- 1Department of Laboratory Medicine, West China Hospital, Sichuan University, Chengdu, China
- 2Department of Microbiology, West China School of Basic Medical Sciences & Forensic Medicine, Sichuan University, Chengdu, China
- 3Department of Laboratory Medicine, West China Second University Hospital, Sichuan University, Chengdu, China
- 4State Key Laboratory of Oral Diseases, Sichuan University, Chengdu, China
Background: Growing evidence points out that a disturbance of gut microbiota may also disturb the gut–brain communication. However, it is not clear to what extent the alteration of microbiota composition can modulate brain function, affecting host behaviors. Here, we investigated the effects of gut microbiota depletion on emotional behaviors.
Methods: Mice in the experimental group were orally administered ceftriaxone sodium solution (250 mg/ml, 0.2 ml/d) for 11 weeks. The open-field test and tail-suspension test were employed for the neurobehavioral assessment of the mice. Fecal samples were collected for 16s rDNA sequencing. The serum levels of cytokines and corticosterone were quantified using enzyme-linked immunosorbent assays. The immunohistochemistry method was used for the detection of brain-derived neurotrophic factor (BDNF) and c-Fos protein.
Results: The gut microbiota for antibiotic-treated mice showed lower richness and diversity compared with normal controls. This effect was accompanied by increased anxiety-like, depression-like, and aggressive behaviors. We found these changes to be possibly associated with a dysregulation of the immune system, abnormal activity of the hypothalamic-pituitary-adrenal axis, and an alteration of neurochemistry.
Conclusions: The findings demonstrate the indispensable role of microbiota in the gut–brain communication and suggest that the absence of conventional gut microbiota could affect the nervous system, influencing brain function.
Introduction
Gut microbiota, known as a reservoir of bacteria, not only plays an essential role in host digestion and energy metabolism but shapes host immunity (Aleshukina, 2012; Antonopoulos and Chang, 2016; Thursby and Juge, 2017). Recently, evidence of its influence extends well-beyond the gut, many studies have begun to report that the gut microbiota may be associated with the development and progression of diseases affecting multiple organ systems such as liver, lung, and brain (Felix et al., 2018; Lee and Jayaraman, 2019; Yuan et al., 2019). Researchers believe that there is a potential connection between the gut and the central nervous system (CNS). Additional studies have defined this connection as a bi-directional communication covering multiple connections, such as immune response, the vagus nerve, and humoral components (Mayer et al., 2015). Recent evidence has unlocked a novel pivotal member, gut microbiota, which plays an important role in this communication. As a result, this concept, now known as the microbiota-gut-brain (MGB) axis, has been prompted and subsequently implicated in multiple disorders, such as digestive, neurological, and psychiatric diseases (Scriven et al., 2018; Iannone et al., 2019).
Antibiotics are one of the most commonly prescribed drugs worldwide. There has been an increasing concern that variations in the microbiota induced by antibiotics may have detrimental consequences for health (Kim et al., 2017). A growing body of evidence confirms the role of specific microbial compositions in the modulation of brain functions as well as host behaviors. To be specific, a complete absence of gut microbiota resulted in alteration of blood–brain barrier (BBB) permeability and brain neurochemistry with decreased social behaviors in mice (Braniste et al., 2014).
While the whole brain is vulnerable to external stimuli, two regions that influence stress responsivity and behavior have been considered as the most likely targets for gut microbiota (Luczynski et al., 2016). The first region is the amygdala, which seems to be involved in many forms of negative emotionality, including anxiety (Davis et al., 1994; Janak and Tye, 2015). After receiving input from disgust stimuli, the amygdala projects to the regions or sub-regions regulating anxious and defensive behaviors (Kovács, 2008). Usually, the activation of the amygdala is measured by c-Fos expression (Kovács, 2008). The second region, the hippocampus, is well-known as for emotion regulation. In this study, germ-free (GF) mice exhibited more anxiety-like behaviors, which were accompanied by higher brain-derived neurotrophic factor (BDNF) levels in the dentate region of the hippocampus (Sudo et al., 2004; Neufeld et al., 2011). Here, we explored the contribution of gut microbiota to the CNS via depleting bacteria with ceftriaxone sodium, a broad spectrum antibiotic.
Materials and Methods
Study Design
Male BALB/c mice (6–8 weeks; Institute of Laboratory Animals of Sichuan Academy of Medical Sciences, Sichuan, China) were maintained (ten mice per cage) under a specific-pathogen-free (SPF) condition at 22–26°C, 40–60% humidity, and 12-h light-dark cycle. The mice were given 1 week to acclimate. All mice were fed with adequate food and clean water. At the end of adaptive phase, all mice (initial weight 23.55 ± 1.49 g) were randomly divided into two groups (n = 20 for each group) and given either sterile saline solution (the control group was defined as the CT group, 0.2 ml/d) or ceftriaxone sodium solution (Qilu Pharmaceutical, Shandong, China) (the antibiotic group was defined as the AB group, 250 mg/ml, 0.2 ml/d) intragastrically once a day for 11 consecutive weeks (details about drug dosages is included in Supplementary Materials). Mice were housed by group (10 mice per cage) from the first day of gavage to avoid interference between different groups. A battery of behavioral tests was administrated weekly, with 1 h of rest between each test.
Eleven weeks after ceftriaxone treatment, the mice in the AB group exhibited a remarkable difference in behavioral parameters. On the second day after the last behavioral experiment was performed, the mice were administered the final gavage exposure, and 1 h later, fresh blood and stool was sampled. The mice were inspected daily for changes in appearance and body weight. All experiments followed the guidelines of the Chinese Council on Animal Care and were approved by the Animal Care Advisory Committee of Sichuan University, Sichuan, China. The experimental design was shown in Figure 1.
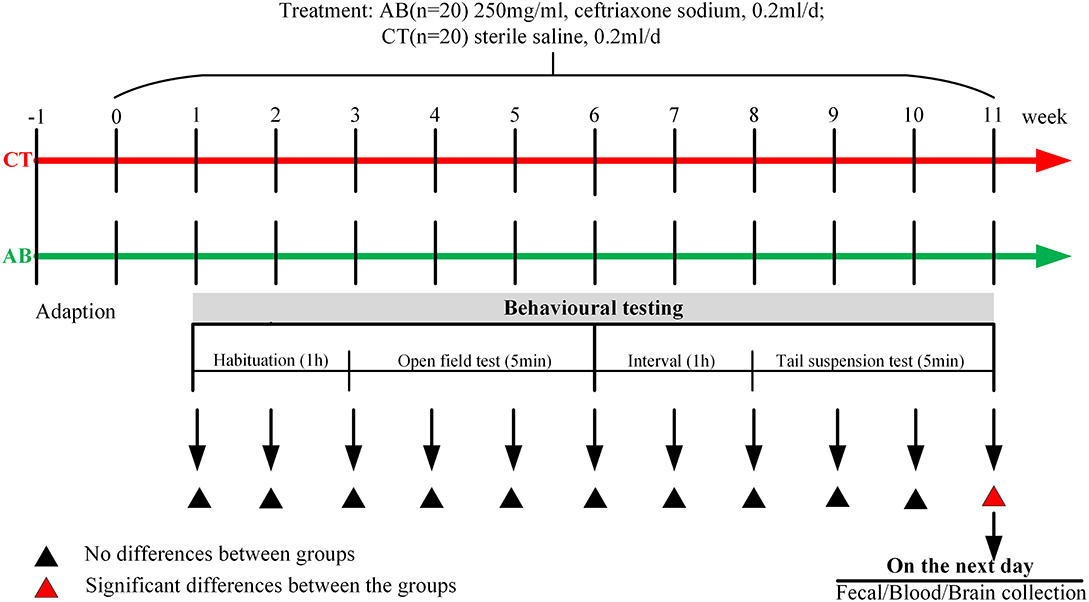
Figure 1. Study design. After seven days of adaptation, the antibiotic (AB) group was given ceftriaxone and the control (CT) group was given saline by gavage once a day. The open-field test (OFT) and tail-suspension test (TST) were given to mice once a week. After 11 weeks of oral gavage, significant behavioral differences were noted between the groups. On the second day after the last behavioral experiment was performed, mice were administered the final gavage exposure, and 1 h later, fresh blood and stool was sampled.
Behavioral Tests
Two behavioral tests were carried out under following sequence (from 8 a.m. to 5 p.m.): open-field test (OFT) → tail-suspension test (TST). The OFT, which involves a low stress level, preceded the TST, which involves a high stress level (Di et al., 2017). Prior to each behavioral test, mice were habituated for at least 1 h to the testing room (Champagne-Jorgensen et al., 2020). The lighting condition was set at 15 lux for all behavioral tests (Dere et al., 2004).
The Open-Field Test
The equipment of the OFT was composed of a square arena 100 × 100 cm with 40 cm walls. The floor was subdivided into a center and periphery compartment with 25 squares. Mice were placed alternatively in the open field for at least 30 min and allowed to explore undisturbedly before the first test (Champagne-Jorgensen et al., 2020). In the formal test, mice were placed singly in the center of the open field and allowed to freely explore for 5 min. Relevant parameters (the total distance, the total time in the periphery, and center of the open field) were recorded by a video monitor. At the end of the test, mice were sent back to their home cages, and the test box was cleaned with 70% ethyl alcohol and air dried. The OFT has been proven to be efficient in detecting anxiety and selecting anxiolytic drugs (Kraeuter et al., 2019).
The Tail-Suspension Test
Mice were suspended in an upside-down position by the tail, so that they could not escape or touch nearby surfaces. The rationale for the test is that mice are under enormous stress, and, if they don't have the desire to live, they will develop a motionless posture quickly and maintain it for a longer period. The total duration of quiescence and activity during 5 min was scored, respectively (Młyniec and Nowak, 2012).
Gut Microbiota Analysis
A TIANamp Bacteria DNA Kit (TIANGEN, China) was used to extract fecal DNA. Then, the extraction was eluted using elution buffer and stored at −80°C until PCR amplification detection by LC-Bio (Hangzhou, China). The V3-V4 region of the prokaryotic 16S rRNA gene was amplified with primers 338F (5′-ACTCCTACGGGAGGCAGCAG-3′) and 806R (5′-GGACTACHVGGGTWTCTAAT-3′) (Fadrosh et al., 2014). The detailed operation was performed as described previously (Li et al., 2019).
