1362 will affect the communication and commuting between the East part of the country and the Greater Reykjavík area (i.e., the capital and its surroundings).
Failure of the electricity provision can be expected due to damages to powerlines during and shortly after the eruption (Figure 11). These data need to be seriously evaluated when planning for mitigation actions and evacuation plans. The impact of a similar scenario would be even more dramatic if an eruption will take place during the winter time, when the daylight time is very short and the need for electricity to illuminate is higher and essential for the daily activities of the society and its economy.
The results shown so far are just the beginning of a long study that is needed to fully address the volcanic hazard at Öræfajökull volcano, but still enough to identify the potential critical scenario that could raise if it will erupt again with a similar intensity as in 1362. The unrest phase declared in November 2017 has been putting emphasis on the need of a proper multi-hazard assessment for Öræfajökull and this study is a contribution to it.
SB worked on the definition of the volcanological scenarios, performed the simulations, processed the data and wrote the interpretation, and discussion of the results. DDR worked on the volcanological scenarios and performed part of the simulations, processed the data and contributed to the discussion of the results. DDR, BBB contributed to the interpretation of the results, used GIS to recalculate the masses, and prepared all the graphical maps. TT worked on the definition of the volcanological scenarios and contributed to the discussion of the results. SK contributed to the discussion and interpretation of the results.
This study has been benefiting from the GOSVÁ project (2012–2018) funded by the Icelandic Government together with the International Civil Aviation Organization (ICAO).
The authors declare that the research was conducted in the absence of any commercial or financial relationships that could be construed as a potential conflict of interest.
A great thank goes to the Steering Commitee of the project for fruitful and constructive discussion. Marco Viccaro, the reviewer and the Chief Editor Valerio Acocella are kindly thanked for their help in improving the paper.
Ágústsdóttir, A. M. (2015). Ecosystem approach for natural hazard mitigation of volcanic tephra in Iceland: building resilience and sustainability. Nat. Hazards 78, 1669–1691. doi: 10.1007/s11069-015-1795-6
Baerbel, L., Arnau, F., Martin, H., and Volker, M. (2012). Volcanic ash over Europe during the eruption of Eyjafjallajökull on Iceland, April–May 2010. Atmos. Environ. 48, 1–8. doi: 10.1016/j.atmosenv.2011.03.054
Barsotti, S., Andronico, D., Neri, A., Del Carlo, P., Baxter, P. J., Aspinall, T., et al. (2010). Quantitative assessment of volcanic ash hazards for health and infrastructure at Mt. Etna (Italy) by numerical simulation. J. Volcanol. Geother. Res. 192, 85–96. doi: 10.1016/j.jvolgeores.2010.02.011
Barsotti, S., Bignami, C., Buongiorno, M. F., Corradini, S., Doumaz, F., Guerrieri, L., et al. (2011). SAFER response to eyjafjallajökull and merapi volcanic eruptions. In Let’s embrace space’Space Research Achievements Under the 7th Framework Programme, ed. European Commission, DG Enterprise and Industry, (Belgium: European Commission, DG Enterprise and Industry)
Barsotti, S., and Neri, A. (2008). The vol-calpuff model for atmospheric ash dispersal: 2. application to the weak mount etna plume of july 2001. J. Geophys. Res. 113:B03209. doi: 10.1029/2006JB004624
Barsotti, S., Neri, A., and Scire, J. S. (2008). The vol-calpuff model for atmospheric ash dispersal: 1. Approach and physical formulation. J. Geophys. Res. 113:B03208. doi: 10.1029/2006JB004623
Baxter, P. (1990). Medical effects of volcanic eruptions. Bull. Volcanol. (1990) 52, 532. doi: 10.1007/BF00301534
Bebbington, M., Cronin, S. J., Chapman, I., and Turnera, M. B. (2008). Quantifying volcanic ash fall hazard to electricity infrastructure. J. Volcanol. Geother. Res. 177:4. doi: 10.1016/j.jvolgeores.2008.07.023
Behnke, S. A., Thomas, R. J., Edens, H. E., Krehbiel, P. R., and Rison, W. (2014). The 2010 eruption of Eyjafjallajökull: lightning and plume charge structure. J. Geophys. Res. Atmos. 119, 833–859. doi: 10.1002/2013JD020781
Bennett, A. J., Odams, P., Edwards, D., and Arason, P. (2010). Monitoring of lightning from the April–May 2010 Eyjafjallajökull volcanic eruption using a very low frequency lightning location network. Environ. Res. Lett. 5:044013. doi: 10.1088/1748-9326/5/4/044013
Biass, S., Scaini, C., Bonadonna, C., Folch, A., Smith, K., and Höskuldsson, A. (2014). A multi-scale risk assessment for tephra fallout and airborne concentration from multiple Icelandic volcanoes – Part 1: hazard assessment. Nat. Hazards Earth Syst. Sci. 14, 2265–2287. doi: 10.5194/nhess-14-2265-2014
Blake, D. M., Wilson, T. M., Cole, J. W., Deligne, N. I., and Lindsay, J. M. (2017). Impact of volcanic ash on road and airfield surface skid resistance. Sustainability 9:1389. doi: 10.3390/su9081389
Blong, R. J. (1996). “Volcanic hazards risk assessment,” in Monitoring and Mitigation of Volcano Hazards, eds R. Scarpa and R. I. Tilling (Berlin: Springer).
