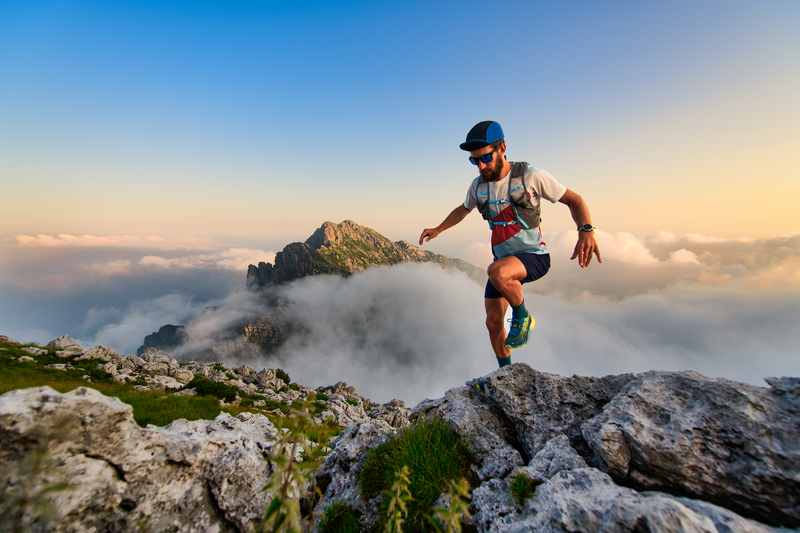
95% of researchers rate our articles as excellent or good
Learn more about the work of our research integrity team to safeguard the quality of each article we publish.
Find out more
MINI REVIEW article
Front. Cardiovasc. Med. , 14 August 2019
Sec. Cardiovascular Metabolism
Volume 6 - 2019 | https://doi.org/10.3389/fcvm.2019.00116
This article is part of the Research Topic Impact of Adipose Tissues on Cardiovascular Diseases View all 6 articles
The number of patients with obesity continues to increase seriously worldwide. It has become clear that, against a background of insulin resistance, obesity induces the so-called metabolic syndrome consisting of diabetes, hypertension, and dyslipidemia, leading, consequently, to an increased incidence of cardiovascular disease in affected individuals. It is shown that environmental factors, e.g., high-fat diet and lack of physical activity, not only promote the onset of obesity but lead to impairment of the action of adiponectin and its receptors, thus accounting in part for the onset of insulin resistance, type 2 diabetes/metabolic syndrome, and atherosclerosis in modern society. This review is intended to highlight some milestones in adipocyte research from the discovery of the insulin-sensitizing properties of adiponectin to the elucidation of the structures of its receptors, as well as to clarify their therapeutic implications and prospects for lifestyle-related diseases.
From the early 1990s onward, besides storing excess energy in the form of triglycerides, adipocytes have also been shown to function as endocrine organs secreting a variety of physiologically active substances currently known as “adipokines” (1–3). Again, while the mechanisms through which obesity induces insulin resistance remained largely unknown, it has now become clear that enlarged adipocytes secrete adipokines, such as TNF-α and MCP-1, in large amounts, thus not only impairing insulin signaling in the skeletal muscle and liver but inducing systemic insulin resistance (4, 5). In contrast, it is shown that the production/secretion of the “beneficial adipokine” adiponectin is decreased in obesity (6).
Adiponectin has been originally identified through different approaches by four independent research groups as a protein secreted and expressed exclusively in adipose tissue (7–10). With a molecular weight of about 30 kDa, adiponectin is shown to consist of an amino-terminal signal sequence, a specific variable region containing cysteine residues essential for multimer formation of adiponectin, a collagen-like domain, and a carboxyl-terminal globular domain. Adiponectin has thus come to be classified by its structural characteristics as a member of the complement 1q family. Despite having no homology to TNF-α in the primary amino-acid sequence, adiponectin is shown to be homologous to TNF-α in structure (11). The adiponectin trimer represents the basic building block of adiponectin multimers and is shown to be formed through hydrophobic interactions within the globular domain and stabilized through interactions within the collagen-like domains in a triple helix structure. In contrast, adiponectin hexamers and high-molecular-weight multimers requires an intermolecular disulfide bond between the cysteine residues conserved within a specific variable region for their formation (12–14).
At the time of its discovery of adiponectin, its functions remained virtually unknown. To date, however, it is shown that while adiponectin is decreased, and insulin resistance and dyslipidemia are induced, in a mouse model of obesity/type 2 diabetes, physiological doses of adiponectin improve these conditions in this mouse model (15). It is also shown that globular adiponectin promotes fatty acid oxidation (16) and increases insulin sensitivity in the liver thus inhibiting hepatic gluconeogenesis and decreasing blood glucose levels (17, 18). These experimental results suggest that adiponectin is decreased in obesity leading to the onset of insulin resistance and the metabolic syndrome, while adiponectin supplementation represents an effective therapeutic option for the metabolic syndrome associated with obesity.