Serum Cytokine Assay
Cytokines secretion is usually induced in an inflammation or infection. Except for their effects on immunity, cytokines can also affect brain function and modulate host behaviors (Köhler et al., 2018). Research has suggested that serum IL-6 and IL-10 levels are putative biomarkers for several mood disorders (Wiener et al., 2019). Here, fresh blood was collected in sterile tubes, coagulated at room temperature, and centrifuged at 1000 × g for 10 min after the last ceftriaxone sodium treatment. The serum was stored at −70°C for later analysis. IL-6 and IL-10 were quantified by enzyme-linked immunosorbent assay (ELISA) (Neobioscience, Shenzhen Xinbosheng Biotechnology Co., Ltd, China). The detection limit of the assay was about 1 pg ml−1. According to the manufacturer's protocol, the assay was performed in triplicate.
Serum Corticosterone Assay
Corticosterone is the end product of the hypothalamus-pituitary-adrenal (HPA) axis in rodents. Rising corticosterone levels suggest increases in HPA axis activity (Hiroshi et al., 2006). Serum corticosterone was measured by ELISA (Cusabio,Wuhan Huamei Biotechnology Co., Ltd, China). The detection limit of the assay was about 1 ng ml−1. The assay was performed in triplicate according to the manufacturer's protocol.
Immunohistochemistry
The immunohistochemistry was used to assess expression of brain-derived neurotropic factor (BDNF) in the hippocampus and c-Fos in the amygdala. The whole process consisted of brain collection, sectioning, and immunolabeling. The details referred to previous literatures (Gareau et al., 2011).
Each maker was quantified by staining intensity and extent. We scored the staining intensity as follows: negative, weak, moderate, and strong (on a scale of zero to four). The staining extent was divided into five grades according to the percentage of positive cells in the region: negative, 0–25, 26–50, 51–75, and 76–100% (on a scale of zero to four) (Liu et al., 2011).
Semi-quantification of BDNF was calculated by multiplying the intensity score and fraction score in the CA1, CA3, and DG (dentate gyrus) regions of the hippocampus (Olympus, Tokyo, Japan, BX53). Similarly, semi-quantification of c-Fos was performed by calculating the intensity score and fraction score in the CeC, CeL, and CeM regions of the amygdala. The immunohistochemical analysis was performed blind.
Statistical Analysis
Data were expressed as the mean ± standard deviation or median (IQR) and analyzed by one-way ANOVA or Wilcoxon rank sum test in SPSS 22.0 software (SPSS Inc., Chicago, IL, USA). P-values less than 0.05 were considered statistically significant.
Results
The Effect of Ceftriaxone Treatment on Body Weight
Mice in the AB group gained less weight than the CT group, with this difference increasing progressively over time. After gavage for 7 weeks, the weight of the AB group was significantly lower than that of the CT group (p < 0.05) (Figure 2).
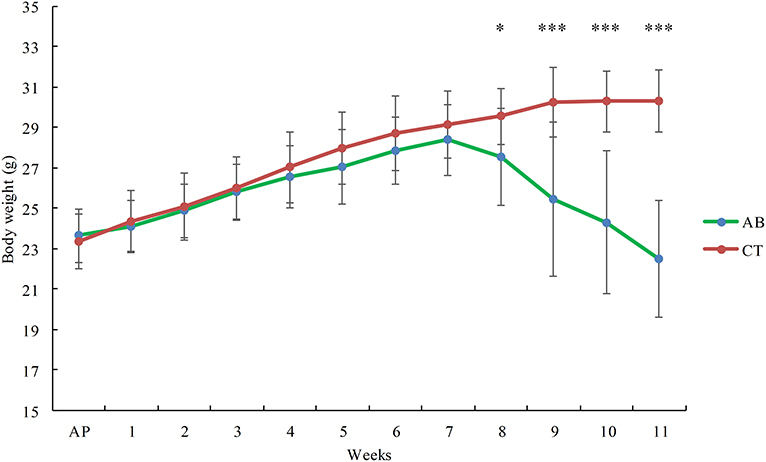
Figure 2. Effect of ceftriaxone on body weight. AB, ceftriaxone administration for 11 weeks (n = 14); CT, saline treatment for 11 weeks (n = 18). AP: adaptive phase. *P < 0.05, ***P < 0.001.
The Effect of Ceftriaxone Treatment on Mice Behaviors
Mice Treated With Ceftriaxone Sodium Exhibited Anxiety-Like Behaviors
OFT is usually performed to assess locomotor activity and exploratory behavior (Kraeuter et al., 2019). The former was represented by the total distance traveled throughout the 5 min, and no differences were observed between the two groups. Previous studies suggest mice prefer staying close to the walls and travel more in the periphery field can be described as showing signs of anxiety (Crawley, 1985). Comparatively, mice with lower anxiety tend to spend more time in the central field. In this study, the AB group spent less time in the center as compared to the CT group (p < 0.001) after gavage for 11 weeks. Meanwhile, the AB group reduced movement in the center (p < 0.001) (Figures 3, 4A). For details of the 11-week behavioral data analysis, see Supplementary Figure 1.
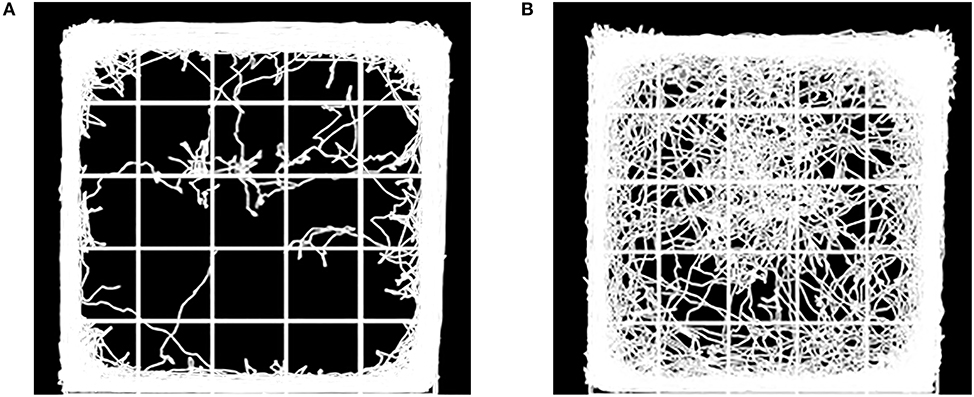
Figure 3. Diagram showing movement of (A) AB group mice and (B) CT group mice in the open field. AB, antibiotic group (n = 14); CT, control group (n = 18).
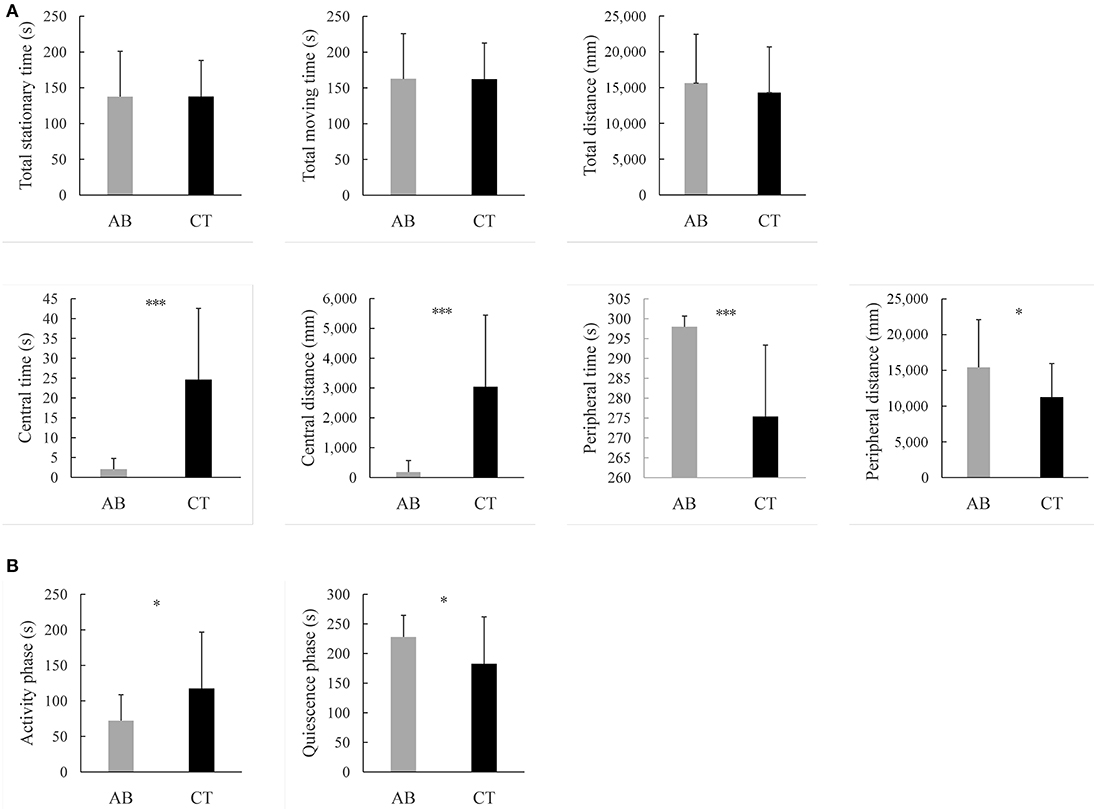
Figure 4. Results of behavioral tests (A) in the open field test, (B) in the suspension tail test. AB, antibiotic group (n = 14); CT, control group (n = 18). *P < 0.05, ***P < 0.001.
Mice Treated With Ceftriaxone Sodium Exhibited Depression-Like Behaviors
TST was often used for evaluating the ability to cope with a stressful situation (Kraeuter et al., 2019). Decreased duration of activity is considered a sign of depressive behavior (Castagne et al., 2011). In the test, the AB group showed decreased activity during the 5 min and stopped escaping earlier than the CT group (p < 0.05) after gavage for 11 weeks (Figure 4B).
Mice Treated With Ceftriaxone Sodium Exhibited High Aggressive Behavior
Eight weeks after ceftriaxone administration, visible injuries were observed in the AB group, suggesting that aggressive behaviors had occurred. Four mice from the AB group were excluded from the experiment 10 weeks later due to serious injuries influencing mobility. In contrast, the CT group did not get injured throughout the experiment.
The Effect of Ceftriaxone Treatment on Gut Microbiota Composition
16S rDNA sequencing was used to identify alterations in gut microbiota after gavage for 11 weeks. Ceftriaxone administration induced a significant change in gut microbiota diversity. For the Venn diagram (Figure 5), the number of shared and unique OTUs indicate a similarity and difference of gut microbiota between groups, respectively (Ren et al., 2018). Based on this, there were 587 OTUs specific to the AB group and 1,570 specific to the CT group, accounting for 19.38 and 39.13% of the total OTU richness, respectively. All samples shared 2,442 OTUs at 97% similarity. The alpha diversity analysis revealed that the AB group had lower species diversity, richness, and evenness than that of the CT group by plotting Chao1, Shannon, Simpson, and Observed_species curves (Figure 6). A strong antibiotic effect was observed in beta diversity analysis. The PCA plot showed an appreciable separation between the two groups, indicating they had low similarity in gut microbiota composition. Likewise, the PCoA plot and MDS plot indicated the microbiota of AB group clustered separately from CT group (Figure 7).