Bolić, T., and Sivčev, Ž. (2011). Eruption of Eyjafjallajökull in Iceland: experience of European air traffic management. Transp. Res. Rec. 2214, 136–143. doi: 10.3141/2214-17
Bonadonna, C., Connor, C. B., Houghton, B. F., Connor, L., Byrne, M., Laing, A., et al. (2005). Probabilistic modeling of tephra dispersal: hazard assessment of a multiphase rhyolitic eruption at Tarawera, New Zealand. J. Geophys. Res. 110:B03203. doi: 10.1029/2003JB002896
Bonadonna, C., FolchSusan, A., and Puempel, L. (2011). Future developments in modelling and monitoring of volcanic ash clouds: outcomes from the first IAVCEI-WMO workshop on Ash Dispersal Forecast and Civil Aviation. Bull. Volcanol. 74, 1–10. doi: 10.1007/s00445-011-0508-6
Bonasia, R., Scaini, C., Capra, L., et al. (2014). Long-range hazard assessment of volcanic ash dispersal for a Plinian eruptive scenario at Popocatépetl volcano (Mexico): implications for civil aviation safety. Bull. Volcanol. 76:789. doi: 10.1007/s00445-013-0789-z
Bursik, M. (2001). Effect of wind on the rise height of volcanic plumes. Geophys. Res. Lett. 28, 3621–3624. doi: 10.1029/2001GL013393
Calder, E., Wagner, K., and Ogburn, S. E. (2015). Volcanic Hazard Maps in Global Volcanic Hazards and Risk. Cambridge: Cambridge University Press, 335–342. doi: 10.1017/CBO9781316276273.022
Carlsen, H. K., Hauksdottir, A., Valdimarsdottir, U. A., Gíslason, T., Einarsdottir, G., Runolfsson, H., et al. (2012). Health effects following the Eyjafjallajökull volcanic eruption: a cohort study. BMJ Open 2:e001851. doi: 10.1136/bmjopen-2012-001851
Casadevall, T. J. (1994). The 1989–1990 eruption of redoubt volcano, alaska: impacts on aircraft operations. J. Volcanol. Geother. Res. 62, 301–316. doi: 10.1016/0377-0273(94)90038-8
Cioni, R., Longo, A., Macedonio, G., Santacroce, R., Sbrana, A., Sulpizio, R., et al. (2003). Assessing pyroclastic fall hazard through field data and numerical simulations: example from Vesuvius. J. Geophys. Res. 108:2063. doi: 10.1029/2001JB000642
Costa, A., Dell’Erba, F., Di Vito, M. A., Isaia, R., Macedonio, G., Orsi, G., et al. (2009). Tephra fallout hazard assessment at the Campi Flegrei caldera (Italy). Bull. Volcanol. 71:259. doi: 10.1007/s00445-008-0220-3
Cronin,S. J., Neall, V. E., Lecointre, J. A., Hedley, M. J., and Loganathan, P. (2003). Environmental hazards of fluoride in volcanic ash: a case study from Ruapehu volcano, New Zealand. J. Volcanol. Geother. Res. 121, 271–291. doi: 10.1016/S0377-0273(02)00465-1
