- Department of Earth and Environmental Science, University of Pennsylvania, Philadelphia, PA, United States
The emerging view of soil organic matter (SOM) persistence asserts that SOM exists as a continuum of organic material, continuously processed by the decomposer community from large biopolymers to small monomers and with increasing oxidation and solubility, protected from decomposition through mineral aggregation and adsorption. Microbial community and ecosystem dynamics regulate the exchange of both nutrients and carbon between the soil and the atmosphere through the mineralization of SOM. Because these ecosystem dynamics are driven by net energy flows, analysis of SOM bioenergetics can provide complementary constraints to SOM models as well as insight into the fundamental conundrum of why thermodynamically unstable organic matter persists in soil. Microbial substrate preference has been shown to depend on the energy status of the potential substrates in terms of energy required and energy returned. Here we propose a framework for assessing the persistence of SOM utilizing thermally determined activation energy (Ea) and energy density (ED), tested on a suite of soils that have undergone alteration in field or laboratory experiments designed to isolate persistent SOM. Comparison of these energetic parameters in this framework will determine whether a chemical or physical change during SOM decomposition resulted in a change in its environmental persistence. An expanded framework of bioenergetics changes during SOM formation, decomposition, and stabilization is proposed as persistent SOM is characterized by decreased ED and Ea, relative to the bulk SOM.
Introduction
Soil organic matter (SOM) contains more actively cycling carbon than the atmosphere and global terrestrial biomass combined (Jobbágy and Jackson, 2000; Stockmann et al., 2013), and for this reason, even small changes in the size of this carbon pool can have large impacts on the release and storage of atmospheric CO2 and other greenhouse gases (CH4, etc.; Davidson and Janssens, 2006; Bond-Lamberty and Thomson, 2010). Microbial community and ecosystem dynamics regulate the exchange of both nutrients and carbon between the soil and the atmosphere through the mineralization of SOM (Falkowski et al., 2008). These microbial community dynamics are driven by net energy flows, in which soil heterotrophs oxidize organic matter, acquiring energy that was fixed during photosynthesis, and channeling this energy into storage in the form of tissues (i.e., microbial biomass) or other organic materials, or expending this energy in metabolic processes (i.e., respiration; Odum et al., 1962; Currie, 2003). Despite its importance, the mechanisms controlling SOM stability and persistence are still debated (Schmidt et al., 2011). However, the growing consensus is that environmental and biological controls (i.e., microbial community composition, organo-mineral associations, temperature, and moisture) exert a stronger control over SOM stability than molecular structure alone (Schmidt et al., 2011; Lehmann and Kleber, 2015).
Microbial substrate preference has been shown to depend on the energy status of the potential substrates in terms of energy required to metabolize the substrate and energy returned from metabolism of the substrate. In a study of thermophilic microbes, Amenabar et al. (2017) showed that the microbial energy demand (or required energy input from the microbial community) dictated the substrate choice rather than the potential energy supply of the substrates, with microbes preferring easier to decompose substrates over more energy rich substrates. Studying pyrogenic carbon, Harvey et al. (2016) demonstrated that the microbial community exhibited a greater preference for organic matter that returned the most energy for the smallest energy investment, which they expressed as the return-on-energy-investment (ROI) ratio. The energy status of SOM should therefore also be an important factor in its persistence. Barré et al. (2016) demonstrated that for a variety of climates and soil types, persistent SOM showed specific thermally determined energetic signatures, namely, that persistent SOM became less energy dense (yielding less energy during ramped combustion) with increasing duration of minimal C inputs via bare fallow treatment, suggesting that soil microorganisms preferentially mineralize high-energy SOM. In a similar study employing thermal decomposition of SOM, Williams et al. (2018) demonstrated distinct bioenergetic signatures between particulate (faster cycling) SOM vs mineral-associated (slower cycling) SOM. The particulate SOM fractions were characterized by larger energy densities and activation energies, whereas the mineral-associated SOM fractions were characterized by smaller energy densities and activation energies.
Here we propose a framework for assessing the persistence of SOM using thermally determined activation energy and energy density (ED). ED is a measure of the total net bond energy released during ramped combustion and can be used as a proxy for the molecular composition of SOM, such that more condensed or polymerized compounds would have higher ED. ED also represents the amount of available or potential chemical energy the microbial community might thus gain through the complete decomposition of the SOM for growth and respiration. Activation energy (Ea) is a measure of the input energy required to combust SOM and can be used as a proxy for the molecular composition of SOM and as a proxy for the energetic barrier to decomposition, and thus SOM persistence. We will test this framework using several soils that have undergone alteration in field or laboratory experiments designed to isolate persistent SOM: bare fallow and chemical fallow field trials, land-use/land-cover changes, long-term laboratory incubations, and acid hydrolysis. Many fractionation methods intended to isolate persistent SOM are still hampered by methodological problems (Bruun et al., 2008). In several persistent-SOM isolation method comparison studies (Jenkinson, 1971; Balesdent, 1996; Balabane and Plante, 2004; Plante et al., 2005; Krull et al., 2006; Paul et al., 2006; Bruun et al., 2008), the authors found variable or no relationships between the isolated soil fractions and environmental persistence. Likewise, uncertainty in estimates of the size of labile and persistent SOM pools are a major source of error in modeling soil organic C turnover (Falloon and Smith, 2000) and response to climatic and environmental changes.
Because the energy status of SOM is a fundamental factor in its environmental persistence, we expect that comparison of changes in these two energetic parameters (ED and Ea) as discussed in the following bioenergetics framework will allow us to determine if a chemical or physical change during SOM decomposition has resulted in a change in its environmental persistence or biodegradability. We hypothesize that the ED will be lower in persistent SOM compared to the bulk soil as energy dense particulate organic matter (mostly comprised of plant residues) decompose into smaller particles and biomolecules. For Ea, we propose two competing hypotheses that offer the opportunity to test opposing theories of persistent SOM formation. If persistent SOM formation follows the humification theory, Ea is hypothesized to increase as persistent SOM would have been formed by the polymeric condensation of decomposition products into large and stable biopolymers (i.e., humus). If persistent SOM follows the progressive decomposition or selective preservation theories, Ea is hypothesized to decrease as the persistent SOM is comprised of smaller biomolecules.
Materials and Methods
The Bioenergetic Framework
The stability and persistence of SOM is dependent upon the balance of the energetic barriers to decomposition (i.e., physical and chemical protection, availability of electron acceptors, or enzyme production) and the energy available or released to the microbial community upon decomposition for metabolic functions. Comparison of these energies (available energy and energy barrier) leads to four possible scenarios (Figure 1) varying in Ea and ED that have previously been postulated by Rovira et al. (2008) for litter decomposition and are now discussed in relation to SOM. Scenario 1, symbolized by the top-left quadrant (A), represents a substrate with a high energy availability for a low energetic investment. Microbial populations could easily grow on quadrant A substrates and thus SOM turnover would be fastest. Scenario 2, symbolized by the bottom-right quadrant (D), represents the opposite relationship in which the substrate has low energy availability but requires a high energy investment. Microbial populations growing on such a substrate will have low metabolic and growth rates and thus SOM would exhibit the slowest turnover and be considered persistent. Scenarios 3 and 4, represented by quadrants B and C, fall between the extremes of scenarios 1 and 2 (quadrants A and D) and in some cases may be equal in quality and biodegradability in terms of energy input and return. However, because of the greater available energy of quadrant B substrates, it is postulated that substrates in quadrant C are generally less biodegradable and more persistent than substrates in quadrant B.
