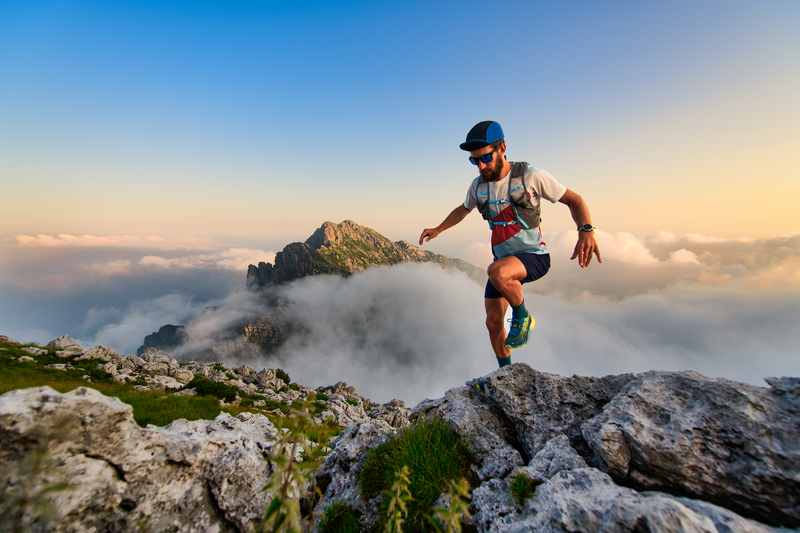
94% of researchers rate our articles as excellent or good
Learn more about the work of our research integrity team to safeguard the quality of each article we publish.
Find out more
ORIGINAL RESEARCH article
Front. Cell. Infect. Microbiol. , 10 January 2020
Sec. Bacteria and Host
Volume 9 - 2019 | https://doi.org/10.3389/fcimb.2019.00457
Uropathogenic Escherichia coli (UPEC), a Gram-negative bacterial pathogen, is a major causative agent of urinary tract infections (UTIs). However, the molecular mechanisms of how UPEC causes infections have not been determined. Recent studies indicated that certain enteric Gram-negative bacteria interact with and hijack innate immune receptors DC-SIGN (CD209a) and SIGNR1 (CD209b), often expressed by antigen-presenting cells (APCs), such as macrophages, leading to dissemination and infection. It was not known whether UPEC could utilize DC-SIGN receptors to promote its infection and dissemination similarly to the enteric pathogens. The results of this study reveal that UPEC interacts with CD209-expressing macrophages and transfectants. This interaction is inhibited by anti-CD209 antibody, indicating that CD209s are receptors for UPEC. Additionally, in contrast to the results of previous studies, mice lacking SIGNR1 are more susceptible to infection of this uropathogen, leading to prolonged bacterial persistence. Overall, the results of our study indicate that the innate immune receptor CD209s participate in the clearance of UPEC during UTIs.
The major causative agent of urinary tract infections (UTIs) is Gram-negative uropathogenic Escherichia coli (UPEC), which accounts for ~80% of community-acquired infections and 25% of nosocomial infections (Ronald, 2002). UTIs are one of the most common bacterial infections, with an annual incidence of 150 million cases worldwide (Stamm and Norrby, 2001). More than half of all women and some men will have at least one UTI in their lifetime (Foxman, 2002). Recent increases in the prevalence of multidrug-resistant uropathogenic strains, together with the lack of an effective vaccine against UPEC infections, have become worldwide concerns and present a substantial challenge to public health (O'Brien et al., 2016; Sanchez et al., 2016). A hallmark of such infections is high incidence of recurrence, which occurs in 20–30% patients (Foxman, 2002).
