- 1NERC Radiocarbon Facility, Scottish Universities Environmental Research Center, East Kilbride, United Kingdom
- 2College of Science and Engineering, Centre for Tropical Environmental and Sustainability, ARC Centre of Excellence for Australian Biodiversity and Heritage, James Cook University, Cairns, QLD, Australia
- 3Department of Chemical and Environmental Engineering, Faculty of Engineering, University of Nottingham, Nottingham, United Kingdom
- 4NIAB EMR, Kent, United Kingdom
- 5EPSRC Solid-State NMR Service, Department of Chemistry, Durham University, Durham, United Kingdom
- 6Department of Environmental Engineering, Federal University of Espírito Santo, Vitória, Brazil
- 7Laboratory of Sustainable Water Engineering, Department of Engineering Science, University of Oxford, Oxford, United Kingdom
Pyrogenic carbon (PyC) is a polyaromatic residue of the incomplete combustion of biomass or fossil fuels. There is a growing recognition that PyC forms an important part of carbon budgets, due to production rates of 116–385 Tg C yr, and the size and ubiquity of PyC stocks in global carbon reservoirs. At least a proportion of PyC exists in a highly recalcitrant chemical form, raising the prospect of long-term carbon sequestration through soil amendment with “biochar,” which is generally produced with the aim of making a particularly recalcitrant form of PyC. However, there is growing evidence that some PyC, including biochar, can be both physically and chemically altered and degraded upon exposure to the environment over annual timescales, yet there is a lack of information concerning the mechanisms and determining factors of degradation. Here, we investigate three main factors; production temperature, feedstock composition, and the characteristics of the environment to which the material is exposed (e.g., pH, organic matter composition, oxygen availability) by analysis of biochar samples in a litterbag experiment before and after a year-long field study in the tropical rainforests of northeast Australia. We find that non-lignocellulosic feedstock has lower aromaticity, plus lower O/C and H/C ratios for a given temperature, and consequently lower carbon sequestration potential. The rate at which samples are altered is production temperature-dependant; however even in the highest temperature samples loss of the semi-labile aromatic carbon component is observed over 1 year. The results of 13C-MAS-NMR measurements suggest that direct oxygenation of aromatic structures may be even more important than carboxylation in environmental alteration of biochar (as a subset of PyC). There is a clear effect of depositional environment on biochar alteration even after the relatively short timescale of this study, as changes are most extensive in the most oxygenated material that was exposed on the soil surface. This is most likely the result of mineral ingress and colonization by soil microbiota. Consequently, oxygen availability and physical or chemical protection from sunlight and/or rainwater is vital in determining the alteration trajectory of this material.
Introduction
Pyrogenic carbon (PyC) is the product of incomplete biomass and fossil fuel combustion, forming a continuum from partially charred organic material to soot (Schmidt and Noack, 2000; Bird and Ascough, 2012). PyC is now known to comprise a fraction of every carbon reservoir on Earth (Kuhlbusch, 1998), yet we are only now beginning to understand its role in carbon cycling and carbon budgets (DeLuca and Aplet, 2008; Donato et al., 2009; Santín et al., 2016). The most recent estimate of global PyC production is 116–385 Tg C yr (Santín et al., 2016), equivalent to ~0.2–0.6% of the terrestrial annual net primary production (Huston and Wolverton, 2009). This emphasizes the importance of PyC in the global carbon cycle, yet raises the question of what happens to PyC after it enters a carbon reservoir. Of particular interest is how PyC is altered by environmental exposure, and what determines the trajectory of this alteration.
We have moved away from understanding PyC as chemically homogeneous and inert in the environment. Some PyC does, however, remain undegraded over millennia, making it one of the most recalcitrant forms of organic carbon, with half-lives on the order of several thousand years (Preston and Schmidt, 2006). This raises the prospect that PyC forms an as yet unquantified long-term carbon sink. These features have generated great interest in the prospect of artificially sequestering carbon in soils over extended timescales in the form of engineered charcoal (or “biochar”) (Lehmann et al., 2006).
Biochar is consistent with other forms of PyC in containing a highly polyaromatic fraction, termed stable polyaromatic carbon (SPAC: McBeath et al., 2015), as defined by quantitative methods that work on a chemical basis, e.g., hydropyrolysis (Meredith et al., 2012) where the highly stable fraction is defined as containing 7 or more aromatic rings. This leads to long-term stability in the environment, for example, in a meta-analysis, Wang et al. (2016) found a mean residence time of 556 years for the recalcitrant biochar C pool, and Spokas (2010) predicted a half-life of >1000 years for biochars with an O:C ratio < 0.2. On the other hand, although Santín et al. (2017) have shown that biochar (i.e., anthropogenically engineered) differs from “naturally” produced PyC in having higher carbon sequestration potential for a given production temperature, Bird et al. (2017) have shown biotic, and potentially abiotic degradation of a woody biochar after 3 years exposure to a tropical biome. It is clear, therefore, that at least a proportion of Biochar is subject to environmental alteration over shorter timescales of <1 year (Zimmermann et al., 2012; Bird et al., 2015; Wang et al., 2016), with examples of mineralization on timescales of 1 month (Hilscher et al., 2009). This has major implications for the use of biochar as a tool for carbon sequestration for periods on the order of >100 years, if a proportion of biochar carbon is not “locked up” over these timescales, but is mineralized to CO2. Along with chemical degradation, physical processes such as freeze-thaw, and water-mediated erosion, may impact the structure of PyC in soils through physical breakdown and subsequent translocation (Carcaillet, 2001; Lehmann et al., 2003; Hammes and Schmidt, 2009; Major et al., 2010).
Three main factors are thought to control the alteration of PyC, and hence also biochar: the production temperature, feedstock composition, and the depositional environment (Cheng et al., 2008a; Ascough et al., 2011). Production temperature is positively related to aromatization (Ascough et al., 2008), theoretically making high-temperature biochar/ PyC less susceptible to biotic and abiotic alteration (Bruun et al., 2008; Ascough et al., 2010c). Regarding feedstock as a factor, biochar from non-woody biomass has been reported to be more “degradable” than that made from wood (Hilscher et al., 2009). Finally, alteration of biochar/PyC has been observed to occur more rapidly in highly alkaline environments (Braadbaart et al., 2009) and in warmer, wetter biomes (Cheng et al., 2008b). Oxidative processes have been proposed as the dominant pathway for alteration, and hence oxygen availability is also thought to be important (Bird et al., 2017).
Our knowledge of alteration/stability according to the factors above is far from complete, including the actual mechanics of any alteration that occurs, i.e., how this process progresses from a molecular standpoint. This study aims to fill this knowledge gap by assessing the effect of (i) production temperature, (ii) starting material, and (iii) depositional environment on the chemical composition of different types of biochar. Here, we focus on the labile and semi-labile components of biochar (sensu Bird et al., 2015), with the highly stable polyaromatic carbon (SPAC) component considered in detail in a separate paper. In particular we seek to establish the effect of these variables on the chemical alteration of different forms of biochar. This is achieved by analysis of biochar (i.e., lab-produced charcoal) from four different starting materials and production conditions before and after exposure to the environment in a litterbag experiment at a field site in the tropical Daintree Rainforest, northeast Australia. Samples from one of these starting materials (biochar prepared from wood of Southern Beech (Nothofagus spp.), have previously been analyzed after a 3 year period of exposure, revealing indigenous carbon loss and ingrowth of exogenous carbon over this time (Bird et al., 2017). However, the chemical changes underlying these changes are unknown, along with the factors that drive observed differences between the dynamics of different biochar types in the environment. Here, we seek to address both these issues, studying material exposed to environmental conditions over a timeframe of 1 year, using major sources of biochar that are either proposed for use, or in commercial production.
Given the information available concerning factors influencing biochar alteration in the environment (outlined above), we hypothesize that for all starting materials, biochar produced at higher temperatures will be less affected by alteration during environmental exposure, and that this will be visible in the chemical characterizations we perform. Also that, for a given temperature, alteration will progress in the order of: commercial biochar < woody biomass < non-woody biomass. Finally, that samples exposed to more alkaline conditions will be more altered than that exposed to lower pH.
Methodology
Samples and Field Site
Nothofagus spp. wood (Southern Beech), chemically consistent with modern angiosperm wood in its lignocellulosic structure (Bird et al., 2014), was obtained from the Yallourn coal seam, as described in Tilston et al. (2016). Sugarcane (Saccharum officinarum) bagasse (fibrous matter remaining after crushing of sugarcane) was obtained from Mossman Sugar Mill, Australia, representing a cellulose-rich material. Macroalgae (Cladophora vagabunda) was collected at the Townsville Barramundi Fish Farm in Kelso, Townsville Australia. This sample represents a non-woody material with lower carbon concentration and surface area than wood or bagasse, but high in nitrogen and inorganic nutrients (Bird et al., 2011; McBeath et al., 2015). All three materials are important potential sources of biochar for carbon sequestration and/ or agricultural amendment. Finally, a commercial wood waste biochar, prepared at 550°C, was obtained from BEST Energies Ltd., Australia.