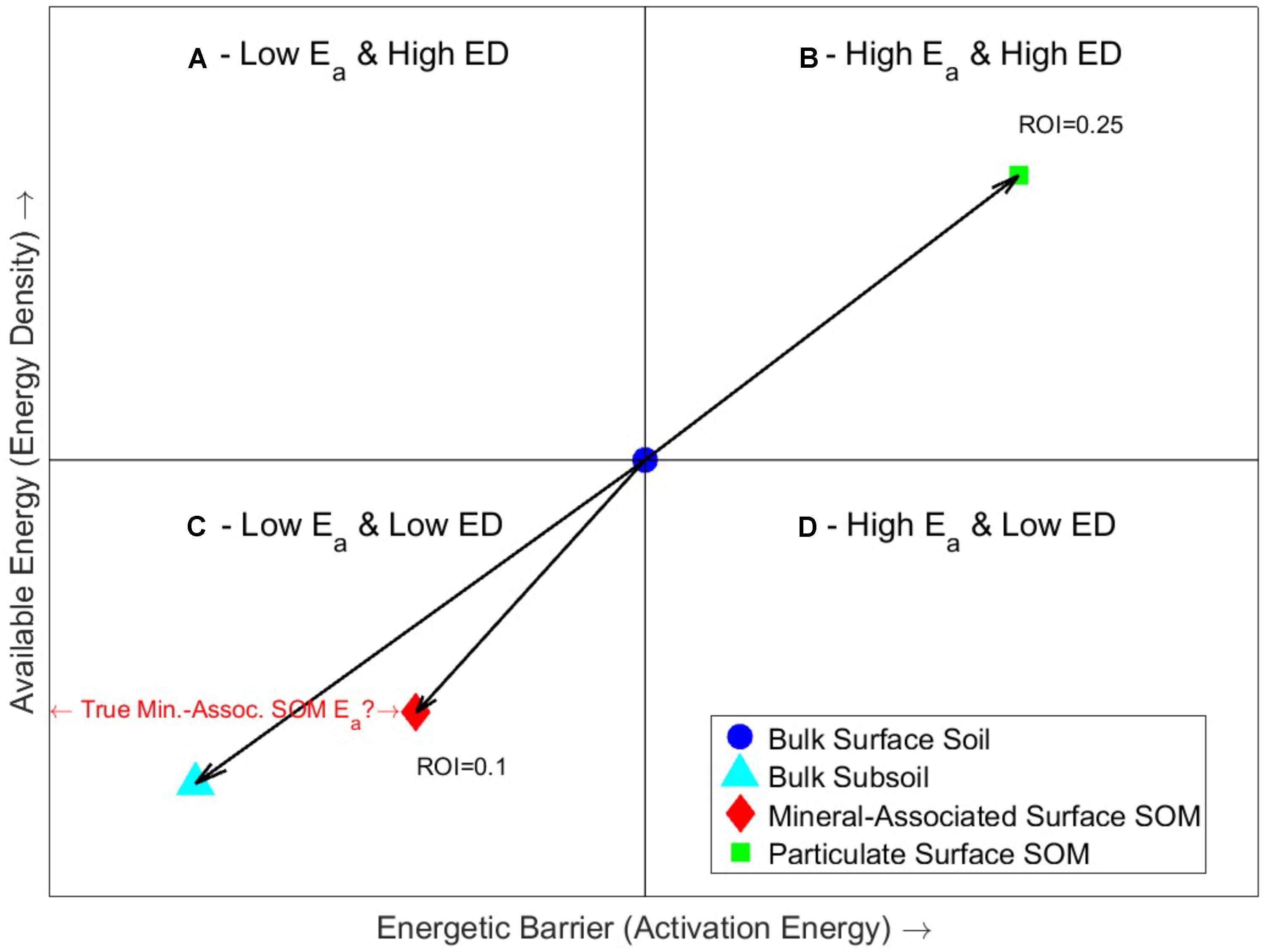
FIGURE 1. The four possible scenarios of the bioenergetics framework, depicted in quadrants A–D (varying in Ea and ED) for SOM decomposition and the formation of persistent SOM. Quadrant A represents a substrate with high energy availability for a low energetic input on which microbial populations could easily grow; SOM turnover is hypothesized to be greatest in this quadrant. Quadrant D represents low energy availability for a high energy input and microbial populations growing on such a substrate will have low metabolic and growth rates; SOM turnover is hypothesized to be slowest in this quadrant. Quadrants B and C represent substrates with either a high/high or low/low energy input/return. We hypothesize that persistent SOM will be characterized by decreasing ED and either decreasing or increasing Ea. Also shown are the results of (Williams et al., 2018) of average changes in bulk soil (n = 16) with depth and fractionation into particulate (faster cycling) and mineral-associated (slower cycling) carbon pools. The centroid represents the starting energy status of the SOM and arrows represent observed trajectories of relatively stable and labile SOM with depth and density fractionation. It is hypothesized that the Ea of the mineral-associated SOM fraction is higher than the organic matter alone because of the presence of and interaction with the soil matrix.
In soils, the interpretation of Ea is complicated by the mineral matrix, which can bind with SOM, forming additional barriers to decomposition reflected in both the thermal and natural decomposition of SOM (Leifeld and von Lützow, 2014). Counterintuitively, Williams et al. (2018) found that organic matter chemically protected by strong mineral bonds required less Ea than particulate organic matter (Figure 1). A similar difference was observed in respiration experiments of mineral and organic soils, in which Ea was lower in mineral soils (Leifeld and von Lützow, 2014). Williams et al. (2018) suggested that while the Ea of the bound-SOM was lower, it is still reflective of the additional barriers to decomposition provided by the mineral matrix, (as evidenced in experimental mixtures of kerogen and common soil minerals; Dembicki, 1994) as well as compositional differences between the particulate and mineral-associated SOM. Williams et al. (2018) also observed that with depth (and increased residence time) in the soil column, there was also a decrease in ED and Ea. Based on these energetic differences observed between mineral-associated and particulate organic matter, we hypothesize that there is a difference in environmental stability and persistence between quadrants B and C, such that organic matter trending toward quadrant C has a greater potential for environmental persistence likely because of increasing mineral association and the preferential loss of energy dense particulate organic matter.
Experimental Soil Sample Sets
Samples for this study were collected from archived soil samples from several previously published field-based and laboratory-based experiments designed to isolate or characterize persistent SOM through the loss or removal of labile SOM (Table 1). Additional details about these soils, experimental conditions, and additional analyses can be found in the following referenced works.
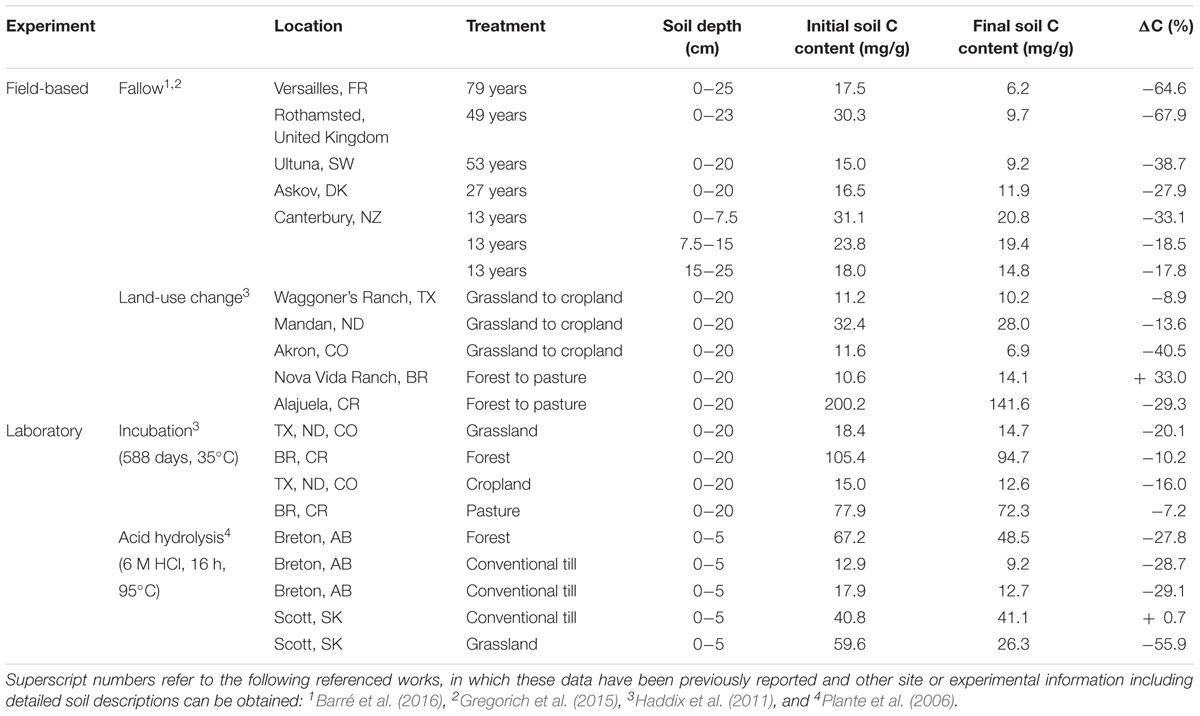
TABLE 1. Location, treatment, depth, soil carbon contents (pre and post), and percent carbon change for the field and laboratory based experiments.