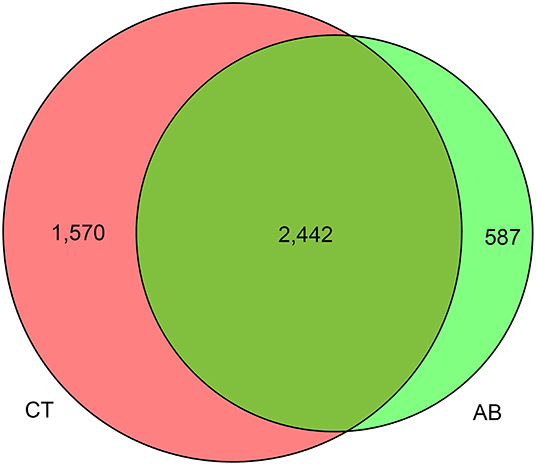
Figure 5. Venn diagram of fecal bacteria. AB, antibiotic group (n = 14); CT, control group (n = 18).
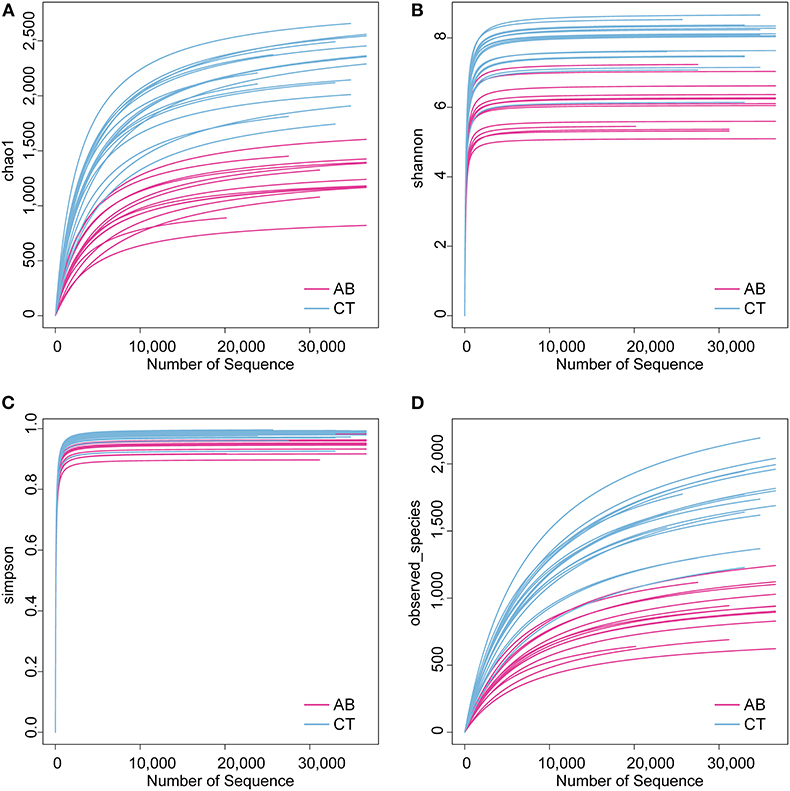
Figure 6. Alpha diversity analysis for gut microbiota. (A) Chao1 curves for each group. (B) Shannon-Wiener curves for each group. (C) Simpson curves for each group. (D) Observed_species curves for each group. AB, antibiotic group (n = 14); CT, control group (n = 18).
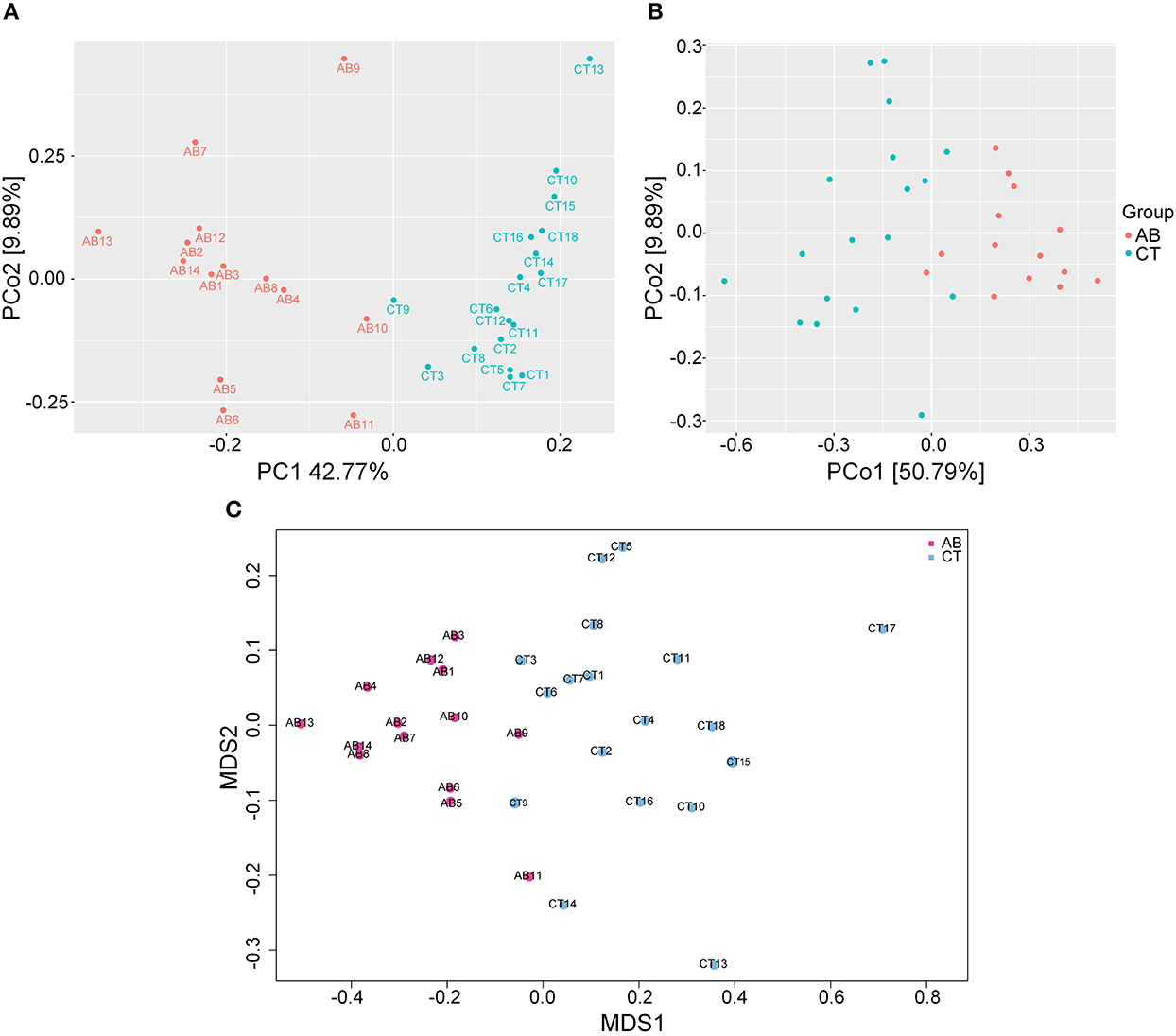
Figure 7. Beta diversity analysis for gut microbiota. (A) PCA plot of weighted UniFrac distances between samples. (B) PCoA plot of weighted UniFrac distances between samples. (C) MDS plot of weighted UniFrac distances between samples. AB, antibiotic group (n = 14); CT, control group (n = 18).
According to abundance analysis, the dominant phyla in AB and CT groups were Bacteroidetes and Firmicutes, while the relative abundance of Firmicutes was lower in the AB group than that in CT group. Significant abundance differences were observed in the following phyla: Proteobacteria increased while five phyla decreased (Firmicutes, Actinobacteria, Candidatus Saccharibacteria, Deferribacteres, and Candidatus_Melainabacteria) in the AB group (Figure 8A, Table 1). At the genus level, ceftriaxone increased the proportion of Proteobacteria, Porphyromonadaceae_unclassified, Escherichia, and Parabacteroides, while Lactobacillus, Acetatifactor, Bacteroidetes_unclassified, Barnesiella, Helicobacter, Prevotella, Alistipes, and Bacteroidales_unclassified declined (Figure 8B, Table 2). Ten phyla and 21 genera were clustered by heatmaps, which demonstrated the relative abundance of species in different samples. In the CT group, the samples got closer to each other, indicating a higher similarity among them (Figure 9).
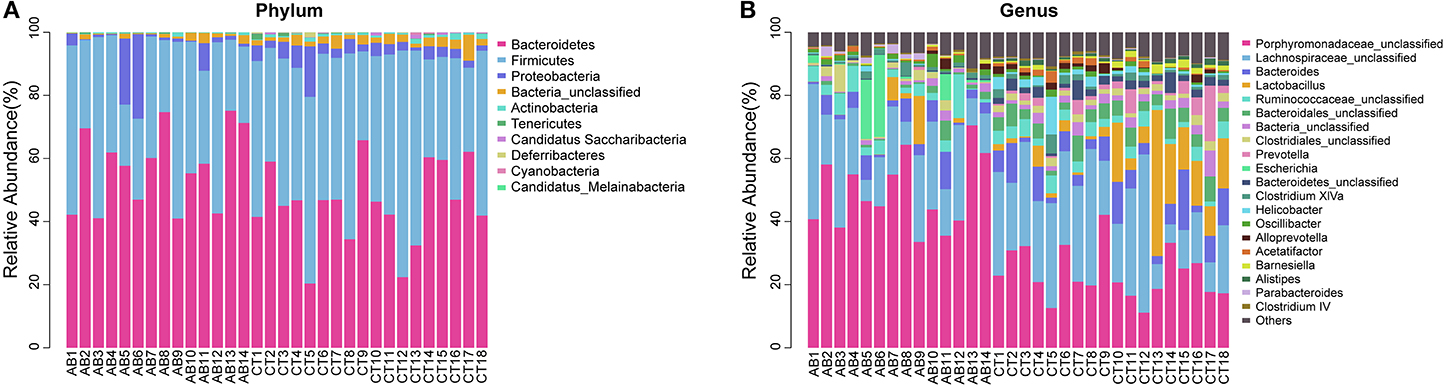
Figure 8. Composition abundance of gut microbiota (A) at the phylum level and (B) the genus level. AB, antibiotic group (n = 14); CT, control group (n = 18).
