- 1APC Microbiome Ireland, University College Cork, Cork, Ireland
- 2Food Bioscience Department, Teagasc Food Research Centre, Fermoy, Ireland
- 3Gut Microbes and Health, Quadram Institute Bioscience, Norwich, United Kingdom
Fungi have been used since ancient times in food and beverage-making processes and, more recently, have been harnessed for the production of antibiotics and in processes of relevance to the bioeconomy. Moreover, they are starting to gain attention as a key component of the human microbiome. However, fungi are also responsible for human infections. The incidence of community-acquired and nosocomial fungal infections has increased considerably in recent decades. Antibiotic resistance development, the increasing number of immunodeficiency- and/or immunosuppression-related diseases and limited therapeutic options available are triggering the search for novel alternatives. These new antifungals should be less toxic for the host, with targeted or broader antimicrobial spectra (for diseases of known and unknown etiology, respectively) and modes of actions that limit the potential for the emergence of resistance among pathogenic fungi. Given these criteria, antimicrobial peptides with antifungal properties, i.e., antifungal peptides (AFPs), have emerged as powerful candidates due to their efficacy and high selectivity. In this review, we provide an overview of the bioactivity and classification of AFPs (natural and synthetic) as well as their mode of action and advantages over current antifungal drugs. Additionally, natural, heterologous and synthetic production of AFPs with a view to greater levels of exploitation is discussed. Finally, we evaluate the current and potential applications of these peptides, along with the future challenges relating to antifungal treatments.
Introduction
Fungi are extraordinary, ubiquitous organisms that play critical roles in complex ecosystems. These eukaryotes range from giant forms to microscopic unicellular molds and yeasts. In recent years fungi have been recognized as an integral part of our commensal microbiota at different body sites (e.g., gut, oral cavity, skin, lung, vagina), although there is no consensus on what constitutes the standard mycobiome composition (Huffnagle and Noverr, 2013; Enaud et al., 2018; Kapitan et al., 2018) and some studies point out that gut fungi may come from oral and dietary sources (Auchtung et al., 2018). Indeed, fungi have been used as a source of food and for food processing for thousands of years (Campbell-Platt and Cook, 2008). Fungi are also routinely employed in many industrial processes including the production of peptides, enzymes, vitamins, organic acids, and antibiotics (Money, 2016; Mukherjee et al., 2018).
However, fungal infections, or mycoses, have become a serious threat to human health, causing a wide range of infections in humans. It is estimated that fungal diseases affect more than one billion people globally, of whom 150 million suffer from severe infections (Bongomin et al., 2017). These range from superficial and subcutaneous life quality-debilitating infections affecting the skin, keratinous tissues and mucous membranes (Kaushik et al., 2015), to systemic infections that can be life-threatening involving the brain, heart, lungs, liver, spleen, and kidneys (Rautemaa-Richardson and Richardson, 2017). The latter are especially worrying in the case of immunocompromised patients with HIV/AIDS or autoimmune diseases, and in those undergoing anticancer chemotherapy or organ transplantation.
The main human fungal pathogens are Candida albicans, Cryptococcus neoformans, and Aspergillus fumigatus, but, worryingly, non-albicans Candida spp. such as C. auris, in addition to other infectious agents such as Histoplasma capsulatum or Malassezia furfur are emerging. For a comprehensive review on main fungal pathogens affecting humans see (Roemer and Krysan, 2014).
Four major classes of antifungal agents dominate the market: azoles, which inhibit the synthesis of ergosterol; polyenes, which interact with fungal membrane sterols physicochemically; echinocandins that inhibit glucan synthesis; and fluorinated pyrimidines, which interfere with pyrimidine metabolism, leading to the inhibition of DNA and RNA biosynthesis (Roemer and Krysan, 2014). However, the high mortality of invasive fungal infections, the long course of treatments required, narrow spectrum activity and cross-resistance due to similar mechanisms of action across drugs has triggered the search for safer alternatives with reduced toxicity or other enhanced features. As eukaryotes, a particularly great challenge is to identify pathogen-specific targets not present in human cells.
Monoclonal antibodies, cytokine immunotherapy, vaccines and antimicrobial peptides (AMPs) have emerged as new biopharmaceuticals to prevent or treat fungal infections (Nicola et al., 2019). There is an increasing interest in peptides as promising novel antibiotic agents. Peptides can mimic natural ligands and therefore function as agonists or antagonists. Regarding their use as drugs, peptides are highly selective, effective and well-tolerated (Fosgerau and Hoffmann, 2015). Among the broader peptide category of antimicrobials, AMPs are gene-encoded conserved molecules produced by all organisms, from bacteria to humans. Compared with conventional antibiotics, which are generally targeted against bacteria or fungi, AMPs can exhibit broad antimicrobial activity including bacteria, fungi, parasites, viruses, protozoa and even some cancer cells (Hancock and Chapple, 1999). Being effective against this broad range of targets might imply different modes of action and prevent bacteria and fungi from developing resistance. AMPs produced by higher organisms are involved in the innate and secondary immune responses against microbes, while those produced by bacteria frequently kill other bacteria competing for the same ecological niche (Zhang and Gallo, 2016). AMPs also confer protection by contributing to gut homeostasis, and modulation of host inflammatory responses. Notably, AMPs with a narrow antimicrobial spectrum have particularly great therapeutic potential as they are less likely to cause disruption of the host microbiota.
In this review we provide an overview of the bioactivity and classification of AMPs with antifungal activity, known as antifungal peptides (AFPs), as well as their mode of action and advantages over current antifungal drugs. Additionally, natural, heterologous and synthetic production of AFPs with a view to greater levels of exploitation is discussed. In this regard, Figure 1 shows a general overview on AFP development. Finally, we evaluate the current and potential applications of these peptides, together with future challenges in relation to antifungal treatments.
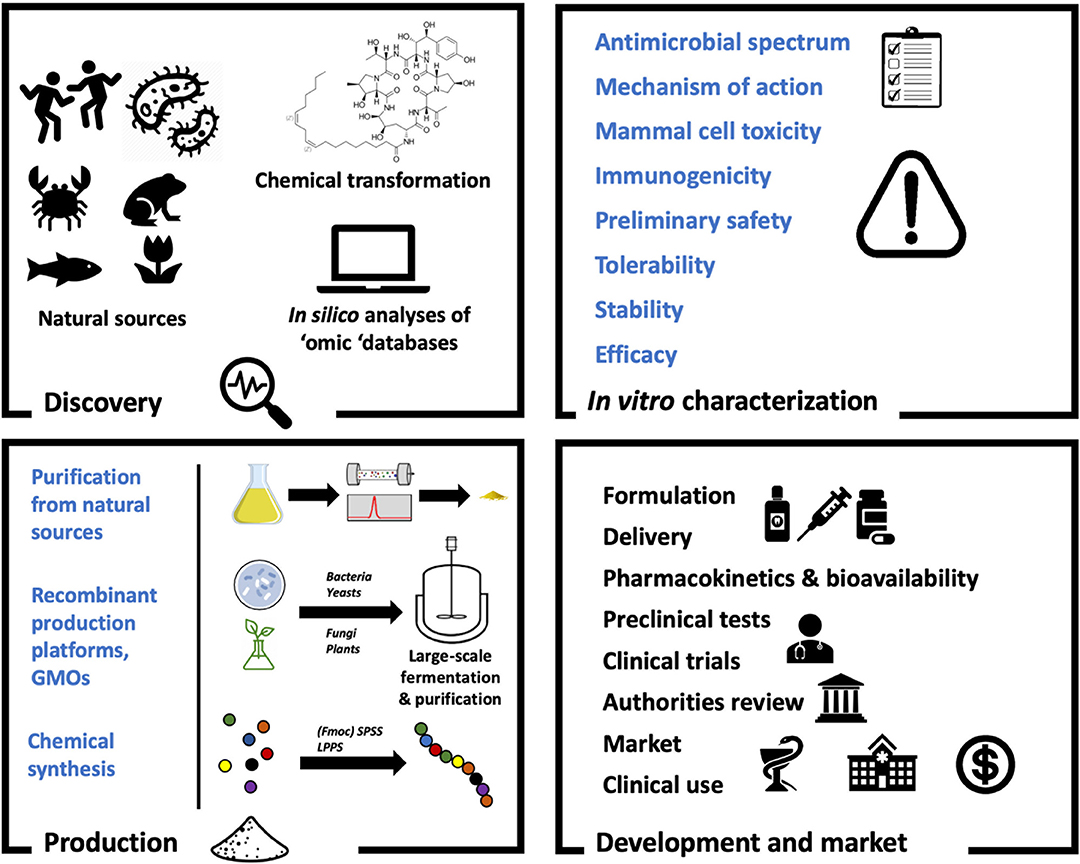
Figure 1. The antifungal peptide development process. As with any drugs, AFPs must undergo several stages of development to reach clinical use. When the candidate molecule shows promise as a therapeutic (Discovery) it must be characterized (In vitro characterization). In order to facilitate this, sufficient amounts of the peptide must be available (Production). Finally, the molecule will be subjected to formulation processes and preclinical tests before going into clinical trials and receive approval (Development and market).
Types of Antifungal Peptides and Bioactivity
As of November 2019, there were 1,133 peptides with antifungal properties reported in the Antimicrobial Peptide Database (APD3) (Wang et al., 2016). AFPs have been classified following a number of different criteria, such as structure or mode of action. However, the most accepted classification is based on the peptide origin: natural, semisynthetic or synthetic (De Lucca, 2000). Here, we summarize some of the most important features of natural peptides and we describe how synthetic AFPs are designed.
Natural Peptides
Natural AFPs are produced by a number of different species of Bacteria, Archaea, and Eukarya isolated from natural sources (De Lucca and Walsh, 1999). Most natural AFPs have been discovered by testing their antagonistic activity in vitro against pathogenic fungi (Mania et al., 2010; Freitas and Franco, 2016; McNair et al., 2018). However, with the rise of sequencing technologies and the drop in associated costs, new strategies for prediction and discovery of new AFPs are emerging. New methods such as template-based, docking simulations, hidden Markov model and other sequence-based methods allow for novel in silico prediction of AFPs (Schneider and Fechner, 2005; Fjell et al., 2007, 2008; Robinson, 2011; Garrigues et al., 2017; Agrawal et al., 2018).
Natural AFPs are grouped in families according to their origin (Table 1). Those produced by bacteria and fungi are arguably of greatest interest with respect to medical applications (Essig et al., 2014). Although some other sources, such as plants, are a rich source of AFPs, they are mainly employed for other purposes, such as the control of phytopathogens, and thus, they are not further discussed in this review (De Lucca, 2000). Notably, the first archaeal AMP with antifungal and anti-biofilm capabilities against clinical fungal pathogens was recently reported (Roscetto et al., 2018).
Typically, natural AFPs adopt an α-helix structure, β-hairpin or sheet (containing two cysteine residues) or mixed α-helix/β-sheet structures upon interaction with membranes. Some of these peptides are rich in specific amino acids and, as a result, are classified as glycine-rich, proline-rich, arginine-rich, histidine-rich, and tryptophan-rich (Bondaryk et al., 2017). However, the structure of most AFPs has not yet been determined.
Posttranslational modifications also play a major role in the final three-dimensional structure and bioactivity of AMPs but, unfortunately, cannot be predicted with current in silico tools (Agrawal et al., 2018). The most common posttranslational modifications in natural AFPs involve glycosylation or the addition of carbohydrates, normally observed in asparagine, or serine/threonine residues (Guo et al., 2012; Bednarska et al., 2017). The latter has been shown to improve antifungal activity in some cases. Other modifications include halogenation such as chlorination (Andreu and Rivas, 1998; Shinnar et al., 2003), phosphorylation, which can increase stability (McDonald et al., 2011) or hydroxylation, mainly observed at lysine, arginine, tryptophan, and phenylalanine residues with differing effects on antifungal activity (Houwaart et al., 2014; Akkam, 2016). Finally, cyclization of AMPs, which is not considered a posttranslational modification, improves antimicrobial activity in general, reduces toxicity and improves stability against proteases (Akkam, 2016).
Semisynthetic and Synthetic Peptides and Structure Activity Relationships (SAR) Criteria for Their Design
Antimicrobial semisynthetic and synthetic peptides are generally made with a view to improving pharmacological properties, reducing side effects and/or lowering the immunogenicity of natural peptides. Pharmaceutical formulation will also help to enhance their stability and bioavailability. As an example of synthetic transformation, it was found that the hemolytic activity of the natural echinocandin B AFP was significantly reduced by the replacement of the linoleoyl side-chain with either octyloxybenzoyl (cilofungin) or pentyloxyterphenyl (anidulafungin) side chains (Emri et al., 2013).
Structure-activity relationships (SAR) are a key element used for the design and development of synthetic peptides (Lum et al., 2015). There are several biophysical properties that can determine antifungal activity, such as net charge, stereospecificity, hydrophobicity, amphipathicity, secondary structure and peptide length, with some of these characteristics being interdependent. These properties have been extensively reviewed previously (Akkam, 2016). Most AFPs are cationic, but neutral and anionic AFPs have also been described. When present, cationic charges play a role in the electrostatic binding of AMPs to negatively charged membranes. Therefore, an increase in the positive net charge beyond a threshold might lead to a stronger activity on the membrane. However, positive charges are not a prerequisite for antimicrobial activity, and anionic peptides interact with the membrane peptide through specific amino acid distributions (Yeaman and Yount, 2003; Lakshminarayanan et al., 2014).
Most AFPs are non-stereospecific (Akkam, 2016). In the same way, there is no dominant conformation among AFPs. Thus, the main differences between peptides come from sequence and secondary structure variation (Emri et al., 2013). Hydrophobicity and amphipathicity are essential factors for peptide membrane interactions and membrane permeabilization (Lum et al., 2015), and important variables in the design of synthetic peptides. Hydrophobicity is defined as the percentage of hydrophobic residues within a peptide, ranging between 30 and 60% for most AMPs. An increase in hydrophobicity and amphipathicity usually correlates with an increase in antifungal activity, but also with higher hemolytic activity. The presence of tryptophan is also linked to hemolytic activity since it interferes with lipid polymorphism in the membranes (Schibli et al., 2002).