The Nothofagus spp. wood, S. officinarum bagasse and C. vagabunda algae were dried at 105°C, chipped and sieved, with the >2mm fraction retained for pyrolysis, and converted to PyC under a 3.5 L min−1 nitrogen flow for 1 h. Three temperatures of 305°C, 414°C, and 512°C were used, as in Bird et al. (2011). This is the temperature range over which the most significant changes in PyC chemistry are observed (Ascough et al., 2008). Following pyrolysis, samples were lightly crushed and sieved to 0.5–2mm. Together with the BEST biochar, this yielded 10 sample types for field exposure.
Samples were exposed to local environmental conditions using a litterbag approach (see below) for 1 year at the Daintree Rainforest Observatory, Queensland, Australia. The field location is in a UNESCO World Heritage Site at Cape Tribulation, 140 km north of Cairns (16.103°S; 145.447°E; 70 m asl; Figure 1). Mean monthly temperature is 22–28°C (annual mean 25°C), and annual rainfall is 3,500mm, with a pronounced December to March wet season. This site was chosen to maximize the rate of PyC-environment interactions, plus no historical burning is recorded, meaning natural PyC abundance in the soil is very low.
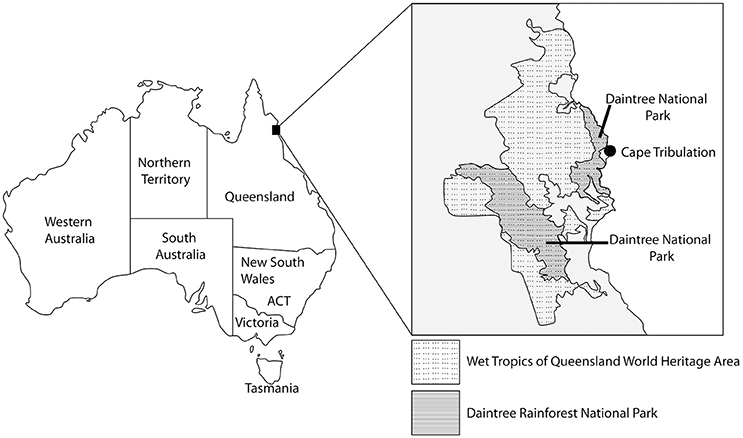
Figure 1. Field site location: Daintree Rainforest National Park at Cape Tribulation, Queensland, Australia.
Vegetation is an evergreen mesophyll to notophyll “tall forest” (Torello-Raventos et al., 2013) producing broadleaf litter to 5–10cm depth in the dry season, which rapidly decays in the wet season. Soils are developed on colluvium from metamorphic and granitic mountains, forming Acidic, Dystrophic, Brown Dermosols (Deckers et al., 1998; Isbell, 2002) or Haplic Cambisols (Hyperdystic, Alumic, Skeletic; Deckers et al., 1998), with a dark grayish brown silty loam to silty clay loam upper A-horizon. The profile contains 20–50% cobbles and stones, and the organic-rich (3.7% C, 0.3% N) soil is mildly acid (pH 5.5–6.5) with the <2mm faction (0–10cm) comprising 28% clay, 54% silt and 19% sand.
For each aliquot c.5 g of dry sample was placed within a 125μm aperture nylon mesh bag, sealed and pegged to the soil surface, which had been cleared of leaf litter. The litterbag approach is well-established in the soil science literature, although potential drawbacks must also be considered, for example partial inaccessibility of litter to decomposers (St John, 1980). Four manipulations (soil chemical environments) were used and samples were duplicated for each of these (i.e., 20 samples per manipulation). The bags were covered with polyester shade cloth and enclosed in a wire mesh cage to exclude foraging wildlife. The treatments comprised:
(i) NL—Samples laid directly on the soil surface without any litter cover;
(ii) L—as for NL but samples covered with a ~5cm layer of local leaf litter;
(iii) NL-LM—as for NL but samples covered with a ~5cm thick layer of limestone chips (sieved at 2–10mm) in order to raise the local pH;
(iv) L-LM—as for NL-LM but the layer of limestone chips was mixed with an equal volume of local leaf litter.
The exact pH at the sample site was not determined, but surface soil and leachate water after 1 year of environmental exposure shows that treatments L and NL had a local pH of 5.6–6.5, and treatments L-LM and NL-LM had a local pH of 6.6–8.0. After recovery samples were gently washed free of loosely adhering soil particles, dried at 105°C and weighed. Both freshly prepared samples and exposed material were analyzed as described below.
Elemental Abundance, Isotopic Analysis and Ash Concentration
Carbon (%C) and nitrogen (%N) concentration was measured using a Costech elemental analyser (EA) (Milan, Italy) fitted with a zero-blank auto-sampler. External reproducibility for %C and %N was better than 0.5%. The stable carbon isotope ratio (expressed as δ13C) of the samples was measured using a ThermoFinnigan Deltaplus XL isotope ratio mass spectrometer (Thermo Finnigan GmbH, Bremen, FRG), linked to the EA via a ConFlo III. Analytical precision was ±0.2‰ for δ13C and ±0.3‰ for δ15N. Oxygen (%O) and hydrogen (%H) concentration was measured using a Thermo Finnigan high temperature conversion elemental analyser (TC/EA) (Thermo Finnigan GmbH, Bremen, FRG). External reproducibility for %O and %H was better than 0.7%. The ash concentration of the samples was determined by loss-on-ignition of 1 g at 900°C in ceramic crucibles in a muffle furnace where reproducibility was better than 1.0%.
PAH Concentration by Hydrogen Pyrolysis and GC-MS
Hydrogen pyrolysis (HyPy; Ascough et al., 2009, 2010b; Meredith et al., 2012) was used to release the < 7 ring polycyclic aromatic hydrocarbons (PAHs, the non-stable PyC fraction), from all samples. Briefly, samples were loaded with an aqueous/methanol solution of ammonium dioxydithiomolybdate [(NH4)2MoO2S2], and pyrolysed by resistive heating to a final temperature of 550°C, all under a hydrogen pressure of 15 MPa and a sweep gas flow of 5 L min−1 (Meredith et al., 2012).
HyPy products were trapped on cooled silica, and desorbed with 10 ml aliquots of n-hexane and dichloromethane (DCM). Eluents were combined and GC–MS analyses in full scan mode (m/z 50–450) were performed on a Varian CP-3800 gas chromatograph equipped with a VF-1MS fused silica capillary column (50m × 0.25mm i.d., 0.25mm thickness) with helium as the carrier gas, interfaced to a Varian 1200 mass spectrometer (EI mode, 70 eV). The abundances of individual PAHs were quantified using the mass chromatograms of the molecular ion of each compound, following the addition of squalane (Aldrich) and 1-1 binapthyl (Acros Organics) as internal standards, assuming a response factor for each compound of 1 (Meredith et al., 2013). The PAHs comprise those that are solvent extractable (“free”) and those covalently bonded to the biochar released by HyPy.
13C-CPMAS NMR Spectroscopy
Solid-state 13C Nuclear Magnetic Resonance Spectroscopy, using cross-polarization magic angle spinning (13C-CPMAS NMR) was used to identify polyaromatic vs. aliphatic and O-alkyl carbons. A 400 MHz Varian VNMRS instrument was used, operating at 100.56 MHz for 13C using a 4 mm magic-angle spinning probe with a zirconium oxide rotor and Teflon end caps. Spectra were referenced to external, neat tetramethylsilane, and for cross polarization, typical acquisition conditions were a 1 s recycle delay, 1 ms contact time and a sample spin-rate of 12 kHz. A variable amplitude 1H spin-lock pulse was used for the cross polarization step. The pulse sequence incorporated a spin-echo to suppress background signal from the Vespel spinner housing; this has resulted in a small amount of signal in some spectra at ~111 ppm.
Change in Carbon Concentration and Sample Mass
Weights of samples and their carbon concentration were accurately determined before and after exposure in the field, making it possible to quantify changes in sample mass and carbon concentration during exposure of the samples to the environment. The proportional mass difference in samples before (MT0) and after exposure (MT1) is obtained by:
The proportional change in carbon concentration (mg C), ash concentration, oxygen, hydrogen, and nitrogen are obtained by substituting values before (XT0) and after exposure (XT1) into Equation (1).
The potential for significant differences between mass and C/O/H/N for samples was assessed after data was scrutinized for normality using a D'Agostino-Pearson normality test. Subsequently, samples were compared using a Two-way ANOVA followed by Tukey's multiple comparisons test, performed using GraphPad Prism version 7.02 for Windows, GraphPad Software, La Jolla California USA, www.graphpad.com. A p-value of < 0.05 indicated a significant difference.
Results
Variations in Sample Mass and Ash Concentration
Full results of measurements for all samples are presented in Supplementary Table S1, and summary data is presented in Table 1. The mass and ash concentration changes following field exposure for Nothofagus spp. wood biochar samples are presented in Bird et al. (2017) (Table 1), but are discussed here in comparison to other samples. Nothofagus wood spp., S. officinarum bagasse, and BEST biochar contained little ash, with most samples below the limit of detection before and after environmental exposure (Table 1). The exception is C. vagabunda algae biochar, which contained 28–38% ash by weight, increasing with production temperature. A large amount (65–83%) of this ash was lost during exposure (Table 2).