Dee, D. P., Uppala, S. M., Simmons, A. J., Berrisford, P., Poli, P., Kobayashi, S., et al. (2011). The ERA-Interim reanalysis: configuration and performance of the data assimilation system. Q. J. R. Meteorol. Soc. 137, 553–597. doi: 10.1002/qj.828
Del Negro, C., Fortuna, L., and Vicari, A. (2005). Modelling lava flows by Cellular Nonlinear Networks (CNN): preliminary results. Nonlinear Process. Geophys. 12, 505–513. doi: 10.5194/npg-12-505-2005
Dufek, J. (2016). The fluid mechanics of pyroclastic density currents. Ann. Rev. Fluid Mech. 48, 459–485. doi: 10.1146/annurev-fluid-122414-034252
Esposti Ongaro, T., Clarke, A. B., Voight, B., Neri, A., and Widiwijayanti, C. (2012). Multiphase flow dynamics of pyroclastic density currents during the May 18, 1980 lateral blast of Mount St. Helens. J. Geophys. Res. Solid Earth 117:B06208. doi: 10.1029/2011JB009081
Esposti Ongaro, T., Neri, A., Todesco, M., Macedonio, G. (2002). Pyroclastic flow hazard assessment at Vesuvius (Italy) by using numerical modeling. II. Analysis of flow variables. Bull. Volcanol. 64, 178–191. doi: 10.1007/s00445-001-0190-1
Favalli, M., Chirico, G. D., and Papale, P. (2009). Lava flow hazard at Nyiragongo volcano, D.R.C. Bull. Volcanol. 71, 363–374. doi: 10.1007/s00445-008-0233-y
Favalli, M., Pareschi, M. T., Neri, A., and Isola, I. (2005). Forecasting lava flow paths by a stochastic approach. Geophys. Res. Lett. 32:L03305. doi: 10.1029/2004GL021718
Felpeto, A., Araña, V., Ortiz, R. et al. (2001). Assessment and Modelling of Lava Flow Hazard on Lanzarote (Canary Islands). Nat. Hazards 23, 247–257. doi: 10.1023/A:1011112330766
Folch, A. (2012). A review of tephra transport and dispersal models: Evolution, current status, and future perspectives. J. Volcanol. Geother. Res. 235–236, 96–115. doi: 10.1016/j.jvolgeores.2012.05.020
Frogner, K. P., Roger, B. H., and Sigurdur, R. G. (2006). A diverse ecosystem response to volcanic aerosols. Chem. Geol. 231, 57–66. doi: 10.1016/j.chemgeo.2005.12.008
Gudmundsson, H. (1998). Holocene Glacier fluctuations and Tephrochronology of the Öræfi district, Iceland. PhD, School of Geosciences, the University of Edinburgh, Edinburgh.
Gudmundsson, M. T., Guðrún, L., Ármann, H., and Ágúst, G. G. (2008). Volcanic hazards in iceland. JÖKULL 58, 251–268.
