- 1IRSD, Université de Toulouse, INSERM, INRA, ENVT, UPS, Toulouse, France
- 2Service de Bactériologie-Hygiène, Hôpital Purpan, CHU de Toulouse, Toulouse, France
Siderophore-microcins are antimicrobial peptides produced by enterobacteria, especially Escherichia coli and Klebsiella pneumoniae strains. The antibiotic peptide is post-translationally modified by the linkage of a siderophore moiety. Therefore, it can enter and kill phylogenetically related bacteria by a “Trojan Horse” stratagem, by mimicking the iron–siderophore complexes. Consequently, these antimicrobial peptides are key determinants of bacterial competition within the intestinal niche, which is the reservoir for pathogenic E. coli. The most frequent extraintestinal infections caused by E. coli are urinary tract infections. Uropathogenic E. coli (UPEC) can produce many virulence factors, including siderophore-microcins. Siderophore-microcins are chromosomally encoded by small genomic islands that exhibit conserved organization. In UPEC, the siderophore-microcin gene clusters and biosynthetic pathways differ from the “archetypal” models described in fecal strains. The gene cluster is shorter. Thus, active siderophore-microcin production requires proteins from two other genomic islands that also code for virulence factors. This functional and modular synergy confers a strong selective advantage for the domination of the colonic niche, which is the first step toward infection. This optimization of genetic resources might favor the selection of additional virulence factors, which are essential in the subsequent steps of pathogenesis in E. coli infection.
Introduction
Escherichia coli lives as a commensal in the gut of warm-blooded animals (Tenaillon et al., 2010). It is also a major pathogen that causes intestinal and extraintestinal diseases, such as neonatal meningitis, nosocomial pneumonia, and bacteremia (Johnson and Russo, 2002; Kaper et al., 2004). The most common extraintestinal E. coli infections are urinary tract infections (UTIs) caused by uropathogenic E. coli (UPEC; Kaper et al., 2004; Flores-Mireles et al., 2015). UTIs are a serious public health issue in terms of morbidity, mortality, selection of antibiotic-resistant bacteria, and health care costs (Flores-Mireles et al., 2015).
E. coli strains are divided into eight phylogroups: A, B1, B2, C, D, E, F, and G (Tenaillon et al., 2010; Chaudhuri and Henderson, 2012; Clermont et al., 2019). Most extraintestinal pathogenic E. coli, including UPEC, belong to the E. coli phylogenetic group B2 (Picard et al., 1999; Johnson and Russo, 2002; Jaureguy et al., 2008; Tourret and Denamur, 2016). The reservoir of UPEC is gut microbiota, and E. coli from the B2 phylogroup are now the most frequently recovered in human fecal samples in industrialized countries (Touchon et al., 2009; Tenaillon et al., 2010). They produce more virulence factors than strains from other phylogroups (Picard et al., 1999), especially siderophore-microcins (Mcc; Budič et al., 2011; Micenková et al., 2016; Massip et al., 2020).
Mcc are antimicrobial peptides with a molecular mass below 10 kDa, produced by enterobacteria (Asensio et al., 1976; Pons et al., 2002). Siderophore-Mcc constitute a subclass of Mcc characterized by their molecular masses (between 5 and 10 kDa) and their post-translational modifications. They are post-translationally linked with a siderophore moiety derived from enterobactin (Azpiroz and Laviña, 2004; Thomas et al., 2004). Enterobactin is an iron uptake system produced by a non-ribosomal peptide synthetase (NRPS) pathway (for reviews, see Fischbach et al., 2006; Miethke and Marahiel, 2007).
Four siderophore-Mcc have been described so far: MccE492, MccH47, MccI47, and MccM. MccE492 is produced by Klebsiella pneumoniae and was first described in human fecal strain RYC492 (de Lorenzo and Pugsley, 1985). MccH47 was initially studied in human fecal strain E. coli H47 (Laviña et al., 1990). Like MccM, it is synthesized by E. coli fecal strains Nissle 1917, CA46, and CA58 (Davies and Reeves, 1975; Patzer, 2003; Vassiliadis et al., 2010) but also by many UPEC strains (Azpiroz et al., 2009; Šmajs et al., 2010; Abraham et al., 2012; Massip et al., 2020). MccI47 has only been mentioned in one study that focuses on the genetic systems that encode Mcc in E. coli strains H47 and CA46 (Poey et al., 2006).
In this review, we provide an overview of the current knowledge concerning siderophore-Mcc genetic systems and biosynthesis, their mechanisms of action, and their biological relevance in E. coli.
Siderophore-Mcc Gene Clusters, Small Genomic Islands
The overall organization of siderophore-Mcc gene clusters is conserved. The G+C contents of siderophore-Mcc gene clusters are significantly lower (from 33 to 43%) than those of their host E. coli (51%) or K. pneumoniae (57.5%), which indicates that they might have been acquired from different bacteria by horizontal transfer (Duquesne et al., 2007). Moreover, siderophore-Mcc gene clusters are generally flanked by direct repeats described as acting as attachment sites in genomic islands (Azpiroz et al., 2011). Genomic islands are mobile genetic elements that help in the adaptation to a given environment. They contribute to the evolution of bacteria and undergo repeated rearrangements, deletions, and insertions (Dobrindt et al., 2010). Azpiroz et al. (2011) demonstrated that the MccH47 genetic system can be mobilized by site-specific recombination. Therefore, it was suggested that the siderophore-Mcc gene cluster is a small genomic island (Azpiroz et al., 2011).
Genes responsible for siderophore-Mcc biosynthesis and export are gathered in large clusters (up to 13 kb) on the bacterial chromosome (Figure 1). The minimal structure comprises the gene that encodes the Mcc precursor peptide and the self-immunity gene, which are grouped in an operon, and genes responsible for the export of the siderophore-Mcc. Genes mceA, mchB, mchS2, and mcmA encode precursor peptides of 103, 75, 77, and 92 amino acids for MccE492, MccH47, MccI47, and MccM, respectively (Gaggero et al., 1993; Lagos et al., 2001; Poey et al., 2006; Duquesne et al., 2007). The mechanism of self-immunity to siderophore-Mcc remains largely elusive. MccE492, MccH47, MccI47, and MccM have specific self-immunity proteins. Studies of their sequence indicate that they are probably membrane-bound, with two or three transmembrane helices (Rodríguez et al., 1999; Lagos et al., 2001; Duquesne et al., 2007).