Again, analysis of adiponectin-deficient mice (19–21) and adiponectin transgenic mice (22, 23) has revealed the long-term action of adiponectin in vivo, where insulin resistance, impaired glucose tolerance, hypertension, and dyslipidemia were shown to be present in the former, while insulin resistance and diabetes were shown to be improved in the latter.
While adiponectin is shown to be present in the blood as varying multimers, it is shown to be available primarily as low-molecular-weight (LMW) trimers, middle-molecular-weight (MMW) hexamers, and high-molecular-weight (HMW) multimers (12, 13), with the HMV multimers being the most potent of all adiponectin multimers in activating AMP kinase (AMPK) (24, 25). It is also reported that adiponectin is regulated by transcription factors in adipose tissue, such as peroxisome proliferator-activated receptor-γ (PPAR-γ) (26), sterol regulatory element-binding protein 1c (SREBP1c) (27), and CCAAT-enhancer-binding protein-α (C/EBP-α) (28). Again, while thiazolidinediones (TZDs) are shown to upregulate the expression of adiponectin via PPAR-γ (26), the mechanisms through which TZDs promote the secretion of HMW adiponectin multimers include endoplasmic reticulum chaperones, such as ERp44 and Ero-1Lα (29). Interestingly, it is also reported that blood adiponectin levels are elevated during fasting or starvation or under caloric restriction, and the mechanisms involved include elevated blood levels of HMW adiponectin associated with the activation of sirtuin 1 (SIRT1) (30, 31), improvements in insulin resistance as shown in the liver of high-fat diet-fed mice (32), increased expression of adiponectin and inhibition of inflammatory cytokines (33) associated with the deacetylation by SIRT1 of Lus268 and Lys293 in PPAR-γ.
On the other hand, adiponectin is found to be decreased in obesity, with its blood level shown to be inversely correlated with insulin resistance in humans (6). The influence of insulin on the blood level of adiponectin has been accounted for by increases in the expression of adiponectin in the adipose tissue of adipocyte-specific insulin receptor-deficient mice (34). Of note, blood adiponectin levels are shown to be elevated even in humans with genetic aberrations in insulin receptors who are associated with severe insulin resistance (35). These findings suggest that insulin stimulation in adipose tissue may lead to inhibition of adiponectin expression. Again, it is shown that decreased SIRT1 signaling, oxidative stress and inflammation lead to decreases in blood adiponectin levels in obesity, in contrast to those seen in starvation and under caloric restriction.
As for the relationship between impaired glucose metabolism and adiponectin, it is shown in a prospective study that the higher the blood adiponectin level, the lower the risk for diabetes in humans (36) and that the blood adiponectin level represents a better marker for the risk of onset of diabetes than blood glucose and insulin levels (37). Again, it has become clear that the greater the HMW adiponectin level, the lower the incidence of diabetes (38). Furthermore, as regards the relationship between dyslipidemia and adiponectin, research suggests a positive correlation between blood adiponectin levels and HDL-cholesterol levels, as well as a negative correlation between blood adiponectin levels and triglyceride levels in humans (39).
Thus, while adiponectin may with its systemic effects on glucose and lipid metabolism indirectly inhibit atherosclerosis, it also acts on vascular endothelial cells and inflammatory cells thus directly inhibiting atherosclerosis in humans. Of note, it is shown that vascular damage is associated with worsening of neointimal formation in adiponectin-deficient mice (20, 40), while adiponectin overexpression is shown to lead to inhibition of atherosclerosis in ApoE-deficient mice, which are known to serve as a mouse model of atherosclerosis (22). Again, it is shown that, even after adjustment for other risk factors, high blood adiponectin levels are associated with significant reductions in the risk of new onset of myocardial infarction in humans, with reports also available to demonstrate the effects of adiponectin on cardiovascular disease (41–43). It is also known that ischemia reperfusion injury increases myocardial apoptosis and the expression of TNF-α in adiponectin-deficient mice leading to increases in myocardial infarct size, suggesting that adiponectin provides direct cardio-protective effects (44).