Field Experiments
We used soils from four long-term bare fallow (LTBF) field experiments from northwestern Europe, one chemical fallow field experiment from New Zealand, and five land-use conversion experiments located across a mean annual temperature gradient (Table 1). The LTBF soils used were from bare fallows located at Château de Versailles in Versailles, France, Rothamsted Research in Harpenden, United Kingdom, the Swedish University of Agricultural Sciences at Ultuna in Uppsala, Sweden, and at the Askov Experimental Station, Denmark (Barré et al., 2016). Surface soils (up to 25 cm depth) were collected from two field replicate plots at each location and from triplicate plots in Askov (see Table 1 for soil depths). Bare fallow treatments span 27 years at Askov to 79 years at Versailles (Table 1), and samples were collected in the following years: (Versailles) 1929, 1934, 1939, 1950, 1960, 1968, 1980, 1991, 2002, 2008; (Rothamsted) 1959, 1963, 1971, 1987, 2000, 2008; (Ultuna) 1956, 1967, 1974, 1985, 1995, 2009; (Askov) 1956, 1962, 1968, 1976, 1983. During the duration of the bare fallows, soil organic C concentrations exhibited a relative decrease of 29–65% (Barré et al., 2016).
Chemical bare fallow soils were collected from three field replicate plots from Lincoln, New Zealand. Soils were sampled at three depths (0–7.5, 7.5–15, and 15–25 cm). These soils span 13 years (beginning in 2000) of chemical fallow conditions (Gregorich et al., 2015), resulting in a relative decrease of 33% in soil organic C concentrations in the surface soil and 18% in the two subsoils.
Soils from land-use conversion experiments were collected from five sites along a mean annual temperature gradient (2–25.6°C; Haddix et al., 2011). The temperate sites had paired native grassland and cultivated land uses (Mandan, ND, United States; Akron, CO, United States; and Waggoner Ranch, TX, United States) and the tropical sites had paired native forest and pasture land uses (Alajuela, Costa Rica, and Nova Vida Ranch, Brazil). Transitions from native grassland or forest to cultivated cropland or pasture occurred between 1957 and 1984. Surface soil samples (0–20 cm) were collected from each land-use, resulting in 10 land-use conversion samples (five native + five altered). The land-use conversion resulted in relative decreases in soil organic C concentration (9–40%) due to decades of intensive agriculture. Only the Brazil site experienced a 33% increase in soil C, where an increase in the proportions of carbohydrates and peptides was noted that was not observed in the other locations (Haddix et al., 2011).
Laboratory Experiments
The 10 previously described land-use conversion soils, comprised of three grassland, three cropland, two forests, and two pasture soils, were subjected to a long-term laboratory incubation experiment. Samples were incubated for 588 days at 35°C and optimal, constant moisture conditions to maximize the microbial mineralization of labile SOM. Based on the total respired carbon (Haddix et al., 2011), the long-term incubations resulted in a relative decrease of 7-20% in soil organic C concentrations.
Five surface soil samples (0–5 cm) were collected from two long-term agricultural field experiments in Alberta, Canada and Saskatchewan, Canada (Plante et al., 2006). The two sites had paired either grassland or forest and cultivated (conventional till) land uses. Soils were hydrolyzed with 25 mL of 6 M HCl at 95°C for 16 h, resulting in relative decreases in soil organic C concentration decreases of 28–56%, although one site experienced a 0.7% increase in soil C.
Activation Energy and Energy Density Determinations
Thermal analyses were performed by ramped combustion using a Netzsch STA 449PC Jupiter simultaneous thermal analyzer equipped with an automatic sample carrier (ASC) and a type-S platinum/ rhodium (Pt/PtRh) sample carrier (Netzsch–Gerätebau GmbH, Selb, Germany). Thermal analysis has been demonstrated to be highly reproducible on a variety of soil types (Plante et al., 2009), and thus thermal analyses analytical replicate aliquots were not performed. Samples were weighed to contain approximately 1 mg C and were heated from ambient temperature (25°C) to 105°C at 10°C min-1 under an oxidizing atmosphere of 40 mL min-1 of CO2-free synthetic air (20% O2 and N2 balance). Samples were held at 105°C for 15 min to allow for drying/moisture equilibration, then heated at 10°C min-1 to 800°C. Evolved CO2 was analyzed using a coupled LICOR LI-820 infrared gas analyzer. Differential scanning calorimetry (DSC) heat flux (the exothermic or endothermic energy flux from the sample, referenced to an empty Pt/Rh crucible), thermogravimetric (TG) mass loss, and evolved CO2 gas (CO2-EGA) signals were recorded every second. DSC and CO2 baselines were corrected a posteriori using the base R package and the smooth.spline() function using a spline and linear baseline, respectively (refer to Plante et al., 2011for additional information on baseline corrections). DSC integration was also performed using the base R package and the sintergral() function.
Total exothermic energy content (in mJ) was determined by integrating the DSC heat flux (in mW) over the exothermic region 190–600°C, which represents the temperature range in which SOM is oxidized (Rovira et al., 2008). TG mass loss was determined for the same range. This mass loss is attributable largely to SOM combustion, with minor contributions from dehydration of minerals such as kaolinite. ED (in J mg-1 OM) was thus determined by dividing energy content by TG mass loss (Rovira et al., 2008; Plante et al., 2011).
Activation energy (Ea, in kJ mol-1 CO2) was determined following the methods of Burnham and Braun (1999) and Williams et al. (2014), assuming first-order reaction kinetics during ramped combustion. The fraction of sample C remaining (x) for each time step was calculated from the evolved CO2 data. Each data point over the decomposition temperature range (105–800°C) is then assumed to represent an instantaneous measurement of the rate constant of combustion (k) at each temperature, and Ea is determined from a modified Arrhenius plot of ln| ln(x)| vs 1/T, in which the slope of the regression is equal to -Ea/R where ln| ln(x)| is the natural log of the absolute value of the natural log of x and R is the universal gas constant. Thermally derived values of Ea are expected to be greater than what occurs naturally in soils or during laboratory soil incubations (Leifeld and von Lützow, 2014) because of the complete decomposition of each soil instead of a relatively biochemically labile portion and because of the lack of microbial and fungal enzymes to catalyze the decomposition reactions.
Additionally, the ROI ratio (Harvey et al., 2016) which has been demonstrated to be directly related to the biodegradability of specific types of organic matter and thus environmental persistence was calculated for each soil by dividing ED by Ea.
Statistical Analyses
Differences in ED, Ea, and ROI between pre- and post-treatment soils were analyzed using an independent one-way ANOVA. Statistical analyses were performed for each of the following paired pre- and post-treatment groupings: all soils (n = 38), soils separated by field (n = 23) or lab (n = 15) setting, and soils grouped by individual experiments [bare fallow; n = 2 (×4 experiments), chemical fallow; n = 3 (×3 soil depths), land-use; n = 5, incubation; n = 10, and hydrolysis; n = 5]. All analyses were performed using MATLAB and differences in Ea, ED, and ROI were considered statistically significant when p ≤ 0.05.
Results
The Ea and ED varied widely among the initial soils. Ea ranged from 57 to 69 kJ/mol CO2 and ED ranged from 7 to 15 J/mg OM, reflecting the range of soil types, climates, land uses, vegetation types, and organic matter inputs. The ROI ratios varied between the initial and final soils, spanning 0.15–0.24 in the untreated soils to 0.07–0.33 in the final soils, implying changes in SOM biodegradability over the course of the experiments (Table 2). The changes in ROI were not strongly correlated to changes in carbon (R2 = 0.20, p = 0.04; least squares linear regression). When analyzed collectively, only the Ea was statistically different (decreased) between pre- and post-treatment soils (ED p = 0.20; Ea p = 0.005; ROI p = 0.48).
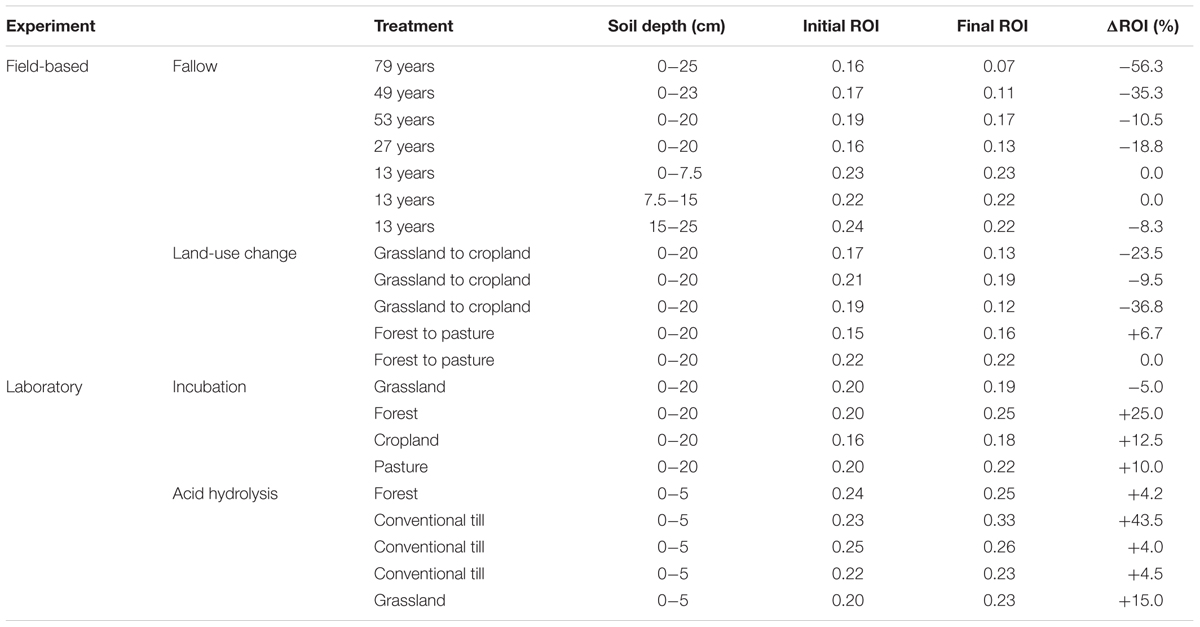
TABLE 2. The changes in the ROI ratio with labile SOM loss or removal from the field and laboratory based experiments.