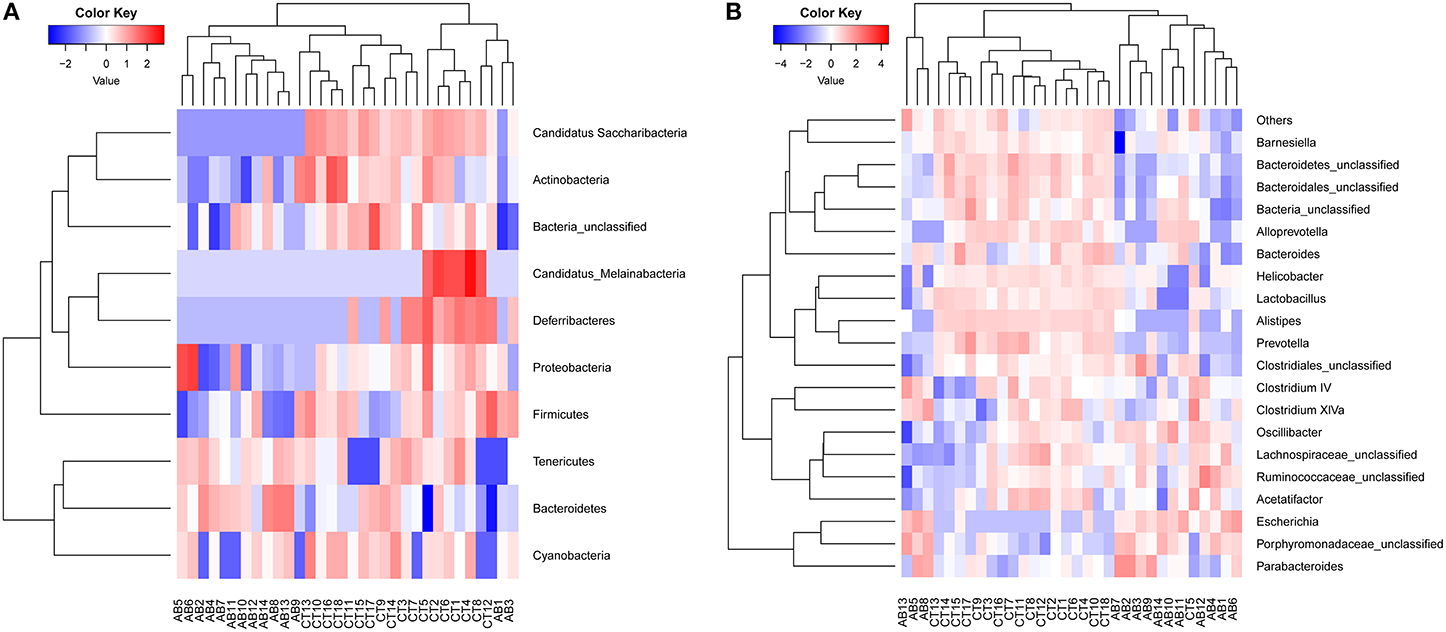
Figure 9. Heat maps of gut microbiota (A) at the phylum level and (B) the genus level. Red and blue colors indicate high and low values of the percent of reads classified at that rank. AB, antibiotic group (n = 14); CT, control group (n = 18).
The Effect of Ceftriaxone Treatment on Serum Cytokines and Corticosterone
Ceftriaxone induced increased IL-6 and IL-10 in the AB group (IL-6: 51.82 ± 9.99 pg/ml and 43.21 ± 10.18 pg/ml for AB and CT, respectively) (IL-10: 274.81 ± 95.59 pg/ml and 173.12 ± 55.31 pg/ml for AB and CT, respectively) (Figures 10A,B). In addition, serum corticosterone was significantly higher in the AB group than in the CT group (10.16 ± 4.97 ng/ml and 5.39 ± 4.03 ng/ml for AB and CT, respectively) (Figure 10C).
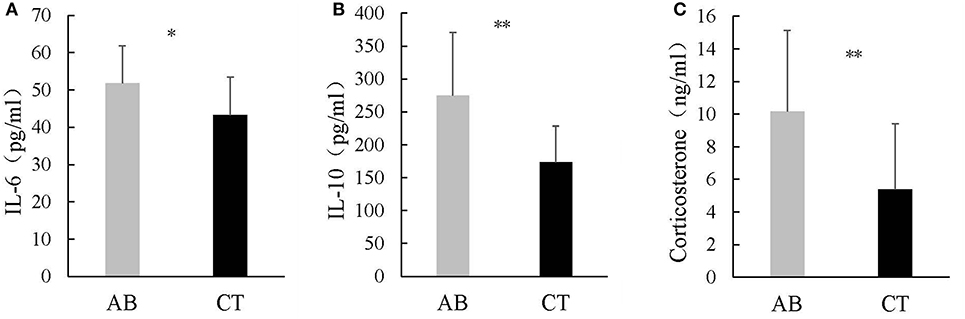
Figure 10. Concentrations of (A) IL-6, (B) IL-10, and (C) corticosterone in serum. AB, antibiotic group (n = 14); CT, control group (n = 18). *P < 0.05, **P < 0.01.
The Effect of Ceftriaxone Treatment on Hippocampal Cell Proliferation and Neural Activity
A slight decrease of BDNF in the CA1, CA3, and DG regions of the hippocampus was observed in the AB group compared to the CT group (Figure 11, Table 3). Meanwhile, c-Fos expression increased in the amygdala of the AB group without a statistically significant difference (Figure 12, Table 3).
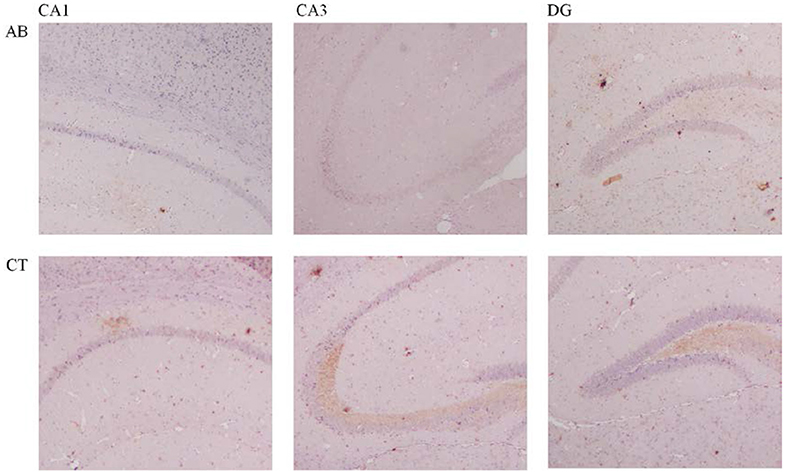
Figure 11. Immunohistochemical results of BDNF in the hippocampus of mice (×400). AB, antibiotic group (n = 14); CT, control group (n = 18). CA1, field CA1 of hippocampus; CA3, field CA3 of hippocampus; DG, dentate gyrus.
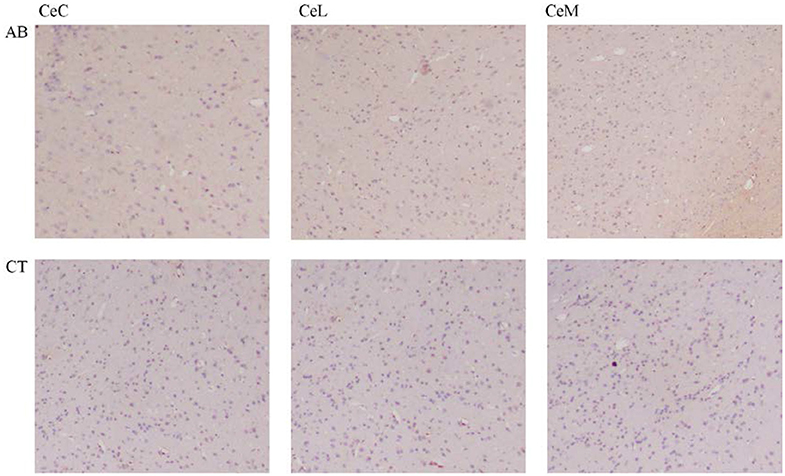
Figure 12. Immunohistochemical results of c-Fos in the amygdala of mice (×400). AB, antibiotic group (n = 14); CT, control group (n = 18). CeC, central amygdaloid nucleus, capsular part; CeL, central amygdaloid nucleus, lateral division; CeM, central amygdaloid nucleus, medial division.
Discussion
The gut, a vulnerable but vital organ, is affected by different factors easily. Antibiotics are one of the common causes leading to gut disturbance, especially given the broad spectrum of antibiotics. Consistently, little is known about adverse effects of these antibiotics on health except for drug resistance. But, recently, medications with antibiotic have been reported to enhance the risk of allergies, inflammatory bowel diseases, obesity, and even mental diseases (Harris and Baffy, 2017; Torres-Fuentes et al., 2017; Guo J. et al., 2019; Slykerman et al., 2019). Also, some investigators suggest that abnormal gut microbiota or some intestinal infections may be responsible for a series of metabolism or immunity-related diseases (Wang and Wang, 2016). The impact of intestinal dysbacteriosis induced by antibiotics on brain functions and behaviors piques interest and is yet to be elucidated. Therefore, this study was designed to determine whether long-term ceftriaxone exposure altered gut microbiota and thus affected host behaviors.
Ceftriaxone administration caused significant weight loss in the study. This is consistent with the previous finding that the weight gain of mice was delayed significantly following the ceftriaxone treatment (Miao et al., 2020). However, it was contradictory with previous findings that antibiotics result in weight gain in the animal production system (Angelakis, 2017). The disparate findings imply that the growth of animals may be impacted by the dosage, intervention time and, above all, types and properties of antibiotics.