With a focus on adiponectin binding, our research has led to the successful identification of adiponectin receptors (AdipoR1 and AdipoR2) (45), which are shown to have 66.7% amino-acid homology, with this homology shown to be maintained from yeast to humans, where, of note, the yeast homolog YOL002c is found to have a pivotal role in the oxidation of fatty acids (46). Of these, AdipoR1 is found to be fairly ubiquitously expressed in all tissues but abundantly expressed in skeletal muscle, while AdipoR2 is found to be expressed abundantly in the liver. One of the key features of AdipoRs is that they are novel seven-transmembrane receptors and that their topology is opposite to that of G-protein-coupled receptors (GPCRs) with an intracellular amino-terminus and an extracellular carboxyl-terminus. Again, the presence of AdipoR1 and AdipoR2 were confirmed in siRNA studies to be essential for the binding of adiponectin to the cell membrane surface in cultured cells (45), with loss of their binding and action shown in AdipoR1/AdipoR2 double-knockout mice, demonstrating that AdipoRs represent essential adiponectin receptors in the body (47).
Again, while AdipoR1/AdipoR2 double-knockout mice were found to be associated with insulin resistance and impaired glucose tolerance, this was accounted for by increased inflammation and oxidative stress in major metabolic organs, such as the skeletal muscle, liver and adipose tissue, leading to increased gluconeogenesis and decreased glucose uptake in these mice (47). Furthermore, the expression of AdipoR1 and AdipoR2 was found to be decreased in a mouse model of obesity/type 2 diabetes, which likely accounted in part for the onset of diabetes in the model, while adenovirus-mediated restoration of AdipoR1 expression in the liver was found to activate the AMPK pathway and restoration of AdipoR2 expression was found to activate PPARα, promote fatty acid oxidation, and improve impaired glucose tolerance in vivo through its anti-oxidative stress effects (47).
As for adiponectin binding proteins, it is shown that the both calreticulin and CD91 are involved in adiponectin-stimulated uptake of apoptotic cells (48), and pretreatment with anti-calreticulin antibodies attenuates the protective effects of adiponectin on myocyte apoptosis (49). It is also shown that T-cadherin is a receptor for hexameric and HMW adiponectin (50). Moreover, it is reported that in T-cadherin-knockout mice, adiponectin fails to act on cardiac tissue, and circulating levels of adiponectin are dramatically elevated (51, 52). Interestingly, T-cadherin is essential for adiponectin-mediated cardioprotection in mice (51). However, T-cadherin is thought to have no effect on adiponectin signaling, because T-cadherin has no intracellular domain. Further research, including studies with AdipoR1 and/or AdipoR2-knockout mice is necessary to elucidate the molecular mechanism by which adiponectin exerts its cardioprotective effects.
In target organs for insulin, e.g., liver and skeletal muscle, the expression of AdipoR1 and AdipoR2 is shown to significantly increase in fasted mice and decrease in refed mice. Consistently, in vitro studies show that insulin leads to reductions in the expression of AdipoR1 and AdipoR2 via the phosphoinositide 3 kinase/FoxO1-dependent pathway. It is shown that the expression of both AdipoR1/R2 is significantly decreased in the adipose tissue and muscle of insulin-resistant ob/ob mice, probably due in part to obesity-associated hyperinsulinemia through FoxO (53), with adiponectin-induced AMPK activation also shown to be impaired in the skeletal muscle of these mice, suggesting that adiponectin resistance is present in ob/ob mice, clearly due to decreases in the expression of AdipoR1/R2. Therefore, obesity is thought to lead to decreases not only in plasma adiponectin levels but also in AdipoR1/R2 expression, thereby reducing adiponectin sensitivity and inducing insulin resistance, which, in turn, leads to hyperinsulinemia thus resulting in a “vicious cycle” (53) (Figure 1). It is also reported that the expression of the AdipoRs is decreased in the skeletal muscle of type 2 diabetic patients (54). Furthermore, it is shown that the expression of the AdipoR gene is correlated with insulin sensitivity in non-diabetic Mexican Americans with or without a family history of type 2 diabetes (55).
Figure 1. The roles of decreased adiponectin/AdipoR effects in obesity-related diseases and potential strategies to increase the effects of AdipoR. Decreased adiponectin/AdipoR effects play causal roles in obesity-related diseases. Increased activation of AdipoR pathways may have beneficial effects on healthy longevity and obesity-related diseases, such as metabolic syndrome, type 2 diabetes, cardiovascular diseases, cancers, and Alzheimer's disease.
The total activity of PGC-1α was found to be decreased in skeletal muscle-specific AdipoR1-deficient mice to one-fourth that seen in normal mice, with decreases also found in mitochondrial mass and function and in proportion of type I muscle fibers, which led not only to decreased exercise endurance but to impaired glucose tolerance and insulin resistance at the organism-level (56). It was also found that adiponectin/AdipoR1 increases intracellular calcium (Ca2+) and AMP concentrations, thus offering exercise-mimicking signaling. One major finding of interest is that Ca2+ signaling and AMPK/SIRT1 activity were only partially restored, even with a range of agents targeting these pathways, in the phenotype represented by skeletal muscle-specific AdipoR1-deficient mice, suggesting the need for such concurrent activation of Ca2+ signaling and the AMPK/SIRT1 pathways as seen with exercise (56).