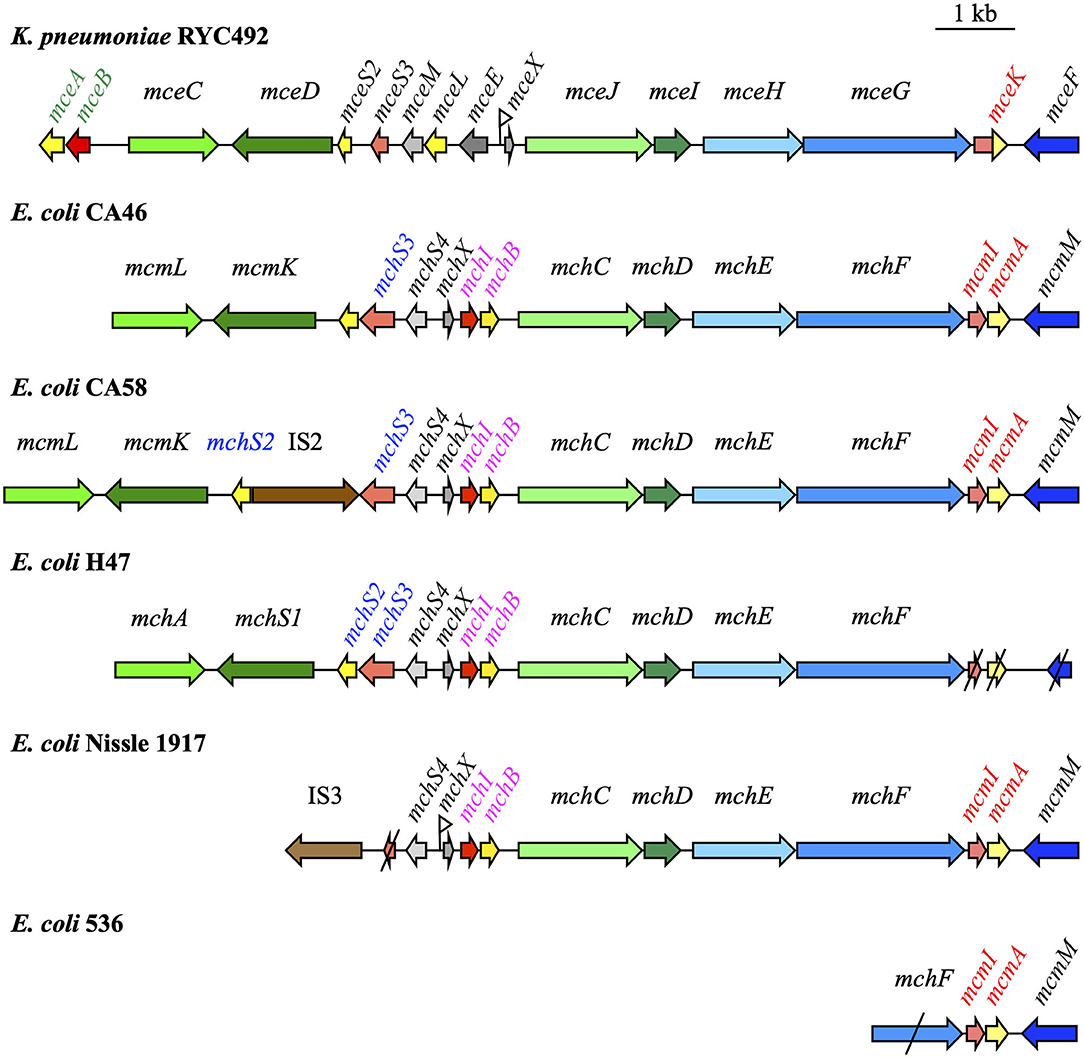
Figure 1. Microcin (Mcc) gene clusters in Klebsiella pneumoniae RYC492, Escherichia coli CA46, CA58, H47, Nissle 1917, and 536 [modified from Massip et al. (2019)]. Mcc precursor genes, immunity genes, and genes involved in Mcc export and post-translational modifications are indicated in yellow, red, blue, and green, respectively. Truncated genes are shown with slashes. The names of the genes specifically involved in MccE492, MccM, MccH47, and MccI47 are in green, red, pink, and blue, respectively. Fur boxes proved important in the regulation of Mcc production are marked by a small flag.
In general, the siderophore-Mcc gene cluster also bears genes that encode post-translational modifications enzymes (Poey et al., 2006; Duquesne et al., 2007). When several siderophore-Mcc are encoded by a single gene cluster (e.g., MccH47 and MccM in E. coli Nissle), genes responsible for post-translational modifications and export can be shared (Poey et al., 2006). Genes involved in post-translational modifications mceC, mchA, and mcmL are homologous to iroB encoding a glycosyltransferase from the salmochelin genetic system iroA (Lagos et al., 2001; Patzer, 2003). Salmochelins are siderophores derived from enterobactin through glycosylation (Fischbach et al., 2006). Similarly, mceD, mchS1, and mcmK are homologous to iroD encoding an enterobactin esterase (Lagos et al., 2001; Patzer, 2003). Proteins MceI, MceJ, and their homologs MchC, MchD link the Mcc precursor peptides with the siderophore moiety derived from enterobactin, but the precise function of these proteins remains unknown (Nolan and Walsh, 2008).
Siderophore-Mcc are transported outside the producing bacteria by a three-component ATP-binding cassette (ABC) exporter. Two components are encoded as an operon on the Mcc gene cluster: the ABC transporter protein (MchF for MccH47 and MccM, MceG for MccE492) and the accessory protein of the membrane fusion protein (MFP) family (MchE for MccH47 and MccM, MceH for MccE492). These proteins are highly similar with 92 and 94% identical amino acids between the two types of ABC and MFP proteins, respectively. The third component of this export system is the outer membrane protein TolC (Azpiroz et al., 2001; Lagos et al., 2001; Patzer, 2003).
Although the general organization of the siderophore-Mcc island is conserved, substantial differences can be found between strains. Traces of recombination events can be detected at the center of siderophore-Mcc gene clusters. In E. coli strain CA58, genes encoding transposases are inserted between genes mchS2 and mchS3 encoding MccI47 precursor and immunity peptides. Since these two genes are normally organized in an operon, the translation process is compromised, at least for mchS2, which is downstream of the insertion sequence. Consequently, unlike strain CA46, E. coli CA58 does not produce MccI47 (Patzer, 2003; Poey et al., 2006; Vassiliadis et al., 2010).