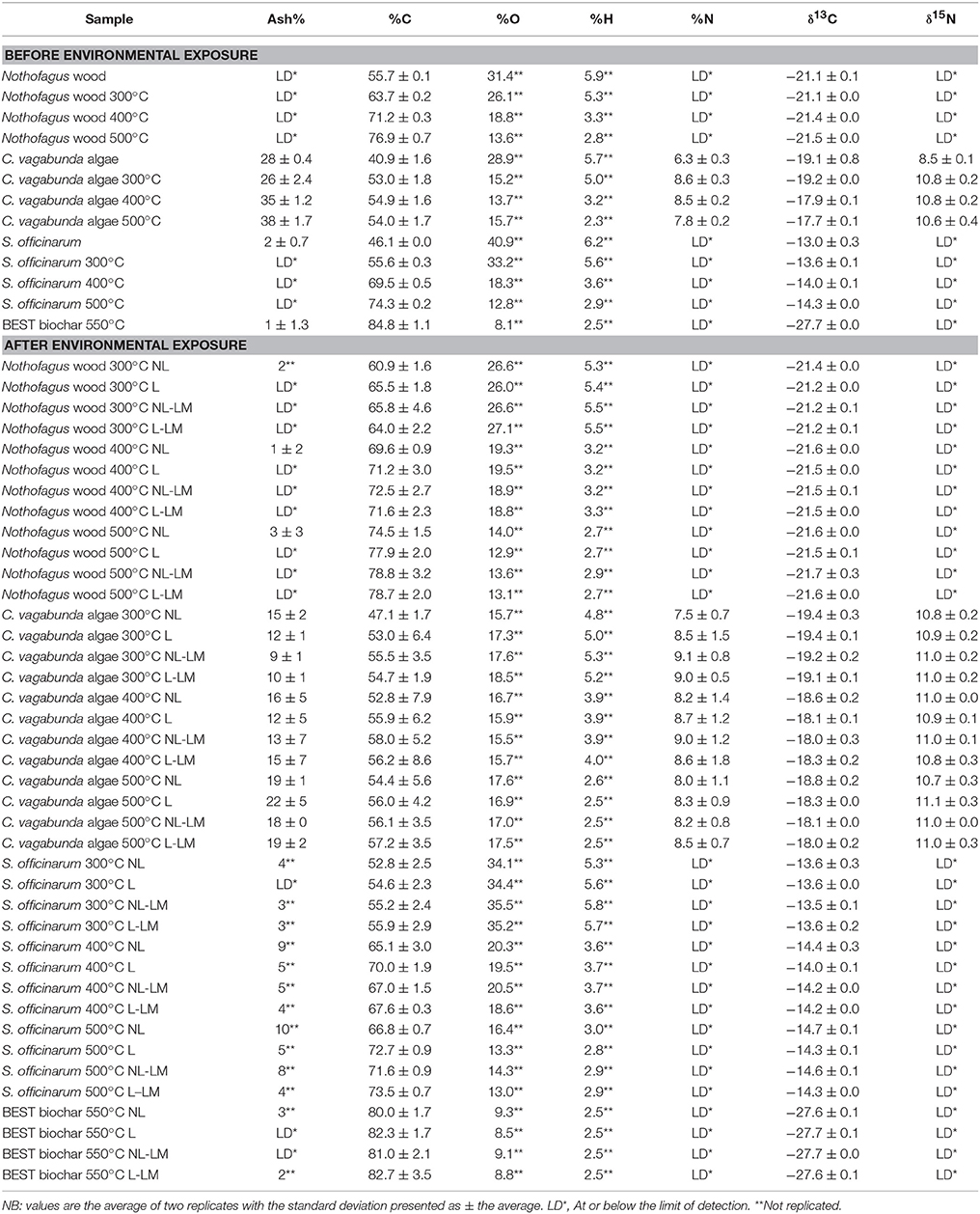
Table 1. Measured ash content, elemental abundance (%C, %O, %H, %N), and stable isotope ratios (δ13C and δ15N) before and after environmental exposure.
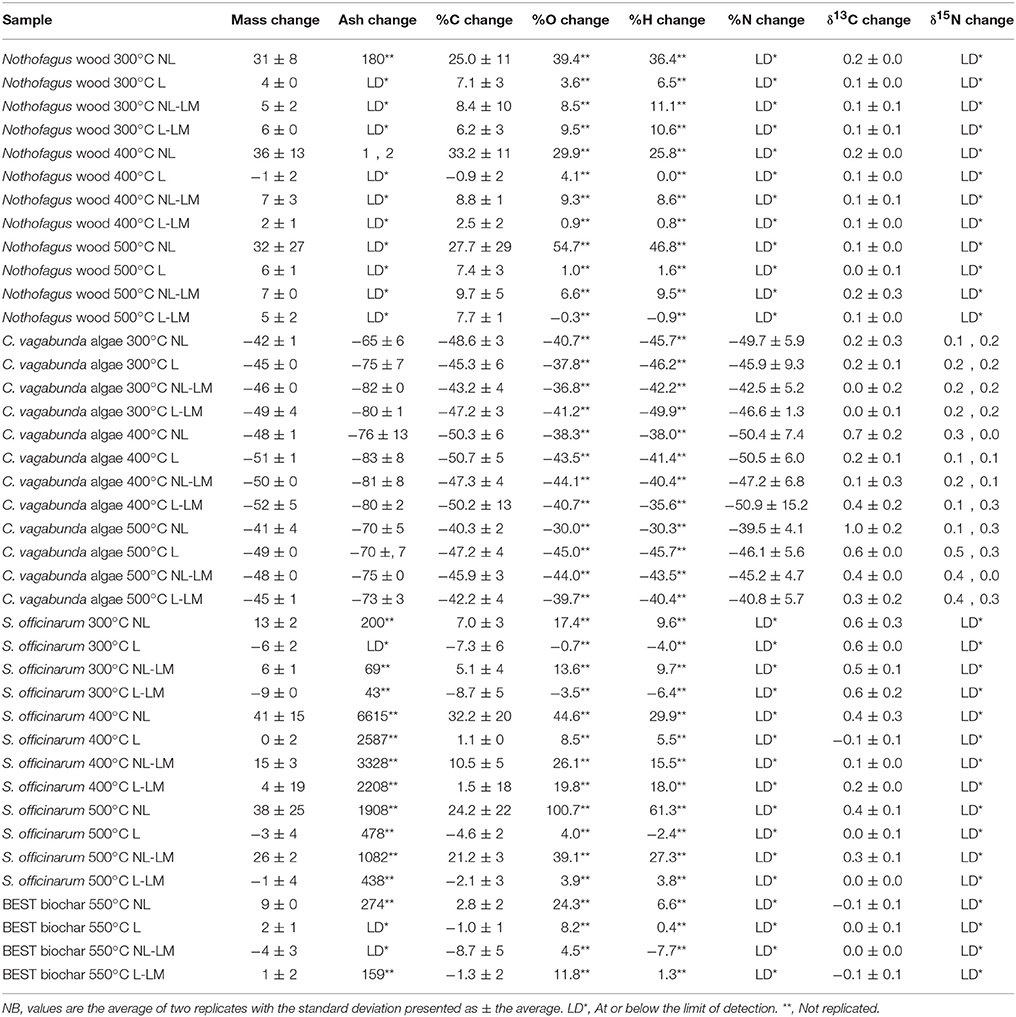
Table 2. The proportional change following environmental exposure in sample mass, ash content, elemental abundance (%C, %O, %H, %N), expressed as % difference from starting values (as reported in Table 1), and the difference in stable isotope ratios (δ13C and δ15N) after environmental exposure, expressed as ‰ deviation.
The mass of BEST biochar changed little with environmental exposure, and (excluding treatment NL), Nothofagus spp. wood mass increased by <10% (Table 2) (NB: isolated reductions in mass of the latter samples are outweighed by the increases). On the other hand, large mass increases of up to +36% occur in treatment NL for Nothofagus spp. wood and S. officinarum bagasse biochar shows changes of −9 to 41% (Table 2). C. vagabunda algae biochar again behaved differently, as its mass decreased by −41 to −52% (Table 2). This was consistently different to both the Nothofagus spp. wood and S. officinarum bagasse biochar (p ≤ 0.005). There were statistically significant differences at 95% confidence between sample types, and treatments. An interaction between sample type and treatment (p ≤ 0.05) was only observed for the 300°C samples, where mass gains in Nothofagus spp. wood biochar were greater than for other materials. At higher temperatures, there was no difference between the overall mass increases for Nothofagus spp. wood and S. officinarum bagasse biochar. C. vagabunda algae biochar showed no significant effect of treatment type at 95% confidence, and there was no clear difference in this between different temperatures, indicating that the mass losses in this sample type were consistent for all samples after 1 year. For the 300°C and 400°C Nothofagus spp. wood and S. officinarum bagasse biochar, treatment NL was associated with higher mass losses than other treatments (p ≤ 0.05). At 500°C this effect was only evident for the S. officinarum bagasse biochar. Overall, C. vagabunda algae biochar showed a very different pattern of change to other sample types, and differences between treatments were most pronounced for samples produced at lower temperatures.