The peptide length of AFPs is important for the secondary structure and mode of action. Most AFPs have 11–40 residues. It has been described that 7–8 amino acids are needed to form amphipathic structures in AMPs (Bahar and Ren, 2013), while <20 amino acids limit the ability of a peptide to form transmembrane structures in the fungal membrane (Rothstein et al., 2001; Akkam, 2016). Nevertheless, longer lengths may also affect cytotoxicity, stability and manufacturing costs. In order to overcome these hurdles, short antimicrobial peptides (SAMPs), containing 2–10 amino acids, are attracting attention as less toxic and more stable alternatives. Overall, SAMPs have simpler amino acid composition and they are easier to synthesize and modify chemically with a view to improving toxicity, stability, half-life or specificity. Moreover, they are also less immunogenic (Duncan and O'Neil, 2013; Fox, 2013).
Some of the semisynthetic and synthetic peptides studied with a view to clinical applications are summarized in Table 2. Considering all of the factors mentioned above, different strategies have been employed for synthetic peptide design. Combinatorial libraries generate model peptides suitable for SAR studies (Blondelle and Lohner, 2000). The template-based strategy, known as de novo design, uses peptides with known antifungal activity as scaffolds (Lum et al., 2015). As an example, Agrawal et al., performed in silico studies by machine learning techniques based on AFP and non-AFP sequences revealing a higher frequency of certain residues (C, G, H, K, R, and Y) and the prevalence of R, V, K at N-terminus and C and H at the C-terminus of peptides (Agrawal et al., 2018).
Another strategy is to target virulence traits (Bondaryk et al., 2017). However, this approach is not efficient enough due to the expression of different virulence factors in fungi cells. For this reason, the generation of molecules with multiple ligands like dendrimers, or tree-like molecules, that have radially distributed layers of branches named generations, have been explored (Esfand and Tomalia, 2001).
Models of Mechanisms of Action
Whereas some peptides have primarily antifungal activity, such as lipopeptides (e.g., echinocandins) or histidine-rich (e.g., the linear histatins or branched HK), membrane-disrupting peptides (e.g., magainins, protegrins) have a broader antimicrobial spectrum, including bacteria, fungi and viruses. Here, we summarize the hypothetical mechanisms of action of AFPs with activity against fungal pathogens.
Peptides With Primarily Antifungal Properties
Inhibition of 1,3-β-Glucan Synthesis
β-glucan synthase is involved in cell wall integrity. Cyclic lipoproteins can non-competitively inhibit it, resulting in destabilization of the cell wall, leading to susceptibility to osmotic stresses and cell lysis. 1,3-β-glucans are involved in the division septum and assembly of the acropore wall as well; consequently, β-glucan synthase inhibitors affect these structures. β-glucan synthase is ubiquitous among fungi including Candida, Aspergillus, Cryptococcus, and Pneumocystis species. However, in mycelious fungi, it is found in tips of the growing hyphae, which makes them less sensitive. Inhibition of β-glucan synthase results in negative feedback, causing cell cycle arrest. Echinocandins and pneumocandins, aculeacins (A-D, F), mulundocandins and WF11899 (A, B, and C) as well as the killer toxin from Williopsis mrakii, WmKT are representative β-glucans synthase inhibitors (Guyard et al., 2002; Matejuk et al., 2010; van der Weerden et al., 2013).
Inhibition of Chitin Biosynthesis in the Cell Wall
Chitin, found in the fungal cell wall, is essential to maintain cell integrity and is absent in vertebrates. Aureobasidins are cyclic lipophilic 8-mer depsipeptides with an α-hydroxyacid that display two mechanisms of action: the disruption of cell wall/membranes by altering the assembly of actin and chitin (Endo et al., 1997) and the interruption of sphingolipids synthesis (Nagiec et al., 1997). Several members of the aureobasidin family exert anti-Candida activity. Nikkomycins are structural analogs of uridine diphosphate N-acetylglucosamine, a major constituent of chitin. These AFPs have been shown to inhibit the synthesis of chitin in C. albicans in both in vitro and in vivo studies (McCarthy et al., 1985) while human cells were not affected. They also show significant activity against C. immitis, B. dermatitidis, and moderate activity against H. capsulatum (Hector et al., 1990; Clemons and Stevens, 1997), but these agents are not active against filamentous fungi.
Selective Activity on Membranes
Rs-ARF2 is a 50 amino-acid residue plant defensin that has three-stranded β-sheets and an α-helix, structure that is stabilized by four disulfide bonds. Rs-ARF2 targets the fungus-specific membrane glucosylceramide inducing membrane permeability, which causes Ca2+ uptake, efflux of K+ and medium alkalinization. This defensin also induces the production of toxic reactive oxygen species intracellularly. Rs-AFP2 and analogs (with arginines replacing neutral amino acids) were found to inhibit A. flavus and Fusarium solani, C. albicans and C. krusei. C. glabrata, which does not contain this fungus-specific ceramide, is not inhibited. This peptide and its analogs (e.g., NaD1, Rs-ARF1, SPE10) have little cytotoxicity against mammalian cells at dosages that are inhibitory to fungal pathogens (Matejuk et al., 2010; Sher Khan et al., 2019).
Iturins, produced by Bacillus subtilis, are cyclic peptides with a lipophylic β-amino acid linked to a D and L amino acids, causing pore formation in membranes and leakage of key ions (Besson and Michel, 1984). Their antimicrobial activity is limited primarily to fungi, with little effect on bacteria. Unfortunately, iturins are toxic to mammalian cell membranes. In contrast to most antimicrobial peptides which are cationic, iturins can be anionic (bacillomycin L) or neutral (iturin A). One member of the family, bacillomycin F effectively inhibits A. niger, C. albicans, and C. tropicalis. Although this group of peptides is effective against dermatomycoses in humans and animals, they induce high levels of hemolysis (Latoud et al., 1986).
The mammalian peptide Histatin 5 contains seven histidines, four arginines, and three lysines which allow the peptide adopting an α-helical conformation in non-aquous environments (Raj et al., 1998). Histatin 5 binds to the Ssa2p, a 70-kDa cell wall protein required for the internalization of histatin 5 into cells (Li et al., 2006). The polyamine transporters Dur3 and Dur31 are necessary for the uptake of the peptide as well (Kumar et al., 2011), which needs to be translocated, not endocyted to exert an effect on C. albicans cells (Jang et al., 2010). If the cell is under respiratory metabolism, histatin 5 disrupts the integrity of mitochondrial membrane (Helmerhorst et al., 1999). Propidium iodide (PI) uptake and ATP release also occurs despite cell lysis does not appear to be induced by the peptide (Helmerhorst et al., 1999; Koshlukova et al., 1999). Subsequent ATP binding to surface P2X receptors induce signaling cascades leading to cell death.
Broad Spectrum Antimicrobial Peptides
Most AMPs affect a number of organisms including bacteria, fungi, and envelope-containing viruses. The most representative non-specific AMPs can be grouped as linear peptides (e.g., cecropins, magainins, cathelicidins, bombinins, lactoferrin-derived peptides) or cyclic peptides (e.g., mammalian defensins, poultry gallinacins, macrocyclic peptides, syringomycins, tachystatins) (Matejuk et al., 2010). Membrane disruption by the formation of toroidal pore is common to most peptides, resulting in leakage of essential molecules and ions with general loss of membrane functionality. Additional mechanisms include membrane thinning, formation of non-bilayer intermediates by interchelation of peptide-membrane, formation of anionic lipid-peptide domains, lipid flip-flop, demixing and clustering, and alteration of membrane potential (Shai, 2002; Bechinger and Lohner, 2006; Huang, 2006; Melo et al., 2009; Nguyen et al., 2011; Rautenbach et al., 2016). In addition, some of the peptides may have other effects such as DNA damage, apoptosis induction, inhibition of DNA replication and RNA or protein synthesis.
For supplementary reviews on the mechanisms of action and classification of antimicrobial peptides see reviews by Matejuk (Matejuk et al., 2010), van der Weerden (van der Weerden et al., 2013), and Rautenbach (Rautenbach et al., 2016).
Advantages of AFPs
Existing antifungal agents exhibit a diversity of drawbacks that reduce their efficiency as therapeutic tools against fungal infections. The existence of only a few approved classes of antifungal drugs and the increasing antifungal resistance further complicates the selection of an appropriate antifungal therapy (Sanguinetti et al., 2015; Pappas et al., 2018). Ergosterol synthesis, ergosterol in membranes and cell wall synthesis are the targets of azoles, polyenes and echinocandis, respectively. The low diversity of mechanisms of action of current treatments represents a problematic situation when fungal pathogens are multi-resistant to antifungals (Rautenbach et al., 2016). Furthermore, existing antifungal drugs are associated with adverse drug reactions such as hypokalemia, infusion reaction, nephrotoxicity, hepatotoxicity and gastrointestinal affections, among others (Chen et al., 2011; de Souza et al., 2016; Kyriakidis et al., 2017; Pappas et al., 2018). Some antifungal drugs affect common eukaryotic targets present both in pathogenic fungi and human cells (Rautenbach et al., 2016). This makes the development of novel efficient and non-toxic antifungal therapies more difficult than that of antibacterial ones.
AFPs have diverse advantages over the current antifungal drugs, which are directly related to their mechanisms of action and molecular targets. AFPs are particularly promising because they can recognize multiple microbial targets, thus reducing the possibility of resistance development (Rautenbach et al., 2016), a topic that is discussed in more detail in the following section. These microbial targets include fungal membranes, different cell wall components and molecules related to physiological processes, such as RNA, DNA and protein synthesis and cell cycle (van der Weerden et al., 2013; Bondaryk et al., 2017).
Regarding the absence of side effects, several AFPs target specific conserved fungal molecules, such as glucosylceramide, mannosyldiinositol phosphorylceramide, enzymes related to ergosterol or β-glucan synthesis, among others. This translates into high pathogen selectivity and reduces the probability of cytotoxicity against mammalian cells (Rautenbach et al., 2016). However, it does not ensure the absence of cytotoxicity (e.g., aculeacins, a type of echinocandin that targets the fungal 1,3-β-glucan synthase, displays hemolytic activity) (Matejuk et al., 2010). Over the last 13 years, three echinocandins, anidulafungin, caspofungin, and micafungin, have been approved in Europe and USA (Pound et al., 2011; Beyda et al., 2012). These peptides are poor substrates for cytochrome P450, which translates into both lower hepatotoxicity and pooled risk for discontinuation of treatment (3.7–4.8%) compared to other antifungals (Kyriakidis et al., 2017). The synthetic peptide killer peptide (KP) is another example that has demonstrated very promising antifungal activity without cytotoxicity against peripheral mononuclear blood cells in vitro or side effects in murine trials. This peptide seems to have a specific interaction with 1,3 β glucans and only the dimeric form of the peptide is active (Magliani et al., 2011).
In addition, AFPs show reduced cytotoxicity (Matejuk et al., 2010). Two reasons may explain this phenomenon. Firstly, there is a stronger interaction between the negatively charged fungal membrane (due to the higher content of phosphatidylinositol and phosphatidic acid) and the cationic charges of the peptides, in contrast to mammalian cell membranes, which are predominantly neutral to host mammals (due to the high content of phosphatidylcholine). Secondly, some AFPs target membrane lipids unique to fungi and absent from mammalian cells, which also reduces toxicity (Nguyen et al., 2011; Rautenbach et al., 2016).
Accumulating evidence demonstrates that the therapeutic activity of AMPs is multifactorial and not mediated only by their direct antimicrobial effect. Host defense peptides (HPDs), like defensins and cathelicidins families, often exert angiogeninc, immunomodulatory and anti-inflammatory effects and may also induce the recruitment of the adaptive immune response, which has been reported in several papers and reviews (Zasloff, 2002; Hirsch et al., 2008; Steinstraesser et al., 2009; Magliani et al., 2011; Hsieh and Hartshorn, 2016; Li et al., 2017).
Resistance to AFPs
Membrane remodeling of C. albicans has been associated with its resistance to current non-peptide antifungal drugs, mainly ergosterol-sphingolipid-rich lipid rafts containing multi-drug resistance (MDR) proteins attached to the membrane (Mukhopadhyay et al., 2004; Pasrija et al., 2005, 2008; Shahi and Moye-Rowley, 2009). In the case of resistance to AMPs and AFPs it is important to note, as discussed previously, that these peptides frequently function through membrane interaction, but that additional modes of action for microbial inhibition have also been demonstrated (Wu et al., 1999). Microorganisms, such as fungi, evolve rapidly, and can adapt quickly when exposed to antibiotics and antifungal drugs. However, it is important to note that cell membranes are slower to evolve. The rapid and potent effect on membrane, coupled with other inhibitory mechanisms exhibited by AFPs, de novo resistance is less likely to emerge in target microorganisms (Yeung et al., 2011).
As is the case for currently used antimicrobial drugs, the overuse of AFPs could accelerate the occurrence of fungal resistance. This issue complicates the application of antifungal therapeutics. Indeed, extensive echinocandin usage in hospitals has led to an increase in the number of strains with acquired (secondary) resistance to these first-line antifungals, especially among strains of C. glabrata (Sanguinetti et al., 2015; Pappas et al., 2018). The potential for the emergence of high level resistance to AMPs has been debated, but it is likely to occur at a reduced rate relative to that observed for other antifungals, though it will depend on how the antimicrobial peptide is administered. Besides, although a lower target concentration is required, the absence or change in the specific fungal target through spontaneous mutation can naturally lead to resistance. However, such modification of conserved molecules could, in turn, result in reduced pathogen virulence. In addition, combination therapy, involving the use of AMPs with antibiotics or with other peptides, will likely reduce the development of resistance markedly (Matejuk et al., 2010; Rautenbach et al., 2016).
Production of AFPs
Despite progress relating to the discovery and characterization of AMPs, their application remains challenging (Wimley and Hristova, 2011). There is a need for effective AMP production in sufficient amounts and purity to more extensively investigate their structure–function relationships, efficacy and safety, especially in clinical treatments. Efficient production is also required to serve market requirements should these investigations be successfully completed. There are three major strategies that one could employ to achieve this, i.e., direct isolation from natural producers, heterologous expression or chemical synthesis.
Natural Production
Currently, there are not many AMPs that are produced from their natural sources for clinical use. The purification of peptides from natural sources is laborious and expensive due to their low abundance and the multiplicity of compounds present in those sources (Vriens et al., 2014). Because of this, most industrial-scale productions of AMPs are performed by heterologous expression or chemical synthesis. Brief descriptions of two approaches for industrial-scale AMP production from natural sources are presented here: microbial fermentation and proteolysis of food proteins.
