- 1Einthoven Laboratory for Experimental Vascular Medicine, Leiden University Medical Center, Leiden, Netherlands
- 2Department of Surgery, Leiden University Medical Center, Leiden, Netherlands
- 3Department of Cardiology, Leiden University Medical Center, Leiden, Netherlands
- 4Department of Immunohematology and Blood Transfusion, Leiden University Medical Center, Leiden, Netherlands
Aims: Vein grafts are frequently used conduits for arterial reconstruction in patients with cardiovascular disease. Unfortunately, vein graft disease (VGD) causes diminished patency rates. Innate immune system components are known to contribute to VGD. However, the role of T cells has yet to be established. The purpose of this study was to investigate the role of T cells and T cell activation pathways via the T cell receptor (TCR), co-stimulation and bystander effect in VGD.
Methods and results: Here, we show upon vein graft surgery in mice depleted of CD4+ T cells or CD8+ T cells, that CD8+ T cells are locally activated and have a major protective role for vein graft patency. In presence of CD8+ T cells vein grafts appear patent while CD8+ T cell depletion results in occluded vein grafts with increases apoptosis. Importantly, the protective effect of CD8+ T cells in VGD development was TCR and co-stimulation independent. This was demonstrated in vein grafts of OT-I mice, CD70−/−, CD80/86−/−, and CD70/80/86−/− mice compared to C57BL/6 mice. Interestingly, cytokines including IL-15, IL-18, IL-33, and TNF are up-regulated in vein grafts. These cytokines are co-operatively capable to activate CD8+ T cells in a bystander-mediated fashion, in contrast to CD4+ T cells.
Conclusions: T cells are modulators of VGD with a specific protective role of CD8+ T cells, which are locally activated in vein grafts. CD8+ T cells may protect against occlusive lesions by providing survival signals, and concert their protection independent of TCR and co-stimulation signaling.
Introduction
The vena saphena magna is the preferred conduit for arterial reconstruction in patients with cardiovascular disease. After vein graft surgery, intimal hyperplasia development through proliferation, and migration of vascular smooth muscle cells (VSMCs), deposition of extracellular matrix, and macrophage accumulation and proliferation, leads to narrowing of the vessel lumen, called vein graft disease (VGD) (1). Therefore, vein grafts display limited patency, with a rate of occlusion of ~60% in 10 years after surgery. Whereas, the innate immune system has been shown to be involved in VGD (2), the role of T cells of the adaptive immune system has yet to be established.
Previous studies have shown that T cells play a role in other cardiovascular diseases such as atherosclerosis (3, 4). Both CD4+ and CD8+ T cells are present in atherosclerotic plaques. Th1 CD4+ T cells have pro-atherogenic effects via production of IFNγ and TNFα, while Th2 CD4+ T cells and regulatory T cells limit atherosclerotic plaque formation via IL-10 and TGFβ production (5–8). Remarkably, CD8+ T cells seem to be locally activated in atherosclerotic plaques (9). CD8+ T cells have demonstrated both protective and pathogenic roles in atherosclerosis (10, 11). Whether CD4+ and CD8+ T cells are positive or negative modulators of VGD, is yet unknown.
T cells can be activated via different stimulatory signals provided by antigen presenting cells (APCs). Antigenic stimulation (signal 1) occurs via the T cell receptor (TCR) upon binding cognate peptide-MHC complexes. The Ig family member CD28 is constitutively expressed on naive T cells (TN), and provides imperative co-stimulation (signal 2) that cooperates with TCR triggering to achieve proper T cell activation. The ligands of CD28, i.e., CD80 and CD86, are up-regulated upon activation of APCs. Interaction of CD80/86 with the inhibitory receptor CTLA-4 leads to dampening of T cell activation (12–14). The co-stimulatory receptor CD27, belonging to the TNFR family, is also present on the majority of T cells (15), and interacts with its unique ligand CD70. Expression of CD70 is transiently up-regulated on subsets of activated APCs, and this constricted regulation is essential to avoid immune over-activation (16, 17). Inflammatory cytokines such as IL-12, IL-18, and type I interferons (IFN) can provide additional bystander signals (signal 3) for T cell activation (18). Macrophages and dendritic cells are well-studied APCs known to provide all these important signals to T cells, and both are present in vein grafts (1, 19). Notably VSMCs are also able to express MHC molecules and co-stimulatory molecules, especially after stimulation with interferon-γ (IFNγ) (20, 21), and in this way, VSMCs might be capable of T cell activation and function as APCs in vein grafts.
In this study, we examined the role of T cells in the development of VGD, and dissected the underlying mechanism of T cell activation pathways involved. Investigations in a murine VGD model revealed a strong protective effect of locally activated CD8+ T cells by depletion of either CD4+ T cells or CD8+ T cells. This protective effect was associated with increased apoptosis in CD8+ T cell depleted mice. Remarkably, by using TCR transgenic mice and co-stimulation knockout mice, we showed that this protective effect of CD8+ T cells in VGD development is TCR and co-stimulation independent. In addition, multiple bystander cytokines are present in the vein grafts and are able to induce CD8+ T cell activation. Thus, CD8+ T cells protect VGD development in a TCR independent and co-stimulation independent way, but most likely via bystander cytokines.
Materials and Methods
Study Approval and Mice
This study was performed in compliance with Dutch government guidelines and the Directive 2010/63/EU of the European Parliament. All animal experiments were approved by the animal welfare committee of the Leiden University Medical Center. C57BL/6 mice were purchased from Charles River Laboratories and crossbred in our own colony for at least 18 generations. CD70−/−, CD80/86−/−, and CD80/86/70−/− were bred in house on a C57BL/6 background (22). T cell receptor (TCR) transgenic mice recognizing the MHC class I-restricted SIINFEKL epitope derived from Ovalbumin (OT-I mice) (23) were obtained from The Jackson Laboratory. The TCR transgenic OT-I mouse is known to have an almost exclusive monoclonal TCR population (Va2/Vb5) (24). This is caused by the strong transgenic TCR expression, which accelerates passing of T cells via the double positive (DP) stage resulting in negligible endogenous segments to be rearranged. The transgenic beta chain induces allelic exclusion and suppresses rearrangement of endogenous beta chains. In TCR beta chain transgenic mice ~90% of peripheral T cells carry the transgenic beta chain and only 0–20% expresses endogenous ones. In the OT-I TCR transgenic mice we used, 99.6% of the CD8+ T cells had a Va2(Vb5) TCR (Figure S1) and 0.021% of CD8+ T cells expressed endogenous TCRs (Vb13 TCR), which we showed in the spleen. Thus, the endogenous TCR is barely present in OT-I TCR transgenic mice. All used mice were male mice aged 10 to 18 weeks old.
Vein Graft Surgery
Vein graft surgery was performed in mice by donor caval vein interpositioning in the carotid artery of recipient mice (25). All recipient mice received donor caval veins from genetic identical littermates. After 28 days mice were sacrificed and blood, draining, and non-draining lymph nodes, spleen, and vein graft or vena cava were harvested for flow cytometric analysis and/or (immuno)histochemical analysis. Mice were anesthetized by intraperitoneal injection of a combination of midazolam (5 mg/kg, Roche), medetomidine (0.5 mg/kg, Orion), and fentanyl (0.05 mg/kg, Janssen) before surgery and at sacrifice. Flumazenil (0.7 mg/kg, Fresenius Kabi) was intraperitoneal injected after surgery to antagonize anesthesia. After surgery and on indication, buprenorphine (0.1 mg/kg, MSD Animal Health) was given as an analgesic. Patency of vein graft was macroscopically determined at sacrifice and stated as clearly pulsatile, not clearly pulsatile or occluded.
Gene Set Enrichment Analysis
Vein grafts of male mice were harvested 14 days after surgery and compared with vein grafts harvested at surgery (day 0) (n = 4/group). Total RNA was isolated from vein grafts using TRIzol® (Ambion® by Life Technologies) and was quantitated using a NanoDrop 1,000 Spectrophotometer (Thermo Scientific). cDNA was synthesized using a High-Capacity cDNA Reverse Transcription Kit (Applied Biosystems) according to the manufacturer's protocol.
Gene set enrichment analysis (GSEA) was performed with the curated gene sets from Kegg, Biocarta, the Reactome, and published studies, resulting in a total of 1,564 gene sets. For each gene set an enrichment score (ES) is calculated representing the difference between expected and observed ranking, which correlates with the phenotype of the vein grafts. By permuting the phenotype labels, a statistical significance (nominal P-value) for the ES is provided. An adjustment for the gene set size generates the normalized enrichment score (NES). To correct for multiple testing the proportion of false positives is calculated to provide the false discovery rate (FDR) corresponding to each NES. A FDR <5% was considered significant. Method is fully described previously (26).
In vivo CD4+ and CD8+ T Cell Depletion
Depletion of either CD4+ T cells, CD8+ T cells or both was performed by intraperitoneal injections of depleting antibodies. Male mice were divided in five groups; CD4+ T cell depletion group (n = 12), CD8+ T cell depletion group (n = 12), CD4+, and CD8+ T cell depletion group (n = 12), control group (n = 12), and a naive (not operated group) group (n = 7). At 1 day prior to operation and 3, 10, 17, and 24 days after operation mice were injected with antibodies to deplete CD4 T cells (anti-CD4 clone GK1.5), CD8+ T cells (anti-CD8 clone 2.43), or both CD4+ T cells and CD8+ T cells or were injected with a control antibody (control mAb clone GL113). Prior to operation the injected amount was 200 μg mAb per mouse and, post-operative 100 μg per mouse was provided. Blood from tail vein was obtained 7 and 14 days after surgery and at sacrifice to check T cell subset depletion with flow cytometry.