Changes in Carbon, Nitrogen, Oxygen, and Hydrogen Concentration
Full results of measurements for all samples are presented in Supplementary Table S1, and summary data is presented in Table 1. For Nothofagus spp. wood, S. officinarum bagasse, and BEST biochar before environmental exposure, %C, concentrations clearly increased and %O and %H concentrations decreased as production temperature increased (Table 1), consistent with previous work (e.g., Ascough et al., 2008). C. vagabunda algae is different; although O is progressively lost at higher production temperatures, C and H show little change with production temperature after 300°C. Only C. vagabunda contained measureable quantities of nitrogen, which increased with initial charring at 300°C, thereafter remaining unchanged at production temperatures up to 500°C (Table 1).
For samples after environmental exposure, in all treatments except NL (exposed on the surface without litter cover) changes in carbon concentration relative to before exposure ranged from −1 to 33% in Nothofagus spp. wood, most S. officinarum bagasse, and BEST biochar (Table 2). Again, C. vagabunda algae is different; after environmental exposure C concentration decreases markedly, between −40 and −50%. There was no interaction between treatment and material type at any temperature (where p ≥ 0.1 for the possibility of significant difference). There was no significant difference between carbon concentration increases in Nothofagus spp. wood, and S. officinarum bagasse except for treatment NL in 300°C samples (p = 0.05), where C increases in Nothofagus spp., wood, exceeded S. officinarum bagasse. Both were, however, consistently different to C. vagabunda biochar (p ≤ 0.01). For at least some Nothofagus spp., wood, and S. officinarum bagasse samples prepared at 300 and 400°C, treatment NL showed higher C increases than other treatments (p ≤ 0.05). This effect was not apparent in the 500°C samples).
After environmental exposure, oxygen concentration rises in Nothofagus spp., S. officinarum, and BEST biochar, in contrast with large decreases in oxygen in C. vagabunda algae biochar (p ≤ 0.05). There are apparent differences between Nothofagus spp., and S. officinarum biochar prepared at 400 and 500°C, where oxygen concentration increases are greater in S. officinarum biochar (e.g., 55% vs. 100% change in treatment NL). For C. vagabunda algae biochar there is no clear effect of treatment upon sample oxygen content, whereas for Nothofagus spp., and S. officinarum biochar at all temperatures, oxygen increases in treatment NL are greatest. Oxygen increases for samples except C. vagabunda algae biochar appear somewhat larger in samples prepared at higher temperatures, although this correlation could not be tested.
After exposure, changes in hydrogen concentration broadly mirror those observed for %O, where the %H changes in C. vagabunda algae biochar (c.40% loss of starting amount) are significantly different to the overall H gains in other samples (p ≤ 0.001). Changes in other samples are not significantly different from each other at any production temperature, and there is no clear effect of production temperature upon results for any specific material. For C. vagabunda algae biochar H change was not clearly different between treatments, whereas for other samples, increases in H were consistently greater in treatment NL. The net effect of %C, %O, and %H changes is that the pattern in O/C and H/C in all samples (i.e., a decrease with production temperature) is still clearly apparent (Figure 2).
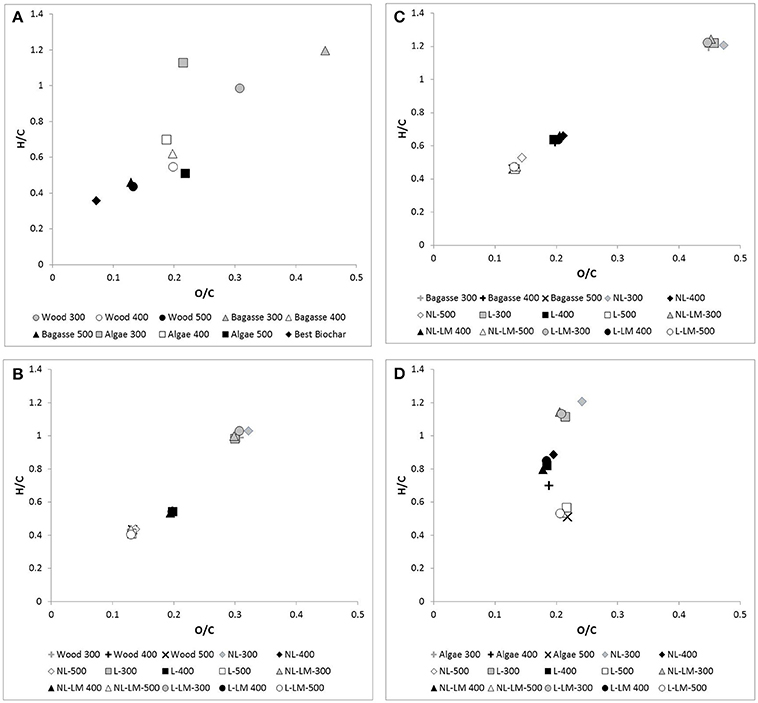
Figure 2. O/C and H/C ratios of samples (Gray symbols, 300°C PyC; White symbols, 400°C samples; Black symbols, 500°C samples). (A) shows samples before environmental exposure: Circles, Nothofagus wood biochar; Triangles, S. officinarum biochar; Squares, C. vagabunda algae biochar; Diamonds, BEST Biochar. (B) (Nothofagus wood biochar), (C) (S. officinarum biochar), and (D) (C. vagabunda algae biochar) show biochar before and after environmental exposure: Crosses, original biochar; Diamonds, treatment NL; Squares, treatment L; Triangles, treatment NL-LM; Circles, treatment L-LM.
There is little difference in %N in C. vagabunda algae biochar following environmental exposure; the majority of measured values do no change by greater than the limit of analytical uncertainty of ±0.5%, and there is no significant difference with production temperature or treatment (p ≥ 0.01 for the possibility of a relationship).
Stable Isotope Measurements (δ13C/ δ15N)
Full results of measurements for all samples are presented in Supplementary Table S1, and summary data is presented in Table 1. Decreases in Nothofagus spp. wood and S. officinarum δ13C during charring are due to loss of cellulose (Ascough et al., 2008). Conversely, C. vagabunda algae shows a small increase in δ13C of ~1‰ above 400°C, this is greater than the analytical uncertainty of ±0.2‰ (Table 2). For the vast majority of samples, δ13C following environmental exposure did not vary in excess of normal analytical uncertainty. The exception is an increase of up to 1.1% in C. vagabunda algae biochar produced at 400°C following exposure. Only C. vagabunda contained sufficient nitrogen to measure δ15N values; these increased with charring to 300°C but were then constant to 500°C. The δ15N of all C. vagabunda algae biochar decreased after environmental exposure by up to 0.5‰; this is greater than the analytical uncertainty of ±0.3‰ with no correlation to production temperature or treatment.
GC-MS of Polycyclic Aromatic Hydrocarbons
The HyPy products consist of the <7 ring polycyclic aromatic hydrocarbons (PAHs) in the samples and comprise both those covalently bound to the biochar and present in the free (solvent extractable) state. The measured concentrations of PAHs in the samples are low overall and probably represent only a fraction of the labile biochar carbon (Table 3). Along with pyrene and phenanthrene a range of compounds known to result from incomplete combustion of biomass are detected (Simoneit, 2002).
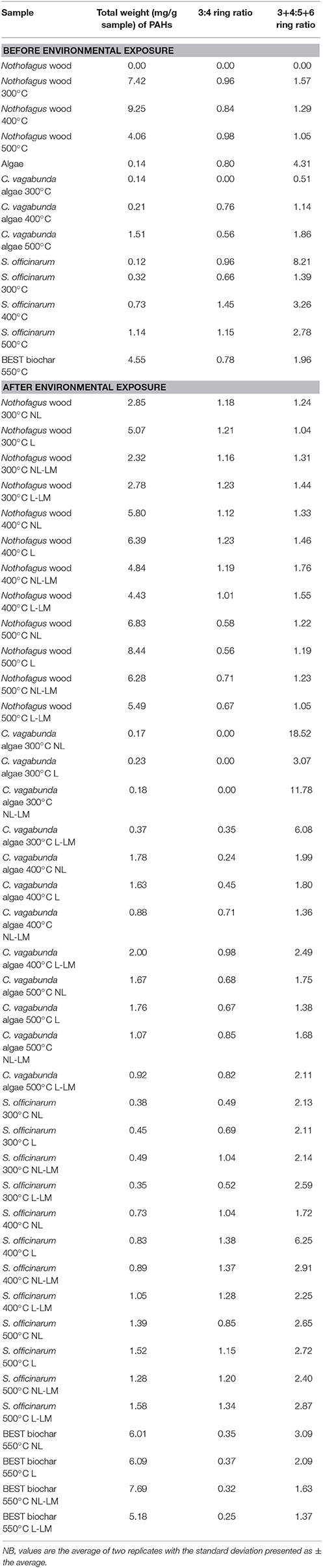
Table 3. The total weight (mg/g sample), 3:4 ring ratio and 3+4:5+6 ring ratio of extractable low molecular weight polycyclic aromatic hydrocarbons before and after environmental exposure.