Echinocandins are exceptional examples of AMPs produced by microbial fermentation, and further chemical modification in case of the semisynthetic variants. Industrial-scale production of echinocandins is based on fermentation since they possess a high-complexity chemical structure. However, little information has been published about these processes. Echinocandin B, pneumocandin B0 and FR901379 are the natural echinocandins produced for commercial purposes from A. rugulosus, Glarea lozoyensis and Coleophoma empetri, respectively. The optimization of the fermentation process is essential to obtain a competitive product, since the fermentation and purification costs of natural echinocandins are the main variables that influence the overall production costs of semisynthetic derivatives. The clinical applications of natural echinocandins is limited by certain undesirable properties, such as a strong hemolytic activity, as discussed previously (Emri et al., 2013).
Industrial scale AMPs can also be obtained by proteolysis of food proteins and the release of encrypted peptides. Agyei and Danquah (2011) offered a brief description of the process for manufacturing pharmaceutical-grade peptides by this approach. The process involves, firstly, the acquisition of raw materials: food protein and proteolytic enzymes/or microorganisms. By-products from dairy, fish and meat industries are suitable cheap sources for proteins (Sibel Akalin, 2014; Ryder et al., 2016). The second step involves protein hydrolysis. The use of enzymatic hydrolysis is preferred over in situ microbial fermentation, particularly in food and pharmaceutical industries due to the lower or absent output of organic solvents and toxic chemical in the process and product (Sibel Akalin, 2014; Ryder et al., 2016). Under industrial-scale conditions, the use of immobilized enzymes offers several advantages over the conventional soluble enzymes, such as milder and controlled conditions and recycling of enzymes used (Sewczyk et al., 2018). The final step is fractionation and isolation of the bioactive peptides. Ultrafiltration, precipitation with solvents and liquid chromatography techniques (e.g., ion exchange, gel filtration) have been proposed for purification of peptides, however, their current implicit high costs make them prohibitive for large scale applications. It is estimated that up to 70% of the capital and operating costs in industrial biotechnology processes may correspond to the separation and purification stages (Brady et al., 2008). Electro-membrane filtration (EMF) is being established as an alternative method for the purification of bioactive peptides. It combines electrophoresis with conventional membrane filtration, being more cost-effective than chromatographic techniques (Bazinet and Firdaous, 2013). AMPs with antifungal activity, such as casocidin-I, kapacin A and lactoferrin-derived peptides can be produced by these methods as well.
Recombinant Production of AFPs
Considering the often low amounts of AMPs obtained by purification from natural sources and the high costs and difficulties that may arise from chemical synthesis (Li et al., 2010; Hou et al., 2017), recombinant production of AMPs provides a solid option to make these peptides accessible at low cost and high efficiency.
Genetically modified microorganisms facilitate the production and functional expression of any bioactive molecule but, importantly, also allow the production of bioengineered and encrypted peptides that would not be achievable otherwise (Wibowo and Zhao, 2019). Moreover, new sequencing technologies have made available a vast amount of genomic, transcriptomic and metabolic data, providing the means to further explore known and novel AMPs and the rational design of new antifungal peptides (Amaral et al., 2012; Porto et al., 2012; Tracanna et al., 2017).
However, the success of recombinant production can be highly variable. Understanding the composition and physicochemical properties of AMPs influences the selection and design of hosts and expression system. The choice of host, codon bias, protein expression vector, number of copies of the plasmid and fusion proteins can influence the correct synthesis, folding, and secretion of the recombinant peptide through the cell machinery (Deng et al., 2017). There are two other aspects to bear in mind: the toxicity of the AMP for the host and the high instability and susceptibility of peptides to degradation by proteases. To overcome this, AMPs can be synthesized in a form that is fused to another protein (fusion proteins) or in inactive forms (Kosobokova et al., 2016) initially.
Escherichia coli, yeasts (mainly Pichia pastoris) and plants are the most common recombinant expression platforms for biopharmaceutical proteins. Comprehensive reviews have previously reviewed the different heterologous production platforms available for AMPs (Sanchez-Garcia et al., 2016; Deng et al., 2017). Most AFPs produced by recombinant platforms target plant phytopathogens rather than human fungal pathogens. However, many of these AFPs may represent underutilized resources whose antifungal activity against human fungal pathogens is waiting to be discovered. In the next subsection, a brief overview of the production of AFPs in different hosts with clinical applications is provided.
Production of AFPs in Bacteria
E. coli is by far the bacterial species that is used most widely as a host for heterologous production of peptides and proteins (Li, 2011). Its genetic configuration is well-known, it is easy to manipulate and there is a broad variety of protein expression vectors and host strains available. The pET vectors (Novagen) are the most commonly used. Among the expression strains, the most popular are E. coli BL21 (DE3), deficient in proteases that may lead to protein degradation, or pLysS Origami and Rosetta and C41 (DE3) (Novagen), employed when disulfide bond formation is needed (Rosano and Ceccarelli, 2014). Many E. coli strains are unable to export proteins across their outer membrane, and proteins are secreted into the cytoplasm or periplasm generating inclusion bodies (Singh et al., 2015). Therefore, AFPs produced by E. coli are usually purified by sonication methods followed by reversed-phase chromatographies, giving relatively low yields. Several examples of AFPs production in E. coli are shown in Table 3.
Bacillus subtilis has also been explored as host for AFPs production. This includes plectasin (Zhang et al., 2015), cathelicidin (Luan et al., 2014), and the hybrid cecropin A– melittin (Ji et al., 2017) (Table 4). B. subtilis is a well-studied species, is non-pathogenic, has been approved by the Food and Drug Administration as a Generally Regarded As Safe (GRAS) microorganism and does not exhibit codon bias. It also reaches high cell density and releases proteins directly to the extracellular medium, simplifying the purification process. However, B. subtilis secretes proteolytic enzymes that can degrade the secreted recombinant proteins. Luckily, optimization of the cloning strategies and construction of protease-negative mutants are fostering its wider use (Cui et al., 2018).
Lactic acid bacteria (LAB) have been extensively used for the heterologous production of bacteriocins, antibacterial peptides secreted by bacteria (Rodríguez et al., 2003; García-Fruitós, 2012). Genera such as Lactobacillus, Leuconostoc, Pediococcus, Lactococcus, Streptococcus, and Enterococcus (König and Fröhlich, 2017) can be part of the human microbiota at different body sites (George et al., 2018) and, for some of these genera, specific strains have been used as probiotics (Harzallah and Belhadj, 2013). However, the production of AFPs in LAB is challenging due to the antimicrobial sensitivity of the host. Choi et al. (2005) attempted the heterologous production of HBD-1 in a nisin Z L. lactis producer, but toxicity to the host was apparent (Choi et al., 2005) (Table 4).
In addition to being outstanding secondary metabolite producers, including of antibacterial and antifungal peptides (Harir et al., 2018), in their own right, Streptomyces species also offer many potential advantages as hosts for the expression and secretion of proteins (Baltz, 2010). In a small number of cases they have also been used for the heterologous production of AFPs (Li et al., 2012; Roldán-Tapia et al., 2017) (Table 4).
Several fusion partners have been used to facilitate the production of AFPs in bacteria (Li, 2009; Costa et al., 2014). Examples of AFPs produced by E. coli, Bacillus, Streptomomyces and L. lactis using different fusion partners are shown in Table 4. The His-tag, widely used beyond E. coli and consists of a short chain of six histidine residues. The His-tag is often combined with other, fusion tags to improve the production, solubility and recovery of the recombinant protein. Thioredoxin (TRX) and glutathione S-transferase (GST) are ubiquitous enzymes involved in redox and detoxification processes, respectively, which are often used as N-terminal fusion tagsTRX helps with the formation of disulphide bridges of the target protein, especially in strains unable to do so. On the other hand, GST functions as a chaperone that enhances the expression and solubility of recombinant proteins. In addition, GST-tagged fusion proteins can be purified by glutathione affinity chromatography, which facilitates the purification process. MMIS is a modified form of the magainin intervening sequence (MIS) that prevents the antimicrobial activity of the fused peptides until they are released. ELK16 is a self-assembling peptide that induces the formation of cytoplasmic inclusion bodies in E. coli. Carbohydrate-binding modules have also been used to enhance purification of AMPs though a cellulose matrix. More innovative fusion partners include protoplast for iturin production (Shi et al., 2018). Overall, fusion partners increase the solubility of the target peptides and protect them from degradation, but there is no evidence of higher yields.
Production of AFPs by Yeasts
With respect to heterologous expression, yeasts are fast growing and easy to manipulate genetically. Moreover, they are capable to perform correct protein processing and post-translational modifications. The methylotrophic yeast Pichia pastoris has been the preferred yeast for AMP production and for recombinant expression of AFPs (Table 4). Pichia protein expression vectors contain the alcohol oxidase gene promoter (AOX 1), inducible by the addition of methanol, which allows the overexpression of the gene introduced downstream. Three strains have been most widely used to produce AFPs: P. pastoris X-33, P. pastoris GS115, and P. pastoris KM71H. The strains differ in their genotypes, which affects the selection of selectable markers, typically antibiotic resistance genes or auxotrophic markers. His-tags are commonly used here also to facilitate the purification of recombinant proteinsaffinity metal-chelating chromatography (Niu et al., 2015; Landim et al., 2017). Plant oleosin fusion technology (Ling, 2007; Bhatla et al., 2010) has also been used for the production of iturin A (Popa et al., 2019). Table 4 shows some examples of AFPs produced by P. pastoris for clinical use.
Production in Fungi
Filamentous fungi are a well-known source of metabolites and enzymes (Hoffmeister and Keller, 2007), e.g., they naturally produce a wide range of primary metabolites such as organic and fatty acids, and important secondary metabolites, including the antibiotics penicillin, cephalosporin and griseofulvin, or the cholesterol lowering agent lovastatin (Alberti et al., 2017). The attraction of filamentous fungi as hosts for protein recombinant production relates to their relatively inexpensive growing requirements and their ability to naturally secrete large amounts of proteins into the growth medium. They can also perform complex posttranslational modifications including glycosylation, proteolytic cleavage and multiple disulphide bond formation. Moreover, they are very useful when whole synthetic pathways need to be recreated. Species such as Aureobasidium pullulans, Penicillium chrysogenum, and P. digitatum have been used to produce AFPs. A. pullulans has been used to produce several bioactive molecules, such as pullulan, a polysaccharide with numerous applications in health and the food industry, β glucan, and a wide variety of extracellular enzymes. It has also been reported to produce the antibacterial exophilin A, liamocins and heavy oils (Chi et al., 2009). Notably, A. pullulans has also been used to produce aureobasidin A as a consequence of homologous recombination (Table 5) (Slightom et al., 2009). P. chrysogenum and P. digitatum have also been successfully used to produce NFAP2 and AfpB, respectively. P. chrysogenum is known to produce a cationic antifungal protein which inhibits zoopathogens and plant-pathogenic fungi. This host has undergone many improvements to optimize fermentation conditions. Finally, P. digitatum was originally known as a fruit pathogen but has been used for the homologous production of AfpB.
Production in Plants
Plant-based expression systems have been explored as production hosts for recombinant expression of AMPs due to their capacity for large scale production and their cost-effectiveness. Advantages of plants are their capability to perform appropriate glycosylation, folding, and disulphide bond formation of recombinant AMPs. There are different genetic approaches to produce AMPs in plants: using whole plants, tissue specific expression, tissue culture, or transient expression (Holaskova et al., 2015). Nuclear transformation has been the preferred technique for plant-derived therapeutic proteins followed by purification from transgenic plants. The tobacco plant (Nicotiana tabacum) is the most commonly used transgenic expression system and strains of the bacterial species Agrobacterium tumefaciens are the most popular intermediate hosts (Desai et al., 2010). Setting up a higher plant production platform is more expensive than using bacteria, yeast or fungi. However, once the system is established, it is easier to handle and provides high capacity for scale-up. Moreover, plant-based systems do not generally need control of production. Chahardoli et al. used tobacco whole plants as a platform to produce a lactoferrin and lactoferrampin 34 amino acid chimera. To our knowledge this is the first study to produce an AFP in plants (Table 5) (Chahardoli et al., 2018).
Chemical Synthesis
Chemical synthesis of peptides can be divided in two types: solid- (SPPS) or liquid (solution) phase peptide synthesis (LPPS). In general terms, LPPS is suitable for large-scale manufacture of short peptides or structures that are not easily prepared by SPPS. SPPS is generally used for lower scales or to provide mechanistic insights about peptides and offers the potential for the creation and production of more cost-effective antifungal therapies (Matejuk et al., 2010). Currently, Fmoc SPPS is the preferred method for peptide chemical synthesis due to the versatility and low cost of very high-quality building blocks. The diversity of synthetic peptides entering clinical trials has increased over the last 13 years, stimulating advances in Fmoc SPPS technologies in response to the growing demand for medicinal chemistry and pharmacology (Behrendt et al., 2016). Several groups have synthesized linear (Tran et al., 2008; Magliani et al., 2011; Konno et al., 2015; Cools et al., 2017; Park et al., 2018) and cyclic peptides (Mosca et al., 2000; Schaaper et al., 2001; Konno et al., 2015; Ng-Choi et al., 2019) using Fmoc SPPS.
The long process of isolation and characterization of new natural AMPs delays their clinical use. In this regard, Fmoc SPPS is at the forefront in the design of therapeutic peptides since it permits the easy alteration of features such as hydrophobicity, polarity, charge, structure, and it may also enhance activity and overcome the limitations of natural peptides (Freitas and Franco, 2016). The rational design of synthetic sequences is a new approach of relevance, and results from optimizing the sequence and chemical characteristics shared by different AMPs (pharmacophoric patron) (Freitas and Franco, 2016). Ideally, an antifungal peptide agent should be short, as mentioned previously. De novo peptide design may help reducing production costs, potential toxicity and lability, as well as increasing the in vivo activity (Steckbeck et al., 2014).
Unfortunately, Fmoc SPPS is currently far from meeting its potential and still cannot compete with the template-based process of expression for the large-scale demand of therapeutics (Behrendt et al., 2016). However, it should be noted that companies specialized in the large-scale manufacture of peptides are currently being established, claiming productions from multi-10 kg/lot (SPPS) to multi-100 kg/lot (LPPS)1. This suggests that the versatility of chemical synthesis is on its way to reach the cost-efficiency and scale of peptide expression.
Current and Potential Applications in Human Medicine
Combined Therapy With Other Drugs
A recurrence of disease and establishment of chronic fungal infections may result when antifungal treatments are not sufficiently effective. Thevissen (2016) proposed that more efforts should focus on combination therapy in addition to screening for novel antifungal compounds. Synergy between the combined compounds is the main objective of combination therapy, thereby increasing their activity and diminishing their toxicity on the host. Another option is combining an antimycotic (either currently used or novel AMP) with an enhancer molecule that, for example, weakens one or multiple pathogen tolerance mechanisms, such as biofilms, without direct antifungal activity. Either way, it is essential to demonstrate efficacy and safety of the combination before proceeding to clinical trials.