Vein Graft Mouse Experiments
To examine if donor caval veins, used as a vein graft, contain (activated) T cells prior to operation, we performed vein graft surgery in male C57BL/6 mice (n = 3) and harvested the vein graft after 28 days, or performed no surgery (n = 3), and harvested the caval vein after 28 days. Vein grafts or caval veins were used for flow cytometry.
Vein graft surgery was performed in CD80/86/70−/− mice (n = 14), CD80/86−/− mice (n = 14), CD70−/− mice (n = 12), and C57BL/6 mice as a control (n = 11), fed a chow diet ad libitum and sacrificed after 28 days. Vein grafts were harvested for immunohistochemical analysis.
Vein graft surgery was performed in male OT-I mice (n = 5) and male C57BL/6 mice as a control (n = 11). Vein grafts were used for flow cytometry.
Flow Cytometry
Flow cytometry was performed on blood, spleen, draining, and non-draining lymph nodes, vena cava, and/or vein grafts. Single-cell suspensions were prepared from spleens and draining and non-draining lymph nodes, by mincing the tissue through a 70 μm cell strainer (BD Biosciences). Vein grafts and vena cavae were pre-treated with a liberase enzyme mix for 30 min and washed with 10 ml Iscove's Modified Dulbecco's Medium (IMDM, Lonza), 8% fetal calf serum (FCS, Life Technologies) and 100 U/mL penicillin/streptomycin (PS, Life Technologies). Erythrocytes were lysed in a red blood cell lysis buffer (hypotonic ammonium chloride buffer). Approximately 400,000 harvested cells from blood, spleen, draining, and non-draining lymph nodes and ~40,000 harvested cells from vena cava and vein grafts were used for the flow cytometry. Conjugated monoclonal antibodies to mouse CD11b (V450, eBioscience), Class II (V500, BD Horizon), Ly6C (fluorescein isothiocyanate [FITC], Biolegend), CD11c (phycoerythrin [PE], Biolegend), CD86 (Allophycocyanin [APC], Biolegend), F4/80 (PE-Cy7, Biolegend), Ly6G (Alexa Fluor 700, Biolegend), CD19 (APC-Cy7, eBioscience), CD44 (V450, eBioscience), CD8 (FITC, Biolegend), TCR Beta (PE, eBioscience), CD25 (APC, eBioscience), KLRG1 (PE-Cy7, eBioscience), CD62L (APC-Cy7, eBioscience), CD4 (Brilliant Violet 605, Biolegend) were used. Dead cells were excluded by positivity for 7-aminoactinomycinD (7-AAD) (Invitrogen). Flow cytometric acquisition was performed on a BD LSR II flow cytometer (BD Biosciences). Data were analyzed using FlowJo V10.1 software (BD). Flow cytometry gating strategies are shown in Figure S2.
RNA Isolation, cDNA Synthesis, and RT-PCR From Vein Graft Tissue
Total RNA was isolated from 10 (20 μm thick) paraffin sections of vein grafts 3, 7, 14, and 28 days after surgery. The method and analysis of RNA isolation, cDNA synthesis, and RT-PCR was performed as previously described (27). In brief, RNA was isolated according to manufacturer's protocol (FFPE RNA isolation kit, Qiagen). FFPE RNA was reverse transcribed using the RT2 First Strand Kit (SA Biosciences). RNA for single qPCR was reverse transcribed using a High-Capacity cDNA Reverse Transcription Kit according to the manufacturer's protocol. Commercially available TaqMan gene expression assays for hypoxanthine phosphoribosyl transferase (HPRT1), and selected genes of interest were used (Applied Biosystems). qPCR analysis was performed using a RT2 Profiler PCR Array (SA Biosciences) according to the manufacturer's protocol. The complete list of the genes analyzed is available at https://www.qiagen.com/us/products/discovery-and-translational-research/pcr-qpcr/qpcr-assays-and-instruments/mrna-incrna-qpcr-assays-panels/rt2-profiler-pcr-arrays/?catno=PAMM-016Z#geneglobe. Prior to RT2 profiler array, all qPCRs were performed on a 7500/7500 Fast Real-Time PCR System. In total we used six vein grafts per group, two pools of three vein grafts were analyzed. With the average of these two pools, the 2-ΔΔCt method was used to analyse the relative changes in gene expression. The fold change compared to the control vein graft was measured.
Vascular Wall Lesion Analysis
Vein graft samples were embedded in paraffin and sequential cross sections (5 μm thick) were made throughout the embedded vein grafts. To quantify the vein graft thickening (intimal hyperplasia), HPS staining was performed using Hematoxylin, Phloxin 0.25%, and Saffron 0.3%. Vessel wall area (vein graft thickening) was defined as the area between lumen and adventitia and determined by subtracting the luminal area from the total vessel wall area. Antibodies directed at Mac-3 (BD Pharmingen) to quantify macrophages and alpha smooth muscle cell actin (αSMactin, Sigma) to quantify smooth muscle cells were used for immunohistochemical staining (28). Sirius red staining (Klinipath 80115) was performed to quantify the amount of collagen present in the vein grafts. The immuno-positive area measured is expressed as a percentage of the vessel wall area. Stained slides were photographed using microscope photography software (Axiovision; Zeiss) and image analysis software was used (Qwin; Leica, Wetzlar). Fluorescent double staining was performed to identify the presence of CD3+ T cells. Vein grafts harvested after 28 days of C57BL/6 mice were stained with CD3+ (Abcam). Slides were covered with Invitrogen Diamond Antifade Mountant (Invitrogen) with 4',6-diamidino-2-phenylindole (DAPI). Fluorescent double staining were acquired with the fluorescent slide scanner (3DHistech).
Cell Culture
Vascular smooth muscle cells were acquired by isolating them from male mouse aortas (29). VSMCs were cultured using medium consisting of DMEM Glutamax with 20% fetal bovine serum (FBS, Sigma), 1% non-essential amino acids, and 1% PS.
D1 cells are a long-term growth factor-dependent immature myeloid dendritic cell line of splenic origin derived from a female C57BL/6 mouse. D1 cells were cultured in IMDM containing 10% heat inactivated FBS, 2 mM GlutaMax (GIBCO), 50 μM β-mercaptoethanol and 30% supernatant from R1 cells (mouse fibroblast NIH/3T3 cells transfected with mouse GM-CSF gene), which was collected from confluent cultures and filtered.
In vitro CD8+ T Cell Activation
CD8+ T cells of OT-I mice were isolated from the spleen of male and female mouse, by using the mouse CD8+ T lymphocyte enrichment set (BD Biosciences). VSMC ability to activate T cells was measured in supernatant of 50,000 VMSCs cultured with 500,000 CD8+ T cells of OT-I mice, and stimulated for 24 h with Ovalbumin (Ova) and lipopolysaccharide (LPS) 100 ng/mL from Escherichia coli K-235 (Sigma-Aldrich). DCs (as described above) were used as positive control. As VSMC control groups, VSMCs were cultured without OTI T cells or without Ovalbumin, or OTI T cells were stimulated with Ovalbumin without VSMCs.
To test the impact of cytokine combinations, 100,000 CD8+ T cells or CD4+ T cells isolated from spleens of C57BL/6 mice, were stimulated for 24 h with agonistic antibodies against CD3 (0.1 μg/ml), or with the following cytokines (either single or combined): IL-2 (20 ng/ml), IL-18 (20 ng/ml), IL-12 (20 ng/ml), IL-33 (20 ng/ml), IL-15 (100 ng/ml), and TNFα (20 ng/ml). Culture medium was used as a control. In all experiments, the concentration of IFNγ was measured in supernatant using ELISA assays (BD Biosciences).
Statistical Analysis
All data are presented as mean ± SEM. GraphPad Prism 7.0 software was used for statistical analyses. Analysis of parametric data, that passed normality and equal variance tests, were performed by using a 2-tailed Student's t-test to compare individual groups and a 2-way ANOVA comparing more than two groups. Mann-Whitney test was used for non-parametric data to compare individual groups that did not pass normality and equal variance tests, and a Kruskal-Wallis test was used to compare more than two groups. P < 0.05 was considered significant.
Results
Presence of Inflammatory Cells in Vein Grafts
To examine whether genes of the adaptive immune system are actively transcribed in vein grafts, we performed pathway analysis by GSEA on 1,564 available gene sets. We identified 176 significantly up-regulated curated gene sets in vein grafts sacrificed after 14 days compared to vena cavae at day 0, whereas 52 gene sets were down-regulated. In the top 35 of significantly up-regulated gene sets found in vein grafts, TCR and CD8+ T cell pathways are found besides the expected extracellular matrix organization pathways (Figures 1A,B). Cytokine/chemokine pathways and Toll and Nod-like receptors are also found among the most up-regulated gene sets, illustrating that pathways of the immune system are active in the development of VGD.