Bacterial ascension into the urinary tract may lead to infection. After reaching the bladder mucosa, UPEC can be internalized by superficial bladder epithelial cells (facet or umbrella cells) and can form intracellular bacterial communities (IBCs) or quiescent intracellular reservoirs (QIRs) that are resistant to phagocytosis by polymorphonuclear leukocytes (PMNs) and macrophages, and are resistant to antibiotic treatment (Schilling et al., 2002; Kerrn et al., 2005; Justice et al., 2006; Mysorekar and Hultgren, 2006; Horvath et al., 2011). Upon lysis of facet cells, the released UPEC can bind and invade neighboring bladder cells to start the cycle anew (Anderson et al., 2003; Justice et al., 2004), suggesting that the UPEC utilization of facet cells provides a shelter, allowing persistence in the bladder. Antigen presenting cells (APCs), such as dendritic cells and macrophages, play essential roles in the host immune response to UTIs (Mora-Bau et al., 2015). In addition to antigen presentation, macrophages are also important sources of pro-inflammatory cytokines that modulate inflammatory responses during UTIs (Abraham and Miao, 2015; Schaale et al., 2016; Waldhuber et al., 2016). Macrophages and neutrophils work together as inducers and effectors upon bacterial infections, and the cooperation between tissue resident macrophages and inflammation-recruited macrophages is involved in neutrophil migration (Schiwon et al., 2014). CD14 is involved in immune cell migration and differential cytokine production in macrophages, and studies by Carey et al. demonstrated a protective role of CD14 expressed by monocytes/macrophages in UTIs (Carey et al., 2016). Current studies have shown that UPEC can subvert the host immune response by suppression of early urothelial cytokine production and inhibition of neutrophil recruitment and function (Klumpp et al., 2001; Billips et al., 2007; Loughman and Hunstad, 2011). Interestingly, it is reported that UPEC can subvert macrophage anti-microbial pathways and survive inside macrophages up to 24 h (Bokil et al., 2011; Stocks et al., 2019). However, the molecular mechanisms of the development of persistent UPEC infections are not fully understood.
Recent studies indicate that several Gram-negative bacteria, including Salmonella Typhimurium (S. Typhimurium), Yersinia pseudotuberculosis (Y. pseudotuberculosis) and Yersinia pestis (Y. pestis), are able to interact with DC-SIGN (CD209s), a C-type lectin and an innate immune receptor expressed by APCs, to hijack the APCs for host dissemination and infection (He et al., 2019; Yang et al., 2019; Ye et al., 2019). These studies were informed by the mechanism of HIV infection of APCs. HIV binds the human DC-SIGN receptor on APCs and hijacks infected DCs as Trojan horses to promote viral dissemination to target cells such as CD4 lymphocytes in lymph nodes (Geijtenbeek et al., 2000; Engering et al., 2002; McDonald et al., 2003). In addition, Helaine et al. found that the phagocytosed S. Typhimurium was able to form persisters inside macrophages, which provide a reservoir for relapsing infection (Helaine et al., 2014; Rycroft et al., 2018). Consequently, macrophages that are supposed to be warriors of innate immunity are subverted by pathogens to promote their persistence in hosts. Understanding the details of these macrophage interactions may provide a better understanding of the pathogenesis of intracellular pathogens.
The lipopolysaccharides (LPS) of many Gram-negative bacterial pathogens promote resistance to serum killing and/or phagocytosis (Burns and Hull, 1998; Cortes et al., 2002; Murray et al., 2003). Over 70% of the cell surface of Gram-negative bacteria is occupied by LPS, which consists of lipid A, the oligosaccharide core (LPS core), and O-antigen. Our previous studies show that N-acetylglucosamine (GlcNAc) sugar residues within the LPS core of several Gram-negative bacteria are exposed by loss of O-antigen, which helps in the targeting of CD209s by these pathogens (Zhang et al., 2006). The host dissemination and infection of some pathogens can be significantly reduced by inhibition of such receptor-ligand interactions with an oligosaccharide or its analogs (He et al., 2019; Yang et al., 2019; Ye et al., 2019), indicating that inhibition of LPS core-CD209s interaction may serve as a treatment or prevention strategy of infections caused by these pathogens.
In this study, we examined whether the Gram-negative UPEC can interact with CD209s to promote host infection and persistence during urinary tract infections.