In UPEC strain 536, the siderophore-Mcc island has undergone considerable changes if strain CA46 is considered as a reference. Only four genes remain: genes encoding MccM precursor and immunity peptides, and two genes involved in Mcc export, mcmM and mchF, which are truncated. In the absence of a functional export system (association between ABC and MFP proteins), strain 536 might not be able to secrete siderophore-Mcc.
Finally, in E. coli Nissle and in the UPEC strains CFT073 and ABU83972, the island is truncated in the 5′ region. Compared to the strain CA46 island, it is deprived of the MccI47 precursor peptide and self-immunity genes, as well as post-translational modification genes mcmL and mcmK (Patzer, 2003; Poey et al., 2006). In strains bearing such a truncated island, genes encoding transposases flank the 5′ region as remnants of recombination events (Patzer, 2003). In the three E. coli strains Nissle, CFT073, and ABU83972, the truncated Mcc gene cluster is located on the genomic island I that covers the serX tRNA locus [for reviews, see Dobrindt et al. (2004, 2010)]. This genomic island also carries the iroA locus with the mcmL and mcmK homologs iroB and iroD. It is highly conserved in the three strains and in the Mcc gene cluster in particular, with 100% similarity (Grozdanov et al., 2004; Vejborg et al., 2010; Reister et al., 2014). Therefore, Poey et al. (2006) suggested considering the siderophore-Mcc gene cluster as “an islet inside an island.” It provides a selective advantage, is acquired by horizontal transfer, and can undergo significant rearrangements.
Biosynthesis of Siderophore-Mcc and Mode of Action
Siderophore-Mcc biosynthesis begins with the production of two separate moieties: (1) a precursor peptide encoded by the Mcc island and (2) enterobactin by an NRPS pathway (Figure 2A). Glycosyltransferases MceC, MchA, or McmL catalyze the transfer of one glucose to the 2,3-dihydroxybenzoic acid moieties of enterobactin (Nolan et al., 2007; Vassiliadis et al., 2007). In fact, unlike its homolog IroB, the catalytic efficiency of MceC decreases with enterobactin glycosylation (Nolan et al., 2007). MceIJ or their homolog MchCD then links the C-glycosylated enterobactin to the serine and glycine-rich C-terminal region of the precursor peptide (Nolan and Walsh, 2008; Vassiliadis et al., 2010). The enterobactin esterase MceD, MchS1, or McmK hydrolyzes the C-glycosylated enterobactin into a glycosylated linear trimer of 2,3-dihydroxybenzoyl-serine (Nolan et al., 2007; Vassiliadis et al., 2007). Alternatively, the glycosylated enterobactin could be linearized prior to its linkage to the precursor peptide. None of these pathways could be excluded in in vitro MccE492 synthesis experiments. MceIJ complex catalyzes the attachment of glycosylated enterobactin, whether or not it is linearized, and MceD can hydrolyze glycosylated enterobactin whether or not it is linked to the precursor peptide (Nolan et al., 2007; Nolan and Walsh, 2008). Enterobactin glycosylation is catalyzed before linearization, because the catalytic efficiency of MceC decreases with the linearization of the enterobactin scaffold (Nolan et al., 2007).
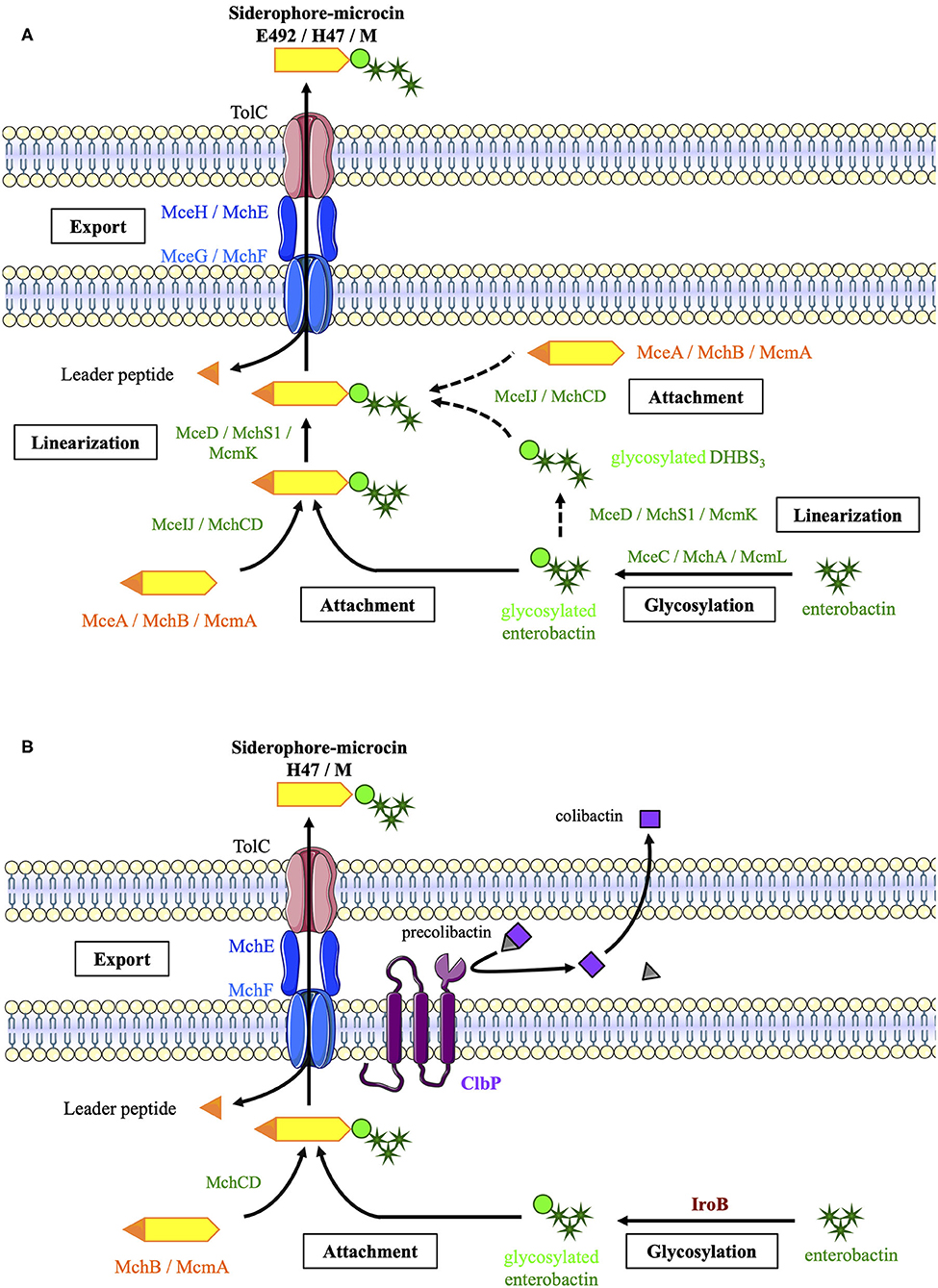
Figure 2. Biosynthetic pathways of siderophore-microcins (Mcc) (A) in strains carrying an “archetypal Mcc gene cluster” with genes encoding the enterobactin esterase and glycosyltransferase (B) in E. coli strains from the B2 phylogroup carrying a “truncated Mcc gene cluster.” This biosynthesis requires the glycosyltransferase IroB encoded by the salmochelin gene cluster and the ClbP protein encoded by the pks island, which are indicated in brown and purple, respectively [adapted from Massip et al. (2019)]. Mcc precursor peptides and proteins involved in Mcc export and post-translational modifications are indicated in orange, blue, and green, respectively.