Ceftriaxone could result in a significant gut microbiota dysbiosis by killing most of the normal flora and providing the living space for other potential pathogens (Cheng et al., 2019). The gut microbiota of mice was altered greatly in quantity and quality by the oral administration of ceftriaxone in this study. Similar to the result, other studies confirmed that oral ceftriaxone significantly decreased the quantity of fecal microbiota (Cheng et al., 2017, 2019; Guo et al., 2017; Miao et al., 2020). At the phylum level, the microbiota diversity of the AB group decreased, Proteobacteria became a dominant phylum, and the abundance of Bacteroidetes, Firmicutes, Actinobacteria, and Deferribacteres decreased. This result is supported by studies that ceftriaxone could characteristically decrease the alpha-diversity of the fecal microbiota accompanied with more Proteobacteria and less Bacteroidetes (Cheng et al., 2017, 2019; Miao et al., 2020). In some dysbiosis and related diseases, an increased Proteobacteria is perceived as a diagnostic characteristic since it is closely related to colon epithelial oxygenation as well as the disruption of the gut anaerobic environment (Zhu et al., 2013; Shin et al., 2015; Miao et al., 2020). Firmicutes has become a controversial strain as some studies identified an increase in Firmicutes after ceftriaxone treatment, but others have demonstrated a declined Firmicutes in the ceftriaxone group (Cheng et al., 2017, 2019; Miao et al., 2020). Evidence from clinics has suggested that patients with depression often have decreased Firmicutes (Huang et al., 2018). Experimental findings further revealed that decreased Firmicutes led to a reduction in short-chain fatty acids, which are an important physiological basis for low-level inflammation during depression (Huang et al., 2018). Bacteroidetes, as an important microbe for short-chain fatty acids, almost disappeared from the feces of the mice during exposure to ceftriaxone (Miao et al., 2020). Significant alteration of fecal microbiota was also observed at the genus level: Porphyromonadaceae, Escherichia, and Parabacteroides dominated the gut microbiota of the AB group mice, while Lactobacillus, Clostridiales, Acetatifactor, Bacteroidetes, Barnesiella, Helicobacter, Prevotella, Bacteroidales, and Alistipes were lowered. In line with this, some researchers have proposed that decreased Barnesiella after ceftriaxone gavage is a common and sensitive gut microbiota of the BALB/c mice and can be used as an indicator for assessing the balance of the gut microbiota (Zhao et al., 2013). Bacteroidetes is closely associated with digestion and interacts with the host's immune system, affecting the growth of other bacteria (Karlsson et al., 2011). In addition, an increase in Escherichia prevalence after oral antibiotic treatment has been reported for vancomycin and imipenem (Stokes, 1949), amoxicillin, bismuth (Dawes and Foster, 1956), and metronidazole (Paege and Gibbs, 1961). It is difficult to discern whether an increase in Escherichia could be beneficial or harmful as Escherichia is both a commensal and pathogenic inhabitant of a host's gastrointestinal tract. But most of the time, Escherichia is considered a potential pro-inflammatory bacteria (Liu et al., 2019). Of particularly note, increased Porphyromonadaceae associates with mental deficits and cognitive disorders as well as anxiety-like behaviors in mice (Scott et al., 2017). Lactobacillus is known as a protective species against long-lasting metabolic disturbances and prevents gut dysbiosis, but was suppressed by ceftriaxone (Robles-Vera et al., 2018). Researchers also discovered that elevated Parabacteroide relates to the etiology of depression (Cheung et al., 2019). These results indicate, once again, that different bacteria may be involved in different functions or biological pathways.
Alterations in gut microbiota were accompanied by behavioral changes in the mice, including anxiety-like, depression-like, and aggressive behaviors. These behavioral changes cannot necessarily be a result of the direct toxic effect of ceftriaxone on the brain, since ceftriaxone is a non-absorbable antibiotic and usually given by injection. Previous studies have demonstrated the complex interaction between gut microbiota and the CNS; this is what is known as the MGB axis (Wang and Wang, 2016). Animal experiments support that absence or change in gut microbiota affects the HPA axis answering to stress, anxiety, and relevant behavior (Koopman and El Aidy, 2017; Lach et al., 2018; Chen et al., 2019). In addition, the rodents infected with intestinal pathogens showed anxiety-like behaviors, which can be partly explained by the activation of vagal afferents (Klarer et al., 2014). In one study of GF BALB/c with a high-anxiety level and NIH Swiss mice with a high exploratory ability, when the two groups exchanged each other's microbiota, the donor behavioral characteristics could be reproduced in recipients (Crumeyrolle-Arias et al., 2014). On the other hand, clinical trials suggest treatment with probiotics could control the stress response and improve anxiety symptoms by restoring the gut microbiota (Liu et al., 2015). Our further work will test whether probiotics could improve the abnormal behaviors. At present, two major types of probiotics are commonly used: Bifidobacterium and Lactobacillus (Logan and Katzman, 2005; Rao et al., 2009; Silk et al., 2009). According to Wang et al., Lactobacillus fermentum strain NS9 administration not only normalized the composition of gut microbiota but reduced the anxiety-like behavior induced by ampicillin (Wang et al., 2015). Furthermore, the antidepressant effect of Bifidobacterium infantis has also been identified in the rat separation model of depression (Desbonnet et al., 2010).
Immune dysregulation was demonstrated by high levels of serum cytokines. This is supported by the evidence that inflammatory factors associate with a profile of behavioral changes (Capuron and Miller, 2011; Salim et al., 2012; Felger and Lotrich, 2013). Vagal sensory neurons express receptors for cytokines, so the inflammatory factors could directly activate the vagal afferents (Reardon et al., 2018). One study proposes that anxiety is related to inflammation; for example, mice infected with Schistosoma mansoni showed a reduction in behaviors such as exploration and grooming (Sulaiman et al., 1989). In addition, abnormal emotions, such as anxiety and neophobia, could happen following bacterial infection or as a response to bacterial products (Capuron and Miller, 2011). Anxiety levels increased when humans were exposed to lipopolysaccharide (LPS) (Grigoleit et al., 2011). Of the numerous cytokines, IL-6 is perceived as an atypical proinflammatory cytokine, having been demonstrated to show elevated levels in depressed animals and patients (Jiang et al., 2020; Lamers et al., 2020). In a study, IL-6 knockout mice became resistant to the development of depression-like symptoms (Monje et al., 2011). The underlying mechanisms involve in two pathways, the HPA axis and neurotransmitter metabolism, both of which are affected by increased IL-6 in depression (Ting et al., 2020). Furthermore, anxious patients also had higher serum levels of IL-6 than common people (Tang et al., 2018; Zou et al., 2020). In addition to impact on HPA axis activity, IL-6 could cross the blood–brain barrier, as they affect the uptake and release of mood-relevant neurotransmitters, including dopamine, 5-HT, noradrenaline, and gamma-aminobutyric acid (Zalcman et al., 1994; Clement et al., 1997; Anisman et al., 2008; Miller, 2009). IL-10, a prototypical anti-inflammatory cytokine, was closely related to depression (Li et al., 2020). Lower IL-10 has been observed in depression, while IL-10 was elevated after antidepressant treatment (Dai et al., 2020; Lee et al., 2020). In contrast, studies have reported higher IL-10 in depressive patients and decreased IL-10 after treatment for depression (Köhler et al., 2018; Himmerich et al., 2019; Wang et al., 2019; Brunoni et al., 2020). One explanation for increased IL-10 is that it is an anti-inflammatory response to correct an inflammatory activation caused by higher levels of proinflammatory cytokines (Bhattacharya and Drevets, 2017). In this way, higher IL-10 levels, as observed in our study, may be associated with the development of abnormal patterns. On the other hand, previous studies found a high dose of IL-10 may induce anxiety in the OFT (Harvey et al., 2006), two other behavioral tests for anxiety detection (Munshi et al., 2019). Taken together with these experiment evidences, the abnormal pattern of mice may be a direct result of increased inflammatory mediators.
Elevated corticosterone, one marker of HPA axis activation, was observed in the mice of the AB group (Borrow et al., 2019). Several studies indicate that the disturbance of gut bacteria affects the HPA axis. Specifically, adrenocorticotrophin and corticosterone levels for GF mice were higher than of mice bearing conventional microbiota (Crumeyrolle-Arias et al., 2014). Besides, the hyperactivity of the HPA response in GF mice could be partially reversed by gut microbiota transplantation (Huo et al., 2017). Probiotics, such as Bifidobacterium species, have been demonstrated to be efficient in restoring HPA axis function (Moya-Pérez et al., 2017).
The BDNF level showed a decreasing trend in the hippocampus of the AB group. According to the previous study, BDNF can maintain and promote development, differentiation and regeneration of neurons as well as affect learning and memory (Bercik et al., 2011). The hippocampus provides the brain with a spatiotemporal framework within which various sensory, emotional, and cognitive components are integrated (Yang and Wang, 2017). Literature has reported that hippocampus degeneration with diminished BDNF leads to a decline in cognition (Deltheil et al., 2008). Recently, gut microbiota is thought to directly affect BDNF expression. The GF mice showed a decreased BDNF in the cortex and hippocampus (Bercik et al., 2011). This coincides with the thesis that reduction of BDNF after gut dysbiosis possibly leads to impairment of cognitive function (Frohlich et al., 2016). Contrary to the findings, some studies observed an increased BDNF in the amygdala and hippocampus when gut microbiota imbalance induced a decline in spatial memory (Desbonnet et al., 2015). In addition, Bifidobaterium adolescentis shows a promising anxiolytic and antidepressant property as it up-regulated BDNF expression by restoring the balance of gut microbiota (Guo Y. et al., 2019). A slight increase of c-Fos was observed in the amygdala of the AB group. C-Fos serves as a component of transcription factor AP-1 and biomarker of neuronal activation, playing a major role in processing emotion and motivation (Baulmann et al., 2000; Roberts et al., 2019). Abnormal activation of c-Fos in the brain may be related to gut disorders; for example, as compared to uninfected mice, a significantly increased c-Fos was observed in mice infected with Campylobacter jejuni (Goehler et al., 2008). Meanwhile, a study indicated c-Fos activation following immune activation; this finding was in accord with our findings that cytokines increased with increasing c-Fos (Lyte et al., 2006).
Conclusion
In general, we found that mice exposed to 11 weeks of ceftriaxone sodium treatment had a lower diversity and abundance of gut microbiota and showed more behavioral changes as compared to mice that were given normal saline. Dysregulation of the nerve-endocrine-immunological network may be a potential mechanism underlying abnormal behaviors induced by impaired gut microbiota. The study revealed the unknown side effects of antibiotics to a certain extent. Follow-up studies rebalancing the gut dysbacteriosis are required to further confirm the relationship between gut microbiota and brain function.
Data Availability Statement
The datasets generated for this study can be found in the NCBI, SRA accession: PRJNA592623.
Ethics Statement
The animal study was reviewed and approved by Animal Care Advisory Committee of Sichuan University.
Author Contributions
ZZ and HY put forward the hypothesis, and BW, CT, LZ, and ML guided and supervised the experimental investigation and practices. LM, HW, and JL were responsible for data collection and analysis. YY provided pathologic diagnosis. ZZ was the main executer and involved in the entire research process, from proposal, planning and execution to implementation and composition. All authors contributed to the article and approved the submitted version.