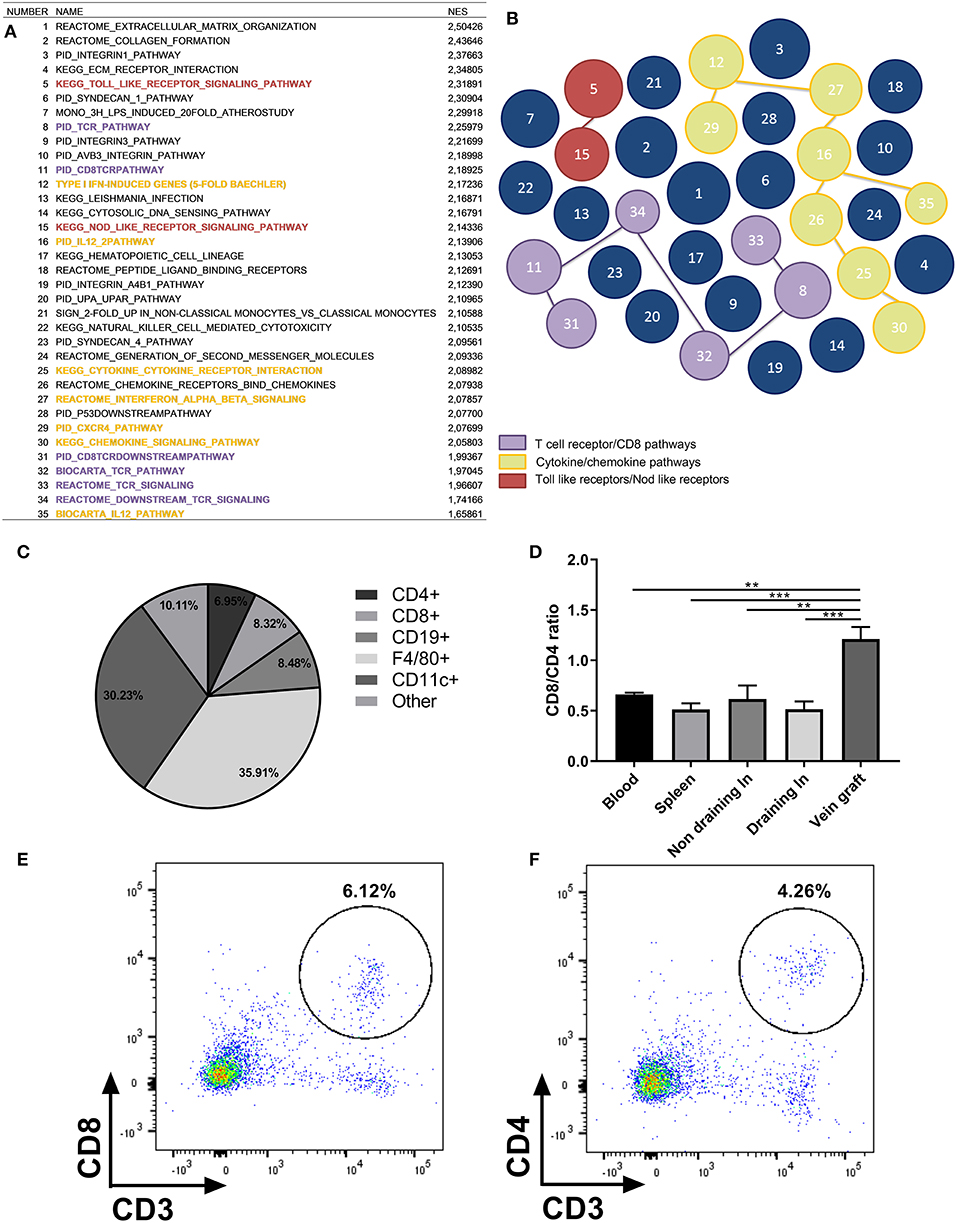
Figure 1. Presence of inflammatory cells in vein grafts. (A) Pathway analysis by gene set enrichment analysis (GSEA) on 1,564 available gene sets. The top 35 of significantly up-regulated gene sets found in vein grafts sacrificed after 14 days compared to vena cavae at day 0 are shown. Normalized enrichment score (NES) is shown. For each gene set (pathway) an enrichment score (ES) is calculated that represents the difference between expected and observed rankings which correlate with the phenotype. An adjustment for the gene set size generates the normalized enrichment score (NES). Colors are similar to the illustration in figure. (B) With TCR/CD8 pathways in purple, cytokine/chemokine pathways in yellow and Toll like receptors/Nod like receptors in red. The bigger the size of the circle, the higher the up-regulation of the gene. (C) Vein graft cell composition measured with FACS analysis. Percentage of CD4+, CD8+, CD19+, CD11b+, and remaining cells in vein grafts of C57BL/6 male mice as a percentage of all hematopoietic cells is shown, n = 4. (D) Ratio of CD8+/CD4+ T cells in vein grafts, blood, spleen, and draining lymph node is shown, n = 4. CD8+ and CD4+ T cells are a percentage of total CD3+ T cells. Significant differences between blood, spleen, (non) draining lymph nodes compared to vein grafts are indicated. (E) Example of flow cytometry plot is shown of CD3+ CD8+ T cells in vein grafts, and (F) CD3+ CD4+ T cells in vein grafts. **P < 0.01, *** P < 0.001, one-way ANOVA.
To show that immune cells are present in vein grafts, we stained vein grafts with the lymphocyte marker CD3. We showed that infiltrating CD3+ T cells are present in vein grafts (Figure S3). In addition, we analyzed vein grafts compared to blood, non-draining lymph nodes, draining lymph nodes, and spleens with flow cytometry. Both CD4+ and CD8+ T cells are abundantly present in vein grafts. Interestingly, CD8+ T cells constitute 8.32% ± 3.15 while the percentage of CD4+ T cells is 6.95% ± 2.13 (Figure 1C). An elevated CD8/CD4+ T cell ratio in vein grafts was not observed in other organs (Figures 1D–F). Other abundant immune subsets in vein grafts are F4/80+ macrophages (35.91% ± 2.83), CD11c+ dendritic cells (30.22% ± 1.44), and CD19+ B cells (8.48%±1.58). Thus, compared to lymphoid organs such as spleen and draining and non-draining lymph nodes (Figure S4), vein grafts have a higher CD8/CD4+ T cell ratio, relatively fewer B cells and more myeloid cells.
T Cells Are Locally Activated in Vein Grafts
Next, we examined the activation status of the T cells upon vein graft surgery. CD4+ and CD8+ T cells were phenotypically divided in TN (CD44-CD62L+), effector T cells (CD44+CD62L-), or central memory T cells (TCM) (CD44+CD62L+) (Figure 2A). In vein grafts of control mice we observed a 4-fold increased percentage of CD8+ (Figure 2B) and CD4+ (Figure 2C) effector T cells compared to other organs. Blood, spleen, draining, and non-draining lymph nodes mainly consisted of TN and TCM (Figure S5). The activation status of T cells in vein grafts was validated using the activation markers KLRG1 (23) and CD25. The percentage of KLRG1+CD62L– of total CD8+ and CD4+ T cells in vein grafts, as well as the percentage of CD25+CD44+ of total CD8+ T cells (but not total CD4+ T cells), was strikingly increased compared to blood, spleen, draining, and non-draining lymph nodes (Figures 2D–I). In addition, the percentage of activated effector T cells (CD44+CD62L-KLRG1+ and CD44+CD62L-CD25+) of total CD8+ and CD4+ T cells was significantly higher in vein grafts compared to other organs (Figure S6). A higher percentage of activated T cell in vein grafts compared to the surrounding draining and non-draining lymph nodes indicates local T cell activation in vein grafts. Furthermore, we compared blood, the spleen and non-draining lymph nodes from operated mice with blood, the spleen, and non-draining lymph nodes from naive, not operated mice. No differences were observed between control and naive mice in the percentage of activated T cells (KLRG1+CD62L– of total CD8+ and CD4+ T cells) and effector T cells (CD44+CD62L– of total CD8+ and CD4+ T cells) (Figure S7). This indicated that the vein graft operation on its own had no systemic T cell activation effect. Importantly, the vena cavae that were used as donor grafts showed comparable percentages of CD8+ T cells and CD4+ T cells as vein grafts (Figures S8A,B), but did not contain activated T cells prior to surgery (Figures S8C,D). Together, these data indicate that T cells are locally activated in vein grafts upon surgery without systemic T cell activation.