Limited data from clinical trials are available in this regard. A study performed by Candoni et al. showed for the first time that early mortality of patients with invasive aspergillosis was reduced by combined treatment with two antifungal agents (Candoni et al., 2014). In a retrospective study published in 2017, Lee et al. analyzed records from a pediatric department in South Korea and described how the combined therapy of voriconazole and caspofungin was an effective and safe treatment for children with leukemia (Lee et al., 2017). In vitro and in vivo studies suggests that AFPs are excellent candidates for this type of approaches and have a great potential for clinical success. Lactoferrin-derived peptides such as Lf(1-11) and bLfcin have shown synergy with azole antifungal drugs or amphotericin B, greatly reducing the minimal inhibitory concentrations (MICs) against C. albicans, C. glabrata, C. krusei, C. parapsilosis, C. tropicalis, and fluconazole-resistant strains of C. albicans (Wakabayashi et al., 1996, 1998; Lupetti et al., 2003; Fernandes and Carter, 2017). Some other examples of these studies are summarized in Table 6.
Commercial Products and Formulations
P113 is a 12-mer peptide developed by the company PacGen Life Science (Vancouver, Canada) as a mouth rinse formulation for the topical treatment of oral candidiasis. Clinical trials from PacGen demonstrated that oral candidiasis was effectively treated by P113, which compared favorably to the efficacy of nystatin, a standard treatments for oral candidiasis. Vaginal, dermatological and ophthalmic applications are on the list of P113 therapeutic potential (Duncan and O'Neil, 2013). Currently, the P113-containing line of products includes oral rinse solution and spray, feminine soothing spray and cleansing wash, and antibacterial hand cream, among others2. NP213/Novexatin was the lead product of NovaBiotics of Aberdeen, UK. It is an arginine-rich cyclic cationic peptide based on human α and β defensins (among others). It was used for treatment of toenails stubborn fungal infections such as onychomycosis (patents PCT/GB2006/004890 and PCT/GB2005/003245). Indeed, independent podiatrist analysis determined the treatment against mild to moderate onychomycosis as being 80% clinically effective. This effectiveness rate is significantly higher than that provided by existing topical treatments for onychomycosis in different territories, including United States and Europe (Duncan and O'Neil, 2013; Fox, 2013)3. However, the clinical study did not acomplish the main goal of a Phase IIb study by not showing differences over the placebo treatment under FDA current guidelines4.
Potential Applications
Despite their promising properties, only a few AFPs have reached the clinical phase. One example is hLF(1-11), a peptide that was developed for the systemic treatment of bloodstream and deep tissue infections produced by fungi and bacteria in severely immunocompromised transplant recipients. However, after favorable safety and tolerability clinical trials of hLF(1–11), no more studies have taken place putting on hold the commercialization of this peptide (van der Velden et al., 2009; Martin et al., 2015; Bruni et al., 2016). Another example is CZEN-002, a synthetic octapeptide derived from alpha-Melanocyte-Stimulating Hormone (a-MSH). CZEN-002 modulates immune and inflammatory responses, and has been shown to kill C. albicans as well (Fjell et al., 2011; Mahlapuu et al., 2016). This AMP had been in phase II clinical trials for the treatment of vulvovaginal candidiasis. However, no recent development has been reported. It was developed by Zengen, Abiogen Pharma and Lee's pharmaceuticals (Duncan and O'Neil, 2013)5.
In addition, there are many cases of peptides with promising properties currently being evaluated pre-clinically (Koo and Seo, 2019). Other pre-clinical examples are described in the review by Duncan and O'Neill (Duncan and O'Neil, 2013) and in the database DRAMP 2.0 (Kang et al., 2019).
Delivery and Formulations
As discussed, different formulations and delivery strategies for AFPs might be explored depending on the peptide properties, the potential toxicity (suitable for topical and/or systemic use) and the marketing strategies of the companies (e.g., mouth rinse, toothpaste, spray, dermal cream, etc.). Different carriers can enhance the pharmacodynamics and stability, and reduce toxicity of the active peptide, such as liposome encapsulation or the use of peptoids, the D-conformation-based peptide and β-peptides (Sajjan et al., 2001; Porter et al., 2002; da Silva Malheiros et al., 2010; Chongsiriwatana et al., 2011). Diverse pre-clinical delivery tools and formulations are being studied. In one case, Park et al. developed a pH-responsive and redox-sensitive polymer-based AmB-delivery carrier system (Park et al., 2017), by conjugation with histatin 5 acting both as a synergistic antifungal molecule and a targeting ligand against C. albicans. Other authors describe that some properties of the peptides could make them suitable for self-delivery systems. Examples include the synthetic killer peptide (KP) and the ultrashort peptide NapFFKK-OH, which, at certain pHs and concentration conditions, undergo a self-assembly process. The results are hydrogel-like aggregates that could slowly release the peptides in physiological conditions, as well as reducing the proteolytic susceptibility and increasing the storage stability of the active compound (Magliani et al., 2011; Albadr et al., 2018). Future clinical studies of these hydrogels and other delivery technologies will determine their safety and efficiency. Additional drug delivery strategies include carbon nanotubes and magnetic nanoparticles (López-Abarrategui et al., 2013; Chaudhari et al., 2016).
Conclusions
Mycoses are a serious and rising threat to humans. Survival rates remain unacceptably low and no new antifungals have been introduced in more than 13 years since echinochandins and pneumocandins. AFPs have obvious potential as more efficient and safer therapeutic agents than conventional antifungal drugs. Research on AFPs has been highly active and over one thousand peptides have been described. Nevertheless, few molecules have reached late clinical stage studies or have entered the market. Major challenges for AFPs commercialization relate to their specificity and safety. Moreover, stability of the formulations, delivery strategies and the overall therapeutic efficiency together with production costs at industrial scale and regulatory barriers remain to be resolved. The mode of action of AFPs is not fully understood which also raises safety concerns.
Regarding exploitation, AFP production yields from natural sources are very low and requires complex and costly procedures for extraction and purification. Their peptidic nature enables production through recombinant platforms, but scaling-up procedures are not always successful and require extensive optimization. Currently, chemical synthesis is economically viable only for short peptides and high value applications, but novel synthesis and purification technologies such as EMF are on the way to meet these requirements for such short peptides and high value applications.
Notably, indications from the FDA and European regulatory entities indicate that regulatory laws are changing, encouraging companies to invest in antimicrobial discovery and development. Among other measures, new guidance documents have been released, including plainspoken clinical criteria for evaluating antimicrobials and broadening the spectrum of volunteers for clinical trials. Other steps will help in trials designed for the evaluation of drug-resistant pathogens (Fox, 2013).
Considering that the global market for antifungals is set to be worth $12.2 billion6, AFPs are commercially attractive candidates in terms of manufacturing costs, options, increasing regulatory acceptance of peptide therapeutics, etc. (Duncan and O'Neil, 2013). Thus, an integral action plan on this field needs to be driven by academy, biotechnological and pharmaceutical companies and regulatory entities in order to enhance and thrust the development of novel antifungal therapies. Nonetheless, the path has been paved for these promising molecules.
Author Contributions
MF, SA, and EG-G designed and wrote the manuscript. PC critically revised the manuscript. All authors listed have made a substantial, direct and intellectual contribution to the work, and approved it for publication.
Funding
This publication has emanated from research conducted with the financial support of Science Foundation Ireland (SFI) under grant number SFI/12/RC/2273. MF and SA have received funding from the European Union's Horizon 2020 research and innovation programme under the Marie Skłodowska-Curie grant agreement number 754535. EG-G was supported by Walsh Fellowship Project 2015066.
Conflict of Interest
The authors declare that the research was conducted in the absence of any commercial or financial relationships that could be construed as a potential conflict of interest.
Footnotes
1. ^Synthesis—PolyPeptide. Available online at: https://www.polypeptide.com/commercial-manufacturing/ (accessed August 12, 2019).
2. ^P113 | Pacgen Life Science. Available online at: http://www.pacgenlife.com/node/171 (accessed August 15, 2019).
3. ^Elewski, B. E. Dermatologists and Podiatrists Interviewed, 14.
4. ^https://www.businesswire.com/news/home/20180928005622/en/Taro-Terminates-Agreement-NovaBiotics
5. ^CZEN-002 Available online at: https://www.pharmacodia.com/yaodu/html/v1/chemicals/f84aa65357bec670cbba3ae77711c233.html (accessed August 15, 2019).
References
Abe, H., Kawada, M., Sakashita, C., Watanabe, T., and Shibasaki, M. (2018). Structure-activity relationship study of leucinostatin A, a modulator of tumor–stroma interaction. Tetrahedron 74, 5129–5137. doi: 10.1016/j.tet.2018.05.064
Aerts, A. M., Bammens, L., Govaert, G., Carmona-Gutierrez, D., Madeo, F., Cammue, B. P. A., et al. (2011). The antifungal plant defensin HsAFP1 from Heuchera sanguinea induces apoptosis in Candida albicans. Front. Microbiol. 2:47. doi: 10.3389/fmicb.2011.00047
Agrawal, P., Bhalla, S., Chaudhary, K., Kumar, R., Sharma, M., and Raghava, G. P. S. (2018). In silico approach for prediction of antifungal peptides. Front. Microbiol. 9:323. doi: 10.3389/fmicb.2018.00323
Agyei, D., and Danquah, M. K. (2011). Industrial-scale manufacturing of pharmaceutical-grade bioactive peptides. Biotechnol. Adv. 29, 272–277. doi: 10.1016/j.biotechadv.2011.01.001
Akkam, Y. (2016). A review of antifungal peptides: basis to new era of antifungal drugs. Jordan J. Pharm. Sci. 9, 51–75.
Albadr, A. A., Coulter, S. M., Porter, S. L., Thakur, R. R. S., and Laverty, G. (2018). Ultrashort self-assembling peptide hydrogel for the treatment of fungal infections. Gels 4:48. doi: 10.3390/gels4020048
Alberti, F., Foster, G. D., and Bailey, A. M. (2017). Natural products from filamentous fungi and production by heterologous expression. Appl. Microbiol. Biotechnol. 101, 493–500. doi: 10.1007/s00253-016-8034-2
Amaral, A. C., Silva, O. N., Mundim, N. C. C. R., de Carvalho, M. J. A., Migliolo, L., Leite, J. R. S. A., et al. (2012). Predicting antimicrobial peptides from eukaryotic genomes: in silico strategies to develop antibiotics. Peptides 37, 301–308. doi: 10.1016/j.peptides.2012.07.021
Andreu, D., and Rivas, L. (1998). Animal antimicrobial peptides: an overview. J. Pept. Sci. 47, 415–433. doi: 10.1002/(SICI)1097-0282(1998)47:6<415::AID-BIP2>3.0.CO;2-D
Auchtung, T. A., Fofanova, T. Y., Stewart, C. J., Nash, A. K., Wong, M. C., Gesell, J. R., et al. (2018). Investigating colonization of the healthy adult gastrointestinal tract by fungi. mSphere 3:e00092–18. doi: 10.1128/mSphere.00092-18
Bahar, A. A., and Ren, D. (2013). Antimicrobial peptides. Pharmaceuticals 6, 1543–1575. doi: 10.3390/ph6121543
Balkovec, J. M., Black, R. M., Hammond, M. L., Heck, J. V., Zambias, R. A., Abruzzo, G., et al. (1992). Synthesis, stability, and biological evaluation of water-soluble prodrugs of a new echinocandin lipopeptide. Discovery of a potential clinical agent for the treatment of systemic candidiasis and Pneumocystis carinii pneumonia (PCP). J. Med. Chem. 35, 194–198. doi: 10.1021/jm00079a027
Baltz, R. H. (2010). Streptomyces and Saccharopolyspora hosts for heterologous expression of secondary metabolite gene clusters. J. Ind. Microbiol. Biotechnol. 37, 759–772. doi: 10.1007/s10295-010-0730-9
Bazinet, L., and Firdaous, L. (2013). Separation of bioactive peptides by membrane processes: technologies and devices. Recent. Pat. Biotechnol. 7, 9–27. doi: 10.2174/1872208311307010003
Bechinger, B., and Lohner, K. (2006). Detergent-like actions of linear amphipathic cationic antimicrobial peptides. Biochim. Biophys. Acta 1758, 1529–1539. doi: 10.1016/j.bbamem.2006.07.001
Bednarska, N. G., Wren, B. W., and Willcocks, S. J. (2017). The importance of the glycosylation of antimicrobial peptides: natural and synthetic approaches. Drug Discov. Today 22, 919–926. doi: 10.1016/j.drudis.2017.02.001
Behrendt, R., White, P., and Offer, J. (2016). Advances in Fmoc solid-phase peptide synthesis. J. Pept. Sci. 22, 4–27. doi: 10.1002/psc.2836
Belaid, A., and Hani, K. (2011). Antiviral and antifungal activity of some dermaseptin S4 analogues. Afr. J. Biotechnol. 10, 14962–14967. doi: 10.5897/AJB11.1108
Besson, F., and Michel, G. (1984). Action of the antibiotics of the iturin group on artificial membranes. J. Antibiot. 37, 646–651. doi: 10.7164/antibiotics.37.646
Bewley, C. A., and Faulkner, D. J. (1994). Theonegramide, an antifungal glycopeptide from the Philippine lithistid sponge Theonella swinhoei. J. Org. Chem. 59, 4849–4852. doi: 10.1021/jo00096a028
Beyda, N. D., Lewis, R. E., and Garey, K. W. (2012). Echinocandin resistance in Candida species: mechanisms of reduced susceptibility and therapeutic approaches. Ann. Pharmacother. 46, 1086–1096. doi: 10.1345/aph.1R020
Bhatla, S. C., Kaushik, V., and Yadav, M. K. (2010). Use of oil bodies and oleosins in recombinant protein production and other biotechnological applications. Biotechnol. Adv. 28, 293–300. doi: 10.1016/j.biotechadv.2010.01.001
Bink, A., Kucharíková, S., Neirinck, B., Vleugels, J., van Dijck, P., Cammue, B. P. A., et al. (2012). The nonsteroidal antiinflammatory drug diclofenac potentiates the in vivo activity of caspofungin against Candida albicans biofilms. J. Infect. Dis. 206, 1790–1797. doi: 10.1093/infdis/jis594
Blondelle, S. E., and Lohner, K. (2000). Combinatorial libraries: a tool to design antimicrobial and antifungal peptide analogues having lytic specificities for structure–activity relationship studies. J. Pept. Sci. 55, 74–87. doi: 10.1002/1097-0282(2000)55:1<74::AID-BIP70>3.0.CO;2-S
Bondaryk, M., Staniszewska, M., Zielinska, P., and Urbanczyk-Lipkowska, Z. (2017). Natural antimicrobial peptides as inspiration for design of a new generation antifungal compounds. J. Fungi 3:46. doi: 10.3390/jof3030046
Bongomin, F., Gago, S., Oladele, R. O., and Denning, D. W. (2017). Global and multi-national prevalence of fungal diseases-estimate precision. J. Fungi 3:E57. doi: 10.3390/jof3040057
Brady, R., Woonton, B., Gee, M. L., and O'Connor, A. J. (2008). Hierarchical mesoporous silica materials for separation of functional food ingredients — a review. Innov. Food Sci. Emerg. Technol. 9, 243–248. doi: 10.1016/j.ifset.2007.10.002
Bruni, N., Capucchio, M. T., Biasibetti, E., Pessione, E., Cirrincione, S., Giraudo, L., et al. (2016). Antimicrobial activity of lactoferrin-related peptides and applications in human and veterinary medicine. Molecules 21:752. doi: 10.3390/molecules21060752
Burrows, L. L., Stark, M., Chan, C., Glukhov, E., Sinnadurai, S., and Deber, C. M. (2006). Activity of novel non-amphipathic cationic antimicrobial peptides against Candida species. J. Antimicrob. Chemother. 57, 899–907. doi: 10.1093/jac/dkl056
Campbell-Platt, G., and Cook, P. E. (2008). Fungi in the production of foods and food ingredients. J. Appl. Microbiol. 67, 117s−131s. doi: 10.1111/j.1365-2672.1989.tb03776.x
Candoni, A., Caira, M., Cesaro, S., Busca, A., Giacchino, M., Fanci, R., et al. (2014). Multicentre surveillance study on feasibility, safety and efficacy of antifungal combination therapy for proven or probable invasive fungal diseases in haematological patients: the SEIFEM real-life combo study. Mycoses 57, 342–350. doi: 10.1111/myc.12161
Cavalcante, C. S. P., Falcão, C. B., Fontenelle, R. O., Andreu, D., and Rádis-Baptista, G. (2017). Anti-fungal activity of Ctn[15–34], the C-terminal peptide fragment of crotalicidin, a rattlesnake venom gland cathelicidin. J. Antibiot. Res. 70, 231–237. doi: 10.1038/ja.2016.135
Chahardoli, M., Fazeli, A., and Ghabooli, M. (2018). Recombinant production of bovine lactoferrin-derived antimicrobial peptide in tobacco hairy roots expression system. Plant Physiol. Biochem. 123, 414–421. doi: 10.1016/j.plaphy.2017.12.037
Chang, W., Li, Y., Zhang, L., Cheng, A., Liu, Y., and Lou, H. (2012). Retigeric acid B enhances the efficacy of azoles combating the virulence and biofilm formation of Candida albicans. Biol. Pharm. Bull. 35, 1794–1801. doi: 10.1248/bpb.b12-00511
Chaudhari, A. A., Ashmore, D., Nath, S., deb Kate, K., Dennis, V., Singh, S. R., et al. (2016). A novel covalent approach to bio-conjugate silver coated single walled carbon nanotubes with antimicrobial peptide. J. Nanobiotechnology 14:58. doi: 10.1186/s12951-016-0211-z
Chen, S. C.-A., Slavin, M. A., and Sorrell, T. C. (2011). Echinocandin antifungal drugs in fungal infections: a comparison. Drugs 71, 11–41. doi: 10.2165/11585270-000000000-00000
Chi, Z., Wang, F., Chi, Z., Yue, L., Liu, G., and Zhang, T. (2009). Bioproducts from aureobasidium pullulans, a biotechnologically important yeast. Appl. Microbiol. Biotechnol. 82, 793–804. doi: 10.1007/s00253-009-1882-2
Choi, H. J., Seo, M. J., Lee, J. C., Cheigh, C. I., Park, H., Ahn, C., et al. (2005). Heterologous expression of human β-defensin-1 in bacteriocin-producing Lactococcus lactis. J. Microbiol. Biotechnol. 15, 330–336.