The last step of the siderophore-Mcc maturation process is concomitant with export. Siderophore-Mcc precursors carry an N-terminal leader peptide of 15–19 amino acids, which probably acts as a recognition signal for the ABC transporter (MceG or MchF; Pons et al., 2002; Vassiliadis et al., 2010). The ABC transporter N-terminal domain with a protease activity cleaves the leader peptide during export (Azpiroz et al., 2001; Vassiliadis et al., 2010). The precise role of the accessory protein (MceH or MchE) has not been examined. However, it may act as a connector between the ABC transporter and the outer membrane protein TolC (Pons et al., 2002; Duquesne et al., 2007).
In strains such as E. coli Nissle, deprived of mcmL and mcmK, the siderophore-Mcc synthesis pathway is slightly different (Figure 2B). The enterobactin moiety is glycosylated by IroB from the salmochelin pathway instead of the missing McmL. Moreover, the periplasmic protein ClbP involved in colibactin synthesis (Nougayrède et al., 2006) is essential for MccH47 and MccM production (Massip et al., 2019). Even if the exact production pathway in such strains has not been elucidated, the C-terminal domain of ClbP has been suggested to facilitate the export of MccH47 and MccM, in which enterobactin moiety would not be linearized in the absence of the esterase McmK (Massip et al., 2019).
Siderophore-Mcc were labeled “Trojan Horse” toxins because they enter target bacteria by the catecholate siderophore receptors FepA, Fiu, or Cir (Figure 3), thanks to their post-translational modification with an enterobactin moiety (Patzer, 2003; Thomas et al., 2004; Vassiliadis et al., 2010). Consequently, they target enterobacteria that are phylogenetically similar and can be competitors for space and resources in a given niche. Once recognized by siderophore receptors, siderophore-Mcc are translocated through the outer membrane using the energy produced by the TonB machinery (Destoumieux-Garzón et al., 2003). Once in the periplasm, MccE492 interacts with the ManY/ManZ inner-membrane components of the mannose permease. It triggers channel or pore formation and TonB-dependent inner-membrane depolarization, followed by cell death (Bieler et al., 2006). MccH47 has a different cellular target: the ATP synthase (Trujillo et al., 2001). To be more precise, the F0 proton channel is required for MccH47 activity. MccH47 provokes an unregulated entry of protons, which dissipates the membrane potential (Rodriguez and Lavina, 2003). The mechanism of action of MccM has never been established, but it is suspected to be the same as that of MccH47.
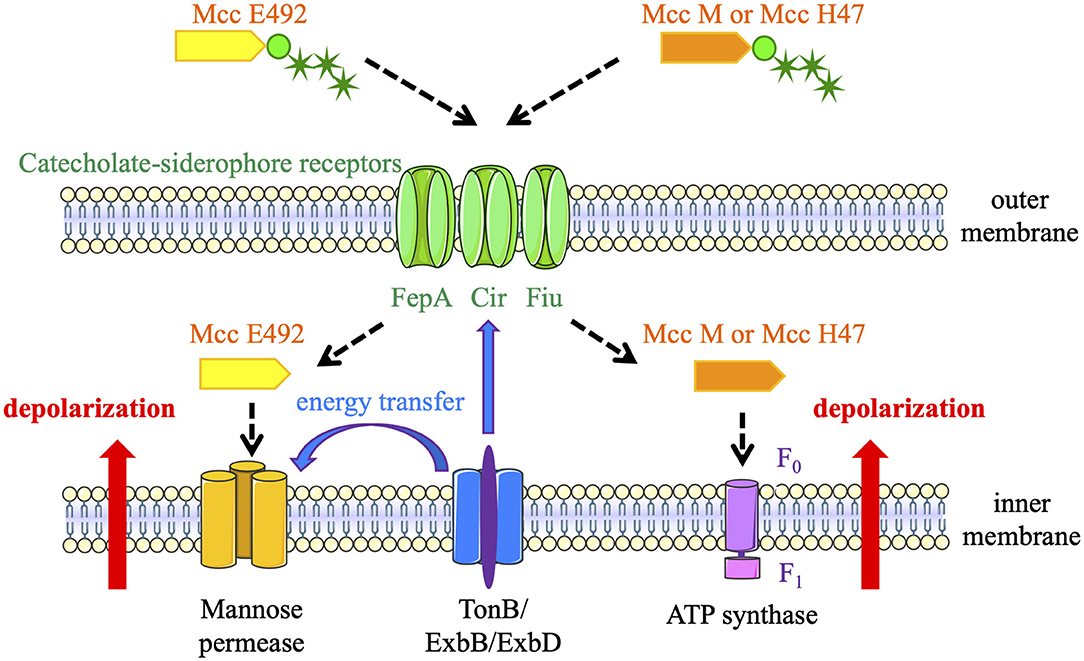
Figure 3. Siderophore-microcins (Mcc) mechanisms of action. MccE492, MccH47, and MccM enter target bacteria by catecholate siderophore receptors (FepA, Fiu, or Cir). MccE492 interacts with the mannose permease, whereas MccH47 and MccM target the F0 proton channel of the ATP synthase. These interactions trigger inner-membrane depolarization and cell death. The energy produced by the TonB machinery is required for translocation of the three siderophore-Mcc and the formation of channels or pores by MccE492 linkage with the mannose permease.