Gudmundsson, M. T, Thordarson, T., Höskuldsson,Á., Larsen, G., Björnsson, H., Prata, A. J, Oddsson B., Magnússon, E., et al. (2012). Ash generation and distribution from the April-May 2010 eruption of Eyjafjallajökull, Iceland. Sci. Rep. 2:572. doi: 10.1038/srep00572
Gudnason, J., Thordarson, T., Houghton, B., and Larsen, G. (2017). The opening subplinian phase of the Hekla 1991 eruption: properties of the tephra fall deposit. Bull. Volanol. 79:34. doi: 10.1007/s00445-017-1118-8
Gudnason, J., Thordarson, T., Houghton, B. F., and Larsen, G. (2018). The 1845 Hekla eruption: grain-size characteristics of a tephra layer, J. Volcanol. Geother. Res. 350, 33–46. doi: 10.1016/j.jvolgeores.2017.11.025
Guffanti, M., Mayberry, G. C., and Casadevall, T. J. (2009). Volcanic hazards to airports. Nat. Hazards 51, 287–302. doi: 10.1007/s11069-008-9254-2
Haynes, K., Barclay, J., and Pidgeon, N. (2007). Volcanic hazard communication using maps: an evaluation of their effectiveness. Bull. Volcanol. 70:123. doi: 10.1007/s00445-007-0124-7
Horwell, C. J., and Baxter, P. J. (2006). The respiratory health hazards of volcanic ash: a review for volcanic risk mitigation. Bull. Volcanol. 69, 1–24. doi: 10.1007/s00445-006-0052-y
Hurst, T., and Smith, W. (2004). A monte carlo methodology for modelling ashfall hazards. J. Volcanol. Geother. Res. 138:15. doi: 10.1016/j.jvolgeores.2004.08.001
IMO (2018a). IMO and The Icelandic Civile Protection Agency Reassess the Status for Öræfajökull. Available at: http://en.vedur.is/about-imo/news/the-imo-and-the-icelandic-civile-protection-agency-reassess-the-status-for-oraefajokull
IMO (2018b). Status of Öræfajökull Volcano. Available at: http://en.vedur.is/about-imo/news/status-of-oraefajokull-volcano
Janebo, M. H., Thordarson, T., Houghton, B. F., et al. (2016). Dispersal of key subplinian–Plinian tephras from Hekla volcano, Iceland: implications for eruption source parameters. Bull. Volcanol. 78:66. doi: 10.1007/s00445-016-1059-7
Jenkins, S., Barsotti, S., Hincks, T. K., Neri, A., Phillips, J. C., Sparks, R. S. J., et al. (2015). Rapid emergency assessment of ash and gas hazard for future eruptions at Santorini Volcano, Greece. J. Appl. Volcanol. Soc. Volcanoes 20154:16. doi: 10.1186/s13617-015-0033-y
Jenkins, S., Magill, C., McAneney, J., Blong, R. (2012). Regional ash fall hazard I: a probabilistic assessment methodology. Bull. Volcanol. 74:1699. doi: 10.1007/s00445-012-0627-8
Jónsson, P., and Valdimar, K. (2007). Eyðing Bæjar í Öræfasveit í Öræfajökulsgosinu 1362. BS ritgerð við Jarðvísindadeild HÍ, Iceland.
Jørgensen, K. A. (1987). Mineralogy and petrology of alkaline granophyric xenoliths from the Thorsmörk ignimbrite, southern Iceland. Lithos 20, 153–168. doi: 10.1016/0024-4937(87)90004-1
Karlsdóttir S., Gylfason, Á. G., Höskuldsson,Á., Brandsdóttir, B., Ilyinskaya, E., Gudmundsson, M. T., et al. (2012). The 2010 Eyjafjallajökull eruption, Iceland. ICAO report, ISBN 978-9979-9975-4-2
Kavanagh, J. L., Engwell, S. L., and Martin, S. A. (2018). A review of laboratory and numerical modelling in volcanology. Solid Earth 9, 531–571. doi: 10.5194/se-9-531-2018
Larsen, G. (2002). “A brief overview of eruptions from ice-covered and ice-capped volcanic systems in Iceland during the past 11 centuries: frequency, periodicity and implications,” in Volcano-Ice Interactions on Earth and Mars, eds J. L. Smellie and M. G. Chapman (London: Geological Society, Special Publications 202), 81–90.
Larsen, G., and Eiríksson, J. (2008a). Holocene tephra archives and tephrochronology in Iceland: a brief review. Jökull 58, 229–250.
Larsen, G., Dugmore, A., and Newton, A. (1999). Geochemistry of historical-age silicic tephras in Iceland. Holocene 9, 463–471. doi: 10.1191/095968399669624108
Larsen, G., and Eiríksson, J. (2008b). Late Quaternary terrestrial tephrochronology of Iceland—frequency of explosive eruptions,type and of tephra deposits. J. Quat. Sci. 23, 109–120. doi: 10.1002/jqs.1129
Larsen, G, Gudmundsson, M. T., Vogfjörð, K., Ilyinskaya, E., Oddsson, B., Pagneux, E. (2015). “The Öræfajökull volcanic system,” in Catalogue of Icelandic Volcanoes. IMO, UI, CPD-NCIP, eds Ilyinskaya, Larsen, and Gudmundsson (Reykjavík: University of Iceland).