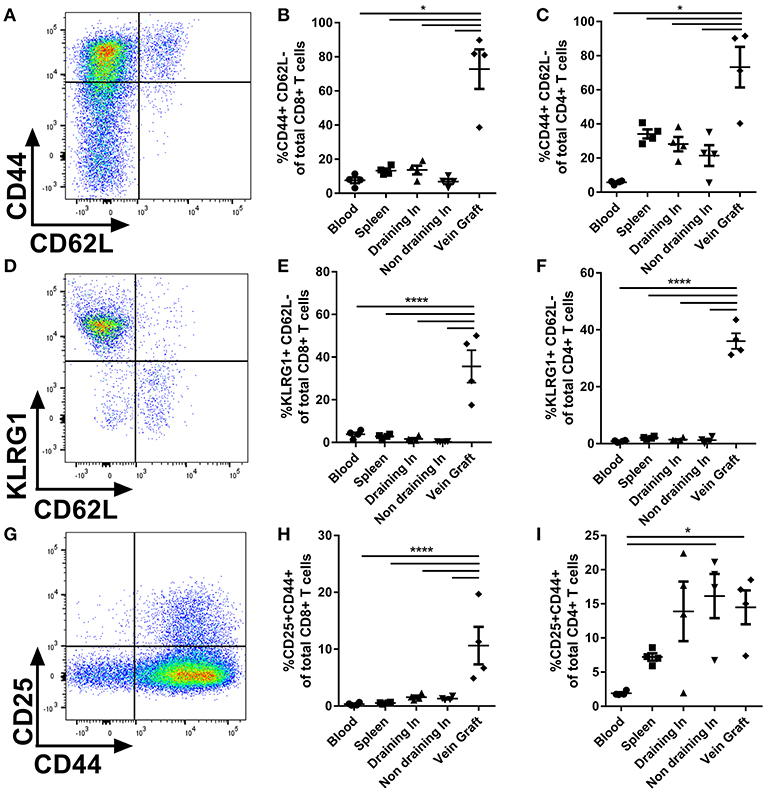
Figure 2. T cell activation in vein grafts. (A) Blood, spleen, (non) draining lymph nodes, and vein grafts of C57BL/6 male mice were analyzed with FACS. CD44 and CD62L are used to quantify the percentage of effector T cells. Example of flow cytometry plot of a vein graft sample is shown. (B) The percentage of effector CD8+ T cells (CD44+ CD62L- of total CD8+ T cells) is shown. (C) The percentage of effector CD4+ T cells (CD44+ CD62L- of total CD4+ T cells) is shown. (D) T cell activation was analyzed with KLRG1 and CD62L. Example of flow cytometry plot of a vein graft sample is shown. (E) The percentage of activated CD8+ T cells (KLRG1+ CD62L- of total CD8+ T cells) is shown. (F) The percentage of CD4+ T cells (KLRG1+ CD62L- of total CD4+ T cells) is shown. (G) T cell activation was analyzed with CD25 and CD44. Example of flow cytometry plot of a vein graft sample is shown. (H) The percentage of activated CD8+ T cells (CD25+ CD44+ of total CD8+ T cells) is shown. (I) The percentage of CD4+ T cells (CD25+ CD44+ of total CD8+ T cells) is shown. (A–I) n = 4/group. Significant differences between blood, spleen, (non) draining lymph nodes compared to vein grafts are indicated. *P < 0.05, ****P < 0.0001, one-way ANOVA and Kruskal-Wallis test.
CD8+ T Cells Are Instrumental in Preventing Vein Graft Occlusions
To investigate the particular role of CD4+ and CD8+ T cells in VGD, we depleted these subsets in mice undergoing vein graft surgery, and subsequently investigated vein graft patency. All mice were successfully depleted of either CD4+, CD8+ T cells or both in the blood, spleen, draining, and non-draining lymph node and vein graft after antibody injection at 7 and 14 days after surgery and at sacrifice (Figures S9, S10). Post-operative 28 days after surgery, in both control and CD4+ T cell depleted group 70% of the vein grafts was clearly pulsatile and patent (Figures 3A–F). A statistically significant difference in the pulsatility of the vein grafts post-operative was seen (p = 0.04), with less clearly pulsatile vein grafts in the CD8+ T cell depleted group and in the CD4/8 T cell depleted group compared to the control group (respectively, 70 vs. 10% and 70 vs. 12.5%) (Figures 3G,H). Interestingly, in the control group no vein graft were occluded compared to respectively, 50 and 37.5% in the CD8+ T cell depleted group and in the CD4/8 T cell depleted group. Thus, CD8+ T cells mediate a robust protective role in VGD. To provide insight into the mechanism of the CD8+ T cell necessity, we performed a Caspase 3 staining to identify apoptotic cells. In the patent control and CD4 T cell depleted mice, almost no cells in the vein grafts were positive for caspase 3 (Figure 4). In contrast, in the occluded CD8 T cell depleted and CD4/CD8 T cell depleted mice the vein grafts were stained abundantly for caspase 3. So, many cells in the vein grafts lacking CD8+ T cells are apoptotic, suggesting that the absence of CD8+ T cells induces apoptosis in vein grafts, which results in occluded vein grafts.
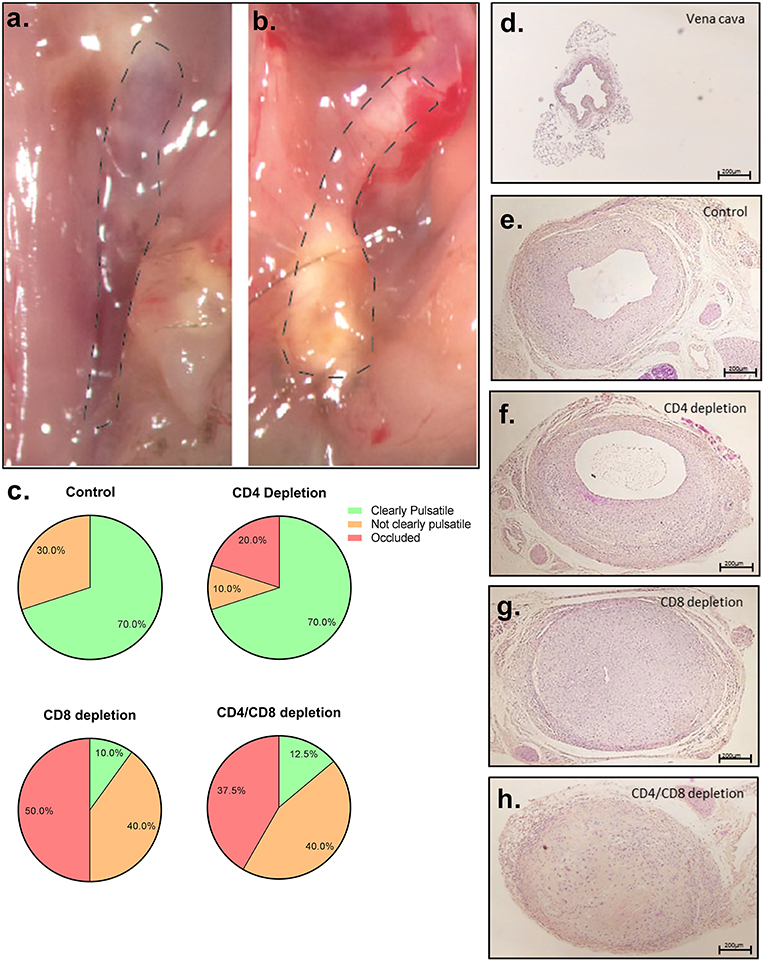
Figure 3. Vein graft patency after T cell depletion. (A) Representative picture of a clearly pulsatile vein graft, 28 days after surgery (B) and an occluded vein graft. (C) Percentage of clearly pulsatile, not clearly pulsatile and occluded vein graft is shown in control (n = 10), CD4+ T cell depleted (n = 10), CD8+ T cell depleted (n = 10), and CD4/CD8+ T cell depleted male mice (n = 8). A statistically significant difference with a Kruskal-Wallis test was seen (p = 0.04) in the pulsatility of the vein grafts postoperative, with less clearly pulsatile vein grafts in the CD8+ T cell depleted group and in the CD4/8 T cell depleted group compared to the control group (respectively, 70 vs. 10% and 70 vs. 12.5%). Representative pictures are shown of HPS stained (D) vena cava prior to operation, (E) control vein graft (F) vein graft of CD4+ T cell depleted mice (G) CD8+ T cell depleted mice and (H) CD4/CD8+ T cell depleted mice, 28 days after surgery. 5× magnification, scale bar 200 μm.
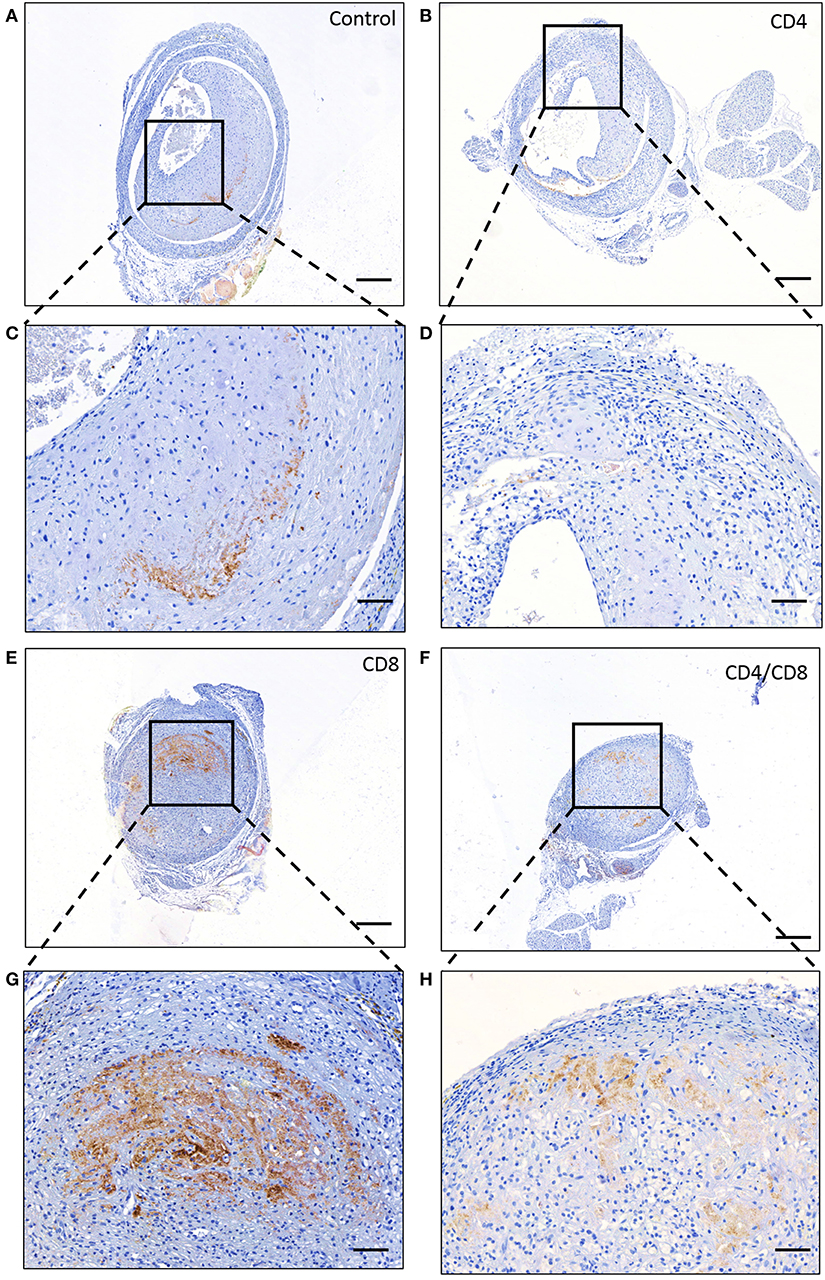
Figure 4. Apoptosis in vein grafts. Representative stainings of caspase 3 in vein grafts of (A) control mice, (B) CD4+ T cell depleted mice, (E) CD8+ T cell depleted male mice and (F) CD4/CD8+ T cell depleted mice is shown. 5× magnification, scale bar 200 μm. A zoom in of the vein graft of the concerned (C) control mice, (D) CD4+ T cell depleted mice, (G) CD8+ T cell depleted mice. (H) CD4/CD8+ T cell depleted mice is shown. 20× magnification, scale bar 50 μm.