The bacterial strains and cell lines used in this study are listed in Table 1. Human uropathogenic Escherichia coli (UPEC) cystitis isolate UTI89 and pyelonephritis strain CFT073 were obtained from Harbin Institute of Veterinary Medicine, Chinese Academy of Agricultural Sciences, Harbin, China. UTI89-GFP is UTI89 transformed with the green fluorescent protein-expressing plasmid pAcGFP1 (Clontech, CS, USA). UTI89-O+ and CFT073-O+ were constructed by transformation with the pAY100.1 plasmid, which carries the genes necessary for Yersinia enterocolitica O-antigen expression (Oyston et al., 2003). UPEC1-UPEC4 strains were clinically isolated from urine samples from the Department of Clinical Laboratory of Tongji Hospital. E. coli K-12 strain CS180 produces a rough LPS lacking O-antigen (Schnaitman and Klena, 1993). CS1861 is a derivative of CS180 harboring pSS37, a plasmid containing all of the genes for O-antigen expression from Shigella dysenteriae 1 (Klena et al., 1992; Klena and Schnaitman, 1993). Y. pseudotuberculosis Y1 is a serotype O:1a strain lacking the virulence plasmid (pYV). This strain was obtained from the Centers for Disease Control (GA, USA) and was used previously as a positive invasion control (Chen et al., 1995), since this bacterium invades almost all epithelial cell lines via an invasin-integrin interaction (Isberg and Leong, 1990).
CHO-mSIGNR1 and CHO-hDC-SIGN were generated by transfecting CHO cells (Type Culture Collection, Chinese Academy of Sciences, Shanghai, China) with mouse SIGNR1 cDNA and human DC-SIGN cDNA, respectively, followed by selection for stable surface expression as originally described (Takahara et al., 2004). CHO-NEO is used as a control cell line that expresses only the neomycin resistance gene.
C57BL/6 wild-type (WT) mice were purchased from Wuhan University Animal Center. SIGNR1 knockout (SIGNR1−/−) mice on a C57BL/6 background were kindly provided by the Consortium for Functional Glycomics (http://www.functionalglycomics.org) and bred in the animal facility of Tongji Hospital. The WT and SIGNR1−/− mice used were matched in age and weight. After obtaining from the vendor, the WT mice were housed in pathogen-free conditions in the animal facility for more than 2 weeks before the experiment. All mice were treated in direct accordance with guidelines drafted by the Animal Care Committees of Tongji Hospital.
After mice were euthanized, their abdomens were immediately exposed, cleaned with 70% ethanol and opened with scissors. Next, 5 ml of RPMI media was injected into the intraperitoneal cavity. Abdomens were gently massaged for 2 min and the lavage fluid was then collected. The suspension containing macrophages was seeded in flasks and placed in a CO2 incubator for 2 h. The cell layers were washed three times to remove non-adherent cells. Macrophages were then removed from the plastic surface by incubating with citrate saline and re-seeded for interaction assays.
The invasion assays were carried out as described previously (Chen et al., 1997, 2001). Briefly, host cells were plated in 24-well plates. Cells were suspended in RPMI media with 2% fetal calf serum (FCS) at a concentration of 4×105 cells/ml, and 0.5 ml aliquots of cell suspensions were added to 24-well plates. After addition of 50 μl of bacterial suspension at a concentration of 2×107 colony forming units (CFUs) /ml (2×106 CFUs/ml for Y. pseudotuberculosis Y1 due to its high level of invasiveness), the cells were incubated for 2.5 h (2 h for macrophages) at 37°C in the presence of 5% CO2. The cell monolayers were then washed three times with phosphate-buffered saline (PBS). Gentamycin, which kills extracellular bacteria but cannot penetrate into host cells, was added into each well to a final concentration of 100 μg/ml, and the cultures were incubated at 37°C for 60 min. Cells were washed twice to remove the antibiotic, suspended in PBS containing 0.2% Triton X-100 (Sigma-Aldrich, MO, USA), diluted, and plated on Luria-Bertani (LB)-agar plates. The level of internalization of bacteria in host cells was calculated by determining the CFUs recovered from lysed cells.
For the inhibition assay, Mannan (500 μg/ml), anti-hDC-SIGN antibody (5 μg/ml), and anti-mSIGNR1 antibody (5 μg/ml) were added 20 min prior to the addition of bacteria. The concentrations used were determined based on our preliminary data and were selected because at these concentrations, the compounds did not affect the survival of bacteria or host cells, as previously shown (Zhang et al., 2006, 2008).
For further analysis of the O-antigen expression pattern of UPEC strains, LPS samples were extracted and purified from UPEC strains using an LPS extraction kit (iNtRON Biotechnology, Korea), performed according to the manufacturer's instructions. The E. coli strains CS180 and CS1861, which show rough LPS (without O-antigen) and smooth LPS (with O-antigen), respectively, were used as control strains. After purification, the LPS samples were analyzed using a PAGE silver staining kit (Solarbio Science & Technology, Beijing, China). After a series of washes, incubation, and termination, the signals were visualized using a Bio-Rad gel imager (Hercules, CA, USA).