Siderophore-Mcc production is highly regulated by iron concentration through the ferric uptake regulator (Fur) protein (Patzer, 2003; Vassiliadis et al., 2007). This protein acts as a transcriptional repressor and senses intracellular iron availability (Bagg and Neilands, 1987). Fur boxes are located upstream of mceX and mchX genes (Patzer, 2003; Vassiliadis et al., 2007). At high iron availability, Fur–Fe2+ complexes repress mceX, in which transcription is coupled with mceIJ genes. Therefore, MceIJ cannot catalyze the attachment of the enterobactin moiety with the MccE492 precursor. Moreover, MceX acts as a negative regulator of mceA and mceB genes. Therefore, a high iron concentration allows a high expression of the MccE492 precursor, leading to a predominant synthesis of unmodified Mcc (Marcoleta et al., 2018). This form has a low antibacterial activity because it is not recognized by siderophore receptors (Thomas et al., 2004).
Impacts of Siderophore-Mcc Produced by E. coli
E. coli causes various extraintestinal infections, of which UTIs are the most frequent. E. coli is also a frequent cause of neonatal meningitis, bacteremia, and nosocomial infections such as nosocomial pneumonia (Johnson and Russo, 2002; Kaper et al., 2004). The patient's own intestinal flora is the reservoir for these so-called extraintestinal pathogenic E. coli (Russo and Johnson, 2000; Starčič Erjavec and Žgur-Bertok, 2015), especially for UPEC (Yamamoto et al., 1997; Moreno et al., 2008). A UPEC strain residing in the gut emerges from the rectal flora, colonizes the perineal region and the urethra, and then migrates to the bladder (Flores-Mireles et al., 2015). Therefore, effective intestinal colonization is a prerequisite for UTI development.
In industrialized countries, most human fecal strains belong to the B2 phylogroup, whereas most strains from Africa, Asia, or South America belong to the A phylogroup (Gordon and O'Brien, 2006; Tenaillon et al., 2010). The differences in phylogroup distribution are probably linked to socioeconomic factors, such as dietary and hygiene habits, which condition the gut microbiota (Tenaillon et al., 2010). A 1-year study in which the fecal strains of Swedish infants were monitored revealed that E. coli from the B2 phylogroup had a capacity for enhanced persistence in their intestinal microflora (Nowrouzian et al., 2005). Studies of fecal E. coli in Australia and the Czech Republic revealed that MccH47- and MccM-producing strains are overrepresented in the B2 phylogroup (Gordon and O'Brien, 2006; Micenková et al., 2016). Since E. coli siderophore-Mcc target phylogenetically related Enterobacteriaceae, they probably confer a selective advantage in the intestinal niche.
The proportion of B2 phylogroup strains seems to be higher in UPEC than in fecal strains: 72 and 69% in UPEC (Abraham et al., 2012; Massip et al., 2020), and 45 and 30% in fecal strains (Tenaillon et al., 2010) in Australia and France, respectively. Similarly, siderophore-Mcc-producing strains represent ~30% of UPEC (Šmajs et al., 2010; Abraham et al., 2012; Massip et al., 2020), compared to ~15% of fecal strains (Gordon and O'Brien, 2006; Micenková et al., 2016). Like in fecal E. coli, there are significantly more UPEC strains carrying siderophore-Mcc genes among B2 phylogroup strains than among non-B2 strains (Azpiroz et al., 2009; Abraham et al., 2012; Massip et al., 2020).
Most UPEC strains that produce siderophore-Mcc carry a “truncated” Mcc gene cluster deprived of genes mcmL and mcmK. Besides very rare exceptions, they also bear the pks and salmochelin islands (Azpiroz et al., 2009; Massip et al., 2020), which correspond with the functional synergy recently demonstrated between these three gene clusters used to synthetize MccH47 and MccM (Massip et al., 2019). The triple combination of “truncated” Mcc gene cluster, pks, and salmochelin islands enables the production of at least five virulence factors in addition to Mcc: the siderophores enterobactin, salmochelins, and yersiniabactin (Martin et al., 2013), colibactin (Nougayrède et al., 2006), and analgesic lipopeptides (Pérez-Berezo et al., 2017). The versatility of these three genomic islands leads to “genomic economy.” At least three pleiotropic proteins are encoded by this triad: IroB, which intervenes in Mcc and salmochelins syntheses (Fischbach et al., 2005; Massip et al., 2019); ClbA, which triggers colibactin, analgesic lipopeptide, and enterobactin production (Nougayrède et al., 2006; Martin et al., 2013; Pérez-Berezo et al., 2017); and ClbP, which is involved in Mcc and colibactin pathways (Dubois et al., 2011; Massip et al., 2019). Therefore, this type of E. coli strain can adapt to various environments with a limited impact on genome size. They persist and emerge from the polymicrobial intestinal niche, adapt to a nutrient-poor environment, and evade the host defense system.
Although this triple combination is frequent among UPEC, it is not correlated with the clinical severity of the infection (Abraham et al., 2012; Massip et al., 2020). Thus, it might not be a virulence factor per se within the urinary tract. However, a transcriptional analysis of the pyelonephritis strain CFT073 grown in urine revealed that mchB and iroB are among the 50 most upregulated genes (Snyder et al., 2004). A similar study performed with the asymptomatic bacteriuria strain ABU83972 and the probiotic strain Nissle, in addition to CFT073, showed that microcin, colibactin, and salmochelin genes are induced when the strains are grown in urine (Hancock et al., 2010). Considering that MccH47 and MccM are the most frequent Mcc in UPEC (Šmajs et al., 2010; Abraham et al., 2012), it suggests that the synergistic triad between microcin, colibactin, and salmochelin islands could promote urinary tract colonization (Massip et al., 2020). This hypothesis is supported by a study that compared the whole genome sequences of UTI and fecal isolates from the same patients. The microcin M activity protein (McmM) was significantly overrepresented in UTI isolates compared to strictly fecal isolates, unlike the classical UPEC virulence factors (e.g., fimbriae; Nielsen et al., 2017).
Moreover, UPEC strains that carry MccM and MccH47 determinants possess a greater number of other virulence factors (e.g., hlyA and cnf1) than isolates deprived of these siderophore-Mcc genes (Abraham et al., 2012; Massip et al., 2020). It suggests that the triad of the truncated Mcc gene cluster, the pks island, and the iroA locus enables the domination of the rectal niche with minimal genetic cost, which might favor the selection of additional virulence factors. The accumulation of these virulence factors eventually determines the strain pathogenicity level in a given host urinary tract.