Field-Based Experiments
In the field-based studies (i.e., bare/chemical fallow and land-use change), post-treatment soils displayed a decrease in Ea and ED (Figure 2) relative to the initial soils during the course of the experiments (ED p < 0.001; Ea p = 0.003), trending toward quadrant C. In the LTBF experiments, the evolution of and variation in ED and Ea with labile SOM loss over time is visible (Figures 2A–D). For the 79 year fallow at Versailles, only the decrease in ED over the course of the experiment was statistically significant (ED p = 0.001; Ea p = 0.08). For the other bare fallows, only the decrease in Ea was statistically significant: Askov (ED p = 0.16; Ea p = 0.03), Rothamsted (ED p = 0.28; Ea p < 0.000), Ultuna (ED p = 0.23; Ea p = 0.003).
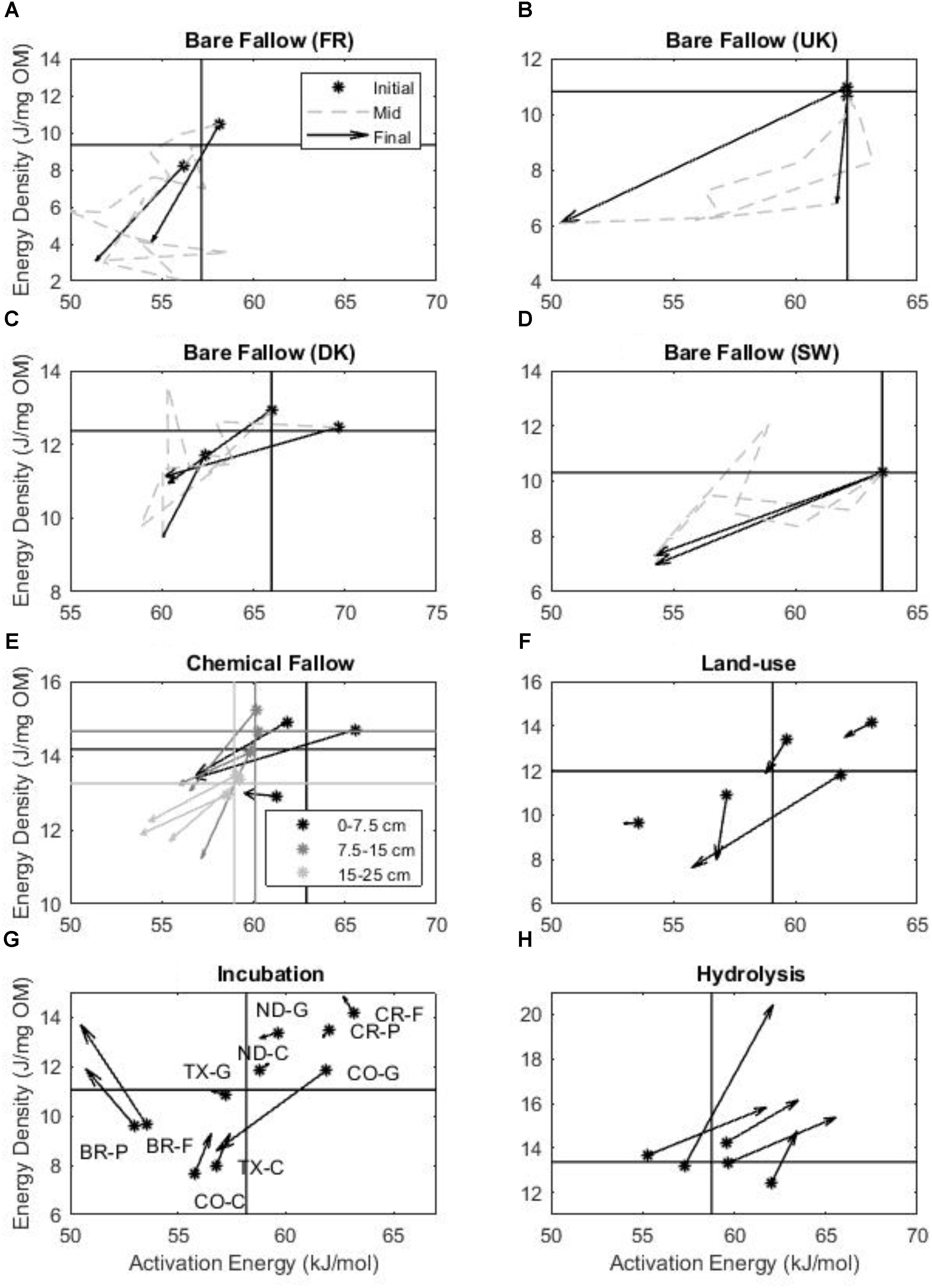
FIGURE 2. The changes in Ea and ED with labile SOM loss or removal from the field and laboratory-based experiments presented in the bioenergetics framework. Changes are indicated by the circles (initial soils) and arrowheads (final soils). For each experiment, the axes cross at the average initial soil Ea and ED to demonstrate the trajectory into one of the four bioenergetics scenarios relative to the initial soil. (A–D) The change in ED and Ea over the course of the long-term bare fallow experiments (A Versailles, FR; B Rothamsted, United Kingdom; C Askov, DK; D Ultuna, SW). (E) The change in ED and Ea after 13 years of chemical fallow for surface soils and soils at depth. (F) The changes in ED and Ea after land-use change from grassland or forest to cultivated cropland or pasture. (G) The change in ED and Ea after laboratory incubation for 588 days at 35°C. Letters indicate the origin and land-use of each soil (G – grassland, P – pasture, C – cropland, F – forest) listed in Table 1. (H) Changes in the ED and Ea of SOM after acid hydrolysis. Please note the different axes scales.
In the one field study considering soil depth (Figure 2E), there was a statistically significant decrease in the Ea with depth and no change in ED (initial ED p = 0.14; initial Ea p = 0.03; final ED p = 0.12; final Ea p = 0.03). At all depths, the 13 year chemical fallow soils demonstrated statistically significant decreases in Ea (0–7.5 cm p = 0.03; 7.5–15 cm p = 0.001; 15–25 cm p = 0.001) over time. However, only the two subsoil samples exhibited significant decreases in ED over the course of the fallow treatment (0–7.5 cm p = 0.25; 7.5–15 cm p = 0.004; 15–25 cm p = 0.04). Neither the changes in ED nor Ea were statistically significant with the changes in land-use (Figure 2F) from forest/grassland to pasture/cropland (ED p = 0.44; Ea p = 0.22).
Although ROIs for the field samples (collectively) decreased after treatment (p = 0.04), the decrease in Ea and ED of the field soils was not always reflected by a decrease in ROI. Only the Rothamsted (p = 0.01), Versailles (p = 0.04), and Ultuna (p = 0.01) bare fallows displayed decreased ROI (and decreased hypothesized biodegradability), while the remaining bare fallow (Askov, p = 0.26), chemical fallow soils (0–7.5 cm p = 0.67; 7.5–15 cm p = 0.17; 15–25 cm p = 0.28), and the land-use soils (p = 0.20) displayed no change in ROI possibly due to proportional changes in ED and Ea.