In freshly-produced Nothofagus spp. biochar, PAH concentration decreased with production temperature (Table 3); this trend was reversed following exposure. Extractable PAHs in Nothofagus spp. PyC are more abundant than in biochar produced from the other starting materials. In fresh S. officinarum bagasse biochar the total weight of PAHs increased with production temperature (Table 3), as does the ratio of 3+4:5+6 ring PAHs. Following exposure there was a slight increase in PAH abundance in all S. officinarum bagasse samples, which was largest in the 300°C S. officinarum. The pattern in ratio of 3+4:5+6 ring PAHs remains the same, although there is an anomalously high ratio (6.25) for 400°C samples in treatment NL.
In fresh C. vagabunda algae biochar PAH concentration also increased with production temperature. One difference from other samples is that naphthalene does not feature in the spectra of C. vagabunda algae samples either before or after environmental exposure. After exposure only the PAH concentration of the 400°C samples increases, particularly pyrene and fluoranthene. In BEST biochar the total content of PAHs is similar to that in Nothofagus spp. samples, although the absolute concentrations remain small. There is no effect of treatment upon PAH concentration.
13C-MAS-NMR
The changes over 300–500°C for lignocellulosic biomass comprise loss of cellulosic carbons at 60–105 ppm, lignin at 55 ppm, and aliphatic carbon (at 10–40 ppm), and increasing dominance of aromatic carbons at c. 129 ppm (Figure 3). In S. officinarum bagasse the process of aromatization is slower than in Nothofagus spp. wood, with peaks for lignin and aliphatic carbons at 400°C. Although C. vagabunda algae is substantially different to the other feedstocks (e.g., higher lipid concentration at 173 ppm), this sample follows the overall charring pattern above, and is dominantly aromatic at 500°C.
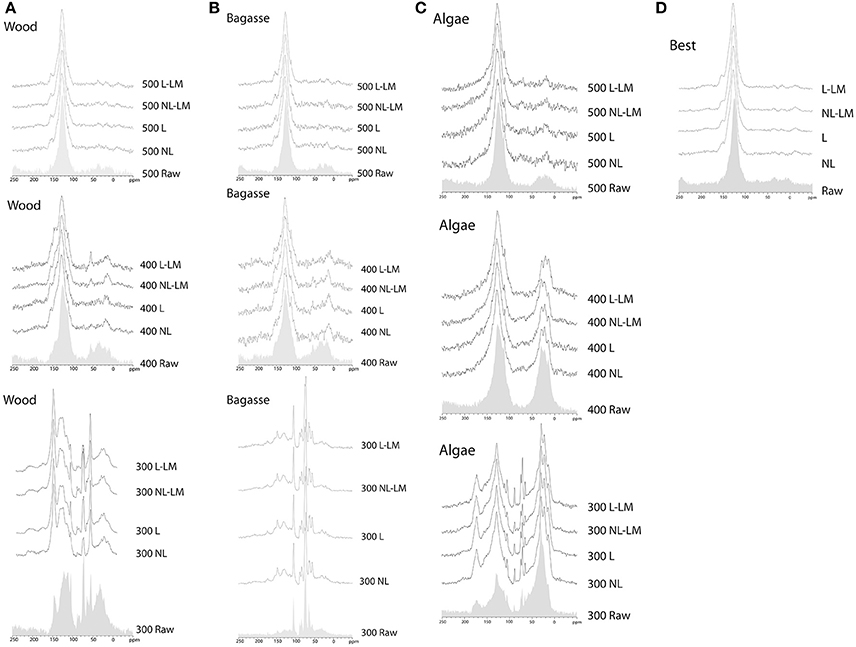
Figure 3. 13C-MAS-NMR spectra of samples before and after exposure to environmental conditions. (A) Nothofagus wood biochar, (B) S. officinarum biochar, (C) Algae biochar, (D) BEST biochar. Spectra are given for uncharred (raw) material and samples prepared at 300°C, 400°C and 500°C emplaced in the four different treatments described in the text.
After environmental exposure there are no clear differences attributable to treatment. Instead, production temperature has the biggest effect upon chemical changes. For samples produced at 300°C, cellulosic carbons are removed in preference to lignin, and many samples are oxidized, with peaks for carboxylic carbons at c.170 ppm, and signal at 150 ppm for oxygenated aromatics. Aromatic carbons increasingly dominate the spectra. Aliphatic signal is reduced, demonstrating the reduction in proportion of aliphatic carbons in the sample, relative to starting material. Remaining aliphatic carbons may be lipids, which are known to resist oxidation (Knicker, 2010). The aromatic peak in samples shifts closer to 130 ppm and narrows after exposure, meaning that the range of different forms of polyaromatic domains is reduced, possibly due to loss of smaller aromatics (Figure 3).
Discussion
Biochar Chemistry Depends Upon both Production Temperature and Feedstock
Production temperature is known to dictate the aromaticity of biochar/PyC, and determine the extent to which non-aromatic material (including oxygen and hydrogen) is removed from the sample (e.g., Ascough et al., 2008; Krull et al., 2009). A finding from this research, however, is that lignocellulosic and non-lignocellulosic biomasses have very different trajectories of chemical change during pyrolysis. In lignocellulosic biomass there is basically a linear relationship between temperature and carbon increase/ oxygen and hydrogen decrease, and aromaticity. At temperatures of c.500°C, samples become increasingly homogeneous. This is likely to have the effect that trajectories of environmental alteration for a specific environment are similar for different types lignocellulosic biomass (where the starting composition is similar) produced at a consistent temperature. This is borne out by the results for Nothofagus spp. and BEST wood biochars presented below. A difference is that the rate of aromatization in S. officinarum bagasse samples appears slower at lower temperatures than in Nothofagus spp. wood biochar or BEST Biochar. We posit that this is the basis for the different patterns of environmental alteration we observe for these samples.
During production of C. vagabunda algae biochar there appears to be a chemical factor that limits aromatization over the 300–500°C window. This could be the effect of chemical reactions involving the high ash concentration during pyrolysis (McBeath et al., 2015), meaning that the potential for environmental alteration of C. vagabunda algae biochar (i.e., non-lignocellulosic material) is greater, compared to lignocellulosic biomass, such as wood, for a given temperature. This is consistent with observations that non-woody biochar is less stable than plant-based material produced at an identical temperature (e.g., Singh et al., 2012). It is also important to consider that there may be physical differences between these types of materials that affect their survival potential in the environment, such as porosity, and micro/ macroscopic cracking. However, the physical structure of the biochars was not assessed in this study, so in the following discussion we focus on chemical factors.
The process of PyC production forms PAHs (e.g., Ballentine et al., 1996; Keiluweit et al., 2012), thought to form a proportion of the “semi-labile” fraction in PyC (McBeath et al., 2015). In Nothofagus spp. wood the highest temperature biochar has the lowest concentration of PAHs, indicating more extensive aromatization, which would raise the carbon content of the biochar, as observed in the results presented here. This would mean that the potential for environmental alteration is lower for a woody biomass such as the Nothofagus spp. biochar sample produced at the same temperature as S. officinarum bagasse and C. vagabunda algae samples, which display lower C contents The potential for alteration/ degradation appears linked to degree of aromaticity (Harvey et al., 2012) and O:C ratio (Spokas, 2010), meaning for the samples analyzed here, predicted alteration potential increases in the order BEST biochar< Nothofagus spp. Wood< S. officinarum bagasse< C. vagabunda algae. These predictions are confirmed by the results observed after environmental exposure of the samples.
Characteristics of Environmental Alteration: Effects of Production Temperature
The results of the study, when considered in total, were consistent with the research hypothesis that material prepared at higher temperatures is less subject to alteration in the environment, supporting previous work (e.g., Zimmerman, 2010). For example, mass losses only displayed an interaction between sample type and treatment (p ≤ 0.05) for samples prepared at 300°C, and treatment effects for both mass losses and changes in C concentration were stronger at lower temperatures. However, the effects we observed were relatively subtle, although also manifest in the 13C-MAS-NMR, and PAH results, as explained below. We posit that these effects are predominantly due to a decrease in less labile carbon vs. resistant polyaromatic domains in higher-temperature biochars. Despite this, some small changes in the 13C-MAS-NMR spectra of even samples produced at 500°C are observed, as the aromatic peak at c.130 ppm was slightly narrowed for all feedstock types and treatments. It is possible this reflects loss of a small proportion of the range of aromatic domains present in the samples before environmental exposure, most probably a fraction of the semi-labile component (sensu Bird et al., 2015). This is supported by the results of PAH measurement, where the greatest reduction in PAH concentration is seen for the lowest temperature biochar. The mechanism by which this could be achieved remains unclear, although growing evidence demonstrates microbial action resulting in degradation of indigenous biochar carbon over timescales on the order of a year (Ameloot et al., 2013; Farrell et al., 2013; Tilston et al., 2016; Bird et al., 2017). Increasing aromatization also increases resistance to oxidative processes, known to be one of the main agents of PyC alteration and degradation. This is shown in the O/C ratios where despite the loss of many oxygen-containing O-alkyl structures, this is more than offset by the additional oxygen introduced to the structure of lower temperature (300–400°C) biochars after 1 year.