Although it is shown that AdipoR1 and AdipoR2 are involved in the regulation of glucose and fatty acid metabolism mainly through the activation of Ca2+ signaling and the AMPK/SIRT1, and PPARα signaling pathways, it appears likely that additional signaling pathways are also implicated in the pleiotropic actions of adiponectin. Indeed, research suggests that ceramide signaling may also be involved as an additional pathway in mediating such pleiotropic effects (57) and that adiponectin reduces cellular ceramide levels through the activation of ceramidase, as it induces conversion of ceramide to sphingosine, thus leading to reductions in hepatic ceramide levels and improvements in insulin sensitivity.
Conversely, adiponectin deficiency is shown to induce increases in hepatic ceramide levels, which may account in part for insulin resistance, while adiponectin is shown to increase sphingosine 1-phosphate (S1P) thus protecting against apoptosis machinery, such as induced by C2-ceramide or palmitate in pancreatic β cells and cardiac myocytes (57). Given that this protective effect is reversed by S1P itself or a ceramide biosynthesis inhibitor, it is likely that adiponectin-induced S1P protects β cells and cardiac myocytes from apoptotic cell death. Most importantly, this ceramide pathway, which is thought likely to be activated by adiponectin, is totally dependent on the expression of AdipoR1 and AdipoR2, given the observation that the overexpression of adiponectin, AdipoR1, and AdipoR2 leads to reductions in hepatic ceramide levels, as well as improvements in insulin sensitivity.
Changes produced in the metabolic capacity of individual organisms by enhanced adiponectin/AdipoR signaling are expected to contribute to the maintenance of their homeostasis. Thus, expectations are mounting for adiponectin/AdipoR-enhancing and AdipoR-activating agents, which appear to have great promise as exercise-mimicking agents providing similar effects to those with exercise, thus opening a new avenue not only for definitive therapy for the diabetes/metabolic syndrome and atherosclerosis but for effective therapeutic options for those unable to exercise due to medical or locomotive conditions. Against this background, our research has led to the successful identification of a small-molecule AdipoR-activating compound (adiponectin receptor agonist; AdipoRon) through scrutiny of candidate compounds available in the University of Tokyo Drug Discovery Innovation Center compound library (58). To date, it is shown that AdipoRon not only improves metabolism at the organ level (e.g., skeletal muscle, liver, and adipose tissue) but provides anti-diabetic properties at the organism level, while it normalizes a shortened lifespan due to obesity (58) (Figure 1).
AdipoR1 and AdipoR2 have been found to have novel structures, with each containing an internal cavity inside the seven transmembrane helices coordinating a zinc ion, demonstrating that their functions and structures are distinct from those of conventional GPCRs (59, 60).
A recent research showed further insights into the AdipoR1/AdipoR2 functions and structures (61), showing that they possess enzymatic activity, despite their extremely low ceramidase activity. Therefore, further research is required to characterize the parameters for their enzymatic activity and their substrate specificity, as well as to identify their further functions that may remain to be unraveled.
Ever since the elucidation in 2007 of the structure of a first human GPCR, GPCR research is being increasingly accelerated, with expectations mounting for further findings from their structural analysis that may also offer important leads for drug discovery. It is hoped that further structural analysis of AdipoRs will lead not only to elucidation of the mechanisms of signal transduction involved in AdipoRs as novel seven-transmembrane receptors but to provide invaluable clues for optimization of the adiponectin receptor agonist AdipoRon for human use (best-in-class). In conclusion, adiponectin receptor-activating agents appear to hold great promise as effective options for the prevention and treatment of type 2 diabetes and atherosclerosis, thereby contributing to healthy longevity in humans (62–64).
MI, MO-I, TY, and TK wrote the manuscript. All authors reviewed the manuscript.
This work was supported by JSPS KAKENHI grant numbers JP18K10988 (MI), JP19K11639, JP16K15487 (MO-I), JP26000012, JP19H01052 (TK), by the Translational Research Network Program (AMED) (MO-I), and by JST, PRESTO (JPMJPR13MF) (MI).
The authors declare that the research was conducted in the absence of any commercial or financial relationships that could be construed as a potential conflict of interest.