Laboratory-Based Experiments
In the laboratory-based experiments (i.e., incubation and acid hydrolysis), post-treatment soils displayed no statistically significant changes in Ea and ED (Figures 2G,H) relative to the initial soils (ED p = 0.14; Ea p = 0.58), when analyzed collectively. The long-term laboratory incubation (35°C, 588 days) resulted in highly variable alterations in ED and Ea (Figure 3A), seemingly dependent upon land-use (ED p = 0.53; Ea p = 0.52). Grassland soils displayed decreased ED and Ea (toward quadrant C) following incubation similar to the signature of mineral associated SOM (Williams et al., 2018) and the fallow soils, whereas forest and pasture soils displayed a decrease in Ea and increase in ED (toward quadrant A) similar to the decomposition of forest litter (Rovira et al., 2008), and croplands displayed increased ED and Ea (toward quadrant B) similar to the response of the hydrolyzed soils (Figure 2H) and the energetic signature of the particulate organic matter (Williams et al., 2018) in Figure 1. However, none of the changes in ED and Ea due to long-term incubation were statistically significant when separated by land-use (grassland ED p = 0.22; grassland Ea p = 0.49; forest ED p = 0.84; forest Ea p = 0.42; cropland ED p = 0.63; cropland Ea p = 0.55; pasture ED p = 0.87; pasture Ea p = 0.68). Labile SOM removal by acid hydrolysis (Figure 3B) resulted in statistically significant increases in Ea and ED relative to the untreated soil, trending toward quadrant B (ED p = 0.02; Ea p = 0.01). The net changes in Ea and ED for all experiments are shown in Figure 3.
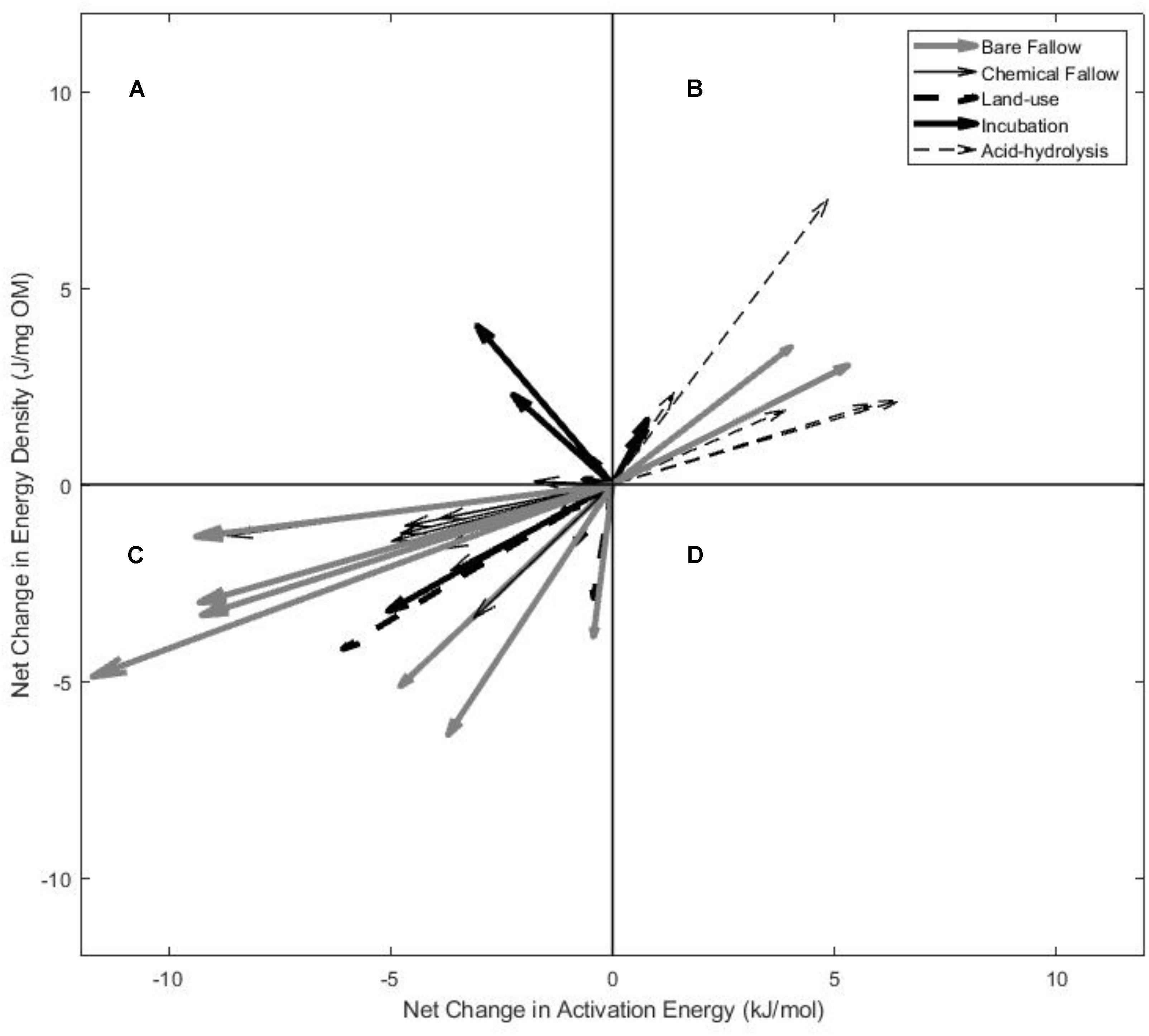
FIGURE 3. Comparison of the net changes in Ea and ED with the removal or loss of labile SOM from the field and laboratory-based experiments. Changes (arrowheads) shown are relative to the initial pre-treatment soils, represented by the origin point (0,0). A–D represent the four scenarios (quadrants) of the bioenergetics framework, as described in Figure 1.
Return-on-energy-investments for the laboratory-based samples (collectively) did not change after treatment (p = 0.14). The ROI also did not change when the lab soils were separated by experiment (incubation; p = 0.29, hydrolysis; p = 0.12), possibly due to proportional changes in ED and Ea. Similar to the lack of pre- and post-treatment differences in ED and Ea with land-use, ROI also did not change (grassland p = 0.64; forest p = 0.21; cropland p = 0.53; pasture p = 0.36) with incubation.
Discussion
The emerging view of SOM persistence asserts that SOM exists as a continuum of organic material, continuously processed by the decomposer community from large biopolymers to small monomers and with increasing oxidation and solubility, protected from decomposition through mineral aggregation and adsorption (Lehmann and Kleber, 2015) and that the persistence of SOM is thus a property of the ecosystem as a whole (Schmidt et al., 2011). Because these ecosystem dynamics are driven by net energy flows, analysis of SOM bioenergetics can provide complementary constraints to SOM models as well as insight into the fundamental conundrum of why thermodynamically unstable organic matter persists in soil.
Long-term bare fallow experiments present the best case for studying the bioenergetic status of persistent SOM (Ruhlmann, 1999). Soils are kept free of vegetation and additional organic inputs for extended periods of time (up to 79 years in the experiments studied here), allowing initially present organic matter to decompose under field conditions and soils to become more enriched in persistent or stable SOM as the more labile SOM decomposes naturally (Barré et al., 2016). Although consisting of different durations and climatic conditions, all fallow experiments (Figures 2A–E) follow the first and third hypotheses of persistent SOM, trending into quadrant C of the bioenergetics framework characterized by decreased ED and decreased Ea. This trajectory is comparable to the observed changes in ED and Ea for bulk soils with depth and fractionation into particulate and mineral-associated SOM (Figure 1). These reductions in ED over time were previously observed (Barré et al., 2016). This trend in the 79 year bare fallow is supported by the observed reduction in energy dense particulate organic matter (Balabane and Plante, 2004) over the course of the experiment, suggesting that organo-mineral complexes (similar to the mineral-associated SOM in Williams et al., 2018) dominate in the latter stages of the fallow and comprise the more persistent SOM. Mineral-associated soil fractions with the longest turnover are assumed to have larger proportions of easily metabolizable organic molecules with low thermodynamic stability (lowest abundance of aromatic groups, highest O-alkyl C/aromatic C ratio, highest proportion of thermally labile materials, and highest ratio of substituted fatty acids to lignin phenols), whereas the younger soil fractions are assumed to have higher proportions of stable organic matter considered more difficult to metabolize, as evidenced by Kleber et al. (2011). This decreased thermodynamic stability is evidenced in the decreased ED or Ea over time, simultaneously the decreased biodegradability is evidenced in the decreasing ROI ratios (Table 2).
Organic matter in subsoils is characterized by longer turnover times compared to surface material (Rumpel and Kögel-Knabner, 2011) and also presents a useful opportunity to investigate SOM biogenetic changes at depth as microbial activity may be reduced by suboptimal environmental conditions, nutrient or energy scarcity, and organic matter may be less accessible because of its association with mineral surfaces (Schmidt et al., 2011). At depth in the chemical fallow experiments (Figure 2B), soils also follow the third hypothesized trajectory of persistent SOM (Figure 1), with deeper soils trending into quadrant C with decreased Ea (as indicated in migrating origin points of the initial soils with depth). However, the ROI ratios (Table 2) do not suggest changes in SOM biodegradability with depth because of the lack of significant change in ED.