Chongsiriwatana, N. P., Miller, T. M., Wetzler, M., Vakulenko, S., Karlsson, A. J., Palecek, S. P., et al. (2011). Short alkylated peptoid mimics of antimicrobial lipopeptides. Antimicrob. Agents Chemother. 55, 417–420. doi: 10.1128/AAC.01080-10
Clemons, K. V., and Stevens, D. A. (1997). Efficacy of nikkomycin Z against experimental pulmonary blastomycosis. Antimicrob. Agents Chemother. 41, 2026–2028. doi: 10.1128/AAC.41.9.2026
Cools, T. L., Struyfs, C., Drijfhout, J. W., Kucharíková, S., Lobo Romero, C., van Dijck, P., et al. (2017). A linear 19-Mer Plant Defensin-Derived peptide acts synergistically with Caspofungin against Candida albicans biofilms. Front. Microbiol. 8:2051. doi: 10.3389/fmicb.2017.02051
Costa, S., Almeida, A., Castro, A., and Domingues, L. (2014). Fusion tags for protein solubility, purification and immunogenicity in Escherichia coli: the novel Fh8 system. Front. Microbiol. 5:63. doi: 10.3389/fmicb.2014.00063
Cui, W., Han, L., Suo, F., Liu, Z., Zhou, L., and Zhou, Z. (2018). Exploitation of Bacillus subtilis as a robust workhorse for production of heterologous proteins and beyond. World J. Microbiol. Biotechnol. 34:145. doi: 10.1007/s11274-018-2531-7
da Silva Malheiros, P., Daroit, D. J., and Brandelli, A. (2010). Food applications of liposome-encapsulated antimicrobial peptides. Trends Food Sci. Technol. 21, 284–292. doi: 10.1016/j.tifs.2010.03.003
De Lucca, A. J. (2000). Antifungal peptides: potential candidates for the treatment of fungal infections. Expert Opin. Investig. Drugs 9, 273–299. doi: 10.1517/13543784.9.2.273
De Lucca, A. J., Bland, J. M., Jacks, T. J., Grimm, C., and Walsh, T. J. (1998). Fungicidal and binding properties of the natural peptides cecropin B and dermaseptin. Med. Mycol. 36, 291–298. doi: 10.1080/02681219880000461
De Lucca, A. J., and Walsh, T. J. (1999). Antifungal peptides: novel therapeutic compounds against emerging pathogens. Antimicrob. Agents Chemother. 43, 1–11. doi: 10.1128/AAC.43.1.1
de O Mello, E., dos Santos, I. S., de O Carvalho, A., de Souza, L. S., de Souza-Filho, G. A., do Nascimento, VV., et al. (2014). Functional expression and activity of the recombinant antifungal defensin PvD1r from Phaseolus vulgaris L. (common bean) seeds. BMC Biochem. 15:7. doi: 10.1186/1471-2091-15-7
de Souza, M. C. P., Santos, A. G. D., and Reis, A. M. M. (2016). Adverse drug reactions in patients receiving systemic antifungal therapy at a high-complexity hospital. J. Clin. Pharmacol. 56, 1507–1515. doi: 10.1002/jcph.772
Deng, T., Ge, H., He, H., Liu, Y., Zhai, C., Feng, L., et al. (2017). The heterologous expression strategies of antimicrobial peptides in microbial systems. Protein Expr. Purif. 140, 52–59. doi: 10.1016/j.pep.2017.08.003
Desai, P. N., Shrivastava, N., and Padh, H. (2010). Production of heterologous proteins in plants: Strategies for optimal expression. Biotechnol. Adv. 28, 427–435. doi: 10.1016/j.biotechadv.2010.01.005
Dimarcq, J.-L., Bulet, P., Hetru, C., and Hoffmann, J. (1998). Cysteine-rich antimicrobial peptides in invertebrates. J. Pept. Sci. 47, 465–477. doi: 10.1002/(SICI)1097-0282(1998)47:6<465::AID-BIP5>3.0.CO;2-#
Duncan, V. M. S., and O'Neil, D. A. (2013). Commercialization of antifungal peptides. Fungal Biol. Rev. 26, 156–165. doi: 10.1016/j.fbr.2012.11.001
Emri, T., Majoros, L., Tóth, V., and Pócsi, I. (2013). Echinocandins: production and applications. Appl. Microbiol. Biotechnol. 97, 3267–3284. doi: 10.1007/s00253-013-4761-9
Enaud, R., Vandenborght, L.-E., Coron, N., Bazin, T., Prevel, R., Schaeverbeke, T., et al. (2018). The mycobiome: a neglected component in the microbiota-gut-brain axis. Microorganisms 6:22. doi: 10.3390/microorganisms6010022
Endo, M., Takesako, K., Kato, I., and Yamaguchi, H. (1997). Fungicidal action of aureobasidin A, a cyclic depsipeptide antifungal antibiotic, against Saccharomyces cerevisiae. Antimicrob. Agents Chemother. 41, 672–676. doi: 10.1128/AAC.41.3.672
Eschenauer, G., Depestel, D. D., and Carver, P. L. (2007). Comparison of echinocandin antifungals. Ther. Clin. Risk Manag. 3, 71–97. doi: 10.2147/tcrm.2007.3.1.71
Esfand, R., and Tomalia, D. A. (2001). Poly(amidoamine) (PAMAM) dendrimers: from biomimicry to drug delivery and biomedical applications. Drug Discov. Today 6, 427–436. doi: 10.1016/S1359-6446(01)01757-3
Essig, A., Hofmann, D., Münch, D., Gayathri, S., Künzler, M., Kallio, P. T., et al. (2014). Copsin, a novel peptide-based fungal antibiotic interfering with the peptidoglycan synthesis. J. Biol. Chem. 289, 34953–34964. doi: 10.1074/jbc.M114.599878
Feng, X.-J., Xing, L.-W., Liu, D., Song, X.-Y., Liu, C.-L., Li, J., et al. (2014). Design and high-level expression of a hybrid antimicrobial peptide LF15-CA8 in Escherichia coli. J. Ind. Microbiol. Biotechnol. 41, 527–534. doi: 10.1007/s10295-013-1382-3
Fernandes, K. E., and Carter, D. A. (2017). The antifungal activity of lactoferrin and its derived peptides: mechanisms of action and synergy with drugs against fungal pathogens. Front. Microbiol. 8:2. doi: 10.3389/fmicb.2017.00002
Fjell, C. D., Hancock, R. E. W., and Cherkasov, A. (2007). AMPer: a database and an automated discovery tool for antimicrobial peptides. Bioinformatics 23, 1148–1155. doi: 10.1093/bioinformatics/btm068
Fjell, C. D., Hiss, J. A., Hancock, R. E. W., and Schneider, G. (2011). Designing antimicrobial peptides: form follows function. Nat. Rev. Drug Discov. 11, 37–51. doi: 10.1038/nrd3591
Fjell, C. D., Jenssen, H., Fries, P., Aich, P., Griebel, P., Hilpert, K., et al. (2008). Identification of novel host defense peptides and the absence of α-defensins in the bovine genome. Proteins 73, 420–430. doi: 10.1002/prot.22059
Fosgerau, K., and Hoffmann, T. (2015). Peptide therapeutics: current status and future directions. Drug Discov. Today 20, 122–128. doi: 10.1016/j.drudis.2014.10.003
Fox, J. L. (2013). Antimicrobial peptides stage a comeback. Nat. Biotechnol. 31, 379–382. doi: 10.1038/nbt.2572
Freitas, C. G., and Franco, O. L. (2016). “Antifungal peptides with potential against pathogenicf fungi” in Recent Trends in Antifungal Agents and Antifungal Therapy, eds A. Basak, R. Chakraborty, and S. M. Mandal (New Delhi: Springer India), 75–95. doi: 10.1007/978-81-322-2782-3_3
García-Fruitós, E. (2012). Lactic acid bacteria: a promising alternative for recombinant protein production. Microb. Cell Fact. 11:157. doi: 10.1186/1475-2859-11-157
Garrigues, S., Gandía, M., Borics, A., Marx, F., Manzanares, P., and Marcos, J. F. (2017). Mapping and identification of antifungal peptides in the putative antifungal protein AfpB from the filamentous fungus Penicillium digitatum. Front. Microbiol. 8:592. doi: 10.3389/fmicb.2017.00592
Garrigues, S., Gandía, M., Castillo, L., Coca, M., Marx, F., Marcos, J. F., et al. (2018). Three antifungal proteins from Penicillium expansum: different patterns of production and antifungal activity. Front. Microbiol. 9:2370. doi: 10.3389/fmicb.2018.02370
George, F., Daniel, C., Thomas, M., Singer, E., Guilbaud, A., Tessier, F. J., et al. (2018). Occurrence and dynamism of lactic acid bacteria in distinct ecological niches: a multifaceted functional health perspective. Front. Microbiol. 9:2899. doi: 10.3389/fmicb.2018.02899
Guo, J., Hu, H., Zhao, Q., Wang, T., Zou, Y., Yu, S., et al. (2012). Synthesis and antifungal activities of glycosylated derivatives of the cyclic peptide fungicide caspofungin. ChemMedChem 7, 1496–1503. doi: 10.1002/cmdc.201200214
Guyard, C., Dehecq, E., Tissier, J.-P., Polonelli, L., Dei-Cas, E., Cailliez, J.-C., et al. (2002). Involvement of [beta]-glucans in the wide-spectrum antimicrobial activity of Williopsis saturnus var. mrakii MUCL 41968 killer toxin. Mol. Med. 8, 686–694. doi: 10.1007/BF03402032
Hancock, R. E., and Chapple, D. S. (1999). Peptide antibiotics. Antimicrob. Agents Chemother. 43, 1317–1323. doi: 10.1128/AAC.43.6.1317
Harir, M., Bendif, H., Bellahcene, M., Fortas, Z., and Pogni, R. (2018). Streptomyces Secondary Metabolites, Basic Biology and Applications of Actinobacteria, Shymaa Enany. IntechOpen. Available online at: https://www.intechopen.com/books/basic-biology-and-applications-of-actinobacteria/streptomyces-secondary-metabolites
Harris, M. R., and Coote, P. J. (2010). Combination of caspofungin or anidulafungin with antimicrobial peptides results in potent synergistic killing of Candida albicans and Candida glabrata in vitro. Int. J. Antimicrob. Agents 35, 347–356. doi: 10.1016/j.ijantimicag.2009.11.021
Harzallah, D., and Belhadj, H. (2013). “Lactic acid bacteria as probiotics: characteristics, selection criteria and role in immunomodulation of human GI muccosal barrier,” in Lactic Acid Bacteria - R & D for Food, Health and Livestock Purposes. Marcelino Kongo (IntechOpen). Available online at: https://www.intechopen.com/books/lactic-acid-bacteria-r-d-for-food-health-and-livestock-purposes/lactic-acid-bacteria-as-probiotics-characteristics-selection-criteria-and-role-in-immunomodulation-o
Hector, R. F., Zimmer, B. L., and Pappagianis, D. (1990). Evaluation of nikkomycins X and Z in murine models of coccidioidomycosis, histoplasmosis, and blastomycosis. Antimicrob. Agents Chemother. 34, 587–593. doi: 10.1128/aac.34.4.587
Helmerhorst, E. J., Breeuwer, P., van't Hof, W., Walgreen-Weterings, E., Oomen, L. C., Veerman, E. C., et al. (1999). The cellular target of histatin 5 on Candida albicans is the energized mitochondrion. J. Biol. Chem. 274, 7286–7291. doi: 10.1074/jbc.274.11.7286
Hirsch, T., Metzig, M., Niederbichler, A., Steinau, H.-U., Eriksson, E., and Steinstraesser, L. (2008). Role of host defense peptides of the innate immune response in sepsis. Shock 30, 117–126. doi: 10.1097/shk.0b013e318160de11
Hoffmeister, D., and Keller, N. P. (2007). Natural products of filamentous fungi: enzymes, genes, and their regulation. Nat. Prod. Rep. 24, 393–416. doi: 10.1039/b603084j
Holaskova, E., Galuszka, P., Frebort, I., and Oz, M. T. (2015). Antimicrobial peptide production and plant-based expression systems for medical and agricultural biotechnology. Biotechnol. Adv. 33, 1005–1023. doi: 10.1016/j.biotechadv.2015.03.007
Hori, M., Kakiki, K., and Misato, T. (1974). Interaction between polyoxin and active center of chitin synthetase. Agric. Biol. Chem. 38, 699–705. doi: 10.1080/00021369.1974.10861226
Hou, W., Zhang, X., and Liu, C.-F. (2017). Progress in chemical synthesis of peptides and proteins. Trans. Tianjin Univ. 23, 401–419. doi: 10.1007/s12209-017-0068-8
Houwaart, S., Youssar, L., and Hüttel, W. (2014). Pneumocandin biosynthesis: involvement of a trans-selective proline hydroxylase. ChemBioChem 15, 2365–2369. doi: 10.1002/cbic.201402175
Hsieh, I.-N., and Hartshorn, K. L. (2016). The role of antimicrobial peptides in influenza virus infection and their potential as antiviral and immunomodulatory therapy. Pharmaceuticals 9:53. doi: 10.3390/ph9030053
Huang, H. W. (2006). Molecular mechanism of antimicrobial peptides: the origin of cooperativity. Biochim. Biophys. Acta 1758, 1292–1302. doi: 10.1016/j.bbamem.2006.02.001