Loughlin, S., Barsotti, S., Bonadonna, C., Calder, E. (2017). “Geophysical risk: volcanic activity,” in Science for Disaster Risk Management: Knowing Better and Losing Less, eds K. Poljanšek, M. Marín Ferrer, T. De Groeve, I. Clark, 3.2. (Luxembourg: EUR 28034 EN, Publications Office of the European Union) doi: 10.2788/688605
Macedonio, A., Costa, A., and Folch, A. (2008). Ash fallout scenarios at Vesuvius: Numerical simulations and implications for hazard assessment. J. Volcanol. Geother. Res. 178, 366–377. doi: 10.1016/j.jvolgeores.2008.08.014
Macedonio, G., and Costa, A. (2012). Brief communication “rain effect on the load of tephra deposits”. Nat. Hazards Earth Syst. Sci. 12:1229. doi: 10.5194/nhess-12-1229-2012
Martin, E., and Sigmarsson, O. (2007). Crustal thermal state and origin of silicic magma in Iceland: the case of Torfajökull, Ljósufjöll and Snæfellsjökull volcanoes. Contrib. Min. Petrol. 153:593. doi: 10.1007/s00410-006-0165-5
Mastin, L. G., Guffanti, M., Servranckx, R., Webley, P., Barsotti, S., Dean, K., et al., (2009). A multidisciplinary effort to assign realistic source parameters to models of volcanic ash-cloud transport and dispersion during eruptions. J. Volcanol. Geotherm. Res. 186, 10–21. doi: 10.1016/j.jvolgeores.2009.01.008
Nave, R., Isaia, R., Vilardo, G., and Barclay J. (2012). Re-assessing volcanic hazard maps for improving volcanic risk communication: application to Stromboli Island, Italy. J. Maps 6, 260–269. doi: 10.4113/jom.2010.1061
NCIP (2017). Emergency Evacuation Plan for Öræfajökull. Available at: https://www.almannavarnir.is/english/english-news/emergency-evacuation-for-oraefajokull/
Newhall, C. G., and Self, S. (1982). The volcanic explosivity index (VEI) an estimate of explosive magnitude for historical volcanism. J. Geophys. Res. 87, 1231–1238. doi: 10.1029/JC087iC02p01231
Nurmawati, A., and Konstantinou, K. I. (2018). Hazard assessment of volcanic ballistic impacts at Mt Chihshin, Tatun Volcano Group, northern Taiwan. Nat. Hazards 92:77. doi: 10.1007/s11069-018-3192-4
Óladóttir, B. A., Larsen, G., and Sigmarsson, O. (2011). Holocene volcanic activity at Grímsvötn, Bárdarbunga and Kverkfjöll subglacial centres beneath Vatnajökull, Iceland. Bull. Volcanol. 73, 1187–1208. doi: 10.1007/s00445-011-0461-4
Pagneux, E., Gudmundsson, M. T., Karlsdóttir, S., and Roberts, M. J. (eds.). (2015). Volcanogenic Floods in Iceland: An Assessment of Hazards and Risks at Öræfajökull and on the Markarfljót outwash Plain. Reykjavík: IMO, IES-UI, NCIP-DCPEM.
Palais, J. M., Taylor, K., Mayewski, P. A, and Grootes, P. (1991). Volcanic Ash from the 1362 A.D. Oræfajokull Eruption (Iceland) in the Greenland Ice Sheet. Earth Science Faculty Scholarship. Orono, ME: University of Maine.