TCR Independent T Cell Activation in Vein Grafts
Next, given the local activation of CD8+ T cells in vein grafts and the importance of this T cell subset in the prevention of vein graft occlusions, we aimed to dissect the mechanisms underlying the local stimulation of CD8+ T cells. First, we questioned whether antigenic triggering via the TCR (signal 1) is required for the protective effect of CD8+ T cells in VGD development. For this we used OT-I mice in which all CD8+ T cells bear the same TCR recognizing chicken ovalbumin, an antigen that is not present in mice. Vein graft surgery was performed in these TCR transgenic OT-I mice, and patency of the grafts and T cell activation was determined after 28 days. The vast majority of the control vein grafts (75%) were patent as well as the OT-I mice (100%), which was not statistically different (p = 0.236) (Figure 5A). Only one vein graft was occluded in the control group. Moreover, the percentage of KLRG1+CD62L–CD8+ T cells in the control and OT-I mice vein grafts were comparable, and clearly significantly increased compared to other tissues (Figure 5B). In addition, no differences in effector CD44+CD62L–CD8+ T cells between vein grafts of control mice and OT-I mice were observed (Figure 5C). Thus, in presence of a transgenic TCR, CD8+ T cells can still be activated in vein grafts, and CD8+ T cells can still mediate a protective effect in VGD development, indicating that this is TCR independent.
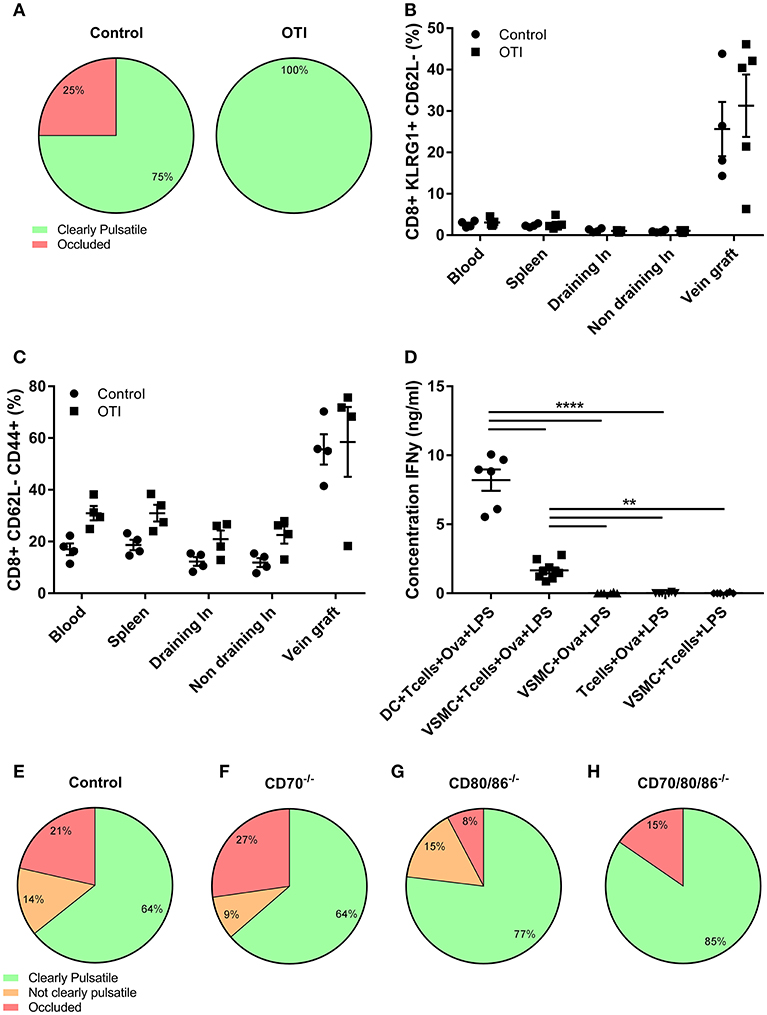
Figure 5. TCR and co-stimulation independent VGD development. (A) Vein graft patency of control and OTI male mice is shown. Percentage of clearly pulsatile and occluded vein graft is shown in control and OTI mice, not statistically different (p = 0.236). A 2-tailed Student's t-test was used. (B) The percentage of activated CD8+ T cells (CD8+ KLRG1+ CD62L-), and (C) effector CD8+ T cells (CD8+CD62L-CD44+) is shown of control and OTI mice in blood, spleen, (non) draining lymph nodes, and vein grafts. (A–C) control n = 4, OTI n = 5. Mann-Whitney test was used. (D) Production of inflammatory cytokines was measured in supernatant of DCs and VMSCs cultured with OTI T cells and Ovalbumin (Ova), stimulated with LPS for 24 h. As control groups, VSMCs were stimulated without OTI T cells or without Ovalbumin and OTI T cell with Ovalbumin were stimulated without VSMCs. The concentration of IFNγ was measured in supernatant. n = 6. **P < 0.01, ****P < 0.0001. One-way ANOVA was used. (E) vein graft patency of control (n = 11) and (F) CD70−/− (n = 12), (G) CD80/86−/− (n = 14), and (H) CD70/80/86−/− (n = 14) mice is shown 28 days after surgery. Percentage of clearly pulsatile, not clearly pulsatile and occluded vein graft is shown. A statistically significant difference was not shown (p = 0.679), Kruskal-Wallis test was used.
The lack of requirement for TCR triggering may be caused by the absence of cell types able to present antigen to CD8+ T cells. Therefore, we examined if VSMCs, a prominent cell type in vein grafts, can function as APCs to activate T cells via the TCR. VSMCs and DCs, known as professional APCs, were stimulated with LPS in absence or presence of OT-I T cells and Ovalbumin. Albeit less than DCs, VSMCs were capable of T cell activation as evidenced by induction of IFNγ production in OT-I cells (Figure 5D).
T Cell Co-stimulation Independent VGD Development
T cell co-stimulation cooperates with antigenic triggering. Since we observed that the protective effect of CD8+ T cells in VGD development is independent of TCR triggering, we investigated whether co-stimulation (signal 2) contributes to CD8+ T cell dependent VGD protection. We performed vein graft surgery in mice deficient in one or more co-stimulatory ligands; i.e., CD70−/−, CD80/86−/−, and CD80/86/70−/− mice. After 28 days, in both control and CD70−/− mice 64% of the vein grafts were clearly pulsatile and patent (Figures 5E,F) and respectively, 77 and 85% of the vein grafts of CD80/86−/− and CD80/86/70−/− mice were clearly pulsatile (Figures 5G,H). A statistically significant difference was not revealed (p = 0.679). The pulsatility of the vein grafts, was supported by histology since HPS stained vein grafts showed open grafts and comparable lumen area and total vessel area in all co-stimulation knockout and control mice vein grafts (Figure S11). Only a small decrease in vessel wall area in CD80/86/70−/− mice compared to control mice vein grafts was observed, showing that co-stimulation is not essential for CD8+ T cells to obtain a robust protective effect in vein grafts.
Cytokines Mediating Bystander CD8+ T Cell Activation Are Present in Vein Grafts
The above described TCR and co-stimulation independent effect of CD8+ T cells in VGD, suggests a role for bystander (non-antigen specific) cytokines (signal 3) of CD8+ T cells in vein grafts. Hence, we examined using the Illumina micro array, whether different cytokines which are capable to provide signals to CD8+ T cells without TCR and co-stimulation activation, are present in vein grafts. Interleukins such IL-6, IL-12a, IL-15, IL18, IL-33, and the effector cytokines TNFα and IFNγ are present and regulated in time in vein grafts after surgery (Figure 6A). Also present are interleukin receptors such as the IL-1, IL-2, and IL-15 receptor. Furthermore, the IFNα and IFNβ receptor Ifnar2 was also up-regulated as well as the Toll-like receptors TLR2 and TLR4, known to induce inflammatory cytokines and up-regulation of co-stimulatory molecules upon ligation.