Huang, L., Ching, C. B., Jiang, R., and Leong, S. S. J. (2008). Production of bioactive human beta-defensin 5 and 6 in Escherichia coli by soluble fusion expression. Protein Expr. Purif. 61, 168–174. doi: 10.1016/j.pep.2008.05.016
Huang, L., Leong, S. S. J., and Jiang, R. (2009). Soluble fusion expression and characterization of bioactive human beta-defensin 26 and 27. Appl. Microbiol. Biotechnol. 84, 301–308. doi: 10.1007/s00253-009-1982-z
Huffnagle, G. B., and Noverr, M. C. (2013). The emerging world of the fungal microbiome. Trends. Microbiol 21, 334–341. doi: 10.1016/j.tim.2013.04.002
Huynh, E., Akhtar, N., and Li, J. (2018). Efficient production of recombinant protegrin-1 from Pichia pastoris, and its antimicrobial and in vitro cell migration activity. Front. Microbiol. 9:12. doi: 10.3389/fmicb.2018.02300
Isono, K., Asahi, K., and Suzuki, S. (1969). Polyoxins, antifungal antibiotics. XIII. Structure of polyoxins. J. Am. Chem. Soc. 91, 7490–7505. doi: 10.1021/ja01054a045
Jang, W. S., Bajwa, J. S., Sun, J. N., and Edgerton, M. (2010). Salivary histatin 5 internalization by translocation, but not endocytosis, is required for fungicidal activity in Candida albicans. Mol. Microbiol. 77, 354–370. doi: 10.1111/j.1365-2958.2010.07210.x
Ji, S., Li, W., Baloch, A. R., Wang, M., Li, H., Cao, B., et al. (2017). Efficient biosynthesis of a Cecropin A-melittin mutant in Bacillus subtilis WB700. Sci. Rep. 7:40587. doi: 10.1038/srep40587
Kang, X., Dong, F., Shi, C., Liu, S., Sun, J., Chen, J., et al. (2019). DRAMP 2.0, an updated data repository of antimicrobial peptides. Sci. Data 6:148. doi: 10.1038/s41597-019-0154-y
Kapitan, M., Niemiec, M. J., Steimle, A., Frick, J. S., and Jacobsen, I. D. (2018). Fungi as part of the microbiota and interactions with intestinal bacteria. Curr. Top. Microbiol. Immunol. 422, 265–301. doi: 10.1007/82_2018_117
Karlowsky, J. A., Harding, G. A., Zelenitsky, S. A., Hoban, D. J., Kabani, A., Balko, T. V., et al. (1997). In vitro kill curves of a new semisynthetic echinocandin, LY-303366, against fluconazole-sensitive and -resistant Candida species. Antimicrob. Agents Chemother. 41, 2576–2578. doi: 10.1128/AAC.41.11.2576
Kaushik, N., Pujalte, G. G. A., and Reese, S. T. (2015). Superficial fungal infections. Prim. Care 42, 501–516. doi: 10.1016/j.pop.2015.08.004
Kawabata, S., Nagayama, R., Hirata, M., Shigenaga, T., Agarwala, K. L., Saito, T., et al. (1996). Tachycitin, a small granular component in horseshoe crab hemocytes, is an antimicrobial protein with chitin-binding activity. J. Biochem. 120, 1253–1260. doi: 10.1093/oxfordjournals.jbchem.a021549
Kim, H.-K., Chun, D.-S., Kim, J.-S., Yun, C.-H., Lee, J.-H., Hong, S.-K., et al. (2006). Expression of the cationic antimicrobial peptide lactoferricin fused with the anionic peptide in Escherichia coli. Appl. Microbiol. Biotechnol. 72, 330–338. doi: 10.1007/s00253-005-0266-5
König, H., and Fröhlich, J. (2017). “Lactic Acid Bacteria,” in Biology of Microorganisms on Grapes, in Must and in Wine, eds H. König, G. Unden, and J. Fröhlich (Cham: Springer International Publishing), 3–41. doi: 10.1007/978-3-319-60021-5_1
Konno, H., Abumi, K., Sasaki, Y., Yano, S., and Nosaka, K. (2015). Structure activity relationship study of burkholdine analogues toward simple antifungal agents. Bioorg. Med. Chem. Lett. 25, 3199–3202. doi: 10.1016/j.bmcl.2015.05.088
Koo, H. B., and Seo, J. (2019). Antimicrobial peptides under clinical investigation. J. Pept. Sci. 111:e24122. doi: 10.1002/pep2.24122
Koshlukova, S. E., Lloyd, T. L., Araujo, M. W., and Edgerton, M. (1999). Salivary histatin 5 induces non-lytic release of ATP from Candida albicans leading to cell death. J. Biol. Chem. 274, 18872–18879. doi: 10.1074/jbc.274.27.18872
Kosobokova, E. N., Skrypnik, K. A., and Kosorukov, V. S. (2016). Overview of fusion tags for recombinant proteins. Biochem. Mosc. 81, 187–200. doi: 10.1134/S0006297916030019
Kumar, R., Chadha, S., Saraswat, D., Bajwa, J. S., Li, R. A., Conti, H. R., et al. (2011). Histatin 5 uptake by Candida albicans utilizes polyamine transporters Dur3 and Dur31 proteins. J. Biol. Chem. 286, 43748–43758. doi: 10.1074/jbc.M111.311175
Kyriakidis, I., Tragiannidis, A., Munchen, S., and Groll, A. H. (2017). Clinical hepatotoxicity associated with antifungal agents. Expert Opin. Drug Saf. 16, 149–165. doi: 10.1080/14740338.2017.1270264
Lafleur, M. D., Sun, L., Lister, I., Keating, J., Nantel, A., Long, L., et al. (2013). Potentiation of azole antifungals by 2-adamantanamine. Antimicrob. Agents Chemother. 57, 3585–3592. doi: 10.1128/AAC.00294-13
Lakshminarayanan, R., Liu, S., Li, J., Nandhakumar, M., Aung, T. T., Goh, E., et al. (2014). Synthetic multivalent antifungal peptides effective against fungi. PLoS ONE 9:e87730. doi: 10.1371/journal.pone.0087730
Landim, P. G. C., Correia, T. O., Silva, F. D. A., Nepomuceno, D. R., Costa, H. P. S., Pereira, H. M., et al. (2017). Production in Pichia pastoris, antifungal activity and crystal structure of a class I chitinase from cowpea (Vigna unguiculata): Insights into sugar binding mode and hydrolytic action. Biochimie 135, 89–103. doi: 10.1016/j.biochi.2017.01.014
Landy, M., Warren, G. H., Rosenman, M. S. B., and Colio, L. G. (1948). Bacillomycin: an antibiotic from Bacillus subtilis active against pathogenic fungi. Proc. Soc. Exp. Biol. Med. 67, 539–541. doi: 10.3181/00379727-67-16367
Latoud, C., Peypoux, F., Michel, G., Genet, R., and Morgat, J. L. (1986). Interactions of antibiotics of the iturin group with human erythrocytes. Biochim. Biophys. Acta 856, 526–535. doi: 10.1016/0005-2736(86)90144-6
Lee, D. G., Kim, P. I., Park, Y., Woo, E.-R., Choi, J. S., Choi, C.-H., et al. (2002). Design of novel peptide analogs with potent fungicidal activity, based on PMAP-23 antimicrobial peptide isolated from porcine myeloid. Biochem. Biophys. Res. 293, 231–238. doi: 10.1016/S0006-291X(02)00222-X
Lee, K. H., Lim, Y. T., Hah, J. O., Kim, Y. K., Lee, C. H., and Lee, J. M. (2017). Voriconazole plus caspofungin for treatment of invasive fungal infection in children with acute leukemia. Blood Res. 52, 167–173. doi: 10.5045/br.2017.52.3.167
Li, C., Blencke, H.-M., Paulsen, V., Haug, T., and Stensvåg, K. (2010). Powerful workhorses for antimicrobial peptide expression and characterization. Bioeng. Bugs 1, 217–220. doi: 10.4161/bbug.1.3.11721
Li, J., Li, L., Feng, C., Chen, Y., and Tan, H. (2012). Novel polyoxins generated by heterologously expressing polyoxin biosynthetic gene cluster in the sanN inactivated mutant of Streptomyces ansochromogenes. Microb. Cell Fact. 11:135. doi: 10.1186/1475-2859-11-135
Li, X. S., Sun, J. N., Okamoto-Shibayama, K., and Edgerton, M. (2006). Candida albicans cell wall ssa proteins bind and facilitate import of salivary histatin 5 required for toxicity. J. Biol. Chem. 281, 22453–22463. doi: 10.1074/jbc.M604064200
Li, Y. (2009). Carrier proteins for fusion expression of antimicrobial peptides in Escherichia coli. Biotechnol. Appl. Biochem. 54, 1–9. doi: 10.1042/BA20090087
Li, Y. (2011). Recombinant production of antimicrobial peptides in Escherichia coli: a review. Protein Expr. Purif. 80, 260–267. doi: 10.1016/j.pep.2011.08.001
Li, Y., Ling, H., Li, W., and Tan, H. (2005). Improvement of nikkomycin production by enhanced copy of sanU and sanV in Streptomyces ansochromogenes and characterization of a novel glutamate mutase encoded by sanU and sanV. Metab. Eng. 7, 165–173. doi: 10.1016/j.ymben.2005.01.002
Li, Z., Mao, R., Teng, D., Hao, Y., Chen, H., Wang, X., et al. (2017). Antibacterial and immunomodulatory activities of insect defensins-DLP2 and DLP4 against multidrug-resistant Staphylococcus aureus. Sci. Rep. 7, 1–16. doi: 10.1038/s41598-017-10839-4
Ling, H. (2007). Oleosin fusion expression systems for the production of recombinant proteins. Biologia 62, 119–123. doi: 10.2478/s11756-007-0041-4
López-Abarrategui, C., Figueroa-Espí, V., Reyes-Acosta, O., Reguera, E., and Otero-Gonzalez, A. J. (2013). Magnetic nanoparticles: new players in antimicrobial peptide therapeutics. Curr. Protein Pept. Sci. 14, 595–606. doi: 10.2174/1389203711209070682
López-García, B., Harries, E., Carmona, L., Campos-Soriano, L., López, J. J., Manzanares, P., et al. (2015). Concatemerization increases the inhibitory activity of short, cell-penetrating, cationic and tryptophan-rich antifungal peptides. Appl. Microbiol. Biotechnol. 99, 8011–8021. doi: 10.1007/s00253-015-6541-1
Luan, C., Zhang, H. W., Song, D. G., Xie, Y. G., Feng, J., and Wang, Y. Z. (2014). Expressing antimicrobial peptide cathelicidin-BF in Bacillus subtilis using SUMO technology. Appl. Microbiol. Biotechnol. 98, 3651–3658. doi: 10.1007/s00253-013-5246-6
Lum, K. Y., Tay, S. T., Le, C. F., Lee, V. S., Sabri, N. H., Velayuthan, R. D., et al. (2015). Activity of novel synthetic peptides against Candida albicans. Sci. Rep. 5, 9657–9657. doi: 10.1038/srep09657
Lupetti, A., Paulusma-Annema, A., Welling, M. M., Dogterom-Ballering, H., Brouwer, C. P. J. M., Senesi, S., et al. (2003). Synergistic activity of the N-terminal peptide of human lactoferrin and fluconazole against Candida species. Antimicrob. Agents Chemother. 47, 262–267. doi: 10.1128/aac.47.1.262-267.2003
Magliani, W., Conti, S., Ciociola, T., Giovati, L., Zanello, P. P., Pertinhez, T., et al. (2011). Killer peptide: a novel paradigm of antimicrobial, antiviral and immunomodulatory auto-delivering drugs. Future Med. Chem. 3, 1209–1231. doi: 10.4155/fmc.11.71
Mahlapuu, M., Håkansson, J., Ringstad, L., and Björn, C. (2016). Antimicrobial peptides: an emerging category of therapeutic agents. Front. Cell. Infect. Microbiol. 6:194. doi: 10.3389/fcimb.2016.00194
Mania, D., Hilpert, K., Ruden, S., Fischer, R., and Takeshita, N. (2010). Screening for antifungal peptides and their modes of action in Aspergillus nidulans. Appl. Environ. Microbiol. 76, 7102–7108. doi: 10.1128/AEM.01560-10
Martin, L., van Meegern, A., Doemming, S., and Schuerholz, T. (2015). Antimicrobial peptides in human sepsis. Front. Immunol. 6:404. doi: 10.3389/fimmu.2015.00404
Matejuk, A., Leng, Q., Begum, M. D., Woodle, M. C., Scaria, P., Chou, S.-T., et al. (2010). Peptide-based antifungal therapies against emerging infections. Drugs Future 35:197. doi: 10.1358/dof.2010.035.03.1452077
Mattay, J., Houwaart, S., and Hüttel, W. (2018). Cryptic production of trans-3-hydroxyproline in echinocandin b biosynthesis. Appl. Environ. Microbiol. 84:e02370-17. doi: 10.1128/AEM.02370-17
McCarthy, P. J., Troke, P. F., and Gull, K. (1985). Mechanism of action of nikkomycin and the peptide transport system of Candida albicans. J. Gen. Microbiol. 131, 775–780. doi: 10.1099/00221287-131-4-775
McDonald, E. E., Goldberg, H. A., Tabbara, N., Mendes, F. M., and Siqueira, W. L. (2011). Histatin 1 resists proteolytic degradation when adsorbed to hydroxyapatite. J. Dent. Res. 90, 268–272. doi: 10.1177/0022034510388653
McNair, L. K. F., Siedler, S., Vinther, J. M. O., Hansen, A. M., Neves, A. R., Garrigues, C., et al. (2018). Identification and characterization of a new antifungal peptide in fermented milk product containing bioprotective Lactobacillus cultures. FEMS Yeast Res. 18:foy094. doi: 10.1093/femsyr/foy094