Conflict of Interest
The authors declare that the research was conducted in the absence of any commercial or financial relationships that could be construed as a potential conflict of interest.
Acknowledgments
The authors thank MY Li and YJ Zhou for their support and help during the study.
Supplementary Material
The Supplementary Material for this article can be found online at: https://www.frontiersin.org/articles/10.3389/fcimb.2020.00258/full#supplementary-material
Supplementary Figure 1. Behavioral data analysis for eleven weeks. (A) Data from the open field test and (B) tail suspension test. AB: antibiotic group (n = 20), CT: control group (n = 20). Four mice of AB group were kicked out at the tenth week of gavage due to serious injuries influencing mobility.
Abbreviations
MGB, microbiota-gut-brain; SPF, specific-pathogen-free; OFT, open-field test; TST, tail-suspension test; OTUs, operational taxonomic units; ELISA, enzyme-linked immunosorbent assay; BDNF, brain-derived neurotrophic factor; IL, interleukin; HPA, hypothalamic-pituitary-adrenal; CNS, central nervous system; BBB, blood–brain barrier; LPS, lipopolysaccharide; GF, germ-free.
References
Aleshukina, A. V. (2012). Pathogenesis of intestinal dysbacteriosis. Zh. Mikrobiol. Epidemiol. Immunobiol. 3, 74–8.
Angelakis, E. (2017). Weight gain by gut microbiota manipulation in productive animals. Microb. Pathog. 106, 162–170. doi: 10.1016/j.micpath.2016.11.002
Anisman, H., Merali, Z., and Hayley, S. (2008). Neurotransmitter, peptide and cytokine processes in relation to depressive disorder: comorbidity between depression and neurodegenerative disorders. Prog. Neurobiol. 85, 1–74. doi: 10.1016/j.pneurobio.2008.01.004
Antonopoulos, D. A., and Chang, E. B. (2016). Transplanting a microbial organ: the good, the bad, and the unknown. mBio 7, e00572–16. doi: 10.1128/mBio.00572-16
Baulmann, J., Spitznagel, H., Herdegen, T., Unger, T., and Culman, J. (2000). Tachykinin receptor inhibition and c-Fos expression in the rat brain following formalin-induced pain. Neuroscience 95, 813–820. doi: 10.1016/S0306-4522(99)00478-9
Bercik, P., Denou, E., Collins, J., Jackson, W., Lu, J., Jury, J., et al. (2011). The intestinal microbiota affect central levels of brain-derived neurotropic factor and behavior in mice. Gastroenterology 141, 599–609, 601–609. doi: 10.1053/j.gastro.2011.04.052
Bhattacharya, A., and Drevets, W. C. (2017). Role of neuro-immunological factors in the pathophysiology of mood disorders: implications for novel therapeutics for treatment resistant depression. Curr. Top. Behav. Neurosci. 31, 339–356. doi: 10.1007/7854_2016_43
Borrow, A. P., Heck, A. L., Miller, A. M., Sheng, J. A., Stover, S. A., Daniels, R. M., et al. (2019). Chronic variable stress alters hypothalamic-pituitary-adrenal axis function in the female mouse. Physiol. Behav. 209:112613. doi: 10.1016/j.physbeh.2019.112613
Braniste, V., Al-Asmakh, M., Kowal, C., Anuar, F., Abbaspour, A., Toth, M., et al. (2014). The gut microbiota influences blood-brain barrier permeability in mice. Sci. Transl. Med. 6, 158r–263r. doi: 10.1126/scitranslmed.3009759
Brunoni, A. R., Supasitthumrong, T., Teixeira, A. L., Vieira, E. L., Gattaz, W. F., Benseñor, I. M., et al. (2020). Differences in the immune-inflammatory profiles of unipolar and bipolar depression. J. Affect. Disord. 262, 8–15. doi: 10.1016/j.jad.2019.10.037
Capuron, L., and Miller, A. H. (2011). Immune system to brain signaling: neuropsychopharmacological implications. Pharmacol. Therapeut. 130, 226–238. doi: 10.1016/j.pharmthera.2011.01.014
Castagne, V., Moser, P., Roux, S., and Porsolt, R. D. (2011). Rodent models of depression: forced swim and tail suspension behavioral despair tests in rats and mice. Curr. Protoc. Neurosci. Chapter 8, Unit 8.10A. doi: 10.1002/0471142301.ns0810as55
Champagne-Jorgensen, K., Mian, M. F., Kay, S., Hanani, H., Ziv, O., McVey Neufeld, K., et al. (2020). Prenatal low-dose penicillin results in long-term sex-specific changes to murine behaviour, immune regulation, and gut microbiota. Brain Behav. Immun. 84, 154–163. doi: 10.1016/j.bbi.2019.11.020
Chen, Y. H., Bai, J., Wu, D., Yu, S. F., Qiang, X. L., Bai, H., et al. (2019). Association between fecal microbiota and generalized anxiety disorder: severity and early treatment response. J. Affect. Disord. 259, 56–66. doi: 10.1016/j.jad.2019.08.014
Cheng, R., Guo, J., Pu, F., Wan, C., Shi, L., Li, H., et al. (2019). Loading ceftriaxone, vancomycin, and Bifidobacteria bifidum TMC3115 to neonatal mice could differently and consequently affect intestinal microbiota and immunity in adulthood. Sci. Rep. 9, 3215–3254. doi: 10.1038/s41598-018-35737-1
Cheng, R. Y., Li, M., Li, S. S., He, M., Yu, X. H., Shi, L., et al. (2017). Vancomycin and ceftriaxone can damage intestinal microbiota and affect the development of the intestinal tract and immune system to different degrees in neonatal mice. Pathog. Dis. 75. doi: 10.1093/femspd/ftx104
Cheung, S. G., Goldenthal, A. R., Uhlemann, A. C., Mann, J. J., Miller, J. M., and Sublette, M. E. (2019). Systematic review of gut microbiota and major depression. Front. Psychiatry 10:34. doi: 10.3389/fpsyt.2019.00034
Clement, H., Buschmann, J., Rex, S., Grote, C., Opper, C., Gemsa, D., et al. (1997). Effects of interferon-γ, interleukin-1 β, and tumor necrosis factor-α on the serotonin metabolism in the nucleus raphe dorsalis of the rat. J. Neural Transm. 104, 981–991. doi: 10.1007/B.F.01273312
Crawley, J. N. (1985). Exploratory behavior models of anxiety in mice. Neurosci. Biobehav. Rev. 9, 37–44. doi: 10.1016/0149-7634(85)90030-2
Crumeyrolle-Arias, M., Jaglin, M., Bruneau, A., Vancassel, S., Cardona, A., Dauge, V., et al. (2014). Absence of the gut microbiota enhances anxiety-like behavior and neuroendocrine response to acute stress in rats. Psychoneuroendocrinology 42, 207–217. doi: 10.1016/j.psyneuen.2014.01.014
Dai, J., Pan, J. Y., Liao, N., Shi, J., Zeng, Q., Huang, L., et al. (2020). Influence of miR-155 on behaviors of depression mice through regulating Wnt/beta-catenin signaling pathway. Eur. Rev. Med. Pharmacol. Sci. 24, 1398–1407. doi: 10.26355/eurrev_202002_20197
Davis, M., Rainnie, D., and Cassell, M. (1994). Neurotransmission in the rat amygdala related to fear and anxiety. Trends Neurosci. 17, 208–214. doi: 10.1016/0166-2236(94)90106-6
Dawes, E. A., and Foster, S. M. (1956). The formation of ethanol in Escherichia coli. Biochim. Biophys. Acta 22, 253–265. doi: 10.1016/0006-3002(56)90148-2
Deltheil, T., Guiard, B. P., Cerdan, J., David, D. J., Tanaka, K. F., Repérant, C., et al. (2008). Behavioral and serotonergic consequences of decreasing or increasing hippocampus brain-derived neurotrophic factor protein levels in mice. Neuropharmacology 55, 1006–1014. doi: 10.1016/j.neuropharm.2008.08.001
Dere, E., De Souza-Silva, M. A., Spieler, R. E., Lin, J. S., Ohtsu, H., Haas, H. L., et al. (2004). Changes in motoric, exploratory and emotional behaviours and neuronal acetylcholine content and 5-HT turnover in histidine decarboxylase-KO mice. Eur. J. Neurosci. 20, 1051–1058. doi: 10.1111/j.1460-9568.2004.03546.x
Desbonnet, L., Clarke, G., Traplin, A. O., Sullivan, O., Crispie, F., Moloney, R. D., et al. (2015). Gut microbiota depletion from early adolescence in mice: implications for brain and behaviour. Brain Behav. Immun. 48, 165–173. doi: 10.1016/j.bbi.2015.04.004
Desbonnet, L., Garrett, L., Clarke, G., Kiely, B., Cryan, J. F., and Dinan, T. G. (2010). Effects of the probiotic Bifidobacterium infantis in the maternal separation model of depression. Neuroscience 170, 1179–1188. doi: 10.1016/j.neuroscience.2010.08.005
Di, T., Zhang, S., Hong, J., Zhang, T., and Chen, L. (2017). Hyperactivity of hypothalamic-pituitary-adrenal axis due to dysfunction of the hypothalamic glucocorticoid receptor in sigma-1 receptor knockout mice. Front. Mol. Neurosci. 10:287. doi: 10.3389/fnmol.2017.00287
Fadrosh, D. W., Ma, B., Gajer, P., Sengamalay, N., Ott, S., Brotman, R. M., et al. (2014). An improved dual-indexing approach for multiplexed 16S rRNA gene sequencing on the Illumina MiSeq platform. Microbiome 2:6. doi: 10.1186/2049-2618-2-6
Felger, J. C., and Lotrich, F. E. (2013). Inflammatory cytokines in depression: neurobiological mechanisms and therapeutic implications. Neuroscience 246, 199–229. doi: 10.1016/j.neuroscience.2013.04.060
Felix, K. M., Jaimez, I. A., Nguyen, T. V., Ma, H., Raslan, W. A., Klinger, C. N., et al. (2018). Gut microbiota contributes to resistance against pneumococcal pneumonia in immunodeficient rag–/– mice. Front. Cell Infect. Microbiol. 8:118. doi: 10.3389/fcimb.2018.00118