WT and SIGNR1−/− mice (five mice/group) at 8 weeks of age were intraperitoneally injected with 106 CFUs of UTI89 suspended in 100 μl PBS. All mice were monitored according to ethics requirements. After 24 h of infection, the mice were sacrificed and livers, spleens, and mesenteric lymph nodes (MLNs) were collected, weighed, and washed in PBS with Gentamycin (100 μg/ml) to remove bacteria that had not invaded tissues. Tissues were washed three times to remove the antibiotic, and then homogenized in 1 ml 1% Triton X-100. Dilutions were spread on LB-agar plates and incubated overnight before CFUs were counted to determine CFUs per organ.
Six- to eight-week-old mice were catheterized for inoculation of 108 CFUs of UTI89 suspended in 30 μl PBS under sodium pentobarbital anesthesia (Chan et al., 2013). Control experiments were performed with application of 30 μl PBS. At the indicated times after infection, mice were individually induced to urinate by applying gentle pressure to the skin just below the occiput. The clean-catch urine samples were collected into sterile Eppendorf tubes (Eppendorf, Hamburg, Germany), and bacterial titers were determined by plating serial dilutions of the material onto LB-agar plates. Dilutions were also examined under microscopy for polymorphonuclear leukocyte (PMN) levels with a hemocytometer (Bioleaf Biotech Co., Ltd, Shanghai, China). Bacterial titers in the bladder were determined by homogenizing the tissue in 1 ml of sterile PBS containing 0.5% Triton X-100, and plating serial dilutions of the homogenate onto LB-agar plates. Mice were sacrificed by cervical dislocation under anesthesia, and their bladders were aseptically removed and frozen before sectioning.
Bladder tissues were aseptically harvested from mice at the indicated time points, and 4% paraformaldehyde fixed tissues were embedded in paraffin, sectioned, and stained with hemotoxylin and eosin and Masson's trichrome. Bladder inflammatory scores over the total area of each section were determined by criteria as previously described (Hopkins et al., 1998).
Peritoneal macrophages collected from WT and SIGNR1−/− mice were cultured in 24-well plates (1×105 cells per well, in a volume of 1 ml). After incubation with 106 CFUs of UTI89-GFP for 2 h, the cell layers were washed three times with PBS to remove unbound bacteria. Cells were fixed in cold methanol for 10 min and incubated for 1 h in 1% BSA (Sigma-Aldrich, MO, USA) and 0.3% Triton X-100 in PBS to block non-specific binding and permeabilize the cells. The cells were then incubated for 1 h at room temperature with a primary rabbit IgG antibody (Sino Biological Inc., Beijing, China) directed against mouse SIGNR1. Primary antibodies were detected with a goat anti–rabbit IgG-conjugated with Alexa Fluor 594 (Invitrogen, CA, USA). The cell-associated bacteria were quantified by counting cells and bacteria that attached to or inside macrophages in three fields under microscope, and bacterial count per 100 cells was calculated.
For immunofluorescence analysis, frozen bladder sections were cut and fixed in acetone at −20°C for 10 min. All sections were hydrated and blocked in 1% BSA, 0.3% Triton X-100 in PBS. After incubation with primary antibodies to detect against mouse SIGNR1 (Sino Biological Inc. Beijing, China) and E. coli (Abcam, MA, USA), incubation with secondary antibodies, and associated washes, slides were stained with bis-benzimide (Sigma, MO, USA). Stained tissues were examined by epifluorescence microscopy on a ZEISS Axioskop 2 MOT Plus microscope.
Bladder tissue was harvested at indicated time points and stored at−80 °C until all sampled had been collected. For measuring interleukin-6 (IL6), tumor necrosis factor (TNF)-α and interleukin-10 (IL10) expression in bladder homogenates, enzyme-linked immunosorbent assay (ELISA) Kits (Shanghai Enzyme-linked Biotechnology Co., Ltd. Shanghai, China) using matched antibody pairs were used according to the manufacturer's instructions.
Statistical tests were obtained using the Prism software version 6 (Graph Pad, San Diego, CA, USA). Statistically significant differences between two groups were determined by non-parametric t-test and comparison among three or more groups was obtained by one-way or two-way analysis of variance (ANOVA) with multiple comparisons test. Additionally, p-values were deemed significant if < 0.05. Data are presented as mean values ± standard error of mean (SEM).