Melo, M. N., Ferre, R., and Castanho, M. A. R. B. (2009). Antimicrobial peptides: linking partition, activity and high membrane-bound concentrations. Nat. Rev. Microbiol. 7, 245–250. doi: 10.1038/nrmicro2095
Mikamo, H., Sato, Y., and Tamaya, T. (2000). In vitro antifungal activity of FK463, a new water-soluble echinocandin-like lipopeptide. J. Antimicrob. Chemother. 46, 485–487. doi: 10.1093/jac/46.3.485
Money, N. P. (2016). Fungi and biotechnology. J. Fungi. 2016, 401–424. doi: 10.1016/B978-0-12-382034-1.00012-8
Mor, A., Nguyen, V. H., Delfour, A., Migliore-Samour, D., and Nicolas, P. (1991). Isolation, amino acid sequence and synthesis of dermaseptin, a novel antimicrobial peptide of amphibian skin. Biochemistry 30, 8824–8830. doi: 10.1021/bi00100a014
Mosca, D. A., Hurst, M. A., So, W., Viajar, B. S., Fujii, C. A., and Falla, T. J. (2000). IB-367, a protegrin peptide with in vitro and in vivo activities against the microflora associated with oral mucositis. Antimicrob. Agents Chemother. 44, 1803–1808. doi: 10.1128/aac.44.7.1803-1808.2000
Mukherjee, D., Singh, S., Kumar, M., Kumar, V., Datta, S., and Dhanjal, D. S. (2018). “Fungal biotechnology: role and aspects,” in Fungi and their Role in Sustainable Development: Current Perspectives, eds P. Gehlot and J. Singh (Singapore: Springer Singapore), 91–103. doi: 10.1007/978-981-13-0393-7_6
Mukhopadhyay, K., Prasad, T., Saini, P., Pucadyil, T. J., Chattopadhyay, A., and Prasad, R. (2004). Membrane sphingolipid-ergosterol interactions are important determinants of multidrug resistance in Candida albicans. Antimicrob. Agents Chemother. 48, 1778–1787. doi: 10.1128/aac.48.5.1778-1787.2004
Nagiec, M. M., Nagiec, E. E., Baltisberger, J. A., Wells, G. B., Lester, R. L., and Dickson, R. C. (1997). Sphingolipid synthesis as a target for antifungal drugs. Complementation of the inositol phosphorylceramide synthase defect in a mutant strain of Saccharomyces cerevisiae by the AUR1 gene. J. Biol. Chem. 272, 9809–9817. doi: 10.1074/jbc.272.15.9809
Ng-Choi, I., Oliveras, À., Planas, M., and Feliu, L. (2019). Solid-phase synthesis of biaryl cyclic peptides containing a histidine-tyrosine linkage. Tetrahedron 75, 2625–2636. doi: 10.1016/j.tet.2019.03.014
Nguyen, L. T., Haney, E. F., and Vogel, H. J. (2011). The expanding scope of antimicrobial peptide structures and their modes of action. Trends. Biotechnol. 29, 464–472. doi: 10.1016/j.tibtech.2011.05.001
Nicola, A. M., Albuquerque, P., Paes, H. C., Fernandes, L., Costa, F. F., Kioshima, E. S., et al. (2019). Antifungal drugs: new insights in research & development. Pharmacol. Ther. 195, 21–38. doi: 10.1016/j.pharmthera.2018.10.008
Niu, M., Chai, S., You, X., Wang, W., Qin, C., Gong, Q., et al. (2015). Expression of porcine protegrin-1 in Pichia pastoris and its anticancer activity in vitro. Exp. Ther. Med. 9, 1075–1079. doi: 10.3892/etm.2015.2202
Ottaviani, M. F., Yordanova, S., Cangiotti, M., Vasileva-Tonkova, E., Coppola, C., Stoyanov, S., et al. (2016). Spectral characterization and in vitro microbiological activity of new bis-1,8-naphthalimides and their Cu(II) complexes. J. Mol. Struct. 1110, 72–82. doi: 10.1016/j.molstruc.2016.01.033
Pappas, P. G., Lionakis, M. S., Arendrup, M. C., Ostrosky-Zeichner, L., and Kullberg, B. J. (2018). Invasive candidiasis. Nat. Rev. Dis. Primers 4:18026. doi: 10.1038/nrdp.2018.26
Park, S.-C., Kim, J.-Y., Kim, E.-J., Cheong, G.-W., Lee, Y., Choi, W., et al. (2018). Hydrophilic linear peptide with histidine and lysine residues as a key factor affecting antifungal activity. Int. J. Mol. Sci. 19:3781. doi: 10.3390/ijms19123781
Park, S.-C., Kim, Y.-M., Lee, J.-K., Kim, N.-H., Kim, E.-J., Heo, H., et al. (2017). Targeting and synergistic action of an antifungal peptide in an antibiotic drug-delivery system. J. Control. Release 256, 46–55. doi: 10.1016/j.jconrel.2017.04.023
Pasrija, R., Panwar, S. L., and Prasad, R. (2008). Multidrug transporters CaCdr1p and CaMdr1p of Candida albicans display different lipid specificities: both ergosterol and sphingolipids are essential for targeting of CaCdr1p to membrane rafts. Antimicrob. Agents Chemother. 52, 694–704. doi: 10.1128/AAC.00861-07
Pasrija, R., Prasad, T., and Prasad, R. (2005). Membrane raft lipid constituents affect drug susceptibilities of Candida albicans. Biochem. Soc. Trans. 33, 1219–1223. doi: 10.1042/BST0331219
Pecorini, C., Savazzini, F., Martino, P. A., Fusi, E., Fogher, C., and Baldi, A. (2005). Heterologous expression of biologically active porcine lactoferrin in Pichia pastoris yeast. Vet. Res. Commun. 29 (Suppl. 2), 379–382. doi: 10.1007/s11259-005-0086-1
Pfaller, M., Gordee, R., Gerarden, T., Yu, M., and Wenzel, R. (1989). Fungicidal activity of cilofungin (LY121019) alone and in combination with anticapsin or other antifungal agents. Eur. J. Clin. Microbiol. Infect. Dis. 8, 564–567. doi: 10.1007/BF01967483
Popa, C., Shi, X., Ruiz, T., Ferrer, P., and Coca, M. (2019). Biotechnological production of the cell penetrating antifungal PAF102 Peptide in Pichia pastoris. Front. Microbiol. 10:1472. doi: 10.3389/fmicb.2019.01472
Porter, E. A., Weisblum, B., and Gellman, S. H. (2002). Mimicry of host-defense peptides by unnatural oligomers: antimicrobial β-peptides. J. Am. Chem. Soc. 124, 7324–7330. doi: 10.1021/ja0260871
Porto, W. F., Silva, O. N., and Franco, O. L. (2012). “Prediction and rational design of antimicrobial Peptides,” in Protein Structure Eshel Faraggi (IntechOpen). Available online at: https://www.intechopen.com/books/protein-structure/prediction-and-rational-design-of-antimicrobial-peptides
Pound, M. W., Townsend, M. L., Dimondi, V., Wilson, D., and Drew, R. H. (2011). Overview of treatment options for invasive fungal infections. Med. Mycol. 49, 561–580. doi: 10.3109/13693786.2011.560197
Raj, P. A., Marcus, E., and Sukumaran, D. K. (1998). Structure of human salivary histatin 5 in aqueous and nonaqueous solutions. Biopolymers 45, 51–67. doi: 10.1002/(SICI)1097-0282(199801)45:1<51::AID-BIP5>3.0.CO;2-Y
Ramos, R., Moreira, S., Rodrigues, A., Gama, M., and Domingues, L. (2013). Recombinant expression and purification of the antimicrobial peptide magainin-2. Biotechnol. Prog. 29, 17–22. doi: 10.1002/btpr.1650
Rautemaa-Richardson, R., and Richardson, M. D. (2017). Systemic fungal infections. Medicine 45, 757–762. doi: 10.1016/j.mpmed.2017.09.007
Rautenbach, M., Troskie, A. M., and Vosloo, J. A. (2016). Antifungal peptides: to be or not to be membrane active. Biochimie 130, 132–145. doi: 10.1016/j.biochi.2016.05.013
Robinson, J. A. (2011). Protein epitope mimetics as anti-infectives. Curr. Opin. Chem. Biol. 15, 379–386. doi: 10.1016/j.cbpa.2011.02.015
Rodríguez, J. M., Martínez, M. I., Horn, N., and Dodd, H. M. (2003). Heterologous production of bacteriocins by lactic acid bacteria. Int. J. Food Microbiol. 80, 101–116. doi: 10.1016/S0168-1605(02)00153-8
Roemer, T., and Krysan, D. J. (2014). Antifungal drug development: challenges, unmet clinical needs, and new approaches. Cold Spring Harb. Perspect. Med. 4:a019703. doi: 10.1101/cshperspect.a019703
Roldán-Tapia, M., Anné, J., Reyes, A. G., Carrasco, U., Millán-Pacheco, C., Barrios-González, J., et al. (2017). Streptomyces as overexpression system for heterologous production of an antimicrobial peptide. Protein Pept. Lett. 24, 483–488. doi: 10.2174/0929866524666170208154327
Rosano, G. L., and Ceccarelli, E. A. (2014). Recombinant protein expression in Escherichia coli: advances and challenges. Front. Microbiol. 5:172. doi: 10.3389/fmicb.2014.00172
Roscetto, E., Contursi, P., Vollaro, A., Fusco, S., Notomista, E., and Catania, M. R. (2018). Antifungal and anti-biofilm activity of the first cryptic antimicrobial peptide from an archaeal protein against Candida spp. clinical isolates. Sci. Rep. 8:17570. doi: 10.1038/s41598-018-35530-0
Rothstein, D. M., Spacciapoli, P., Tran, L. T., Xu, T., Roberts, F. D., Dalla Serra, M., et al. (2001). Anticandida activity is retained in p-113, a 12-amino-acid fragment of histatin 5. Antimicrob. Agents Chemother. 45, 1367–1373. doi: 10.1128/AAC.45.5.1367-1373.2001
Ryder, K., Bekhit, A. E.-D., McConnell, M., and Carne, A. (2016). Towards generation of bioactive peptides from meat industry waste proteins: generation of peptides using commercial microbial proteases. Food Chem. 208, 42–50. doi: 10.1016/j.foodchem.2016.03.121
Saito, T., Kawabata, S., Shigenaga, T., Takayenoki, Y., Cho, J., Nakajima, H., et al. (1995). A novel big defensin identified in horseshoe crab hemocytes: isolation, amino acid sequence, and antibacterial activity. J. Biochem. 117, 1131–1137. doi: 10.1093/oxfordjournals.jbchem.a124818
Sajjan, U. S., Tran, L. T., Sole, N., Rovaldi, C., Akiyama, A., Friden, P. M., et al. (2001). P-113D, an antimicrobial peptide active against Pseudomonas aeruginosa, retains activity in the presence of sputum from cystic fibrosis patients. Antimicrob. Agents Chemother. 45, 3437–3444. doi: 10.1128/AAC.45.12.3437-3444.2001
Sanchez-Garcia, L., Martín, L., Mangues, R., Ferrer-Miralles, N., Vázquez, E., and Villaverde, A. (2016). Recombinant pharmaceuticals from microbial cells: a 2015 update. Microb. Cell Fact. 15:33. doi: 10.1186/s12934-016-0437-3
Sanguinetti, M., Posteraro, B., and Lass-Flörl, C. (2015). Antifungal drug resistance among Candida species: mechanisms and clinical impact. Mycoses 58, 2–13. doi: 10.1111/myc.12330
Schaaper, W. M., Posthuma, G. A., Plasman, H. H., Sijtsma, L., Fant, F., Borremans, F. A., et al. (2001). Synthetic peptides derived from the beta2-beta3 loop of Raphanus sativus antifungal protein 2 that mimic the active site. J. Pept. Res. 57, 409–418. doi: 10.1034/j.1399-3011.2001.00842.x
Schibli, D. J., Epand, R. F., Vogel, H. J., and Epand, R. M. (2002). Tryptophan-rich antimicrobial peptides: comparative properties and membrane interactions. Biochem. Cell Biol. 80, 667–677. doi: 10.1139/o02-147
Schneider, G., and Fechner, U. (2005). Computer-based de novo design of drug-like molecules. Nat. Rev. Drug Discov. 4, 649–663. doi: 10.1038/nrd1799
Semlali, A., Leung, K. P., Curt, S., and Rouabhia, M. (2011). Antimicrobial decapeptide KSL-W attenuates Candida albicans virulence by modulating its effects on toll-like receptor, human β-defensin, and cytokine expression by engineered human oral mucosa. Peptides 32, 859–867. doi: 10.1016/j.peptides.2011.01.020
Sewczyk, T., Hoog Antink, M., Maas, M., Kroll, S., and Beutel, S. (2018). Flow rate dependent continuous hydrolysis of protein isolates. AMB Express 8:18. doi: 10.1186/s13568-018-0548-9
Shahi, P., and Moye-Rowley, W. S. (2009). Coordinate control of lipid composition and drug transport activities is required for normal multidrug resistance in fungi. Biochim. Biophys. Acta 1794, 852–859. doi: 10.1016/j.bbapap.2008.12.012
Shai, Y. (2002). Mode of action of membrane active antimicrobial peptides. Biopolymers 66, 236–248. doi: 10.1002/bip.10260
Shams, M. V., Nazarian-Firouzabadi, F., Ismaili, A., and Shirzadian-Khorramabad, R. (2019). Production of a recombinant dermaseptin peptide in nicotiana tabacum hairy roots with enhanced antimicrobial activity. Mol. Biotechnol. 61, 241–252. doi: 10.1007/s12033-019-00153-x