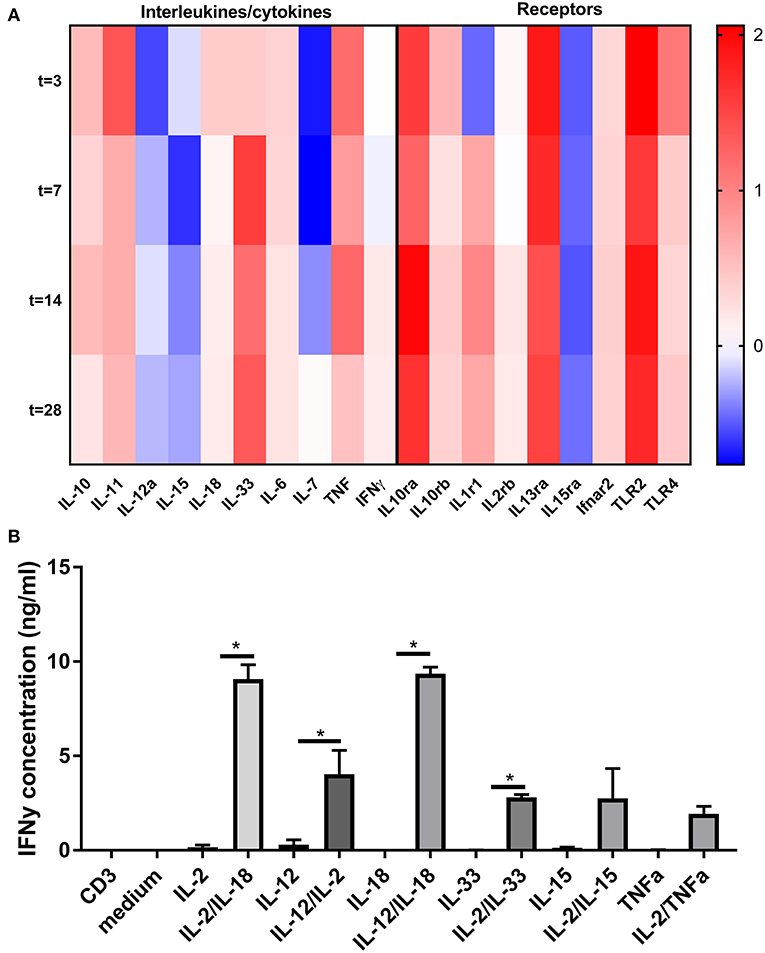
Figure 6. Bystander cytokine presence in vein grafts and contribution to CD8+ T cell activation. (A) Heat map of cytokine and cytokine receptor regulation in time in vein grafts. RT2 profiler array was performed on RNA isolated of vein grafts in male mice 3, 7, 14, and 28 days after surgery. In total two pools were analyzed (n = 3 vein grafts per pool). With the average of these two pools, the 2-ΔΔCt method was used to analyse the relative changes in gene expression. The fold change compared to the control vein graft is shown. (B) CD8+ T cells were stimulated for 24 h with agonistic CD3 antibodies, interleukins and TNFα in different combinations. Statistical tests performed compared cytokine combinations to cytokines alone. IFNγ was measured as CD8+ T cell activation marker with ELISA. *P < 0.05, One-way ANOVA was used.
To investigate CD8+ T cell bystander activation in relation to the plethora of signals present in vein grafts, single, and combinatorial effects of interleukins and co-signals from the TCR were examined ex vivo (Figure 6B). Single interleukins such as IL-2, IL-12, IL-15, IL-18, and IL-33 were not capable to elicit IFNγ secretion of CD8+ T cells significantly. Conversely, combinations of interleukins, such as IL-2/IL-18, IL-12/IL-2, IL-12/IL-18, or IL-2/IL-33 induced substantial CD8+ T cell activation as compared to interleukins alone or TCR triggering (mimicked byanti-CD3 monoclonal antibody) alone. Interestingly, CD8+ T cell bystander activation was stronger compared to CD4+ T cell bystander activation (Figure S12). Single interleukins or combinations of interleukins, such as IL-2/IL-18, IL-12/IL-2, or IL-2/IL-33 did not induce IFNγ secretion by CD4+ T cells. Mainly a combination of IL-12 and IL-18 induced CD4+ T cell activation. Collectively, these data indicate that bystander cytokines are present in vein grafts, and can collectively activate CD8+ T cells.
Discussion
In the current study we show that T cells are modulators of VGD with a specific protective role of CD8+ T cells. Both CD4+ and CD8+ T cells are present in vein grafts and locally activated without systemic activation. Depletion of CD8+ T cells resulted in fully occluded vein grafts, and this was associated with increased apoptosis. Remarkably, the protective effect of CD8+ T cells in vein grafts was TCR and co-stimulation independent. However, bystander signals provided via cytokines can, in a collective manner, activate CD8+ T cells. Importantly, these cytokines are abundantly present in vein grafts.
Previous studies showed that CD8+ T cells modulate cardiovascular diseases (30) and are present in atherosclerotic lesions (31), however, others showed that CD8+ T cells are not functional in atherosclerosis (32, 33). Recent studies have described a role for CD8+ T cells in atherosclerosis, either pro-atherogenic or anti-atherogenic (10). Pro-atherogenic effects of CD8+ T cells (11, 34) have been revealed via their impact on circulating monocyte levels though increased CCL2 and VCAM1 expression, leading to macrophage accumulation in the arterial intima promoting atherosclerotic lesion formation (34). This is in distinction with our results of occlusive vein grafts after depletion of CD8+ T cells. Anti-atherogenic effects of CD8+ T cells have been shown after arterial injury in mice (35). All pro-atherogenic studies described above showed no effect in CD4+ T cell levels after CD8+ T cell depletion, indicating that there is no compensation mechanism of the CD8+ T cell loss by CD4+ T cells. This is in line with our observations in both CD4+ and CD8+ T cell depleted mice where no compensation mechanism after depletion of a T cell subset was observed.
A striking impact of CD8+ T cells was apparent since vein grafts of CD8 T cell depleted mice were occluded. Previous studies showed that CD8+ T cells play a role in anti-tumor immunity (36, 37) and immune checkpoint inhibitors can be effective in the activation of CD8+ T cell responses and can restore anti-tumor immune responses (38). CD8+ T cells in tumors can function via direct and indirect tumor killing. CD8+ T cells are described to recognize target cells via the expression of antigens, which causes direct tumor killing. After recognition, they kill target cells by apoptosis, via the release of perforin and granzymes out of cytotoxic granules. However, CD8+ T cells in vein grafts are activated in an antigen independent manner, which is more similar to indirect tumor killing. Via the release of cytokines, CD8+ T cells can indirectly kill the tumor cells (39, 40). Presence of CD8+ T cells in the tumor environment is associated with beneficial clinical outcomes in several tumor types (37). However, in the studies described above, not only effector CD8+ T cells are described, but also memory CD8 T+ cells that have been previously activated by their cognate antigen are described as indirect tumor killers. Here we observed that many cells in the CD8+ T cell depleted vein grafts and CD4/CD8+ T cells depleted vein grafts are apoptotic, suggesting that the absence of CD8+ T cells induces apoptosis in vein grafts, which results in occluded vein grafts. Thus, CD8 T cells may protect against occlusive lesions by providing survival signals in vein grafts. In addition, CD8+ regulatory T cells are also part of the CD8+ T cell subset, although in small subsets, which possess important immunosuppressive functions. However, in this study we did not specifically describe this T cell subset, but CD8+ regulatory T cells might be of great value in the protection of VGD by secreting various inhibitory cytokines and chemokines (41).
We questioned whether the protective CD8+ T cell effect was TCR and co-stimulation dependent. In presence of a transgenic TCR, specific for an antigen that is not present in mice, we showed that CD8+ T cells are still activated in vein grafts. Furthermore, vein grafts of OT-I mice were clearly pulsatile, which suggests that the CD8+ T cells can still mediate their protective effect in VGD development, and indicates that the protective CD8+ T cell is TCR independent. In vivo we showed no effects of the co-stimulation pathways CD27-CD70 and CD28-CD80/CD86 in VGD development. We showed that vein grafts occlude in CD8+ T cell depleted mice, but in the presence of activated CD8+ T cells, vein grafts are clearly pulsatile. Deficiency of one or both co-stimulatory pathways did not lead to a reduction in vein graft pulsatility, which indicates that the protective effect of CD8+ T cells in VGD development is co-stimulation independent. Interestingly, the vein graft vessel wall area was decreased in CD70/80/86−/− mice compared to control mice, suggesting that the protective CD8+ T cell response is stronger than the CD4+ T cell effect. In Figure 6 and Figure S10 we showed that CD8+ T cells can be activated via a plethora of bystander cytokines, in contrast to CD4+ T cells. With the lack of co-stimulation molecules, CD8+ T cells can still be activated via bystander cytokines and may explain the strong CD8+ T cell effect.