2. Zhang Y, Proenca R, Maffei M, Barone M, Leopold L, Friedman JM. Positional cloning of the mouse obese gene and its human homologue. Nature. (1994) 372:425–32. doi: 10.1038/372425a0
3. Spiegelman BM, Flier JS. Obesity and the regulation of energy balance. Cell. (2001) 104:531–43. doi: 10.1016/s0092-8674(01)00240-9
4. Hotamisligil GS, Shargill NS, Spiegelman BM. Adipose expression of tumor necrosis factor-alpha: direct role in obesity-linked insulin resistance. Science. (1993) 259:87–91.
5. Lazar MA. The humoral side of insulin resistance. Nat Med. (2006) 12:43–4. doi: 10.1038/nm0106-43
6. Arita Y, Kihara S, Ouchi N, Takahashi M, Maeda K, Miyagawa J, et al. Paradoxical decrease of an adipose-specific protein, adiponectin, in obesity. Biochem Biophys Res Commun. (1999) 257:79–83.
7. Scherer PE, Williams S, Fogliano M, Baldini G, Lodish HF. A novel serum protein similar to C1q, produced exclusively in adipocytes. J Biol Chem. (1995) 270:26746–9.
8. Hu E, Liang P, Spiegelman BM. AdipoQ is a novel adipose-specific gene dysregulated in obesity. J Biol Chem. (1996) 271:10697–703.
9. Maeda K, Okubo K, Shimomura I, Funahashi T, Matsuzawa Y, Matsubara K. cDNA cloning and expression of a novel adipose specific collagen-like factor, apM1 (AdiPose Most abundant Gene transcript 1). Biochem Biophys Res Commun. (1996) 221:286–9. doi: 10.1006/bbrc.1996.0587
10. Nakano Y, Tobe T, Choi-Miura NH, Mazda T, Tomita M. Isolation and characterization of GBP28, a novel gelatin-binding protein purified from human plasma. J Biochem. (1996) 120:803–12.
11. Shapiro L, Scherer PE. The crystal structure of a complement-1q family protein suggests an evolutionary link to tumor necrosis factor. Curr Biol. (1998) 8:335–8.
12. Pajvani UB, Du X, Combs TP, Berg AH, Rajala MW, Schulthess T, et al. Structure-function studies of the adipocyte-secreted hormone Acrp30/adiponectin. Implications fpr metabolic regulation and bioactivity. J Biol Chem. (2003) 278:9073–85. doi: 10.1074/jbc.M207198200
13. Waki H, Yamauchi T, Kamon J, Ito Y, Uchida S, Kita S, et al. Impaired multimerization of human adiponectin mutants associated with diabetes. Molecular structure and multimer formation of adiponectin. J Biol Chem. (2003) 278:40352–63. doi: 10.1074/jbc.M300365200
14. Tsao TS, Tomas E, Murrey HE, Hug C, Lee DH, Ruderman NB, et al. Role of disulfide bonds in Acrp30/adiponectin structure and signaling specificity. Different oligomers activate different signal transduction pathways. J Biol Chem. (2003) 278:50810–7. doi: 10.1074/jbc.M309469200
15. Yamauchi T, Kamon J, Waki H, Terauchi Y, Kubota N, Hara K, et al. The fat-derived hormone adiponectin reverses insulin resistance associated with both lipoatrophy and obesity. Nat Med. (2001) 7:941–6. doi: 10.1038/90984
16. Fruebis J, Tsao TS, Javorschi S, Ebbets-Reed D, Erickson MR, Yen FT, et al. Proteolytic cleavage product of 30-kDa adipocyte complement-related protein increases fatty acid oxidation in muscle and causes weight loss in mice. Proc Natl Acad Sci USA. (2001) 98:2005–10. doi: 10.1073/pnas.041591798
17. Berg AH, Combs TP, Du X, Brownlee M, Scherer PE. The adipocyte-secreted protein Acrp30 enhances hepatic insulin action. Nat Med. (2001) 7:947–53. doi: 10.1038/90992
18. Combs TP, Berg AH, Obici S, Scherer PE, Rossetti L. Endogenous glucose production is inhibited by the adipose-derived protein Acrp30. J Clin Invest. (2001) 108:1875–81. doi: 10.1172/JCI14120