Characteristics of Environmental Alteration: Effect of Feedstock
Differences in biochar chemistry after environmental exposure attributed to specific feedstock are relatively low between Nothofagus spp. wood PyC, and S. officinarum bagasse biochar, and although changes appear somewhat smaller in BEST Biochar, for all these sample types, changes in mass, C/O/H/N, and other characteristics are in the same direction. Changes in C. vagabunda algae biochar for these parameters are always in the opposite direction, however, and are consistently larger than those in other samples. The results therefore support the hypothesis that, although different types of woody biomass show relatively small differences in change with environmental exposure, at least at a gross scale, non-woody biomass is more susceptible to alteration than woody biomass (c.f. Hilscher et al., 2009; McBeath et al., 2014). This appears largely due to the removal of ash that is readily solubilised and/or physically migrated away from the sample. This ash contains essential plant nutrients (e.g., Na, Ca, Cl, Mg, K), and so this type of biochar may have a beneficial effect for soil fertility (e.g., Novak et al., 2009), although this will be over a restricted timescale. This type of biochar contains more labile carbon than the other samples, particularly aliphatic material indigenous to the sample and sorbed to its structure (Middelburg et al., 1997), and the C concentration decrease observed in the samples, potentially due to loss of labile C, is so large that any introduction of exogenous carbon, such as that observed in other samples (e.g., via soil microorganisms c.f. Steinbeiss et al., 2009; Bird et al., 2017), is not visible. It should be noted that along with complete degradation to CO2, there is the possibility that some C is removed by solubilization and leaching during environmental exposure (Major et al., 2010; Abiven et al., 2011; Bird et al., 2017).
C. vagabunda algae biochar is the only sample in which δ15N and %N can be observed, although there is no effect of treatment or production temperature. There is a slight increase in δ15N overall, although this is very close to the limit of analytical precision. This may indicate that microbial action has removed lighter N isotopes via fractionation during decomposition (bonds involving 14N being broken more easily), possibly through decomposition of “black nitrogen” (Knicker, 2010; McBeath et al., 2015).
Characteristics of Environmental Alteration: Effect of Depositional Environment
There is a clear effect of depositional environment on biochar alteration even after the relatively short timescale of this study. However, this is not consistent with the hypothesis that material in alkaline environments is more subject to alteration. Although for C. vagabunda algae biochar there is no effect from different treatments upon chemical changes, for other samples, increases in mass, and C/O/H concentrations are far more extensive in the material under the NL treatment. Mass increases due to ash and carbon increases are much greater in all samples under this treatment; the mass increase indicates the potential for a significant proportion of soil mineral ingress (c.f. Bird et al., 2008). Over time in the environment, it is evident that mineral material can strongly associate with both external and internal surfaces of PyC (Lehmann et al., 2011; Jaafar et al., 2015). This can be the result of abiotic processes such as water movement and biotic processes such as the movement of soil macrofauna. This interpretation is further supported by the increases in ash content in many samples, a phenomenon previously observed by Bird et al. (2014). An increase in sample carbon could result from removal of non-carbon elements (e.g., H), due to consumption by microbes. This labile carbon removal has been observed in incubations of the Nothofagus spp. wood charcoal samples (Bird et al., 2017). Conversely, increases in sample carbon concentration and mass could result from the ingress of exogenous carbon, e.g., fungi, soil biota, humic acids (Steinbeiss et al., 2009; Lehmann et al., 2011). For example, Mohan et al. (2014), and others demonstrate the sorption of organic compounds from the depositional environment onto the structure of PyC. Fungal ingress to samples equivalent to some of the material in this study has observational support in field (Bird et al., 2014), and laboratory (Ascough et al., 2010c) studies. The concept of an increase in mass due to introduction of exogenous carbon for the NL treatments is supported by the findings of Bird et al. (2017), using equivalent material to the Nothofagus spp. biochar described in the present study. Here, an increase in radiocarbon concentration of ~0.5–1 pMC clearly demonstrates the addition of exogenous carbon to the radiocarbon-dead biochar. Using δ13C measurements, the same study indicated a 5–10% contribution of exogenous carbon to the lower temperature biochars. This is also found in our results, where a decrease in δ13C occurs overall, albeit to a lower extent than observed for the sample group analyzed by Bird et al. (2017) after 3 years. In this case, the larger %C increases observed in NL may be due to the higher oxygen availability for these samples on the soil surface, leading to unhindered access for microbiota, leading to decomposition of the more labile fraction of the biochar structure (containing non-C elements), raising the %C content as polyaromatic material, dominated by C remains behind. This use of biochar carbon by microbiota is consistent with the findings of Bird et al. (2017) for biochars inoculated with microbial material after drying following environmental exposure. Respiration of CO2 from these samples was linked to both use of biochar carbon and some of the exogenous carbon from the deposition environment, using δ13C measurements. This would explain why mass and %C increases on the same scale are not observed in the NL-LM treatment, even though this was also on the soil surface without litter. Here the limestone covering could physically (through reduced oxygen availability), or chemically (through increased pH) retard the ingress of microorganisms.
The ingress of exogenous carbon is supported by the δ13C measurements, as the sample materials all have higher δ13C values, by up to 15.4‰, than the surrounding leaf litter (−29‰). The δ13C are small, but in the negative direction. Based on the observations of Bird et al. (2017), this ingress is limited to 1–2% carbon; therefore as the carbon increase for the NL treatment is so large, these low changes in δ13C indicates use of indigenous sample carbon by microbiota inhabiting biochar surfaces.
Chemical changes in the samples following environmental exposure include oxidation of the biochar structure, which is most complete for samples under the NL treatment, where significant increases in oxygen concentration are observed. The results of the 13C-MAS-NMR measurement suggest that direct oxygenation of aromatic structures may be even more important than carboxylation in biochar/ PyC alteration, as signal at 150 ppm for oxygenated aromatics (Freitas et al., 1999) is present, while carboxylic peaks are small. Similarly, changes in the amounts of PAHs in samples after exposure indicate removal of pre-existing small-ring aromatics, and possibly removal of material that has been formed by cleavage from the larger aromatic skeleton of the biochar. However, the fact that these PAHs are extractable from the samples after environmental exposure points to these being sorbed to the sample structure, slowing their biodegradation and/or removal from site of formation after being cleaved from initially larger aromatic structures (c.f. Manilal and Alexander, 1991).
Conclusions
It is clear that at a given production temperature, the chemistry of lignocellulosic and non-lignocellulosic material differs significantly, directly impacting its environmental behavior. In particular, the chemistry and alteration pathway of biochar/ PyC from non-lignocellulosic biomass is very different to that of lignocellulosic materials such as wood. While this reduces the carbon sequestration potential of this material, benefits lie in the direction of a short-term fertilization effect, as key soil nutrients are readily released into the soil environment. It is on this basis that high-N biomass has been proposed as a source of biochar (e.g., algae, poultry litter Chan et al., 2008), enhancing the physical and chemical benefits offered by the polyaromatic biochar structure itself. The requirement for continued biochar inputs to maintain this effect clearly depends upon environment and agricultural need, which is an important consideration for application of this type of material. Further, this heterogeneity in starting materials and corresponding environmental behavior means it is not possible to extend the results obtained on one sample type or one production temperature to different material; analyses must be performed on “like for like” material.
After 12 months of exposure in a tropical biome, alteration of biochar is observable, predominantly degradation of the “labile” component (sensu Bird et al., 2015). There is also evidence for alteration of a proportion of the sample aromatic fraction, due to the rise in extractable 2–7 ring PAHs in some samples, potentially due to the degradation of larger aromatic structures. In this, oxidation may play a role, and we have identified direct oxygenation of aromatic structures as a possible mechanism, operating in conjunction with the more commonly-cited carboxylation (e.g., Cohen-Ofri et al., 2006). Despite this, a significant proportion of the non-aromatic material and small-ring aromatics remained, indicating this material was not yet accessible for degradation due to a physical or chemical protection mechanism.
In terms of depositional environment, it appears that oxygen availability and physical or chemical protection from sunlight and/or rainwater, is vital in determining the alteration trajectory of biochar, whereby buried material will be altered more slowly than that exposed on the soil surface, supporting the results of previous work (Zimmermann et al., 2012; Bird et al., 2017). A further implication of this work is that even for material with a very different δ13C to the surrounding environment, changes due to the ingress of exogenous carbon are likely to be small. Carbon isotope values are a valuable source of (palaeo) environmental proxy data, and it appears that the δ13C of biochar recovered for analysis from natural environmental deposits is not distorted by a large amount from the original sample δ13C. It is clearly important however, that non-PyC material is removed as far as possible; techniques such as hydropyrolysis (Ascough et al., 2009, 2010a; Meredith et al., 2012) are very useful in this regard.
Author Contributions
PA led preparation of the manuscript, undertook fieldwork, performed data analysis and interpretations, MB coordinated fieldwork and sample recovery, assisted with data analysis and interpretations, WM performed hydropyrolysis measurements, analysis of GC-MS measurements and scientific interpretations, CS coordinated chemical analyses, assisted with data analysis, and interpretation of analyses, DL coordinated chemical analyses and assisted with interpretations, ET performed chemical analyses, coordinated elemental and isotopic measurements and performed data interpretation, DA performed NMR measurements, data analysis and interpretations, AB performed GC-MS analyses and interpretation of results, LS performed hydropyrolysis measurements and interpretation of results. All authors had intellectual input to the study, and contributed to the preparation of the manuscript.
Conflict of Interest Statement
The authors declare that the research was conducted in the absence of any commercial or financial relationships that could be construed as a potential conflict of interest.