Frohlich, E. E., Farzi, A., Mayerhofer, R., Reichmann, F., Jacan, A., Wagner, B., et al. (2016). Cognitive impairment by antibiotic-induced gut dysbiosis: analysis of gut microbiota-brain communication. Brain Behav. Immun. 56, 140–155. doi: 10.1016/j.bbi.2016.02.020
Gareau, M. G., Wine, E., Rodrigues, D. M., Cho, J. H., Whary, M. T., Philpott, D. J., et al. (2011). Bacterial infection causes stress-induced memory dysfunction in mice. Gut 60, 307–317. doi: 10.1136/gut.2009.202515
Goehler, L. E., Park, S. M., Opitz, N., Lyte, M., and Gaykema, R. P. A. (2008). Campylobacter jejuni infection increases anxiety-like behavior in the holeboard: possible anatomical substrates for viscerosensory modulation of exploratory behavior. Brain Behav. Immun 22, 354–366. doi: 10.1016/j.bbi.2007.08.009
Grigoleit, J. S., Kullmann, J. S., Wolf, O. T., Hammes, F., Wegner, A., Jablonowski, S., et al. (2011). Dose-dependent effects of endotoxin on neurobehavioral functions in humans. PLoS ONE 6:e28330. doi: 10.1371/journal.pone.0028330
Guo, J., Lv, Q., Ariff, A., Zhang, X., Peacock, C. S., Song, Y., et al. (2019). Western oropharyngeal and gut microbial profiles are associated with allergic conditions in Chinese immigrant children. World Allergy Organ J. 12:100051. doi: 10.1016/j.waojou.2019.100051
Guo, Y., Xie, J., Deng, K., Li, X., Yuan, Y., Xuan, Q., et al. (2019). Prophylactic effects of Bifidobacterium adolescentis on anxiety and depression-like phenotypes after chronic stress: a role of the gut microbiota-inflammation axis. Front. Behav. Neurosci. 13:126. doi: 10.3389/fnbeh.2019.00126
Guo, Y., Yang, X., Qi, Y., Wen, S., Liu, Y., Tang, S., et al. (2017). Long-term use of ceftriaxone sodium induced changes in gut microbiota and immune system. Sci. Rep. 7:43035. doi: 10.1038/srep43035
Harris, L. A., and Baffy, N. (2017). Modulation of the gut microbiota: a focus on treatments for irritable bowel syndrome. Postgrad. Med. 129, 872–888. doi: 10.1080/00325481.2017.1383819
Harvey, D., Smith, R., English, K., Mahon, B., and Commins, S. (2006). Interleukin-10 (IL-10) but not Lipopolysaccharide (LPS) produces increased motor activity and abnormal exploratory patterns while impairing spatial learning in Balb/c mice. Physiol. Behav. 87, 842–847. doi: 10.1016/j.physbeh.2006.03.002
Himmerich, H., Patsalos, O., Lichtblau, N., Ibrahim, M., and Dalton, B. (2019). Cytokine research in depression: principles, challenges, and open questions. Front. Psychiatry 10:30. doi: 10.3389/fpsyt.2019.00030
Hiroshi, K., Itsuro, I., Toshimi, O., Mahito, K., Yumiko, I., Shin, N., et al. (2006). Assessment of the dexamethasone/CRH test as a state-dependent marker for hypothalamic-pituitary-adrenal (HPA) axis abnormalities in major depressive episode: a multicenter study. Neuropsychopharmacology 31, 212–220. doi: 10.1038/sj.npp.1300868
Huang, Y., Shi, X., Li, Z., Shen, Y., Shi, X., Wang, L., et al. (2018). Possible association of firmicutes in the gut microbiota of patients with major depressive disorder. Neuropsychiatr Dis Treat. 14, 3329–3337. doi: 10.2147/NDT.S188340
Huo, R., Zeng, B., Zeng, L., Cheng, K., Li, B., Luo, Y., et al. (2017). Microbiota modulate anxiety-like behavior and endocrine abnormalities in hypothalamic-pituitary-adrenal axis. Front. Cell. Infect. Microbiol. 7:489. doi: 10.3389/fcimb.2017.00489
Iannone, L. F., Preda, A., Blottiere, H. M., Clarke, G., Albani, D., Belcastro, V., et al. (2019). Microbiota-gut brain axis involvement in neuropsychiatric disorders. Expert Rev. Neurother. 19, 1037–1050. doi: 10.1080/14737175.2019.1638763
Janak, P. H., and Tye, K. M. (2015). From circuits to behaviour in the amygdala. Nature 517, 284–292. doi: 10.1038/nature14188
Jiang, N., Lv, J., Wang, H., Huang, H., Wang, Q., Lu, C., et al. (2020). Ginsenoside Rg1 ameliorates chronic social defeat stress-induced depressive-like behaviors and hippocampal neuroinflammation. Life Sci. 252:117669. doi: 10.1016/j.lfs.2020.117669
Karlsson, F. H., Ussery, D. W., Nielsen, J., and Nookaew, I. (2011). A closer look at bacteroides: phylogenetic relationship and genomic implications of a life in the human gut. Microb. Ecol. 61, 473–485. doi: 10.1007/s00248-010-9796-1
Kim, S., Covington, A., and Pamer, E. G. (2017). The intestinal microbiota: antibiotics, colonization resistance, and enteric pathogens. Immunol. Rev. 279, 90–105. doi: 10.1111/imr.12563
Klarer, M., Arnold, M., Gunther, L., Winter, C., Langhans, W., and Meyer, U. (2014). Gut vagal afferents differentially modulate innate anxiety and learned fear. J. Neurosci. 34, 7067–7076. doi: 10.1523/JNEUROSCI.0252-14.2014
Köhler, C. A., Freitas, T. H., Stubbs, B., Maes, M., Solmi, M., Veronese, N., et al. (2018). Peripheral alterations in cytokine and chemokine levels after antidepressant drug treatment for major depressive disorder: systematic review and meta-analysis. Mol. Neurobiol. 55, 4195–4206. doi: 10.1007/s12035-017-0632-1
Koopman, M., and El Aidy, S. (2017). Depressed gut? The microbiota-diet-inflammation trialogue in depression. Curr. Opin. Psychiatr. 30, 369–377. doi: 10.1097/YCO.0000000000000350
Kovács, K. J. (2008). Measurement of immediate-early gene activation- c-fos and beyond. J. Neuroendocrinol. 20, 665–672. doi: 10.1111/j.1365-2826.2008.01734.x
Kraeuter, A. K., Guest, P. C., and Sarnyai, Z. (2019). The open field test for measuring locomotor activity and anxiety-like behavior. Methods Mol. Biol. 1916, 99–103. doi: 10.1007/978-1-4939-8994-2_9
Lach, G., Schellekens, H., Dinan, T. G., and Cryan, J. F. (2018). Anxiety, depression, and the microbiome: a role for gut peptides. Neurotherapeutics 15, 36–59. doi: 10.1007/s13311-017-0585-0
Lamers, F., Milaneschi, Y., Vinkers, C. H., Schoevers, R. A., Giltay, E. J., and Penninx, B. W. J. H. (2020). Depression profilers and immuno-metabolic dysregulation: longitudinal results from the NESDA study. Brain Behav. Immun. doi: 10.1016/j.bbi.2020.04.002
Lee, H., Song, M., Lee, J., Kim, J., and Lee, M. (2020). Prospective study on cytokine levels in medication-naïve adolescents with first-episode major depressive disorder. J. Affect. Disord. 266, 57–62. doi: 10.1016/j.jad.2020.01.125
Lee, K., and Jayaraman, A. (2019). Interactions between gut microbiota and non-alcoholic liver disease: the role of microbiota-derived metabolites. Pharmacol. Res. 142:314. doi: 10.1016/j.phrs.2019.02.013
Li, K., Yan, L., Zhang, Y., Yang, Z., Zhang, C., Li, Y., et al. (2020). Seahorse treatment improves depression-like behavior in mice exposed to CUMS through reducing inflammation/oxidants and restoring neurotransmitter and neurotrophin function. J. Ethnopharmacol. 250:112487. doi: 10.1016/j.jep.2019.112487
Li, S., Li, J., Mao, G., Wu, T., Lin, D., Hu, Y., et al. (2019). Fucosylated chondroitin sulfate from Isostichopus badionotus alleviates metabolic syndromes and gut microbiota dysbiosis induced by high-fat and high-fructose diet. Int. J. Biol. Macromol. 124, 377–388. doi: 10.1016/j.ijbiomac.2018.11.167
Liu, D., Huang, Y., Chen, B., Zeng, J., Guo, N., Zhang, S., et al. (2011). Activation of mammalian target of rapamycin pathway confers adverse outcome in nonsmall cell lung carcinoma. Cancer 117, 3763–3773. doi: 10.1002/cncr.25959
Liu, Q., Li, F., Zhuang, Y., Xu, J., Wang, J., Mao, X., et al. (2019). Alteration in gut microbiota associated with hepatitis B and non-hepatitis virus related hepatocellular carcinoma. Gut Pathog. 11, 1–13. doi: 10.1186/s13099-018-0281-6
Liu, X., Cao, S., and Zhang, X. (2015). Modulation of gut microbiota–brain axis by probiotics, prebiotics, and diet. J. Agr. Food Chem. 63, 7885–7895. doi: 10.1021/acs.jafc.5b02404
Logan, A. C., and Katzman, M. (2005). Major depressive disorder: probiotics may be an adjuvant therapy. Med. Hypotheses 64, 533–538. doi: 10.1016/j.mehy.2004.08.019
Luczynski, P., Whelan, S. O., O'Sullivan, C., Clarke, G., Shanahan, F., Dinan, T. G., et al. (2016). Adult microbiota-deficient mice have distinct dendritic morphological changes: differential effects in the amygdala and hippocampus. Eur. J. Neurosci. 44, 2654–2666. doi: 10.1111/ejn.13291
Lyte, M., Li, W., Opitz, N., Gaykema, R. P. A., and Goehler, L. E. (2006). Induction of anxiety-like behavior in mice during the initial stages of infection with the agent of murine colonic hyperplasia Citrobacter rodentium. Physiol. Behav. 89, 350–357. doi: 10.1016/j.physbeh.2006.06.019
Mayer, E. A., Tillisch, K., and Gupta, A. (2015). Gut/brain axis and the microbiota. J. Clin. Invest. 125, 926–938. doi: 10.1172/JCI76304
Miao, Z., Cheng, R., Zhang, Y., Liang, H., Jiang, F., Shen, X., et al. (2020). Antibiotics can cause weight loss by impairing gut microbiota in mice and the potent benefits of Lactobacilli. Biosci. Biotechnol. Biochem. 84, 411–420. doi: 10.1080/09168451.2019.1676696