Changes in land-use are often disruptive to soil structure, alter natural C inputs, and often result in degradation of biogeochemically labile SOM (Guo and Gifford, 2002). However, when statistically analyzed collectively, changes in land-use in the soils were insufficient in addressing changes in the energy status of SOM, likely due to the continued input of labile organic matter inputs as well as the lack of field/site replicates, and thus there was no true isolation of persistent SOM with the land-use conversions.
Incubations offer a second-best option for studying the bioenergetic status of persistent SOM, as SOM is allowed to decompose in optimal conditions and positive correlations between ED and microbial respiration have been observed in several studies (Plante et al., 2011; Peltre et al., 2013; Stone and Plante, 2015). Although conducted at an elevated temperature, compared to the native MATs, and for almost 2 years to speed the microbial degradation of labile SOM, these incubation experiments were also insufficient to observe differences in SOM Ea and ED. The variable responses in Ea and ED are likely a result of the different land-uses, soil types, climates, and vegetation and the interpretations are limited again by the lack of field/site replicates. Incubations are also limited by the fact that truly persistent SOM is not accessed by the microbes, but rather isolate a large intermediate pool of SOM (Haddix et al., 2011).
In direct contrast to the fallow experiments, the acid-hydrolyzed soils trend toward quadrant B (Figure 3B) following none of the hypothesized trajectories of persistent SOM but rather following the observed trajectories of labile SOM as presented in Figure 1. The use of acid hydrolysis (and other wet chemical oxidation methods such as with hydrogen peroxide) rests on the premise that organic molecules resistant to chemical hydrolysis are also resistant to soil enzymatic attack and are therefore also more biologically stable and persistent in the environment (Krull et al., 2006; Greenfield et al., 2013). While radiocarbon analysis of acid hydrolysis residues has been demonstrated to be older than the bulk soils (Trumbore et al., 1989; Paul et al., 2006), acid hydrolysis is known to preferentially hydrolyze nucleic acids, proteins, and carbohydrates with O-alkyl functional, while leaving alkyl and aromatic compounds (Kiem et al., 2000; Bruun et al., 2008) and has been shown to not equate with resistance (Greenfield et al., 2013). Additionally, in the study of young (40-days-old) and old (40-years-old) carbon mixtures, Bruun et al. (2008) found that acid hydrolysis consistently removed more old carbon, suggesting that the remaining material contained a large portion of faster cycling carbon. Similar to the results of Williams et al. (2018) in which unsaturated/aromatic soil (low H:C) carbon pools, trended toward scenario B, these results are also suggestive of the loss of non-aromatic organic material during acid hydrolysis or artifacts from the conversion of carbohydrates into molecules compositionally indistinguishable from non-hydrolyzable SOM (Greenfield et al., 2013).
The fact that none of the experimental soils trended into quadrant D (Figures 2, 3), or the most energetically unfavorable pool of organic substrates characterized by low ED and high Ea (Hypotheses 1 and 2), supports emerging concepts and models of SOM formation and stabilization away from classic humification theories. Decreased ED is expected with SOM decomposition as larger plant biopolymers are processed into smaller molecules; however, increased Ea is postulated to be the result of mineral association, condensation or polymerization reactions, or the loss of less molecularly complex OM. Mineral association is not expected to significantly affect the Ea of SOM in organic soils, but is expected to increase the Ea of SOM in mineral soils due to additional organic matter-mineral adsorption and complexation bonds which must be broken for SOM decomposition, mineralization or combustion (as conceptualized in Figure 1). However, it is expected that this increased Ea may be relatively small as evidenced in a study of the effects of various mineral matrices (quartz, calcite, dolomite, kaolinite, and bentonite) on kerogen decomposition kinetics in which measured Ea was increased compared to kerogen alone, but only at low organic carbon concentrations (Dembicki, 1994) and by the observation that mineral-associated SOM displays lower Ea than particulate SOM (Williams et al., 2018). However, the presence of carbonates would increase the measured Ea as carbonates decompose into CO2 at relatively high temperatures (∼600–800°C) and would be indiscriminately incorporated into the Ea calculation. Condensation reactions, such as those occurring during the classic soil humification models, suggest the synthesis of large and stable biopolymers and molecules (humus) from decomposition products. However, the lack of evidence for the physical existence of humic substances in situ independent of the alkaline extraction procedure (Schmidt et al., 2011; Lehmann and Kleber, 2015) has led to the acceptance of other models of SOM formation that can be observed in the soil. Additionally, condensation or polymerization reactions occurring during SOM processing would result in an increase in ED as well as an increase in Ea and would likely not appear in quadrant D, but instead in quadrant B. Similarly, the loss of less molecularly complex OM would increase Ea but would also increase ED, as what is postulated to have happened during the acid hydrolysis experiments. Hypothesized substrates in quadrant D were expected to have the slowest turnover and thus be the most environmentally persistent. However, it does not seem plausible within the accepted theories of SOM formation and stabilization for SOM to exist in this hypothetical state, rather the most persistent SOM likely exists in the lower ED extremes of quadrant C (Figure 4) as suggested by the fallow and density fractionation experiments.
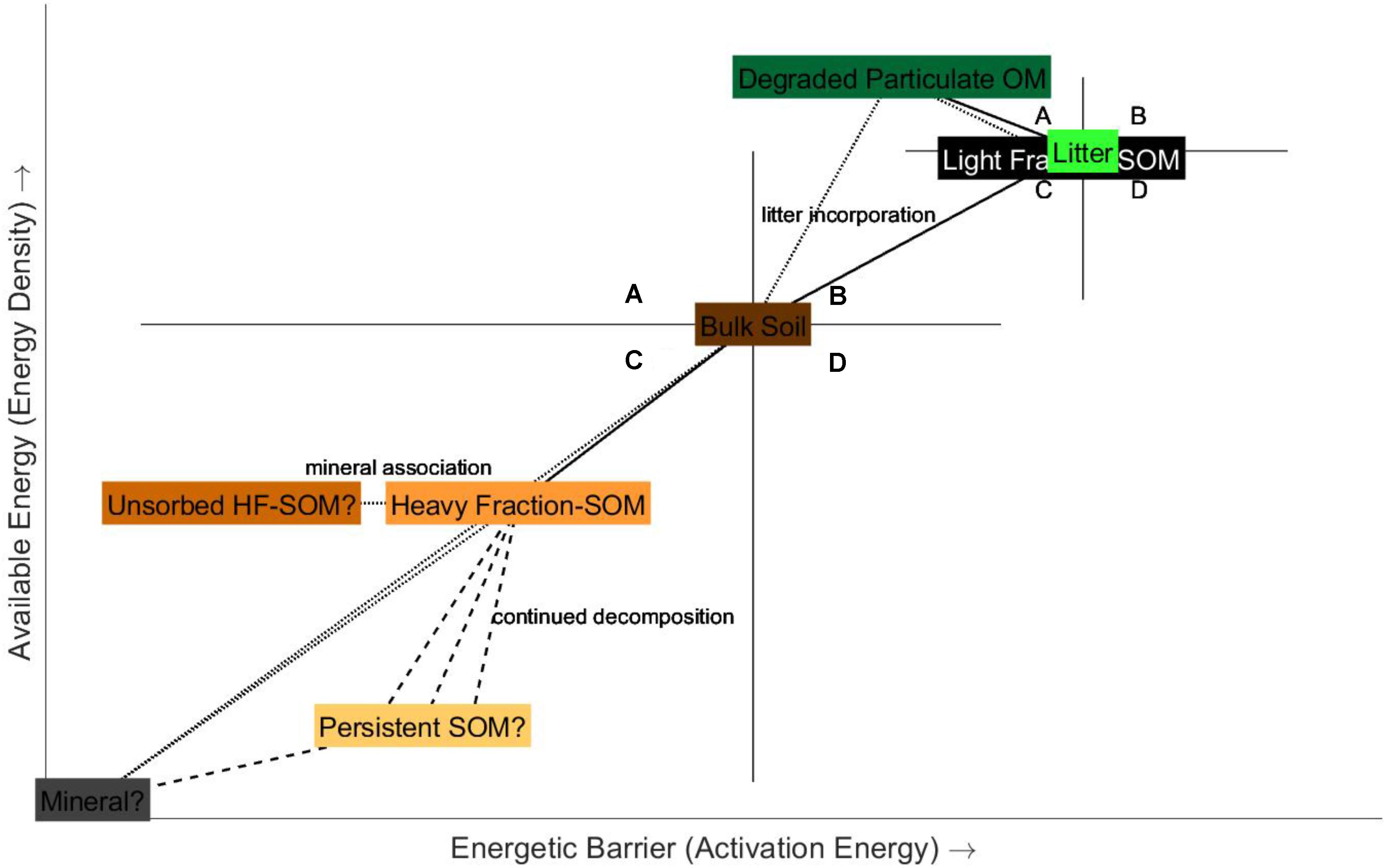
FIGURE 4. Expanded conceptual bioenergetics framework for SOM persistence, based on the observed SOM ED and Ea with depth and fractionation into particulate (LF) and mineral-associated (HF) carbon pools and of litter relative to the bulk surface soil (Williams et al., 2018) and on the observed ED and Ea (modified from DSC-T50) of litter decomposition (Rovira et al., 2008), relative to litter. A–D represent the four scenarios (quadrants) of the bioenergetics framework, as described in Figure 1. Changes are linked by solid lines. Dashed lines represent hypothesized trajectories in ED-Ea space based on the results of this study. Dotted lines represent composition (i.e., the HF-SOM or mineral-associated SOM is comprised of the mineral matrix and the SOM adsorbed to or otherwise interacting with those mineral surfaces). The mineral matrix exists at a hypothetical zero ED and zero Ea.