Petersen, G. N. (2010). A short meteorological overview of the Eyjafjallajökull eruption 14 April–23 May 2010. Weather 65, 203–207. doi: 10.1002/wea.634
Pilcher, J., Bradley, R. S., Francus, P., and Andreson, L. (2005). A Holocene tephra record from the Lofoten Islands, Arctic Norway. Boreas 34, 136–156. doi: 10.1111/j.1502-3885.2005.tb01011.x
Prata, A. J., and Tupper, A. (2009). Aviation hazards from volcanoes: the state of the science. Natur. Hazards 51:239. doi: 10.1007/s11069-009-9415-y
Rarik (2010). Yearly Report. Available at: https://rafhladan.is/bitstream/handle/10802/13208/Arsskyrsla_RARIK_2010.pdf?sequence=9
Reichardt, U., Ulfarsson, G. F., and Pétursdóttir, G. (2018). Volcanic ash and aviation: recommendations to improve preparedness for extreme events. Transp. Res. Part A Policy Pract. 113, 101–113. doi: 10.1016/j.tra.2018.03.024
Sandri, L., Tierz, P., Costa, A., and Marzocchi, W. (2018). Probabilistic hazard from pyroclastic density currents in the Neapolitan area (Southern Italy). J. Geophys. Res. Solid Earth 123, 3474–3500. doi: 10.1002/2017JB014890
Scaini, C., Folch, A., and Navarro, M. (2012). Tephra hazard assessment at Concepción Volcano, Nicaragua. J. Volcanol. Geother. Res. 219–220, 41–51 doi: 10.1016/j.jvolgeores.2012.01.007
Scaini, C., Biass, S., Galderisi, A., Bonadonna, C., Folch, A., Smith, K., et al. (2014). A multi-scale risk assessment for tephra fallout and airborne concentration from multiple Icelandic volcanoes – Part 2:Vulnerability and impact. Nat. Hazards Earth Syst. Sci. 14, 2289–2312. doi: 10.5194/nhess-14-2289-2014
Scollo, S., Coltelli, M., Bonadonna, C., and Del Carlo, P. (2013). Tephra hazard assessment at Mt. Etna (Italy). Natur. Hazards Earth Syst. Sci. 13, 3221–3233. doi: 10.5194/nhess-13-3221-2013
Selbekk, R. S., and Tronnes, R. G. (2007). The 1362 AD Öræfajökull eruption, Iceland: petrology and geochemistry of large-homogeneous rhyolite. J. Volcanol. Geother. Res. 160, 42–58. doi: 10.1016/j.jvolgeores.2006.08.005
Sharma, K., Self, S., Blake, S., Thordarson, T., and Larsen, G. (2008). The AD 1362 Öræfajökull eruption, S.E. Iceland: physical volcanology and volatile release. J. Volcanol. Geother. Res. 178, 719–739. doi: 10.1016/j.jvolgeores.2008.08.003
Sigmarsson, O., Maclennan, J., and Carpentier, M. (2008). Geochemistry of igneous rocks in Iceland: a review. JÖKULL 58, 139–160.
Sigmarsson, O., Condomines, M., and Fourcade, S. (1992). Mantle and crustal contribution in the genesis of Recent basalts from off-rift zones in Iceland: constraints from Th, Sr and O isotopes. Earth Planet. Sci. Lett. 110, 149–162. doi: 10.1016/0012-821X(92)90045-W
Sparks, R. S. J., and Aspinall, W. (2013). “Volcanic activity: frontiers and challenges in forecasting, prediction and risk assessment, in The State of the Planet: Frontiers and Challenges in Geophysics, eds Sparks and Hawkesworth, (Hoboken: Blackwell)
Spence, R. J. S., Kelman, I., Baxter, P. J., Zuccaro, G., and Petrazzuoli, S. (2005). Residential building and occupant vulnerability to tephra fall. Natur. Hazards Earth Syst. Sci. 5, 477–494. doi: 10.5194/nhess-5-477-2005
Spinetti, C., Barsotti, S., Neri, A., Buongiorno, M. F., Doumaz, F., and Nannipieri, L. (2013). Investigation of the complex dynamics and structure of the 2010 Eyjafjallajökull volcanic ash cloud using multispectral images and numerical simulations. J. Geophys. Res. Atmos. 118, 4729–4747. doi: 10.1002/jgrd.50328
Stevenson, J., Larsen, G., and Thordarson, T. (2015). Physical volcanology of the prehistoric Hekla 3 and Hekla 4 eruptions, Iceland. EGU General Assembly. Geophys. Res. Abstr. 17:EGU2015–EGU4207.