We have previously shown that human DC-SIGN (hDC-SIGN, hCD209a) is a receptor for E.coli K-12 with core lipopolysaccharide (LPS core) (Klena et al., 2005; Zhang et al., 2006). However, we had evidence showing that mouse SIGNR1 (mSIGNR1, mCD209b), not mouse DC-SIGN (mCD209a), serves as a receptor for the LPS core of other Gram-negative bacteria, such as Yersinia spp. (Klena et al., 2005; Zhang et al., 2006, 2008; He et al., 2019; Yang et al., 2019). To assess the involvement of mSIGNR1 in the interaction of uropathogenic E. coli and macrophages in vitro, we evaluated the phagocytic capacity of peritoneal macrophages derived from SIGNR1−/− mice in six UPEC strains. The UTI89 strain carrying a pAcGFP1 plasmid (UTI89-GFP) was used for immunofluorescence analysis. After incubation with UTI89-GFP, peritoneal macrophages from WT mice or SIGNR1−/− mice were stained for SIGNR1, and the distributions of SIGNR1 and bacteria were investigated by immunofluorescence (Figure 1A). Macrophages from WT mice expressed high levels of SIGNR1. The cell-associated bacteria were also quantified, and macrophages from SIGNR1−/− mice showed less cell-associated bacteria than macrophages from WT mice (Figure 1B). Additionally, phagocytosis of UPEC by peritoneal macrophages from SIGNR1−/− mice was significantly reduced compared to that of macrophages from WT mice (Figure 1C). The reduced phagocytosis of SIGNR1−/− macrophages was not attributed to the effect of SIGNR1 absence on macrophage viability or reactive oxygen species (ROS), which can be produced as early as 30 min post-phagocytosis (West et al., 2011) (Figures S1, S2). Taken together, this result indicates that macrophages lacking SIGNR1 exhibit reduced phagocytosis of UPEC strains, suggesting the involvement of SIGNR1 in the interaction between UPEC and macrophages.
Figure 1. Reduced phagocytosis of uropathogenic E. coli by macrophages from SIGNR1−/− mice. (A) Immunofluorescence (IF) microscopy of SIGNR1 expression and its interaction with UTI89. Green: UTI89; red: anti-SIGNR1; blue: DAPI. Scale bars ~10 μm in length. (B) Quantitation of cell-associated bacteria in macrophages from SIGNR1−/− and WT mice. (C) Invasion assays for phagocytosis of UPEC strains by mouse macrophages. Y. pseudotuberculosis Y1 and E. coli CS180 served as control strains. The results presented were pooled from three independent experiments. Mean ± SEM. **p < 0.01; ***p < 0.001. Mann-Whitney test (B); Two-way ANOVA with Sidak's multiple comparisons test (C).
To investigate whether hDC-SIGN and mSIGNR1 were responsible for the interaction of UPEC with human DCs and mouse macrophages, stably transfected cell lines expressing human DC-SIGN (CHO-hDC-SIGN) and murine SIGNR1 (CHO-mSIGNR1) were tested for their ability to phagocytose UPEC strains. Y1 (cultured at 26°C) and E. coli CS180, mediating SIGNR1-independent and SIGNR1-dependent interactions, respectively, were again used as control strains. UPEC strains invaded CHO-hDC-SIGN (Figure 2A) and CHO-mSIGNR1 (Figure 2B) cells more effective than that of CHO-NEO cells, indicating that hDC-SIGN and mSIGNR1 are receptors for the phagocytosis of UPEC strains by epithelial cells and macrophages. Moreover, the interaction between CHO-hDC-SIGN (Figure 2C) or CHO-mSIGNR1 (Figure 2D) and UPEC strains was significantly blocked by the addition of antibodies, suggesting a specific UPEC-CD209s interaction, which promotes bacterial invasion into mouse macrophages. Interestingly, after transformation with a plasmid that expresses O-antigen, phagocytosis of UTI89-O+ and CFT073-O+ by CHO-hDC-SIGN or CHO-mSIGNR1 cells was significantly reduced (Figure S3). Thus, the epitopes involved in UPEC-CD209s interaction may be shielded by over-expression of O-antigen.