Miller, A. H. (2009). Mechanisms of cytokine-induced behavioral changes: psychoneuroimmunology at the translational interface. Brain Behav. Immun. 23, 149–158. doi: 10.1016/j.bbi.2008.08.006
Młyniec, K., and Nowak, G. (2012). Zinc deficiency induces behavioral alterations in the tail suspension test in mice. Effect of antidepressants. Pharmacol. Rep. 64, 249–255. doi: 10.1016/S1734-1140(12)70762-4
Monje, F. J., Cabatic, M., Divisch, I., Kim, E. J., Herkner, K. R., Binder, B. R., et al. (2011). Constant darkness induces IL-6-dependent depression-like behavior through the NF- κB signaling pathway. J. Neurosci. 31, 9075–9083. doi: 10.1523/JNEUROSCI.1537-11.2011
Moya-Pérez, A., Perez-Villalba, A., Benítez-Páez, A., Campillo, I., and Sanz, Y. (2017). Bifidobacterium CECT 7765 modulates early stress-induced immune, neuroendocrine and behavioral alterations in mice. Brain Behav. Immun. 65, 43–56. doi: 10.1016/j.bbi.2017.05.011
Munshi, S., Parrilli, V., and Rosenkranz, J. A. (2019). Peripheral anti-inflammatory cytokine Interleukin-10 treatment mitigates interleukin-1β - induced anxiety and sickness behaviors in adult male rats. Behav. Brain Res. 372:112024. doi: 10.1016/j.bbr.2019.112024
Neufeld, K. M., Kang, N., Bienenstock, J., and Foster, J. A. (2011). Reduced anxiety-like behavior and central neurochemical change in germ-free mice. Neurogastroenterol. Motil. 23, 119–255. doi: 10.1111/j.1365-2982.2010.01620.x
Paege, L. M., and Gibbs, M. (1961). Anaerobic dissimilation of glucose-c14 by Escherichia coli. J. Bacteriol. 81, 107–110. doi: 10.1128/JB.81.1.107-110.1961
Rao, A. V., Bested, A. C., Beaulne, T. M., Katzman, M. A., Iorio, C., Berardi, J. M., et al. (2009). A randomized, double-blind, placebo-controlled pilot study of a probiotic in emotional symptoms of chronic fatigue syndrome. Gut Pathog. 1:6. doi: 10.1186/1757-4749-1-6
Reardon, C., Murray, K., and Lomax, A. E. (2018). Neuroimmune communication in health and disease. Physiol. Rev. 98, 2287–2316. doi: 10.1152/physrev.00035.2017
Ren, D., Gong, S., Shu, J., Zhu, J., Liu, H., and Chen, P. (2018). Effects of mixed lactic acid bacteria on intestinal microbiota of mice infected with Staphylococcus aureus. BMC Microbiol. 18:109. doi: 10.1186/s12866-018-1245-1
Roberts, A. J., Khom, S., Bajo, M., Vlkolinsky, R., Polis, I., Cates-Gatto, C., et al. (2019). Increased IL-6 expression in astrocytes is associated with emotionality, alterations in central amygdala GABAergic transmission, and excitability during alcohol withdrawal. Brain Behav. Immun. 82, 188–202. doi: 10.1016/j.bbi.2019.08.185
Robles-Vera, I., Toral, M., de la Visitacion, N., Sanchez, M., Romero, M., Olivares, M., et al. (2018). The probiotic Lactobacillus fermentum prevents dysbiosis and vascular oxidative stress in rats with hypertension induced by chronic nitric oxide blockade. Mol. Nutr. Food Res. 62:e1800298. doi: 10.1002/mnfr.201800298
Salim, S., Chugh, G., and Asghar, M. (2012). Inflammation in anxiety. Adv. Protein Chem. Struct. Biol. 88, 1–25. doi: 10.1016/B978-0-12-398314-5.00001-5
Scott, K. A., Ida, M., Peterson, V. L., Prenderville, J. A., Moloney, G. M., Izumo, T., et al. (2017). Revisiting metchnikoff: age-related alterations in microbiota-gut-brain axis in the mouse. Brain Behav. Immun. 65, 20–32. doi: 10.1016/j.bbi.2017.02.004
Scriven, M., Dinan, T., Cryan, J., and Wall, M. (2018). Neuropsychiatric disorders: influence of gut microbe to brain signalling. Diseases 6:78. doi: 10.3390/diseases6030078
Shin, N., Whon, T. W., and Bae, J. (2015). Proteobacteria: microbial signature of dysbiosis in gut microbiota. Trends Biotechnol. 33, 496–503. doi: 10.1016/j.tibtech.2015.06.011
Silk, D. B. A., Davis, A., Vulevic, J., Tzortzis, G., and Gibson, G. R. (2009). Clinical trial: the effects of a trans-galactooligosaccharide prebiotic on faecal microbiota and symptoms in irritable bowel syndrome. Aliment. Pharmacol. Ther. 29, 508–518. doi: 10.1111/j.1365-2036.2008.03911.x
Slykerman, R. F., Coomarasamy, C., Wickens, K., Thompson, J., Stanley, T. V., Barthow, C., et al. (2019). Exposure to antibiotics in the first 24 months of life and neurocognitive outcomes at 11 years of age. Psychopharmacology 236, 1573–1582. doi: 10.1007/s00213-019-05216-0
Stokes, J. L. (1949). Fermentation of glucose by suspensions of Escherichia coli. J. Bacteriol. 57, 147–158. doi: 10.1128/JB.57.2.147-158.1949
Sudo, N., Chida, Y., Aiba, Y., Sonoda, J., Oyama, N., Yu, X. N., et al. (2004). Postnatal microbial colonization programs the hypothalamic-pituitary-adrenal system for stress response in mice. J. Physiol. 558, 263–275. doi: 10.1113/jphysiol.2004.063388
Sulaiman, M. I., Amin, A. M., and Mikhail, E. G. (1989). Effect of schistosomiasis mansoni on open-field exploratory behavior in mice. J. Egypt. Soc. Parasitol. 19, 369–379.
Tang, Z., Ye, G., Chen, X., Pan, M., Fu, J., Fu, T., et al. (2018). Peripheral proinflammatory cytokines in Chinese patients with generalised anxiety disorder. J. Affect. Disord. 225, 593–598. doi: 10.1016/j.jad.2017.08.082
Thursby, E., and Juge, N. (2017). Introduction to the human gut microbiota. Biochem. J. 474, 1823–1836. doi: 10.1042/BCJ20160510
Ting, E. Y., Yang, A. C., and Tsai, S. (2020). Role of interleukin-6 in depressive disorder. Int. J. Mol. Sci. 21:2194. doi: 10.3390/ijms21062194
Torres-Fuentes, C., Schellekens, H., Dinan, T. G., and Cryan, J. F. (2017). The microbiota–gut–brain axis in obesity. Lancet Gastroenterol. Hepatol. 2, 747–756. doi: 10.1016/S2468-1253(17)30147-4
Wang, H., and Wang, Y. (2016). Gut Microbiota-brain Axis. Chinese Med. J. Peking 129, 2373–2380. doi: 10.4103/0366-6999.190667
Wang, L., Wang, R., Liu, L., Qiao, D., Baldwin, D. S., and Hou, R. (2019). Effects of SSRIs on peripheral inflammatory markers in patients with major depressive disorder: a systematic review and meta-analysis. Brain Behav. Immun. 79, 24–38. doi: 10.1016/j.bbi.2019.02.021
Wang, T., Hu, X., Liang, S., Li, W., and Jin, F. (2015). Lactobacillus fermentum NS9 restores the antibiotic induced physiological and psychological abnormalities in rats. Benef. Microbes 6, 707–717. doi: 10.3920/BM2014.0177
Wiener, C. D., Moreira, F. P., Portela, L. V., Strogulski, N. R., Lara, D. R., Da Silva, R. A., et al. (2019). Interleukin-6 and Interleukin-10 in mood disorders: a population-based study. Psychiatry Res. 273, 685–689. doi: 10.1016/j.psychres.2019.01.100
Yang, Y., and Wang, J. (2017). From structure to behavior in basolateral amygdala-hippocampus circuits. Front. Neural Circuit. 11:86. doi: 10.3389/fncir.2017.00086
Yuan, X., Kang, Y., Zhuo, C., Huang, X., and Song, X. (2019). The gut microbiota promotes the pathogenesis of schizophrenia via multiple pathways. Biochem. Bioph. Res. Commun. 512, 373–380. doi: 10.1016/j.bbrc.2019.02.152
Zalcman, S., Green-Johnson, J. M., Murray, L., Nance, D. M., Dyck, D., Anisman, H., et al. (1994). Cytokine-specific central monoamine alterations induced by interleukin-1,−2 and−6. Brain Res. 643, 40–49. doi: 10.1016/0006-8993(94)90006-X
Zhao, Y., Wu, J., Li, J. V., Zhou, N. Y., Tang, H., and Wang, Y. (2013). Gut microbiota composition modifies fecal metabolic profiles in mice. J. Proteome Res. 12, 2987–2999. doi: 10.1021/pr400263n
Zhu, L., Baker, S. S., Gill, C., Liu, W., Alkhouri, R., Baker, R. D., et al. (2013). Characterization of gut microbiomes in nonalcoholic steatohepatitis (NASH) patients: a connection between endogenous alcohol and NASH. Hepatology 57, 601–609. doi: 10.1002/hep.26093
Keywords: gut microbiota, emotional behaviors, ceftriaxone sodium, anxiety, depression, aggressive behavior
Citation: Zhao Z, Wang B, Mu L, Wang H, Luo J, Yang Y, Yang H, Li M, Zhou L and Tao C (2020) Long-Term Exposure to Ceftriaxone Sodium Induces Alteration of Gut Microbiota Accompanied by Abnormal Behaviors in Mice. Front. Cell. Infect. Microbiol. 10:258. doi: 10.3389/fcimb.2020.00258
Received: 24 December 2019; Accepted: 04 May 2020;
Published: 24 June 2020.
Edited by:
Andreas Martin Grabrucker, University of Limerick, IrelandReviewed by:
Kiran Veer Sandhu, University College Cork, IrelandAli-Akbar Salari, Salari Institute of Cognitive and Behavioral Disorders (SICBD), Iran
Copyright © 2020 Zhao, Wang, Mu, Wang, Luo, Yang, Yang, Li, Zhou and Tao. This is an open-access article distributed under the terms of the Creative Commons Attribution License (CC BY). The use, distribution or reproduction in other forums is permitted, provided the original author(s) and the copyright owner(s) are credited and that the original publication in this journal is cited, in accordance with accepted academic practice. No use, distribution or reproduction is permitted which does not comply with these terms.
*Correspondence: Linlin Zhou, emhvdWxpbmxpbkBzY3UuZWR1LmNu; Chuanmin Tao, dGFvY21Ac2N1LmVkdS5jbg==
†Lead author