Sher Khan, R., Iqbal, A., Malak, R., Shehryar, K., Attia, S., Ahmed, T., et al. (2019). Plant defensins: types, mechanism of action and prospects of genetic engineering for enhanced disease resistance in plants. Biotechnology 9:192. doi: 10.1007/s13205-019-1725-5
Shi, J., Zhu, X., Lu, Y., Zhao, H., Lu, F., and Lu, Z. (2018). Improving iturin a production of bacillus amyloliquefaciens by genome shuffling and its inhibition against Saccharomyces cerevisiae in orange juice. Front. Microbiol. 9:2683. doi: 10.3389/fmicb.2018.02683
Shinnar, A. E., Butler, K. L., and Park, H. J. (2003). Cathelicidin family of antimicrobial peptides: proteolytic processing and protease resistance. Bioorg. Chem. 31, 425–436. doi: 10.1016/S0045-2068(03)00080-4
Sibel Akalin, A. (2014). Dairy-derived antimicrobial peptides: action mechanisms, pharmaceutical uses and production proposals. Trends Food Sci. Technol. 36, 79–95. doi: 10.1016/j.tifs.2014.01.002
Sinden, S. L., DeVay, J. E., and Backman, P. A. (1971). Properties of syringomycin, a wide spectrum antibiotic and phytotoxin produced by Pseudomonas syringae, and its role in the bacterial canker disease of peach trees. Physiol. Plant Pathol. 1, 199–213. doi: 10.1016/0048-4059(71)90029-4
Singh, A., Upadhyay, V., Upadhyay, A. K., Singh, S. M., and Panda, A. K. (2015). Protein recovery from inclusion bodies of Escherichia coli using mild solubilization process. Microb. Cell Fact. 14:41. doi: 10.1186/s12934-015-0222-8
Singh, K., Shekhar, S., Yadav, Y., Xess, I., and Dey, S. (2017). DS6: anticandidal, antibiofilm peptide against Candida tropicalis and exhibit synergy with commercial drug. J. Pept. Sci. 23, 228–235. doi: 10.1002/psc.2973
Slightom, J. L., Metzger, B. P., Luu, H. T., and Elhammer, A. P. (2009). Cloning and molecular characterization of the gene encoding the aureobasidin A biosynthesis complex in Aureobasidium pullulans BP-1938. Gene 431, 67–79. doi: 10.1016/j.gene.2008.11.011
Song, D., Chen, Y., Li, X., Zhu, M., and Gu, Q. (2014). Heterologous expression and purification of dermaseptin s4 fusion in Escherichia coli and recovery of biological activity. Prep. Biochem. Biotech. 44, 598–607. doi: 10.1080/10826068.2013.835735
Steckbeck, J. D., Deslouches, B., and Montelaro, R. C. (2014). Antimicrobial peptides: new drugs for bad bugs? Expert Opin. Biol. Ther. 14, 11–14. doi: 10.1517/14712598.2013.844227
Steinstraesser, L., Kraneburg, U. M., Hirsch, T., Kesting, M., Steinau, H.-U., Jacobsen, F., et al. (2009). Host defense peptides as effector molecules of the innate immune response: a sledgehammer for drug resistance? Int. J. Mol. Sci. 10, 3951–3970. doi: 10.3390/ijms10093951
Suetake, T., Aizawa, T., Koganesawa, N., Osaki, T., Kobashigawa, Y., Demura, M., et al. (2002). Production and characterization of recombinant tachycitin, the Cys-rich chitin-binding protein. Protein Eng. 15, 763–769. doi: 10.1093/protein/15.9.763
Suzuki, S., Isono, K., Nagatsu, J., Mizutani, T., Kawashima, Y., and Mizuno, T. (1965). A new antibiotic, polyoxin A. J. Antibiot. 18:131.
Tabbene, O., Azaiez, S., Grazia, A. D., Karkouch, I., Slimene, I. B., Elkahoui, S., et al. (2016). Bacillomycin D and its combination with amphotericin B: promising antifungal compounds with powerful antibiofilm activity and wound-healing potency. J. Appl. Microb.120, 289–300. doi: 10.1111/jam.13030
Tanida, T., Rao, F., Hamada, T., Ueta, E., and Osaki, T. (2001). Lactoferrin peptide increases the survival of Candida albicans- inoculated mice by upregulating neutrophil and macrophage functions, especially in combination with amphotericin B and granulocyte-macrophage colony-stimulating factor. Infect. Immun. 69, 3883–3890. doi: 10.1128/IAI.69.6.3883-3890.2001
Tavanti, A., Maisetta, G., Del Gaudio, G., Petruzzelli, R., Sanguinetti, M., Batoni, G., et al. (2011). Fungicidal activity of the human peptide hepcidin 20 alone or in combination with other antifungals against Candida glabrata isolates. Peptides 32, 2484–2487. doi: 10.1016/j.peptides.2011.10.012
Taveira, G. B., Mello, É. O., Carvalho, A. O., Regente, M., Pinedo, M., Canal, L., et al. (2017). Antimicrobial activity and mechanism of action of a thionin-like peptide from Capsicum annuum fruits and combinatorial treatment with fluconazole against Fusarium solani. Pept. Sci. 108:e23008. doi: 10.1002/bip.23008
Terras, F. R., Schoofs, H. M., de Bolle, M. F., van Leuven, F., Rees, S. B., Vanderleyden, J., et al. (1992). Analysis of two novel classes of plant antifungal proteins from radish (Raphanus sativus L.) seeds. J. Biol. Chem. 267, 15301–15309.
Thevissen, K. (2016). How promising are combinatorial drug strategies in combating Candida albicans biofilms? Future Med. Chem. 8, 1383–1385. doi: 10.4155/fmc-2016-0127
Tóth, L., Váradi, G., Borics, A., Batta, G., Kele, Z., Vendrinszky, Á., et al. (2018). Anti-candidal activity and functional mapping of recombinant and synthetic Neosartorya fischeri antifungal protein 2 (NFAP2). Front. Microbiol. 9:393. doi: 10.3389/fmicb.2018.00393
Tracanna, V., de Jong, A., Medema, M. H., and Kuipers, O. P. (2017). Mining prokaryotes for antimicrobial compounds: from diversity to function. FEMS Microbiol. Rev. 41, 417–429. doi: 10.1093/femsre/fux014
Tran, D., Tran, P., Roberts, K., Osapay, G., Schaal, J., Ouellette, A., et al. (2008). Microbicidal properties and cytocidal selectivity of rhesus macaque theta defensins. Antimicrob. Agents Chemother. 52, 944–953. doi: 10.1128/AAC.01090-07
Uppuluri, P., Nett, J., Heitman, J., and Andes, D. (2008). Synergistic effect of calcineurin inhibitors and fluconazole against Candida albicans biofilms. Antimicrob. Agents Chemother. 52, 1127–1132. doi: 10.1128/AAC.01397-07
van der Velden, W. J., van Iersel, T. M., Blijlevens, N. M., and Donnelly, J. P. (2009). Safety and tolerability of the antimicrobial peptide human lactoferrin 1-11 (hLF1-11). BMC Med. 7:44. doi: 10.1186/1741-7015-7-44
van der Weerden, N. L., Bleackley, M. R., and Anderson, M. A. (2013). Properties and mechanisms of action of naturally occurring antifungal peptides. Cell. Mol. Life Sci. 70, 3545–3570. doi: 10.1007/s00018-013-1260-1
Vouldoukis, I., Shai, Y., Nicolas, P., and Mor, A. (1996). Broad spectrum antibiotic activity of skin-PYY. FEBS Lett. 380, 237–240. doi: 10.1016/0014-5793(96)00050-6
Vriens, K., Cammue, B. P. A., and Thevissen, K. (2014). Antifungal plant defensins: mechanisms of action and production. Molecules 19, 12280–12303. doi: 10.3390/molecules190812280
Vriens, K., Cools, T. L., Harvey, P. J., Craik, D. J., Braem, A., Vleugels, J., et al. (2016). The radish defensins RsAFP1 and RsAFP2 act synergistically with caspofungin against Candida albicans biofilms. Peptides 75, 71–79. doi: 10.1016/j.peptides.2015.11.001
Vriens, K., Cools, T. L., Harvey, P. J., Craik, D. J., Spincemaille, P., Cassiman, D., et al. (2015). Synergistic activity of the plant defensin hsafp1 and caspofungin against Candida albicans biofilms and planktonic cultures. PLoS ONE 10:e0132701. doi: 10.1371/journal.pone.0132701
Wakabayashi, H., Abe, S., Okutomi, T., Tansho, S., Kawase, K., and Yamaguchi, H. (1996). Cooperative anti-Candida effects of lactoferrin or its peptides in combination with azole antifungal agents. Microbiol. Immunol. 40, 821–825. doi: 10.1111/j.1348-0421.1996.tb01147.x
Wakabayashi, H., Abe, S., Teraguchi, S., Hayasawa, H., and Yamaguchi, H. (1998). Inhibition of hyphal growth of azole-resistant strains of Candida albicans by triazole antifungal agents in the presence of lactoferrin-related compounds. Antimicrob. Agents Chemother. 42, 1587–1591. doi: 10.1128/AAC.42.7.1587
Wang, G., Li, X., and Wang, Z. (2016). APD3: the antimicrobial peptide database as a tool for research and education. Nucleic Acids Res. 44, D1087–D1093. doi: 10.1093/nar/gkv1278
Wang, M., Zheng, K., Lin, J., Huang, M., Ma, Y., Li, S., et al. (2018). Rapid and efficient production of cecropin A antibacterial peptide in Escherichia coli by fusion with a self-aggregating protein. BMC Biotechnol. 18:62. doi: 10.1186/s12896-018-0473-7
Wei, G.-X., and Bobek, L. A. (2004). In vitro synergic antifungal effect of MUC7 12-mer with histatin-5 12-mer or miconazole. J. Antimicrob. Chemother. 53, 750–758. doi: 10.1093/jac/dkh181
Wibowo, D., and Zhao, C.-X. (2019). Recent achievements and perspectives for large-scale recombinant production of antimicrobial peptides. Appl. Microbiol. Biotechnol. 103, 659–671. doi: 10.1007/s00253-018-9524-1
Wimley, W. C., and Hristova, K. (2011). Antimicrobial Peptides: successes, challenges and unanswered questions. J. Membr. Biol. 239, 27–34. doi: 10.1007/s00232-011-9343-0
Wu, M., Maier, E., Benz, R., and Hancock, R. E. (1999). Mechanism of interaction of different classes of cationic antimicrobial peptides with planar bilayers and with the cytoplasmic membrane of Escherichia coli. Biochemistry 38, 7235–7242. doi: 10.1021/bi9826299
Yeaman, M. R., and Yount, N. Y. (2003). Mechanisms of antimicrobial peptide action and resistance. Pharmacol. Rev. 55, 27–55. doi: 10.1124/pr.55.1.2
Yeung, A. T. Y., Gellatly, S. L., and Hancock, R. E. W. (2011). Multifunctional cationic host defence peptides and their clinical applications. Cell. Mol. Life Sci. 68, 2161–2176. doi: 10.1007/s00018-011-0710-x
Zasloff, M. (2002). Antimicrobial peptides of multicellular organisms. Nature 415, 389–395. doi: 10.1038/415389a
Zhang, L., and Gallo, R. L. (2016). Antimicrobial peptides. Curr. Biol. 26, R14–R19. doi: 10.1016/j.cub.2015.11.017
Zhang, L., Li, X., Wei, D., Wang, J., Shan, A., and Li, Z. (2015). Expression of plectasin in Bacillus subtilis using SUMO technology by a maltose-inducible vector. J. Ind. Microbiol. Biotechnol. 42, 1369–1376. doi: 10.1007/s10295-015-1673-y
Keywords: antifungal peptides, antimicrobial peptides, mycoses, antimicrobial resistance, production, new therapies
Citation: Fernández de Ullivarri M, Arbulu S, Garcia-Gutierrez E and Cotter PD (2020) Antifungal Peptides as Therapeutic Agents. Front. Cell. Infect. Microbiol. 10:105. doi: 10.3389/fcimb.2020.00105
Received: 09 December 2019; Accepted: 27 February 2020;
Published: 17 March 2020.
Edited by:
Philippe Bulet, INSERM U1209 Institut pour l'Avancée des Biosciences (IAB), FranceReviewed by:
Stéphane Cociancich, Centre de Coopération Internationale en Recherche Agronomique pour le Développement (CIRAD), FranceTonyia Eaves-Pyles, University of Texas Medical Branch at Galveston, United States
Copyright © 2020 Fernández de Ullivarri, Arbulu, Garcia-Gutierrez and Cotter. This is an open-access article distributed under the terms of the Creative Commons Attribution License (CC BY). The use, distribution or reproduction in other forums is permitted, provided the original author(s) and the copyright owner(s) are credited and that the original publication in this journal is cited, in accordance with accepted academic practice. No use, distribution or reproduction is permitted which does not comply with these terms.
*Correspondence: Paul D. Cotter, cGF1bC5jb3R0ZXImI3gwMDA0MDt0ZWFnYXNjLmll