Tarquini, S., and Favalli, M. (2013), Uncertainties in lava flow hazard maps derived from numerical simulations: the case study of Mount Etna. J. Volcanol. Geother. Res. 260, 90–102. doi: 10.1016/j.jvolgeores.2013.04.017
Thompson, M. A., Lindsay, J. M., and Gaillard, J. (2015). The influence of probabilistic volcanic hazard map properties on hazard communication. J. Appl. Volcanol. 4:6. doi: 10.1186/s13617-015-0023-0
Thorarinsson, S. (1979). “On the damage caused by volcanic eruptions with special reference to tephra and gases,” in Volcanic Activity and Human Geology, eds P. D. Sheets, and D. K. Grayson (New York, NY: Academic press),125–159.
Thordarson, T. (2013). “Hraun,” in Nattúruvá á Íslandi: Eldgos og Jarðskjálftar: Viðlagatrygging Íslands/Háskólaútgáfan, eds J. Sólnes, F. Sigmundsson and B. Bessason (Reykjavík: Háskólaútgáfan), 105–129.
Thordarson, T., and Höskuldsson, Á. (2007). The Eruption of Öræfajökull 1362 and the Destruction of the District Herad, SE Iceland. Shimabara: Cities onVolcanoes 5.
Thordarson, T., and Larsen, G. (2007). Volcanism in Iceland in historical time: volcano types, eruption styles and eruptive history. J. Geodynam. 43, 118–152. doi: 10.1016/j.jog.2006.09.005
Thorvaldsdóttir, S., and Sigbjörnsson, R. (2015). Framing the 2010 Eyjafjallajökull volcanic eruption from a farming-disaster perspective. Nat. Hazards 77:1619. doi: 10.1007/s11069-015-1667-0
Tomlinson, E. L., Thordarson, T., Müller, W., Thirlwall, M., and Menzies, M. A. (2010). Microanalysis of tephra by LA-ICP-MS — Strategies, advantages and limitations assessed using the Thorsmörk ignimbrite (Southern Iceland), Chem. Geol. 279, 73–89. doi: 10.1016/j.chemgeo.2010.09.013
Walker, G. P. L. (1962). Tertiary welded tuffs in Eastern Iceland. Quart. J. Geol. Soc. 118, 275–290. doi: 10.1144/gsjgs.118.1.0275
Wardman, J. B., Wilson, T., Bodger, P. S., Cole, J. W., and Johnston, D. M. (2012), Investigating the electrical conductivity of volcanic ash and its effect on HV power systems. Phys. Chem. Earth 45–46, 128–145. doi: 10.1016/j.pce.2011.09.003
Williams. R. S. (1983). Man Against Volcano: The Eruption on Heimaey. Vestmannaeyjar: United States Department Of The Interior Geological Survey Usgs: INF-75-22. doi: 10.3133/70039211
Keywords: Öræfajökull volcano, Iceland, tephra fallout, 1362 eruption, hazard assessment, numerical model, critical infrastructure
Citation: Barsotti S, Di Rienzo DI, Thordarson T, Björnsson BB and Karlsdóttir S (2018) Assessing Impact to Infrastructures Due to Tephra Fallout From Öræfajökull Volcano (Iceland) by Using a Scenario-Based Approach and a Numerical Model. Front. Earth Sci. 6:196. doi: 10.3389/feart.2018.00196
Received: 02 June 2018; Accepted: 19 October 2018;
Published: 13 November 2018.
Edited by:
Sonia Calvari, Istituto Nazionale di Geofisica e Vulcanologia (INGV), ItalyReviewed by:
Marco Viccaro, Università degli Studi di Catania, ItalyCopyright © 2018 Barsotti, Di Rienzo, Thordarson, Björnsson and Karlsdóttir. This is an open-access article distributed under the terms of the Creative Commons Attribution License (CC BY). The use, distribution or reproduction in other forums is permitted, provided the original author(s) and the copyright owner(s) are credited and that the original publication in this journal is cited, in accordance with accepted academic practice. No use, distribution or reproduction is permitted which does not comply with these terms.
*Correspondence: Sara Barsotti, c2FyYUB2ZWR1ci5pcw==
Disclaimer: All claims expressed in this article are solely those of the authors and do not necessarily represent those of their affiliated organizations, or those of the publisher, the editors and the reviewers. Any product that may be evaluated in this article or claim that may be made by its manufacturer is not guaranteed or endorsed by the publisher.
Research integrity at Frontiers
Learn more about the work of our research integrity team to safeguard the quality of each article we publish.