In conclusion, MccH47 and MccM seem to be key determinants for effective intestinal colonization by B2 phylogroup E. coli. They might be particularly important in UPEC strains, for which domination and emergence from the rectal reservoir is a prerequisite for urinary tract colonization. Their production in B2 phylogroup strains seems to be optimized to minimize genetic cost and allow further selection of virulence factors. Hence, siderophore-Mcc could be a cornerstone of extraintestinal pathogenic E. coli versatility.
Author Contributions
CM wrote the first draft of the manuscript. Both authors contributed to manuscript revision, read, and approved the submitted version.
Funding
This work was supported by research grant ANR-17-CE35-0010 from the Agence Nationale de la Recherche.
Conflict of Interest
The authors declare that the research was conducted in the absence of any commercial or financial relationships that could be construed as a potential conflict of interest.
References
Abraham, S., Chapman, T. A., Zhang, R., Chin, J., Mabbett, A. N., Totsika, M., et al. (2012). Molecular characterization of Escherichia coli strains that cause symptomatic and asymptomatic urinary tract infections. J. Clin. Microbiol. 50:1027. doi: 10.1128/JCM.06671-11
Asensio, C., Pérez-Díaz, J. C., Martínez, M. C., and Baquero, F. (1976). A new family of low molecular weight antibiotics from enterobacteria. Biochem. Biophys. Res. Commun. 69, 7–14. doi: 10.1016/S0006-291X(76)80264-1
Azpiroz, M. F., Bascuas, T., and Laviña, M. (2011). Microcin H47 system: an Escherichia coli small genomic island with novel features. PLoS ONE 6:e26179. doi: 10.1371/journal.pone.0026179
Azpiroz, M. F., and Laviña, M. (2004). Involvement of enterobactin synthesis pathway in production of microcin H47. Antimicrob. Agents Chemother. 48, 1235–1241. doi: 10.1128/AAC.48.4.1235-1241.2004
Azpiroz, M. F., Poey, M. E., and Laviña, M. (2009). Microcins and urovirulence in Escherichia coli. Microb. Pathog. 47, 274–280. doi: 10.1016/j.micpath.2009.09.003
Azpiroz, M. F., Rodríguez, E., and Laviña, M. (2001). The structure, function, and origin of the microcin H47 ATP-binding cassette exporter indicate its relatedness to that of colicin V. Antimicrob. Agents Chemother. 45, 969–972. doi: 10.1128/AAC.45.3.969-972.2001
Bagg, A., and Neilands, J. B. (1987). Ferric uptake regulation protein acts as a repressor, employing iron(II) as a cofactor to bind the operator of an iron transport operon in Escherichia coli. Biochemistry 26, 5471–5477. doi: 10.1021/bi00391a039
Bieler, S., Silva, F., Soto, C., and Belin, D. (2006). Bactericidal activity of both secreted and nonsecreted microcin E492 requires the mannose permease. J. Bacteriol. 188:7049. doi: 10.1128/JB.00688-06
Budič, M., Rijavec, M., Petkovšek, Z., and Zgur-Bertok, D. (2011). Escherichia coli bacteriocins: antimicrobial efficacy and prevalence among isolates from patients with bacteraemia. PLoS ONE 6:e28769. doi: 10.1371/journal.pone.0028769
Chaudhuri, R. R., and Henderson, I. R. (2012). The evolution of the Escherichia coli phylogeny. Infect. Genet. Evol. 12, 214–226. doi: 10.1016/j.meegid.2012.01.005
Clermont, O., Dixit, O. V. A., Vangchhia, B., Condamine, B., Dion, S., Bridier-Nahmias, A., et al. (2019). Characterization and rapid identification of phylogroup G in Escherichia coli, a lineage with high virulence and antibiotic resistance potential. Environ. Microbiol. 21, 3107–3117. doi: 10.1111/1462-2920.14713
Davies, J. K., and Reeves, P. (1975). Genetics of resistance to colicins in Escherichia coli K-12: cross-resistance among colicins of group A. J. Bacteriol. 123, 102–117. doi: 10.1128/JB.123.1.102-117.1975
de Lorenzo, V., and Pugsley, A. P. (1985). Microcin E492, a low-molecular-weight peptide antibiotic which causes depolarization of the Escherichia coli cytoplasmic membrane. Antimicrob. Agents Chemother. 27:666. doi: 10.1128/AAC.27.4.666
Destoumieux-Garzón, D., Thomas, X., Santamaria, M., Goulard, C., Barthélémy, M., Boscher, B., et al. (2003). Microcin E492 antibacterial activity: evidence for a TonB-dependent inner membrane permeabilization on Escherichia coli. Mol. Microbiol. 49, 1031–1041. doi: 10.1046/j.1365-2958.2003.03610.x
Dobrindt, U., Chowdary, M. G., Krumbholz, G., and Hacker, J. (2010). Genome dynamics and its impact on evolution of Escherichia coli. Med. Microbiol. Immunol. 199, 145–154. doi: 10.1007/s00430-010-0161-2
Dobrindt, U., Hochhut, B., Hentschel, U., and Hacker, J. (2004). Genomic islands in pathogenic and environmental microorganisms. Nat. Rev. Microbiol. 2:414. doi: 10.1038/nrmicro884
Dubois, D., Baron, O., Cougnoux, A., Delmas, J., Pradel, N., Boury, M., et al. (2011). ClbP is a prototype of a peptidase subgroup involved in biosynthesis of nonribosomal peptides. J. Biol. Chem. 286, 35562–35570. doi: 10.1074/jbc.M111.221960
Duquesne, S., Destoumieux-Garzón, D., Peduzzi, J., and Rebuffat, S. (2007). Microcins, gene-encoded antibacterial peptides from enterobacteria. Nat. Prod. Rep. 24, 708–734. doi: 10.1039/b516237h
Fischbach, M. A., Lin, H., Liu, D. R., and Walsh, C. T. (2005). In vitro characterization of IroB, a pathogen-associated C-glycosyltransferase. Proc. Natl. Acad. Sci. U.S.A. 102:571. doi: 10.1073/pnas.0408463102
Fischbach, M. A., Lin, H., Liu, D. R., and Walsh, C. T. (2006). How pathogenic bacteria evade mammalian sabotage in the battle for iron. Nat. Chem. Biol. 2, 132–138. doi: 10.1038/nchembio771