Acknowledgments
Overall financial support was provided by NERC (NE/F017456/1). Financial support for fieldwork was provided via the Carnegie Trust for the Universities of Scotland Small Grant Scheme. This publication represents a contribution from the Scottish Alliance for Geoscience, Environment, and Society (SAGES). Samples of 14C-depleted Nothofagus wood were kindly provided by Mr B. Wood (TRUenergy, Australia). We thank Barry Thornton (James Hutton Institute, UK) for the elemental analyses for oxygen and hydrogen, and Kerry Sayle (SUERC) for elemental and stable isotopic analyses of carbon and nitrogen. Stephanie Ascough (Imperial College, London) is thanked for assistance with statistical testing.
Supplementary Material
The Supplementary Material for this article can be found online at: https://www.frontiersin.org/articles/10.3389/feart.2018.00061/full#supplementary-material
References
Abiven, S., Hengartner, P., Schneider, M. P. W., Singh, N., and Schmidt, M. W. I. (2011). Pyrogenic carbon soluble fraction is larger and more aromatic in aged charcoal than in fresh charcoal. Soil Biol. Biochem. 43, 1615–1617. doi: 10.1016/j.soilbio.2011.03.027
Ameloot, N., Graber, E. R., Verheijen, F. G. A., and De Neve, S. (2013). Interactions between biochar stability and soil organisms: review and research needs. Eur. J. Soil Sci. 64, 379–390. doi: 10.1111/ejss.12064
Ascough, P. L., Bird, M. I., Brock, F., Higham, T. F. G., Meredith, W., Snape, C., et al. (2009). Hydropyrolysis as a new tool for radiocarbon pretreatment and the quantification of black carbon. Quat. Geochronol. 4, 140–147. doi: 10.1016/j.quageo.2008.11.001
Ascough, P. L., Bird, M. I., Francis, S. M., Thornton, B., Midwood, A., Scott, A. C., et al. (2011). Variability in oxidative degradation of charcoal: influence of production variables and environmental exposure. Geochim. Cosmochim. Acta 75, 2361–2378. doi: 10.1016/j.gca.2011.02.002
Ascough, P. L., Bird, M. I., Meredith, W., Wood, R. E., Snape, C. E., Brock, F., et al. (2010a). Hydropyrolysis: implications for radiocarbon pre-treatment and characterization of Black Carbon. Radiocarbon 52, 1336–1350. doi: 10.1017/S0033822200046427
Ascough, P. L., Bird, M. I., Scott, A. E., Collinson, M. E., Cohen-Ofri, I., Snape, C. E., et al. (2010b). Charcoal reflectance measurements: implications for structural characterization and assessment of diagenetic alteration. J. Archaeol. Sci. 37, 1590–1599. doi: 10.1016/j.jas.2010.01.020
Ascough, P., Bird, M. I., Wormald, P., Snape, C. E., and Apperley, D. (2008). Influence of pyrolysis variables and starting material on charcoal stable isotopic and molecular characteristics. Geochim. Cosmochim. Acta 72, 6090–6102. doi: 10.1016/j.gca.2008.10.009
Ascough, P. L., Sturrock, C. J. L., and Bird, M. I. (2010c). Investigation of growth responses in saprophytic fungi to charred biomass. Isotopes Environ. Health Stud. 46, 64–77. doi: 10.1080/10256010903388436
Ballentine, D. C., Macko, S. A., Turekian, V. C., Gilhooly, W. P., and Martincigh, B. (1996). Compound specific isotope analysis of fatty acids and polycyclic aromatic hydrocarbons in aerosols: implications for biomass burning. Org. Geochem. 25, 97–104. doi: 10.1016/S0146-6380(96)00110-6
Bird, M. I., and Ascough, P. L. (2012). Isotopes in pyrogenic carbon: a review. Org. Geochem. 42, 1529–1539. doi: 10.1016/j.orggeochem.2010.09.005
Bird, M. I., Ascough, P. L., Young, I. M., and Wood, C. V. (2008). X-ray ray Microtomographic microtomographic Imaging imaging of Charcoalcharcoal. J. Archaeol. Sci. 35, 2698–2706. doi: 10.1016/j.jas.2008.04.018
Bird, M. I., Levchenko, V., Ascough, P. L., Meredith, W., Wurster, C. M., Williams, A., et al. (2014). The efficiency of charcoal decontamination for radiocarbon dating by three pre-treatments–ABOX, ABA and hypy. Quat. Geochronol. 22, 25–32. doi: 10.1016/j.quageo.2014.02.003
Bird, M. I., McBeath, A., Ascough, P. L., Levchenko, V., Wurster, C. M., Munksgaard, N. C., et al. (2017). Loss and gain of carbon during char degradation. Soil Biol. Biochem. 106, 80–89. doi: 10.1016/j.soilbio.2016.12.012
Bird, M. I., Wurster, C. M., de Paula Silva, P. H., Bass, A., and de Nys, R. (2011). Algal biochar – production and properties. Bioresour. Technol. 102, 1886–1891. doi: 10.1016/j.biortech.2010.07.106
Bird, M. I., Wynn, J. G., Saiz, G., Wurster, C. M., and McBeath, A. (2015). The pyrogenic carbon cycle. Annu. Rev. Earth Planet. Sci. 43, 9–1. doi: 10.1146/annurev-earth-060614-105038
Braadbaart, F., Poole, I., and Van Brussel, A. A. (2009). Preservation potential of charcoal in alkaline environments: an experimental approach and implications for the archaeological record. J. Archaeol. Sci. 36, 1672–1679. doi: 10.1016/j.jas.2009.03.006
Bruun, S., Jensen, E. S., and Jensen, L. S. (2008). Microbial mineralization and assimilation of black carbon: Dependency on degree of thermal alteration. Org. Geochem. 39, 839–845. doi: 10.1016/j.orggeochem.2008.04.020
Carcaillet, C. (2001). Are Holocene wood-charcoal fragments stratified in alpine and subalpine soils? Evidence from the Alps based on AMS C-14 dates. Holocene 11, 231–242. doi: 10.1191/095968301674071040
Chan, K. Y., Van Zwieten, L., Meszaros, I., Downie, A., and Joseph, S. (2008). Using poultry litter biochars as soil amendments. Soil Res. 46, 437–444. doi: 10.1071/SR08036
Cheng, C.-H., Lehmann, J., and Engelhard, M. H. (2008a). Natural oxidation of black carbon in soils: changes in molecular form and surface charge along a climosequence, Geochimica et Cosmochimica. Acta 72, 1598–1610. doi: 10.1016/j.gca.2008.01.010
Cheng, C.-H., Lehmann, J., Thies, J. E., and Burton, S. D. (2008b). Stability of black carbon in soils across a climatic gradient. J. Geophys. Res. 113:G02027. doi: 10.1029/2007JG000642
Cohen-Ofri, I., Weiner, L., Boaretto, E., Mintz, G., and Weiner, S. (2006). Modern and fossil charcoal: aspects of structure and diagenesis. J. Archaeol. Sci. 33, 428–439. doi: 10.1016/j.jas.2005.08.008
Deckers, J. A., Nachtergaele, F., and Spaargaren, O. C. (1998). World Reference Base for Soil Resources: Introduction, Vol. 1. Leuven: Acco.
DeLuca, T. H., and Aplet, G. H. (2008). Charcoal and carbon storage in forest soils of the Rocky Mountain west. Front. Ecol. Environ. 6, 18–24. doi: 10.1890/070070
Donato, D. C., Campbell, J. L., Fontaine, J. B., and Law, B. E. (2009). Quantifying char in postfire woody detritus inventories. Fire Ecol. 5, 104–115. doi: 10.4996/fireecology.0502104
Farrell, M., Kuhn, T. K., Macdonald, L. M., Maddern, T. M., Murphy, D. V., Hall, P. A., et al. (2013). Microbial utilisation of biochar-derived carbon. Sci. Total Environ. 465, 288–297. doi: 10.1016/j.scitotenv.2013.03.090
Freitas, J. C. C., Bonagamba, T. J., and Emmerich, F. G. (1999). 13C high-resolution solid-state NMR study of peat carbonization. Energy Fuels 11, 53–59. doi: 10.1021/ef980075c
Hammes, K., and Schmidt, M. W. I. (2009). “Changes of biochar in soil,” in Biochar for Environmental Management, eds J. Lehmann, and S. Joseph (London: Earthscan), 169–182.
Harvey, O. R., Kuo, L. J., Zimmerman, A. R., Louchouarn, P., Amonette, J. E., and Herbert, B. E. (2012). An index-based approach to assessing recalcitrance and soil carbon sequestration potential of engineered black carbons (biochars). Environ. Sci. Technol. 46, 1415–1421. doi: 10.1021/es2040398
Hilscher, A., Heister, K., Siewert, C., and Knicker, H. (2009). Mineralisation and structural changes during the initial phase of microbial degradation of pyrogenic plant residues in soil. Org. Geochem. 40, 332–342. doi: 10.1016/j.orggeochem.2008.12.004
Huston, M. A., and Wolverton, S. (2009). The global distribution of net primary production: resolving the paradox. Ecol. Monogr. 79, 343–377. doi: 10.1890/08-0588.1
Isbell, R. (2002). The Australian Soil Classification. Revised Edition, Vol. 4. Collingwood, VIC: CSIRO Publishing.