We here showed that T cells present in vein grafts are locally activated. Even in the draining lymph nodes, located close to the vein grafts, no activated T cells were detected. The CD8+ T cell activation in vein grafts was found to be TCR independent. In infection models it is clear that TCR triggering is, by definition, essential to activate antigen-specific T cells. T cell activation, however, can also occur in absence of the TCR (42–47). TCR independent CD8+ T cell activation, allows CD8+ T cells to modulate e.g., non-pathogen specific diseases such as VGD, where a vein graft is placed in a sterile environment (18). Here, we describe TCR independent T cell activation in vein grafts of mice, and propose that CD8+ T cell activation in vein grafts is due to bystander signals. Certain cytokines can induce bystander proliferation and activation of CD8+ T cells (18, 48). Especially IL-12 and IL-18 are key regulators of CD8+ T cell activation (49, 50). IFNγ leads to production of IL-12, IL-18, and especially IL-15, which can activate CD8+ T cells (48, 51). CD4+ T cells are mainly activated via a combination of IL12 and IL18. Interestingly, as shown in the heatmap of Figure 6A, IL33 and TNFα are the highest expressed cytokines in vein grafts. However, IL15, IL33, and TNFα could not activate CD4+ T cells (Figure S12), in contrast to CD8+ T cells (Figure 6B). Type I interferons, IFNα, and IFNβ also showed to enhance IFNγ production, synergistically with IL-18 in human T cells (52). In contradiction, CD8+ T cell inhibition via type I Interferons was shown via suppression of the IL-12 pathway (53). Several other cytokines, including IL-1β and TNF-α, can enhance IFN-γ production in CD8+ T cells (18, 54). IL-33 is mainly expressed by Th2 cells and mast cell, but IL-33 has also been shown to enhance TCR independent virus specific CD8+ T cell activation (18, 55). However, in line with our results, these cytokines do not activate CD8+ T cells individually, but combinations of cytokines are functional and show synergic effects.
However, it should be taken in to account that VGD is a complex progress involving multiple components, besides CD8+ T cells. As indicated, non-T cells including macrophages, DCs and B cells represent around 75% of the graft infiltrate. It was previously shown that these cells contribute to the vein graft outcome. In the current study we showed a protective effect of CD8+ T cells in VGD via bystander cytokines. However, this effect can be either directly via bystander cytokines or indirectly via the activation of innate immune cells via bystander cytokines. In this study, we mainly focused on the direct effect of CD8+ T cells. However, in the pathophysiology of VGD, both innate and immune cells are involved.
In conclusion, we here show that T cells play a role in VGD, with a specific protective role of CD8+ T cells. The protective effect of CD8+ T cells in vein grafts is TCR and co-stimulation independent. Bystander cytokines are abundantly present in vein grafts and have the capacity to activate CD8+ T cells in a collective manner independent of TCR and co-stimulation signals. This gives rise to potential new strategies to use T cell bystander cytokines in a therapeutic approach to prevent VGD.
Data Availability
All datasets generated for this study are included in the manuscript and/or the Supplementary Files.
Ethics Statement
This study was performed in compliance with Dutch government guidelines and the Directive 2010/63/EU of the European Parliament. All animal experiments were approved by the animal welfare committee of the Leiden University Medical Center.
Author Contributions
KS, HP, MV, JJ, PQ, and RA: designing research studies. KS, HP, and RA: conducting experiments. KS and HP: acquiring data. KS, RA, MV, JJ, and PQ: analyzing data. RA, JJ, and PQ: providing reagents. KS, RA, PQ, and MV: writing the manuscript.
Conflict of Interest Statement
The authors declare that the research was conducted in the absence of any commercial or financial relationships that could be construed as a potential conflict of interest.
Acknowledgments
The authors thank Dennis Gout for technical assistance.
Supplementary Material
The Supplementary Material for this article can be found online at: https://www.frontiersin.org/articles/10.3389/fcvm.2019.00077/full#supplementary-material
References
1. de Vries MR, Simons KH, Jukema JW, Braun J, Quax PHA. Vein graft failure: from pathophysiology to clinical outcomes. Nat Rev Cardiol. (2016) 13:451–70. doi: 10.1038/nrcardio.2016.76
2. de Vries MR, Quax PHA. Inflammation in vein graft disease. Front Cardiovasc Med. (2018) 5:3. doi: 10.3389/fcvm.2018.00003
3. Hansson GK, Jonasson L. The discovery of cellular immunity in the atherosclerotic plaque. Arterioscler Thromb Vasc Biol. (2009) 29:1714–7. doi: 10.1161/ATVBAHA.108.179713
4. Hansson GK, Hermansson A. The immune system in atherosclerosis. Nat Immunol. (2011) 12:204–12. doi: 10.1038/ni.2001
5. Ait-Oufella H, Salomon BL, Potteaux S, Robertson AK, Gourdy P, Zoll J, et al. Natural regulatory T cells control the development of atherosclerosis in mice. Nat Med. (2006) 12:178–80. doi: 10.1038/nm1343
6. Tedgui A, Mallat Z. Cytokines in atherosclerosis: pathogenic and regulatory pathways. Physiol Rev. (2006) 86:515–81. doi: 10.1152/physrev.00024.2005
7. Koltsova EK, Garcia Z, Chodaczek G, Landau M, McArdle S, Scott SR, et al. Dynamic T cell-APC interactions sustain chronic inflammation in atherosclerosis. J Clin Invest. (2012) 122:3114–26. doi: 10.1172/JCI61758
8. Engelbertsen D, Rattik S, Wigren M, Vallejo J, Marinkovic G, Schiopu A, et al. IL-1R and MyD88 signaling in CD4+ T cells promote Th17 immunity and atherosclerosis. Cardiovasc Res. (2017) 114:180–7. doi: 10.1093/cvr/cvx196
9. Grivel JC, Ivanova O, Pinegina N, Blank PS, Shpektor A, Margolis LB, et al. Activation of T lymphocytes in atherosclerotic plaques. Arterioscler Thromb Vasc Biol. (2011) 31:2929–37. doi: 10.1161/ATVBAHA.111.237081
10. Cochain C, Zernecke A. Protective and pathogenic roles of CD8+ T cells in atherosclerosis. Basic Res Cardiol. (2016) 111:71. doi: 10.1007/s00395-016-0589-7
11. Kyaw T, Winship A, Tay C, Kanellakis P, Hosseini H, Cao A, et al. Cytotoxic and proinflammatory CD8+ T lymphocytes promote development of vulnerable atherosclerotic plaques in apoE-deficient mice. Circulation. (2013) 127:1028–39. doi: 10.1161/CIRCULATIONAHA.112.001347
12. Sharpe AH, Freeman GJ. The B7-CD28 superfamily. Nat Rev Immunol. (2002) 2:116–26. doi: 10.1038/nri727
13. Simons KH, de Jong A, Jukema JW, de Vries MR, Arens R, Quax PHA. T cell co-stimulation and co-inhibition in cardiovascular disease: a double-edged sword. Nat Rev Cardiol. (2019) 16:325–43. doi: 10.1038/s41569-019-0164-7
14. Chen L, Flies DB. Molecular mechanisms of T cell co-stimulation and co-inhibition. Nat Rev Immunol. (2013) 13:227–42. doi: 10.1038/nri3405
15. Denoeud J, Moser M. Role of CD27/CD70 pathway of activation in immunity and tolerance. J Leukoc Biol. (2011) 89:195–203. doi: 10.1189/jlb.0610351
16. Tesselaar K, Xiao Y, Arens R, van Schijndel GM, Schuurhuis DH, Mebius RE, et al. Expression of the murine CD27 ligand CD70 in vitro and in vivo. J Immunol. (2003) 170:33–40. doi: 10.4049/jimmunol.170.1.33
17. Arens R, Baars PA, Jak M, Tesselaar K, van der Valk M, van Oers MH, et al. Cutting edge: CD95 maintains effector T cell homeostasis in chronic immune activation. J Immunol. (2005) 174:5915–20. doi: 10.4049/jimmunol.174.10.5915
18. Freeman BE, Hammarlund E, Raue HP, Slifka MK. Regulation of innate CD8+ T-cell activation mediated by cytokines. Proc Natl Acad Sci USA. (2012) 109:9971–6. doi: 10.1073/pnas.1203543109
19. Cherian SM, Bobryshev YV, Liang H, Inder SJ, Wang AY, Lord RS, et al. Immunohistochemical and ultrastructural evidence that dendritic cells infiltrate stenotic aortocoronary saphenous vein bypass grafts. Cardiovasc Surg. (2001) 9:194–200. doi: 10.1016/S0967-2109(00)00102-2
20. Mosquera JA. Interferon-gamma induces class II antigen expression on cultured rat mesangial smooth muscle cells. Clin Immunol Immunopathol. (1989) 53(Pt 1):341–5. doi: 10.1016/0090-1229(89)90062-7
21. Pober JS, Tellides G. Participation of blood vessel cells in human adaptive immune responses. Trends Immunol. (2012) 33:49–57. doi: 10.1016/j.it.2011.09.006