Flores-Mireles, A. L., Walker, J. N., Caparon, M., and Hultgren, S. J. (2015). Urinary tract infections: epidemiology, mechanisms of infection and treatment options. Nat. Rev. Microbiol. 13, 269–284. doi: 10.1038/nrmicro3432
Gaggero, C., Moreno, F., and Laviña, M. (1993). Genetic analysis of microcin H47 antibiotic system. J. Bacteriol. 175, 5420–5427. doi: 10.1128/JB.175.17.5420-5427.1993
Gordon, D. M., and O'Brien, C. L. (2006). Bacteriocin diversity and the frequency of multiple bacteriocin production in Escherichia coli. Microbiology 152, 3239–3244. doi: 10.1099/mic.0.28690-0
Grozdanov, L., Raasch, C., Schulze, J., Sonnenborn, U., Gottschalk, G., Hacker, J., et al. (2004). Analysis of the genome structure of the nonpathogenic probiotic Escherichia coli strain Nissle 1917. J. Bacteriol. 186, 5432–5441. doi: 10.1128/JB.186.16.5432-5441.2004
Hancock, V., Vejborg, R. M., and Klemm, P. (2010). Functional genomics of probiotic Escherichia coli Nissle 1917 and 83972, and UPEC strain CFT073: comparison of transcriptomes, growth and biofilm formation. Mol. Genet. Genomics 284, 437–454. doi: 10.1007/s00438-010-0578-8
Jaureguy, F., Landraud, L., Passet, V., Diancourt, L., Frapy, E., Guigon, G., et al. (2008). Phylogenetic and genomic diversity of human bacteremic Escherichia coli strains. BMC Genomics 9:560. doi: 10.1186/1471-2164-9-560
Johnson, J. R., and Russo, T. A. (2002). Extraintestinal pathogenic Escherichia coli: “The other bad E. coli.” J. Lab. Clin. Med. 139, 155–162. doi: 10.1067/mlc.2002.121550
Kaper, J. B., Nataro, J. P., and Mobley, H. L. T. (2004). Pathogenic Escherichia coli. Nat. Rev. Microbiol. 2, 123–140. doi: 10.1038/nrmicro818
Lagos, R., Baeza, M., Corsini, G., Hetz, C., Strahsburger, E., Castillo, J. A., et al. (2001). Structure, organization and characterization of the gene cluster involved in the production of microcin E492, a channel-forming bacteriocin. Mol. Microbiol. 42, 229–243. doi: 10.1046/j.1365-2958.2001.02630.x
Laviña, M., Gaggero, C., and Moreno, F. (1990). Microcin H47, a chromosome-encoded microcin antibiotic of Escherichia coli. J. Bacteriol. 172, 6585–6588. doi: 10.1128/JB.172.11.6585-6588.1990
Marcoleta, A. E., Gutiérrez-Cortez, S., Hurtado, F., Argandoña, Y., Corsini, G., Monasterio, O., et al. (2018). The Ferric uptake regulator (Fur) and iron availability control the production and maturation of the antibacterial peptide microcin E492. PLoS ONE 13:e0200835. doi: 10.1371/journal.pone.0200835
Martin, P., Marcq, I., Magistro, G., Penary, M., Garcie, C., Payros, D., et al. (2013). Interplay between siderophores and colibactin genotoxin biosynthetic pathways in Escherichia coli. PLoS Pathog. 9:e1003437. doi: 10.1371/journal.ppat.1003437
Massip, C., Branchu, P., Bossuet-Greif, N., Chagneau, C. V., Gaillard, D., Martin, P., et al. (2019). Deciphering the interplay between the genotoxic and probiotic activities of Escherichia coli Nissle 1917. PLoS Pathog. 15:e1008029. doi: 10.1371/journal.ppat.1008029
Massip, C., Chagneau, C. V., Boury, M., and Oswald, E. (2020). The synergistic triad between microcin, colibactin, and salmochelin gene clusters in uropathogenic Escherichia coli. Microbes Infect. 22, 144–147. doi: 10.1016/j.micinf.2020.01.001
Micenková, L., Bosák, J., Štaudová, B., Kohoutová, D., Cejková, D., Woznicová, V., et al. (2016). Microcin determinants are associated with B2 phylogroup of human fecal Escherichia coli isolates. MicrobiologyOpen 5, 490–498. doi: 10.1002/mbo3.345
Miethke, M., and Marahiel, M. A. (2007). Siderophore-based iron acquisition and pathogen control. Microbiol. Mol. Biol. Rev. 71, 413–451. doi: 10.1128/MMBR.00012-07
Moreno, E., Andreu, A., Pigrau, C., Kuskowski, M. A., Johnson, J. R., and Prats, G. (2008). Relationship between Escherichia coli strains causing acute cystitis in women and the fecal E. coli population of the host. J. Clin. Microbiol. 46, 2529–2534. doi: 10.1128/JCM.00813-08
Nielsen, K. L., Stegger, M., Kiil, K., Godfrey, P. A., Feldgarden, M., Lilje, B., et al. (2017). Whole-genome comparison of urinary pathogenic Escherichia coli and faecal isolates of UTI patients and healthy controls. Int. J. Med. Microbiol. 307, 497–507. doi: 10.1016/j.ijmm.2017.09.007
Nolan, E. M., Fischbach, M. A., Koglin, A., and Walsh, C. T. (2007). Biosynthetic tailoring of microcin E492m: post-translational modification affords an antibacterial siderophore-peptide conjugate. J. Am. Chem. Soc. 129, 14336–14347. doi: 10.1021/ja074650f
Nolan, E. M., and Walsh, C. T. (2008). Investigations of the MceIJ-catalyzed posttranslational modification of the microcin E492 C-terminus: linkage of ribosomal and nonribosomal peptides to form “Trojan Horse” antibiotics. Biochemistry 47, 9289–9299. doi: 10.1021/bi800826j
Nougayrède, J.-P., Homburg, S., Taieb, F., Boury, M., Brzuszkiewicz, E., Gottschalk, G., et al. (2006). Escherichia coli induces DNA double-strand breaks in eukaryotic cells. Science 313, 848–851. doi: 10.1126/science.1127059
Nowrouzian, F. L., Wold, A. E., and Adlerberth, I. (2005). Escherichia coli strains belonging to phylogenetic group B2 have superior capacity to persist in the intestinal microflora of infants. J. Infect. Dis. 191, 1078–1083. doi: 10.1086/427996
Patzer, S. I. (2003). The colicin G, H and X determinants encode microcins M and H47, which might utilize the catecholate siderophore receptors FepA, Cir, Fiu and IroN. Microbiology 149, 2557–2570. doi: 10.1099/mic.0.26396-0
Pérez-Berezo, T., Pujo, J., Martin, P., Faouder, P., Galano, J.-M., Guy, A., et al. (2017). Identification of an analgesic lipopeptide produced by the probiotic Escherichia coli strain Nissle 1917. Nat. Commun. 8:1314. doi: 10.1038/s41467-017-01403-9