19. Maeda N, Shimomura I, Kishida K, Nishizawa H, Matsuda M, Nagaretani H, et al. Diet-induced insulin resistance in mice lacking adiponectin/ACRP30. Nat Med. (2002) 8:731–7. doi: 10.1038/nm724
20. Kubota N, Terauchi Y, Yamauchi T, Kubota T, Moroi M, Matsui J, et al. Disruption of adiponectin causes insulin resistance and neointimal formation. J Biol Chem. (2002) 277:25863–6. doi: 10.1074/jbc.C200251200
21. Nawrocki AR, Rajala MW, Tomas E, Pajvani UB, Saha AK, Trumbauer ME, et al. Mice lacking adiponectin show decreased hepatic insulin sensitivity and reduced responsiveness to peroxisome proliferator-activated receptor gamma agonists. J Biol Chem. (2006) 281:2654–60. doi: 10.1074/jbc.M505311200
22. Yamauchi T, Kamon J, Waki H, Imai Y, Shimozawa N, Hioki K, et al. Globular adiponectin protected ob/ob mice from diabetes and ApoE-deficient mice from atherosclerosis. J Biol Chem. (2003) 278:2461–8. doi: 10.1074/jbc.M209033200
23. Combs TP, Pajvani UB, Berg AH, Lin Y, Jelicks LA, Laplante M, et al. A transgenic mouse with a deletion in the collagenous domain of adiponectin displays elevated circulating adiponectin and improved insulin sensitivity. Endocrinology. (2004) 145:367–83. doi: 10.1210/en.2003-1068
24. Yamauchi T, Kamon J, Minokoshi Y, Ito Y, Waki H, Uchida S, et al. Adiponectin stimulates glucose utilization and fatty-acid oxidation by activating AMP-activated protein kinase. Nat Med. (2002) 8:1288–95. doi: 10.1038/nm788
25. Kobayashi H, Ouchi N, Kihara S, Walsh K, Kumada M, Abe Y, et al. Selective suppression of endothelial cell apoptosis by the high molecular weight form of adiponectin. Circ Res. (2004) 94:e27–31. doi: 10.1161/01.RES.0000119921.86460.37
26. Maeda N, Takahashi M, Funahashi T, Kihara S, Nishizawa H, Kishida K, et al. PPARgamma ligands increase expression and plasma concentrations of adiponectin, an adipose-derived protein. Diabetes. (2001) 50:2094–9. doi: 10.2337/diabetes.50.9.2094
27. Seo JB, Moon HM, Noh MJ, Lee YS, Jeong HW, Yoo EJ, et al. Adipocyte determination- and differentiation-dependent factor 1/sterol regulatory element-binding protein 1c regulates mouse adiponectin expression. J Biol Chem. (2004) 279:22108–17. doi: 10.1074/jbc.M400238200
28. Saito K, Tobe T, Yoda M, Nakano Y, Choi-Miura NH, Tomita M. Regulation of gelatin-binding protein 28 (GBP28) gene expression by C/EBP. Biol Pharm Bull. (1999) 22:1158–62.
29. Wang ZV, Schraw TD, Kim JY, Khan T, Rajala MW, Follenzi A, et al. Secretion of the adipocyte-specific secretory protein adiponectin critically depends on thiol-mediated protein retention. Mol Cell Biol. (2007) 27:3716–31. doi: 10.1128/MCB.00931-06
30. Guarente L. Sirtuins as potential targets for metabolic syndrome. Nature. (2006) 444:868–74. doi: 10.1038/nature05486
31. Rodgers JT, Lerin C, Haas W, Gygi SP, Spiegelman BM, Puigserver P. Nutrient control of glucose homeostasis through a complex of PGC-1alpha and SIRT1. Nature. (2005) 434:113–8. doi: 10.1038/nature03354
32. Banks AS, Kon N, Knight C, Matsumoto M, Gutierrez-Juarez R, Rossetti L, et al. SirT1 gain of function increases energy efficiency and prevents diabetes in mice. Cell Metab. (2008) 8:333–41. doi: 10.1016/j.cmet.2008.08.014
33. Qiang L, Wang L, Kon N, Zhao W, Lee S, Zhang Y, et al. Brown remodeling of white adipose tissue by SirT1-dependent deacetylation of Ppargamma. Cell. (2012) 150:620–32. doi: 10.1016/j.cell.2012.06.027
34. Bluher M, Michael MD, Peroni OD, Ueki K, Carter N, Kahn BB, et al. Adipose tissue selective insulin receptor knockout protects against obesity and obesity-related glucose intolerance. Dev Cell. (2002) 3:25–38.