22. Welten SP, Redeker A, Franken KL, Oduro JD, Ossendorp F, Cicin-Sain L, et al. The viral context instructs the redundancy of costimulatory pathways in driving CD8+ T cell expansion. eLife. (2015) 4:e07486. doi: 10.7554/eLife.07486
23. Welten SP, Redeker A, Franken KL, Benedict CA, Yagita H, Wensveen FM, et al. CD27–CD70 costimulation controls T cell immunity during acute and persistent cytomegalovirus infection. J Virol. (2013) 87:6851–65. doi: 10.1128/JVI.03305-12
24. Miyagawa F, Gutermuth J, Zhang H, Katz SI. The use of mouse models to better understand mechanisms of autoimmunity and tolerance. J Autoimmun. (2010) 35:192–8. doi: 10.1016/j.jaut.2010.06.007
25. de Vries MR, Wezel A, Schepers A, van Santbrink PJ, Woodruff TM, Niessen HW, et al. Complement factor C5a as mast cell activator mediates vascular remodeling in vein graft disease. Cardiovasc Res. (2013) 97:311–20. doi: 10.1093/cvr/cvs312
26. van der Pouw Kraan TC, van der Laan AM, Piek JJ, Horrevoets AJ. Surfing the data tsunami, a bioinformatic dissection of the proangiogenic monocyte. Vasc Pharmacol. (2012) 56:297–305. doi: 10.1016/j.vph.2012.02.008
27. Simons KH, de Vries MR, Peters HAB, Hamming JF, Jukema JW, Quax PHA. The protective role of Toll-like receptor 3 and type-I interferons in the pathophysiology of vein graft disease. J Mol Cell Cardiol. (2018) 121:16–24. doi: 10.1016/j.yjmcc.2018.06.001
28. Chappell J, Harman JL, Narasimhan VM, Yu H, Foote K, Simons BD, et al. Extensive proliferation of a subset of differentiated, yet plastic, medial vascular smooth muscle cells contributes to neointimal formation in mouse injury and atherosclerosis models. Circul Res. (2016) 119:1313–23. doi: 10.1161/CIRCRESAHA.116.309799
29. de Jong RCM, Ewing MM, de Vries MR, Karper JC, Bastiaansen AJNM, Peters HAB, et al. The epigenetic factor PCAF regulates vascular inflammation and is essential for intimal hyperplasia development. PLoS ONE. (2017) 12:e0185820. doi: 10.1371/journal.pone.0185820
30. Kolbus D, Ljungcrantz I, Andersson L, Hedblad B, Fredrikson GN, Bjorkbacka H, et al. Association between CD8+ T-cell subsets and cardiovascular disease. J Intern Med. (2013) 274:41–51. doi: 10.1111/joim.12038
31. Jonasson L, Holm J, Skalli O, Bondjers G, Hansson GK. Regional accumulations of T cells, macrophages, and smooth muscle cells in the human atherosclerotic plaque. Arteriosclerosis. (1986) 6:131–8. doi: 10.1161/01.ATV.6.2.131
32. Legein B, Janssen EM, Theelen TL, Gijbels MJ, Walraven J, Klarquist JS, et al. Ablation of CD8α+ dendritic cell mediated cross-presentation does not impact atherosclerosis in hyperlipidemic mice. Sci Rep. (2015) 5:15414. doi: 10.1038/srep15414
33. Elhage R, Gourdy P, Brouchet L, Jawien J, Fouque MJ, Fievet C, et al. Deleting TCR alpha beta+ or CD4+ T lymphocytes leads to opposite effects on site-specific atherosclerosis in female apolipoprotein E-deficient mice. Am J Pathol. (2004) 165:2013–8. doi: 10.1016/S0002-9440(10)63252-X
34. Cochain C, Koch M, Chaudhari SM, Busch M, Pelisek J, Boon L, et al. CD8+ T Cells regulate monopoiesis and circulating Ly6C-high monocyte levels in atherosclerosis in mice. Circ Res. (2015) 117:244–53. doi: 10.1161/CIRCRESAHA.117.304611
35. Dimayuga PC, Chyu KY, Kirzner J, Yano J, Zhao X, Zhou J, et al. Enhanced neointima formation following arterial injury in immune deficient Rag-1−/− mice is attenuated by adoptive transfer of CD8 T cells. PLoS ONE. (2011) 6:e20214. doi: 10.1371/journal.pone.0020214
36. Yang W, Bai Y, Xiong Y, Zhang J, Chen S, Zheng X, et al. Potentiating the antitumour response of CD8(+) T cells by modulating cholesterol metabolism. Nature. (2016) 531:651–5. doi: 10.1038/nature17412
37. Fridman WH, Pages F, Sautes-Fridman C, Galon J. The immune contexture in human tumours: impact on clinical outcome. Nat Rev Cancer. (2012) 12:298–306. doi: 10.1038/nrc3245
38. Chen L, Han X. Anti-PD-1/PD-L1 therapy of human cancer: past, present, and future. J Clin Invest. (2015) 125:3384–91. doi: 10.1172/JCI80011
39. Andersen MH, Schrama D, Thor Straten P, Becker JC. Cytotoxic T cells. J Invest Dermatol. (2006) 126:32–41. doi: 10.1038/sj.jid.5700001
40. Zhang N, Bevan MJ. CD8(+) T cells: foot soldiers of the immune system. Immunity. (2011) 35:161–8. doi: 10.1016/j.immuni.2011.07.010
41. Yu Y, Ma X, Gong R, Zhu J, Wei L, Yao J. Recent advances in CD8(+) regulatory T cell research. Oncol Lett. (2018) 15:8187–94. doi: 10.3892/ol.2018.8378
42. Lau LL, Jamieson BD, Somasundaram T, Ahmed R. Cytotoxic T-cell memory without antigen. Nature. (1994) 369:648–52. doi: 10.1038/369648a0
43. Hou S, Hyland L, Ryan KW, Portner A, Doherty PC. Virus-specific CD8+ T-cell memory determined by clonal burst size. Nature. (1994) 369:652–4. doi: 10.1038/369652a0
44. Mullbacher A. The long-term maintenance of cytotoxic T cell memory does not require persistence of antigen. J Exp Med. (1994) 179:317–21. doi: 10.1084/jem.179.1.317
45. Swain SL, Hu H, Huston G. Class II-independent generation of CD4 memory T cells from effectors. Science. (1999) 286:1381–3. doi: 10.1126/science.286.5443.1381
46. Murali-Krishna K, Lau LL, Sambhara S, Lemonnier F, Altman J, Ahmed R. Persistence of memory CD8 T cells in MHC class I-deficient mice. Science. (1999) 286:1377–81. doi: 10.1126/science.286.5443.1377
47. Tanchot C, Lemonnier FA, Perarnau B, Freitas AA, Rocha B. Differential requirements for survival and proliferation of CD8 naive or memory T cells. Science. (1997) 276:2057–62. doi: 10.1126/science.276.5321.2057
48. Tough DF, Zhang X, Sprent J. An IFN-gamma-dependent pathway controls stimulation of memory phenotype CD8+ T cell turnover in vivo by IL-12, IL-18, and IFN-gamma. J Immunol. (2001) 166:6007–11. doi: 10.4049/jimmunol.166.10.6007
49. Raue HP, Brien JD, Hammarlund E, Slifka MK. Activation of virus-specific CD8+ T cells by lipopolysaccharide-induced IL-12 and IL-18. J Immunol. (2004) 173:6873–81. doi: 10.4049/jimmunol.173.11.6873
50. Berg RE, Cordes CJ, Forman J. Contribution of CD8+ T cells to innate immunity: IFN-gamma secretion induced by IL-12 and IL-18. Eur J Immunol. (2002) 32:2807–16. doi: 10.1002/1521-4141(2002010)32:10<2807::AID-IMMU2807>3.0.CO;2-0
51. Zhang X, Sun S, Hwang I, Tough DF, Sprent J. Potent and selective stimulation of memory-phenotype CD8+ T cells in vivo by IL-15. Immunity. (1998) 8:591–9. doi: 10.1016/S1074-7613(00)80564-6
52. Sareneva T, Matikainen S, Kurimoto M, Julkunen I. Influenza A virus-induced IFN-alpha/beta and IL-18 synergistically enhance IFN-gamma gene expression in human T cells. J Immunol. (1998) 160:6032–8.
53. Nguyen KB, Cousens LP, Doughty LA, Pien GC, Durbin JE, Biron CA. Interferon alpha/beta-mediated inhibition and promotion of interferon gamma: STAT1 resolves a paradox. Nat Immunol. (2000) 1:70–6. doi: 10.1038/76940
54. Hunter CA, Chizzonite R, Remington JS. IL-1 beta is required for IL-12 to induce production of IFN-gamma by NK cells. A role for IL-1 beta in the T cell-independent mechanism of resistance against intracellular pathogens. J Immunol. (1995) 155:4347–54.
Keywords: T cell, co-stimulation, CD8+ activation, vein graft disease, vein graft failure, TCR–T cell receptor, costimulatary pathway
Citation: Simons KH, de Vries MR, Peters HAB, Jukema JW, Quax PHA and Arens R (2019) CD8+ T Cells Protect During Vein Graft Disease Development. Front. Cardiovasc. Med. 6:77. doi: 10.3389/fcvm.2019.00077
Received: 25 March 2019; Accepted: 28 May 2019;
Published: 13 June 2019.
Edited by:
Paul Evans, University of Sheffield, United KingdomReviewed by:
Beatrice Charreau, Université de Nantes, FranceXiaofeng Yang, Temple University, United States
Copyright © 2019 Simons, de Vries, Peters, Jukema, Quax and Arens. This is an open-access article distributed under the terms of the Creative Commons Attribution License (CC BY). The use, distribution or reproduction in other forums is permitted, provided the original author(s) and the copyright owner(s) are credited and that the original publication in this journal is cited, in accordance with accepted academic practice. No use, distribution or reproduction is permitted which does not comply with these terms.
*Correspondence: Paul H. A. Quax, cC5oLmEucXVheEBsdW1jLm5s
†These authors have contributed equally to this work