Picard, B., Garcia, J. S., Gouriou, S., Duriez, P., Brahimi, N., Bingen, E., et al. (1999). The link between phylogeny and virulence in Escherichia coli extraintestinal infection. Infect. Immun. 67, 546–553. doi: 10.1128/IAI.67.2.546-553.1999
Poey, M. E., Azpiroz, M. F., and Laviña, M. (2006). Comparative analysis of chromosome-encoded microcins. Antimicrob. Agents Chemother. 50, 1411–1418. doi: 10.1128/AAC.50.4.1411-1418.2006
Pons, A.-M., Lanneluc, I., Cottenceau, G., and Sable, S. (2002). New developments in non-post translationally modified microcins. Biochimie 84, 531–537. doi: 10.1016/S0300-9084(02)01416-5
Reister, M., Hoffmeier, K., Krezdorn, N., Rotter, B., Liang, C., Rund, S., et al. (2014). Complete genome sequence of the gram-negative probiotic Escherichia coli strain Nissle 1917. J. Biotechnol. 187, 106–107. doi: 10.1016/j.jbiotec.2014.07.442
Rodríguez, E., Gaggero, C., and Laviña, M. (1999). The structural gene for microcin H47 encodes a peptide precursor with antibiotic activity. Antimicrob. Agents Chemother. 43, 2176–2182. doi: 10.1128/AAC.43.9.2176
Rodriguez, E., and Lavina, M. (2003). The proton channel is the minimal structure of ATP synthase necessary and sufficient for microcin H47 antibiotic action. Antimicrob. Agents Chemother. 47, 181–187. doi: 10.1128/AAC.47.1.181-187.2003
Russo, T. A., and Johnson, J. R. (2000). Proposal for a new inclusive designation for extraintestinal pathogenic isolates of Escherichia coli: ExPEC. J. Infect. Dis. 181, 1753–1754. doi: 10.1086/315418
Šmajs, D., Micenková, L., Šmarda, J., Vrba, M., Ševčíková, A., Vališová, Z., et al. (2010). Bacteriocin synthesis in uropathogenic and commensal Escherichia coli: colicin E1 is a potential virulence factor. BMC Microbiol. 10:288. doi: 10.1186/1471-2180-10-288
Snyder, J. A., Haugen, B. J., Buckles, E. L., Lockatell, C. V., Johnson, D. E., Donnenberg, M. S., et al. (2004). Transcriptome of uropathogenic Escherichia coli during urinary tract infection. Infect. Immun. 72, 6373–6381. doi: 10.1128/IAI.72.11.6373-6381.2004
Starčič Erjavec, M., and Žgur-Bertok, D. (2015). Virulence potential for extraintestinal infections among commensal Escherichia coli isolated from healthy humans—the Trojan horse within our gut. FEMS Microbiol. Lett. 362:fnu061. doi: 10.1093/femsle/fnu061
Tenaillon, O., Skurnik, D., Picard, B., and Denamur, E. (2010). The population genetics of commensal Escherichia coli. Nat. Rev. Microbiol. 8, 207–217. doi: 10.1038/nrmicro2298
Thomas, X., Destoumieux-Garzón, D., Peduzzi, J., Afonso, C., Blond, A., Birlirakis, N., et al. (2004). Siderophore peptide, a new type of post-translationally modified antibacterial peptide with potent activity. J. Biol. Chem. 279, 28233–28242. doi: 10.1074/jbc.M400228200
Touchon, M., Hoede, C., Tenaillon, O., Barbe, V., Baeriswyl, S., Bidet, P., et al. (2009). Organised genome dynamics in the Escherichia coli species results in highly diverse adaptive paths. PLoS Genet. 5:e1000344. doi: 10.1371/journal.pgen.1000344
Tourret, J., and Denamur, E. (2016). Population phylogenomics of extraintestinal pathogenic Escherichia coli. Microbiol. Spectr. 4:UTI-0010-2012. doi: 10.1128/microbiolspec.UTI-0010-2012
Trujillo, M., Rodríguez, E., and Laviña, M. (2001). ATP synthase is necessary for microcin H47 antibiotic action. Antimicrob. Agents Chemother. 45, 3128–3131. doi: 10.1128/AAC.45.11.3128-3131.2001
Vassiliadis, G., Destoumieux-Garzón, D., Lombard, C., Rebuffat, S., and Peduzzi, J. (2010). Isolation and characterization of two members of the siderophore-microcin family, microcins M and H47. Antimicrob. Agents Chemother. 54, 288–297. doi: 10.1128/AAC.00744-09
Vassiliadis, G., Peduzzi, J., Zirah, S., Thomas, X., Rebuffat, S., and Destoumieux-Garzón, D. (2007). Insight into siderophore-carrying peptide biosynthesis: enterobactin is a precursor for microcin E492 posttranslational modification. Antimicrob. Agents Chemother. 51, 3546–3553. doi: 10.1128/AAC.00261-07
Vejborg, R. M., Friis, C., Hancock, V., Schembri, M. A., and Klemm, P. (2010). A virulent parent with probiotic progeny: comparative genomics of Escherichia coli strains CFT073, Nissle 1917 and ABU 83972. Mol. Genet. Genomics 283, 469–484. doi: 10.1007/s00438-010-0532-9
Keywords: microcins, Escherichia coli, ExPEC, UPEC, genomic island (GI), intestinal colonization, pathogenesis, B2 phylogroup
Citation: Massip C and Oswald E (2020) Siderophore-Microcins in Escherichia coli: Determinants of Digestive Colonization, the First Step Toward Virulence. Front. Cell. Infect. Microbiol. 10:381. doi: 10.3389/fcimb.2020.00381
Received: 01 April 2020; Accepted: 22 June 2020;
Published: 21 August 2020.
Edited by:
Mauricio J. Farfan, University of Chile, ChileReviewed by:
Arun K. Bhunia, Purdue University, United StatesXingmin Sun, University of South Florida, United States
Copyright © 2020 Massip and Oswald. This is an open-access article distributed under the terms of the Creative Commons Attribution License (CC BY). The use, distribution or reproduction in other forums is permitted, provided the original author(s) and the copyright owner(s) are credited and that the original publication in this journal is cited, in accordance with accepted academic practice. No use, distribution or reproduction is permitted which does not comply with these terms.
*Correspondence: Clémence Massip, massip.c@chu-toulouse.fr; Eric Oswald, eric.oswald@inserm.fr