35. Semple RK, Soos MA, Luan J, Mitchell CS, Wilson JC, Gurnell M, et al. Elevated plasma adiponectin in humans with genetically defective insulin receptors. J Clin Endocrinol Metab. (2006) 91:3219–23. doi: 10.1210/jc.2006-0166
36. Li S, Shin HJ, Ding EL, van Dam RM. Adiponectin levels and risk of type 2 diabetes: a systematic review and meta-analysis. JAMA. (2009) 302:179–88. doi: 10.1001/jama.2009.976
37. Lindsay RS, Funahashi T, Hanson RL, Matsuzawa Y, Tanaka S, Tataranni PA, et al. Adiponectin and development of type 2 diabetes in the Pima Indian population. Lancet. (2002) 360:57–8. doi: 10.1016/S0140-6736(02)09335-2
38. Nakashima R, Kamei N, Yamane K, Nakanishi S, Nakashima A, Kohno N. Decreased total and high molecular weight adiponectin are independent risk factors for the development of type 2 diabetes in Japanese-Americans. J Clin Endocrinol Metab. (2006) 91:3873–7. doi: 10.1210/jc.2006-1158
39. Zoccali C, Mallamaci F, Tripepi G, Benedetto FA, Cutrupi S, Parlongo S, et al. Adiponectin, metabolic risk factors, and cardiovascular events among patients with end-stage renal disease. J Am Soc Nephrol. (2002) 13:134–41.
40. Matsuda M, Shimomura I, Sata M, Arita Y, Nishida M, Maeda N, et al. Role of adiponectin in preventing vascular stenosis. The missing link of adipo-vascular axis. J Biol Chem. (2002) 277:37487–91. doi: 10.1074/jbc.M206083200
41. Ouchi N, Kihara S, Arita Y, Maeda K, Kuriyama H, Okamoto Y, et al. Novel modulator for endothelial adhesion molecules: adipocyte-derived plasma protein adiponectin. Circulation. (1999) 100:2473–6.
42. Kumada M, Kihara S, Sumitsuji S, Kawamoto T, Matsumoto S, Ouchi N, et al. Association of hypoadiponectinemia with coronary artery disease in men. Arterioscler Thromb Vasc Biol. (2003) 23:85–9.
43. Hara K, Yamauchi T, Imai Y, Manabe I, Nagai R, Kadowaki T. Reduced adiponectin level is associated with severity of coronary artery disease. Int Heart J. (2007) 48:149–53.
44. Shibata R, Sato K, Pimentel DR, Takemura Y, Kihara S, Ohashi K, et al. Adiponectin protects against myocardial ischemia-reperfusion injury through AMPK- and COX-2-dependent mechanisms. Nat Med. (2005) 11:1096–103. doi: 10.1038/nm1295
45. Yamauchi T, Kamon J, Ito Y, Tsuchida A, Yokomizo T, Kita S, et al. Cloning of adiponectin receptors that mediate antidiabetic metabolic effects. Nature. (2003) 423:762–9. doi: 10.1038/nature01705
46. Karpichev IV, Cornivelli L, Small GM. Multiple regulatory roles of a novel Saccharomyces cerevisiae protein, encoded by YOL002c, in lipid and phosphate metabolism. J Biol Chem. (2002) 277:19609–17. doi: 10.1074/jbc.M202045200
47. Yamauchi T, Nio Y, Maki T, Kobayashi M, Takazawa T, Iwabu M, et al. Targeted disruption of AdipoR1 and AdipoR2 causes abrogation of adiponectin binding and metabolic actions. Nat Med. (2007) 13:332–9. doi: 10.1038/nm1557
48. Takemura Y, Ouchi N, Shibata R, Aprahamian T, Kirber MT, Summer RS, et al. Adiponectin modulates inflammatory reactions via calreticulin receptor-dependent clearance of early apoptotic bodies. J Clin Invest. (2007) 117:375–86. doi: 10.1172/JCI29709
49. Maruyama S, Shibata R, Ohashi K, Ohashi T, Daida H, Walsh K, et al. Adiponectin ameliorates doxorubicin-induced cardiotoxicity through Akt protein-dependent mechanism. J Biol Chem. (2011) 286:32790–800. doi: 10.1074/jbc.M111.245985
50. Hug C, Wang J, Ahmad NS, Bogan JS, Tsao TS, Lodish HF. T-cadherin is a receptor for hexameric and high-molecular-weight forms of Acrp30/adiponectin. Proc Natl Acad Sci USA. (2004) 101:10308–13. doi: 10.1073/pnas.0403382101
51. Denzel MS, Scimia MC, Zumstein PM, Walsh K, Ruiz-Lozano P, Ranscht B. T-cadherin is critical for adiponectin-mediated cardioprotection in mice. J Clin Invest. (2010) 120:4342–52. doi: 10.1172/JCI43464
52. Matsuda K, Fujishima Y, Maeda N, Mori T, Hirata A, Sekimoto R, et al. Positive feedback regulation between adiponectin and T-cadherin impacts adiponectin levels in tissue and plasma of male mice. Endocrinology. (2015) 156:934–46. doi: 10.1210/en.2014-1618