Soil organic matter substrates characterized by high ED and low Ea (quadrant A) are expected to turnover relatively rapidly within the bioenergetics framework and thus would not become a dominant feature of the soils where persistent SOM dominates over labile SOM such as the post-treatment samples in this study. Only the coupled native forest/pasture incubation soils trended into quadrant A (Figures 2, 3). A trajectory into quadrant A, however, was observed by Rovira et al. (2008) during litter decomposition (Figure 4). Litter decay products were characterized by increased ED and decreased DSC-T50 (the temperature at which half of the total exothermic energy has been released) relative to fresh litter. For all of the soils included in this study as well as the soils, soil density fractions, and litter of Williams et al. (2018), DSC-T50 is positively correlated with Ea (n = 254; R2 = 0.6, p < 0.0001). Thus, Ea is expected to decrease with decreasing DSC-T50 (Ea = 0.25 × DSC-T50 – 27.55) and this relationship also reinforces the usage of DSC-T50 as a measure of SOM decomposability as utilized by Rovira et al. (2008), Plante et al. (2011), Peltre et al. (2013), and Leifeld and von Lützow (2014). The native forest/pasture soils were characterized by the highest carbon contents (Table 1) and it is possible that greater litter inputs into these soils compared to the grassland/cultivated soils caused them to respond to the incubation in the same way as litter, with increasing ED.
In an expanded conceptual bioenergetics framework (Figure 4), we combine the results of this study, the litter decomposition study of Rovira et al. (2008), and the soil density fractionation study of Williams et al. (2018) and summarize proposed bioenergetic changes during SOM formation, decomposition, and stabilization. Litter decomposes into relatively more energy-dense and biodegradable decay products (Rovira et al., 2008), plotting in quadrant A relative to the fresh litter. We expect that pyrogenic organic matter derived from litter would have a higher degree of molecular condensation and thus higher Ea (Harvey et al., 2012). The ED of pyrogenic organic matter would depend on the fire/charring intensity, as Harvey et al. (2016) demonstrated that chars produced at higher temperatures were characterized by lower net energies. However, some litter included in the study by Williams et al. (2018) contained visible pyrogenic organic matter and were from an energetics viewpoint indistinguishable from non-pyrogenic containing litter, thus pyrogenic organic matter is not explicitly depicted in Figure 4. When bulk soil is separated into particulate organic matter (represented by the LF from density fractionation) and mineral-associated organic matter (represented by the HF from density fractionation) pools, particulate SOM is more energy dense and requires greater energy input than bulk SOM or mineral-associated SOM. Particulate SOM is also likely composed to some extent of litter decay products or litter; hence, they are similar in ED and Ea in the framework. Mineral-associated SOM is comprised of the mineral matrix and the adsorbed or chemically complexed SOM, the former increasing the required energy input of the latter due to the additional bonds of SOM sorption onto mineral surfaces. As the bulk soil decomposes, there is a net loss of the more labile particulate SOM, bringing the energetic signature of the bulk soil closer to that of the mineral-associated SOM. The mineral matrix exists at the hypothesized point of zero ED and zero Ea, as it offers no SOM for microbial decomposition and thus would yield no CO2. With continued decomposition, the energetic benefit to cost ratio continues to decrease, resulting in relatively thermodynamically unstable SOM being the most persistent.
Conclusion
By analyzing the energetics of a suite of soils from field and laboratory experiments designed to isolate persistent SOM within the proposed bioenergetics framework, this study has demonstrated that the signature of persistent SOM is that of decreasing available energy supply (ED) and of decreasing required energy input (Ea). This finding contradicts soil formation theories that propose that persistent SOM is formed by the polymeric condensation of decomposition products into large and stable biopolymers (humus), and instead supports theories that persistent SOM is comprised of smaller and smaller particulate SOM fragments and biomolecules, preserved due to interaction with soil minerals. SOM stabilization mechanisms, such as physical and chemical protection, can be viewed as energetic barriers to decomposition such that the stabilized SOM requires additional energies from the microbial community to access. In this framework, we propose that this stabilized SOM is also less biodegradable than labile SOM because of its decreased energy contents. The conclusions of this framework should not be applied to carbonate soils as thermal decomposition of carbonate mineral may result in extraneous CO2 incorporated into the calculation of Ea. The variable or increased ED and Ea exhibited by the laboratory experimental soils suggests that these methods do not always effectively isolate environmentally persistent SOM, but in some cases may target physically or chemically protected SOM.
Author Contributions
EW conceived the conceptual framework, performed the thermal analyses, analyzed the data, and wrote the manuscript with input and contributions from AP.
Funding
Funding to support this project was provided by the University of Pennsylvania Vice Provost for Research – Postdoctoral Fellowship for Academic Diversity and the National Science Foundation (EAR 1541588).
Conflict of Interest Statement
The authors declare that the research was conducted in the absence of any commercial or financial relationships that could be construed as a potential conflict of interest.
Acknowledgments
We thank Pierre Barré and Folk van Oort for supplying samples from the Long-Term Bare Fallow network, Mike Beare and Denis Curtin from Landcare New Zealand for supplying samples from the chemical fallow experiments, and Michelle Haddix and Rich Conant for supplying samples from the land-use conversion/MAT transect.
References
Amenabar, M. J., Shock, E. L., Roden, E. E., Peters, J. W., and Boyd, E. S. (2017). Microbial substrate preference dictated by energy demand rather than supply. Nat. Geosci. 10, 577–581. doi: 10.1038/Ngeo2978
Balabane, M., and Plante, A. F. (2004). Aggregation and carbon storage in silty soil using physical fractionation techniques. Eur. J. Soil Sci. 55, 415–427. doi: 10.1111/j.1351-0754.2004.0608.x
Balesdent, J. (1996). The significance of organic separates to carbon dynamics and its modelling in some cultivated soils. Eur. J. Soil Sci. 47, 485–493. doi: 10.1111/j.1365-2389.1996.tb01848.x
Barré, P., Plante, A. F., Cecillon, L., Lutfalla, S., Baudin, F., Bernard, S., et al. (2016). The energetic and chemical signatures of persistent soil organic matter. Biogeochemistry 130, 1–12. doi: 10.1007/s10533-016-0246-0
Bond-Lamberty, B., and Thomson, A. (2010). Temperature-associated increases in the global soil respiration record. Nature 464, 579–582. doi: 10.1038/nature08930
Bruun, S., Thomsen, I. K., Christensen, B. T., and Jensen, L. S. (2008). In search of stable soil organic carbon fractions: a comparison of methods applied to soils labelled with C-14 for 40 days or 40 years. Eur. J. Soil Sci. 59, 247–256. doi: 10.1111/j.1365-2389.2007.00985.x
Burnham, A. K., and Braun, R. L. (1999). Global kinetic analysis of complex materials. Energy Fuels 13, 1–22. doi: 10.1021/ef9800765
Currie, W. S. (2003). Relationships between carbon turnover and bioavailable energy fluxes in two temperate forest soils. Glob. Change Biol. 9, 919–929. doi: 10.1046/j.1365-2486.2003.00637.x
Davidson, E. A., and Janssens, I. A. (2006). Temperature sensitivity of soil carbon decomposition and feedbacks to climate change. Nature 440, 165–173. doi: 10.1038/nature04514
Dembicki, H. (1994). The effects of the mineral matrix on the determination of kinetic-parameters using modified rock-eval pyrolysis - reply. Organ. Geochem. 21, 982–984.