Jaafar, N. M., Clode, P. L., and Abbott, L. K. (2015). Soil microbial responses to biochars varying in particle size, surface and pore properties. Pedosphere 255, 770–780. doi: 10.1016/S1002-0160(15)30058-8
Keiluweit, M., Kleber, M., Sparrow, M. A., Simoneit, B. R., and Prahl, F. G. (2012). Solvent-extractable polycyclic aromatic hydrocarbons in biochar: influence of pyrolysis temperature and feedstock. Environ. Sci. Technol. 46, 9333–9341. doi: 10.1021/es302125k
Knicker, H. (2010). “Black nitrogen” – an important fraction in determining the recalcitrance of charcoal. Orga. Geochem. 41, 947–950. doi: 10.1016/j.orggeochem.2010.04.007
Krull, E. S., Baldock, J. A., Skjemstad, J. O., and Smernik, R. J. (2009). “Characteristics of biochar: organo-chemical properties,” in Biochar for Environmental Management, eds J. Lehmann and S. Joseph (Routledge: Earthscan Publications Ltd.), 53–65.
Kuhlbusch, T. A. J. (1998). Black carbon and the carbon cycle. Science 280, 1903–1904. doi: 10.1126/science.280.5371.1903
Lehmann, J., da Silva, J. P., Steiner, C., Nehls, T., Zech, W., and Glaser, B. (2003). Nutrient availability and leaching in an archaeological Anthrosol and a Ferralsol of the Central Amazon basin: fertilizer, manure and charcoal amendments. Plant Soil 249, 343–357. doi: 10.1023/A:1022833116184
Lehmann, J., Gaunt, J., and Rondon, M. (2006). Bio-char sequestration in terrestrial ecosystems. Mitig. Adapt. Strat. Glob. Change 11, 395–419. doi: 10.1007/s11027-005-9006-5
Lehmann, J., Rillig, M. C., Thies, J., Masiello, C. A., Hockaday, W. C., and Crowley, D. (2011). Biochar effects on soil biota – a review. Soil Biol. Biochem. 43, 1812–1836. doi: 10.1016/j.soilbio.2011.04.022
Major, J., Lehmann, J., Rondon, M., and Goodale, C. (2010). Fate of soil-applied black carbon: downward migration, leaching and soil respiration. Glob. Chang. Biol. 16, 1366–1379. doi: 10.1111/j.1365-2486.2009.02044.x
Manilal, V. B., and Alexander, M. (1991). Factors affecting the microbial degradation of phenanthrene in soil. Appl. Microbiol. Biotechnol. 35, 401–405. doi: 10.1007/BF00172733
McBeath, A. V., Smernik, R. J., Krull, E. S., and Lehmann, J. (2014). The influence of feedstock and production temperature on biochar carbon chemistry: a solid-state 13C NMR study. Biomass Bioenergy 60, 121–129. doi: 10.1016/j.biombioe.2013.11.002
McBeath, A. V., Wurster, C. M., and Bird, M. I. (2015). Influence of feedstock properties and pyrolysis conditions on biochar carbon stability as determined by hydrogen pyrolysis. Biomass Bioenergy 73, 155–173. doi: 10.1016/j.biombioe.2014.12.022
Meredith, W., Ascough, P. L., Bird, M. I., Large, D. J., Snape, C. E., Sun, Y., et al. (2012). Assessment of hydropyrolysis as a method for the quantification of black carbon using standard reference materials. Geochim. Cosmochim. Acta 97, 131–147. doi: 10.1016/j.gca.2012.08.037
Meredith, W., Ascough, P. L., Bird, M. I., Large, D. J., Snape, C. E., Song, J., et al. (2013). Direct evidence from hydropyrolysis for the retention of long alkyl moieties in soil black carbon fractions isolated by acid dichromate oxidation. J. Anal. Appl. Pyrolysis 103, 232–239. doi: 10.1016/j.jaap.2012.11.001
Middelburg, J. J., Nieuwenhuize, J., Lubberts, R. K., and van de Plassche, O. (1997). Organic carbon isotope systematics of coastal marshes. Estuar. Coast. Shelf Sci. 45, 681–687. doi: 10.1006/ecss.1997.0247
Mohan, D., Sarswat, A., Ok, Y. S., and Pittman, C. U. (2014). Organic and inorganic contaminants removal from water with biochar, a renewable, low cost and sustainable adsorbent a critical review. Bioresour. Technol. 160, 191–202. doi: 10.1016/j.biortech.2014.01.120
Novak, J. M., Busscher, W. J., Laird, D. L., Ahmedna, M., Watts, D. W., and Niandou, M. (2009). Impact of biochar amendment on fertility of a southeastern coastal plain soil. Soil Sci. 174, 105–112. doi: 10.1097/SS.0b013e3181981d9a
Preston, C. M., and Schmidt, M. W. I. (2006). Black (pyrogenic) carbon in boreal forests: a synthesis of current knowledge and uncertainties. Biogeosci. Discuss 3, 211–271. doi: 10.5194/bgd-3-211-2006
Santín, C., Doerr, S. H., Kane, E. S., Masiello, C. A., Ohlson, M., de la Rosa, J. M., et al. (2016). Towards a global assessment of pyrogenic carbon from vegetation fires. Glob. Chang. Biol. 22, 76–91. doi: 10.1111/gcb.12985
Santín, C., Doerr, S. H., Merino, A., Bucheli, T. D., Bryant, R., Ascough, P., et al. (2017). Carbon sequestration potential and physicochemical properties differ between wildfire charcoals and slow-pyrolysis biochars. Sci. Rep. 7:11233. doi: 10.1038/s41598-017-10455-2
Schmidt, M. W. I., and Noack, A. G. (2000). Black carbon in soils and sediments: analysis, distribution, implications, and current challenges. Global Biogeochem. Cycles 14, 777–793. doi: 10.1029/1999GB001208
Simoneit, B. R. T. (2002). Biomass burning - a review of organic tracers for smoke from incomplete combustion. Appl. Geochem. 17, 129–162. doi: 10.1016/S0883-2927(01)00061-0
Singh, B. P., Cowie, A. L., and Smernik, R. J. (2012). Biochar carbon stability in a clayey soil as a function of feedstock and pyrolysis temperature. Environ. Sci. Technol. 46, 11770–11778. doi: 10.1021/es302545b
Spokas, K. A. (2010). Review of the stability of biochar in soils: predictability of O:C molar ratios. Carbon Manage. 1, 289–303. doi: 10.4155/cmt.10.32
St John, T. V. (1980). Influence of litterbags on growth of fungal vegetative structures. Oecologia 46, 130–132. doi: 10.1007/BF00346977
Steinbeiss, S., Gleixner, G., and Antonietti, M. (2009). Effect of biochar amendment on soil carbon balance and soil microbial activity. Soil Biol. Biochem. 41, 1301–1310. doi: 10.1016/j.soilbio.2009.03.016
Tilston, E. L., Ascough, P. L., Garnett, M. H., and Bird, M. I. (2016). Quantifying charcoal degradation and negative priming of soil organic matter with a radiocarbon-dead tracer. Radiocarbon 58, 905–919. doi: 10.1017/RDC.2016.45
Torello-Raventos, M., Feldpausch, T. R., Veenendaal, E., Schrodt, F., Saiz, G., Domingues, T. F., et al. (2013). On the delineation of tropical vegetation types with an emphasis on forest/savanna transitions. Plant Ecol. Divers. 6, 101–137. doi: 10.1080/17550874.2012.762812
Wang, J., Xiong, Z., and Kuzyakov, Y. (2016). Biochar stability in soil: meta-analysis of decomposition and priming effects. GCB Bioenergy 8, 512–523. doi: 10.1111/gcbb.12266
Zimmerman, A. R. (2010). Abiotic and microbial oxidation of laboratory-produced black carbon (biochar). Environ. Sci. Technol. 44, 1295–1301. doi: 10.1021/es903140c
Keywords: pyrogenic carbon, black carbon, PAHs, charcoal, biochar
Citation: Ascough PL, Bird MI, Meredith W, Snape C, Large D, Tilston E, Apperley D, Bernabé A and Shen L (2018) Dynamics of Charcoal Alteration in a Tropical Biome: A Biochar-Based Study. Front. Earth Sci. 6:61. doi: 10.3389/feart.2018.00061
Received: 09 August 2017; Accepted: 07 May 2018;
Published: 04 June 2018.
Edited by:
Samuel Abiven, Universität Zürich, SwitzerlandReviewed by:
Bernardo Maestrini, Michigan State University, United StatesChristophe Naisse, RITTMO Agroenvironnement, France
Copyright © 2018 Ascough, Bird, Meredith, Snape, Large, Tilston, Apperley, Bernabé and Shen. This is an open-access article distributed under the terms of the Creative Commons Attribution License (CC BY). The use, distribution or reproduction in other forums is permitted, provided the original author(s) and the copyright owner are credited and that the original publication in this journal is cited, in accordance with accepted academic practice. No use, distribution or reproduction is permitted which does not comply with these terms.
*Correspondence: Philippa L. Ascough, philippa.ascough@glasgow.ac.uk