53. Tsuchida A, Yamauchi T, Ito Y, Hada Y, Maki T, Takekawa S, et al. Insulin/Foxo1 pathway regulates expression levels of adiponectin receptors and adiponectin sensitivity. J Biol Chem. (2004) 279:30817–22. doi: 10.1074/jbc.M402367200
54. Civitarese AE, Jenkinson CP, Richardson D, Bajaj M, Cusi K, Kashyap S, et al. Adiponectin receptors gene expression and insulin sensitivity in non-diabetic Mexican Americans with or without a family history of Type 2 diabetes. Diabetologia. (2004) 47:816–20. doi: 10.1007/s00125-004-1359-x
55. Debard C, Laville M, Berbe V, Loizon E, Guillet C, Morio-Liondore B, et al. Expression of key genes of fatty acid oxidation, including adiponectin receptors, in skeletal muscle of Type 2 diabetic patients. Diabetologia. (2004) 47:917–25. doi: 10.1007/s00125-004-1394-7
56. Iwabu M, Yamauchi T, Okada-Iwabu M, Sato K, Nakagawa T, Funata M, et al. Adiponectin and AdipoR1 regulate PGC-1alpha and mitochondria by Ca(2+) and AMPK/SIRT1. Nature. (2010) 464:1313–9. doi: 10.1038/nature08991
57. Holland WL, Miller RA, Wang ZV, Sun K, Barth BM, Bui HH, et al. Receptor-mediated activation of ceramidase activity initiates the pleiotropic actions of adiponectin. Nat Med. (2011) 17:55–63. doi: 10.1038/nm.2277
58. Okada-Iwabu M, Yamauchi T, Iwabu M, Honma T, Hamagami K, Matsuda K, et al. A small-molecule AdipoR agonist for type 2 diabetes and short life in obesity. Nature. (2013) 503:493–9. doi: 10.1038/nature12656
59. Tanabe H, Motoyama K, Ikeda M, Wakiyama M, Terada T, Ohsawa N, et al. Expression, purification, crystallization, and preliminary X-ray crystallographic studies of the human adiponectin receptors, AdipoR1 and AdipoR2. J Struct Funct Genomics. (2015) 16:11–23. doi: 10.1007/s10969-014-9192-z
60. Tanabe H, Fujii Y, Okada-Iwabu M, Iwabu M, Nakamura Y, Hosaka T, et al. Crystal structures of the human adiponectin receptors. Nature. (2015) 520:312–6. doi: 10.1038/nature14301
61. Vasiliauskaite-Brooks I, Sounier R, Rochaix P, Bellot G, Fortier M, Hoh F, et al. Structural insights into adiponectin receptors suggest ceramidase activity. Nature. (2017) 544:120–3. doi: 10.1038/nature21714
62. Iwabu M, Okada-Iwabu M, Yamauchi T, Kadowaki T. Adiponectin/adiponectin receptor in disease and aging. NPJ Aging Mech Dis. (2015) 1:15013. doi: 10.1038/npjamd.2015.13
63. Okada-Iwabu M, Iwabu M, Ueki K, Yamauchi T, Kadowaki T. Perspective of small-molecule AdipoR agonist for type 2 diabetes and short life in obesity. Diabetes Metab J. (2015) 39:363–72. doi: 10.4093/dmj.2015.39.5.363
Keywords: adiponectin, AdipoR, AdipoRon, exercise-mimicking agents, lifestyle-related diseases
Citation: Iwabu M, Okada-Iwabu M, Yamauchi T and Kadowaki T (2019) Adiponectin/AdipoR Research and Its Implications for Lifestyle-Related Diseases. Front. Cardiovasc. Med. 6:116. doi: 10.3389/fcvm.2019.00116
Received: 11 June 2019; Accepted: 29 July 2019;
Published: 14 August 2019.
Edited by:
Jose Javier Fuster, Centro Nacional de Investigaciones Cardiovasculares (CNIC), SpainReviewed by:
Yasumasa Ikeda, Tokushima University, JapanCopyright © 2019 Iwabu, Okada-Iwabu, Yamauchi and Kadowaki. This is an open-access article distributed under the terms of the Creative Commons Attribution License (CC BY). The use, distribution or reproduction in other forums is permitted, provided the original author(s) and the copyright owner(s) are credited and that the original publication in this journal is cited, in accordance with accepted academic practice. No use, distribution or reproduction is permitted which does not comply with these terms.
*Correspondence: Masato Iwabu, aXdhYnUtdGt5QHVtaW4uYWMuanA=
Disclaimer: All claims expressed in this article are solely those of the authors and do not necessarily represent those of their affiliated organizations, or those of the publisher, the editors and the reviewers. Any product that may be evaluated in this article or claim that may be made by its manufacturer is not guaranteed or endorsed by the publisher.
Research integrity at Frontiers
Learn more about the work of our research integrity team to safeguard the quality of each article we publish.