Falkowski, P. G., Fenchel, T., and Delong, E. F. (2008). The microbial engines that drive Earth’s biogeochemical cycles. Science 320, 1034–1039. doi: 10.1126/science.1153213
Falloon, P. D., and Smith, P. (2000). Modelling refractory soil organic matter. Biol. Fertil. Soils 30, 388–398.
Greenfield, L. G., Gregorich, E. G., van Kessel, C., Baldock, J. A., Beare, M. H., Billings, S. A., et al. (2013). Acid hydrolysis to define a biologically-resistant pool is compromised by carbon loss and transformation. Soil Biol. Biochem. 64, 122–126. doi: 10.1016/j.soilbio.2013.04.009
Gregorich, E. G., Gillespie, A. W., Beare, M. H., Curtin, D., Sanei, H., and Yanni, S. F. (2015). Evaluating biodegradability of soil organic matter by its thermal stability and chemical composition. Soil Biol. Biochem. 91, 182–191. doi: 10.1016/j.soilbio.2015.08.032
Guo, L. B., and Gifford, R. M. (2002). Soil carbon stocks and land use change: a meta analysis. Glob. Change Biol. 8, 345–360. doi: 10.1046/j.1354-1013.2002.00486.x
Haddix, M. L., Plante, A. F., Conant, R. T., Six, J., Steinweg, J. M., Magrini-Bair, K., et al. (2011). The role of soil characteristics on temperature sensitivity of soil organic matter. Soil Sci. Soc. Am. J. 75, 56–68. doi: 10.2136/sssaj2010.0118
Harvey, O. R., Kuo, L. J., Zimmerman, A. R., Louchouarn, P., Amonette, J. E., and Herbert, B. E. (2012). An index-based approach to assessing recalcitrance and soil carbon sequestration potential of engineered black carbons (Biochars). Environ. Sci. Technol. 46, 1415–1421. doi: 10.1021/es2040398
Harvey, O. R., Myers-Pigg, A. N., Kuo, L. J., Singh, B. P., Kuehn, K. A., and Louchouarn, P. (2016). Discrimination in degradability of soil pyrogenic organic matter follows a return-on-energy-investment principle. Environ. Sci. Technol. 50, 8578–8585. doi: 10.1021/acs.est.6b01010
Jenkinson, D. S. (1971). Studies on decomposition of C14 labelled organic matter in soil. Soil Sci. 111, 64–70. doi: 10.1097/00010694-197101000-00008
Jobbágy, E. G., and Jackson, R. B. (2000). The vertical distribution of soil organic carbon and its relation to climate and vegetation. Ecol. Appl. 10, 423–436.
Kiem, R., Knicker, H., Korschens, M., and Kogel-Knabner, I. (2000). Refractory organic carbon in C-depleted arable soils, as studied by C-13 NMR spectroscopy and carbohydrate analysis. Organ. Geochem. 31, 655–668. doi: 10.1016/S0146-6380(00)00047-4
Kleber, M., Nico, P. S., Plante, A. F., Filley, T., Kramer, M., Swanston, C., et al. (2011). Old and stable soil organic matter is not necessarily chemically recalcitrant: implications for modeling concepts and temperature sensitivity. Glob. Change Biol. 17, 1097–1107. doi: 10.1111/j.1365-2486.2010.02278.x
Krull, E. S., Swanston, C. W., Skjemstad, J. O., and McGowan, J. A. (2006). Importance of charcoal in determining the age and chemistry of organic carbon in surface soils. J. Geophys. Res. Biogeosci. 111:G04001.
Lehmann, J., and Kleber, M. (2015). The contentious nature of soil organic matter. Nature 528, 60–68. doi: 10.1038/nature16069
Leifeld, J., and von Lützow, M. (2014). Chemical and microbial activation energies of soil organic matter decomposition. Biol. Fertil. Soils 50, 147–153. doi: 10.1007/s00374-013-0822-6
Odum, E. P., Connell, C. E., and Davenport, L. B. (1962). Population energy-flow of 3 primary consumer components of old-field ecosystems. Ecology 43, 88–96. doi: 10.2307/1932043
Paul, E. A., Morris, S. J., Conant, R. T., and Plante, A. F. (2006). Does the acid hydrolysis-incubation method measure meaningful soil organic carbon pools? Soil Sci. Soc. Am. J. 70, 1023–1035. doi: 10.2136/sssaj2005.0103
Peltre, C., Fernandez, J. M., Craine, J. M., and Plante, A. F. (2013). Relationships between biological and thermal indices of soil organic matter stability differ with soil organic carbon level. Soil Sci. Soc. Am. J. 77, 2020–2028. doi: 10.2136/sssaj2013.02.0081
Plante, A. F., Conant, R. T., Paul, E. A., Paustian, K., and Six, J. (2006). Acid hydrolysis of easily dispersed and microaggregate-derived silt- and clay-sized fractions to isolate resistant soil organic matter. Eur. J. Soil Sci. 57, 456–467. doi: 10.1111/j.1365-2389.2006.00792.x
Plante, A. F., Fernandez, J. M., Haddix, M. L., Steinweg, J. M., and Conant, R. T. (2011). Biological, chemical and thermal indices of soil organic matter stability in four grassland soils. Soil Biol. Biochem. 43, 1051–1058. doi: 10.1016/j.soilbio.2011.01.024
Plante, A. F., Fernandez, J. M., and Leifeld, J. (2009). Application of thermal analysis techniques in soil science. Geoderma 153, 1–10. doi: 10.1016/j.geoderma.2009.08.016
Plante, A. F., Pernes, M., and Chenu, C. (2005). Changes in clay-associated organic matter quality in a C depletion sequence as measured by differential thermal analyses. Geoderma 129, 186–199. doi: 10.1016/j.geoderma.2004.12.043
Rovira, P., Kurz-Besson, C., Couteaux, M. M., and Vallejo, V. R. (2008). Changes in litter properties during decomposition: a study by differential thermogravimetry and scanning calorimetry. Soil Biol. Biochem. 40, 172–185. doi: 10.1016/j.soilbio.2007.07.021
Ruhlmann, J. (1999). A new approach to estimating the pool of stable organic matter in soil using data from long-term field experiments. Plant Soil 213, 149–160. doi: 10.1023/A:1004552016182
Rumpel, C., and Kögel-Knabner, I. (2011). Deep soil organic matter—a key but poorly understood component of terrestrial C cycle. Plant Soil 338, 143–158. doi: 10.1007/s11104-010-0391-5
Schmidt, M. W., Torn, M. S., Abiven, S., Dittmar, T., Guggenberger, G., Janssens, I. A., et al. (2011). Persistence of soil organic matter as an ecosystem property. Nature 478, 49–56. doi: 10.1038/nature10386
Stockmann, U., Adams, M. A., Crawford, J. W., Field, D. J., Henakaarchchi, N., Jenkins, M., et al. (2013). The knowns, known unknowns and unknowns of sequestration of soil organic carbon. Agric. Ecosyst. Environ. 164, 80–99.
Stone, M. M., and Plante, A. F. (2015). Relating the biological stability of soil organic matter to energy availability in deep tropical soil profiles. Soil Biol. Biochem. 89, 162–171. doi: 10.1016/j.soilbio.2015.07.008
Trumbore, S. E., Vogel, J. S., and Southon, J. R. (1989). Ams C-14 measurements of fractionated soil organic-matter - an approach to deciphering the soil carbon-cycle. Radiocarbon 31, 644–654.
Williams, E. K., Fogel, M. L., Berhe, A. A., and Plante, A. F. (2018). Distinct bioenergetic signatures in particulate versus mineral-associated soil organic matter. Geoderma 330, 107–116. doi: 10.1016/j.geoderma.2018.05.024
Keywords: soil organic matter, bioenergetics, thermal analysis, carbon cycling, activation energy
Citation: Williams EK and Plante AF (2018) A Bioenergetic Framework for Assessing Soil Organic Matter Persistence. Front. Earth Sci. 6:143. doi: 10.3389/feart.2018.00143
Received: 22 June 2018; Accepted: 10 September 2018;
Published: 26 September 2018.
Edited by:
Philippe C. Baveye, AgroParisTech Institut des Sciences et Industries du Vivant et de L’environnement, FranceReviewed by:
Carsten W. Mueller, Technische Universität München, GermanyFederico Maggi, University of Sydney, Australia
Copyright © 2018 Williams and Plante. This is an open-access article distributed under the terms of the Creative Commons Attribution License (CC BY). The use, distribution or reproduction in other forums is permitted, provided the original author(s) and the copyright owner(s) are credited and that the original publication in this journal is cited, in accordance with accepted academic practice. No use, distribution or reproduction is permitted which does not comply with these terms.
*Correspondence: Elizabeth K. Williams, d2VsaXpAc2FzLnVwZW5uLmVkdQ==