Figure 2. Human DC-SIGN and mouse SIGNR1 are receptors for phagocytosis of UPEC strains. Y. pseudotuberculosis Y1 and E. coli CS180 were control strains. Phagocytosis of UPEC strains by (A) CHO/CHO-hDC-SIGN and (B) CHO/CHO-mSIGNR1 cells and inhibition by (C) anti-hDC-SIGN or (D) anti-mSIGNR1 were conducted as described in Methods. The number of phagocytosed bacteria was determined by counting CFUs recovered following gentamycin treatment. The results presented were pooled from three independent experiments. Mean ± SEM. **p < 0.01; ***p < 0.001. Two-way ANOVA with Sidak's multiple comparisons test.
Our recent studies showed that the LPS core of several Gram-negative bacteria, including Yersinia spp. and S. Typhimurium, exposed by loss of O-antigen, interacts with CD209s (He et al., 2019; Yang et al., 2019; Ye et al., 2019). To determine whether the LPS core of UPEC strains is a ligand for CD209s, we conducted silver staining of LPS from clinical isolates. The isolates UPEC2 and UPEC4 showed no expression of O-antigen, which is in accordance with CS180, a rough strain (Figure 3A). The invasion assays showed that there were no significant differences between these isolates upon interaction with macrophages or CD209s-expressing cell lines (Figures 1, 2), indicating that the interaction between UPEC strains and CD209s may not be mediated by a LPS core-CD209s interaction.
Figure 3. The UPEC-CD209s interaction could not be inhibited by mannan. (A) Silver-staining of the LPS of UPEC strains. Controls: E. coli strains CS180 and CS1861, which show rough LPS (without O-antigen) and smooth LPS (with O-antigen), respectively. The data presented are representative of three independent experiments. Phagocytosis of UPEC by (B) CHO-hDC-SIGN cells and (C) CHO-mSIGNR1 cells. The phagocytosis rate was determined by the recovery of bacteria following gentamycin treatment. The data presented were pooled from three independent experiments. Mean ± SEM. **p < 0.01; ***p < 0.001. Two-way ANOVA with Sidak's multiple comparisons test (B,C).
Mannan, which specifically binds mannose-related receptors and LPS core, inhibiting HIV-CD209 or LPS core-CD209s interactions (Geijtenbeek et al., 2000; Zhang et al., 2006), was used for an inhibition assay. Mannan inhibits the interactions between CS180 and CHO-hDC-SIGN or CHO-mSIGNR1 cells, as shown in our previous publication (Klena et al., 2005), but can limitedly inhibit hDC-SIGN- or mSIGNR1-mediated interactions with UPEC strains (Figures 3B,C). We therefore speculate that other sugar residues or LPS core with altered conformation on the surface of UPEC strains may mediate UPEC' interactions with host cells (Zhang et al., 2006; Yang et al., 2015).
Recent studies showed that Salmonella spp. and Yersinia spp. could target hDC-SIGN and mSIGNR1 receptors, leading to their dissemination and infection (He et al., 2019; Yang et al., 2019; Ye et al., 2019). We therefore hypothesized that the dissemination of UPEC to livers, spleens, and mesenteric lymph nodes (MLNs) would also be facilitated by this host-pathogen interaction. Therefore, if the interaction could be inhibited, dissemination should be reduced. SIGNR1−/− mice and WT mice were intraperitoneally injected with UTI89 to evaluate UPEC dissemination in the murine host. Lower numbers of UTI89 were isolated from the livers and spleens of SIGNR1−/− mice compared to those of WT mice (Figure 4). Upon infection, both WT and SIGNR1−/− mice showed weight loss and reduced activities, but the amount of weight lost did not statistically differ between the WT and SIGNR1−/− mice at 24 h post-infection (Figure S4). Thus, the decreased dissemination of bacteria in SIGNR1−/− mice may mainly reflect the reduced phagocytosis of UPEC by macrophages.
Figure 4. Fewer bacteria were disseminated to livers and spleens in SIGNR1−/− mice than in WT mice. WT mice and SIGNR1−/− mice (N = 5 mice/group) were intraperitoneally infected with 106 CFUs of UTI89. Mice were sacrificed at 24 h post-infection, and homogenates of livers, spleens, and mesenteric lymph nodes were plated onto LB plates. The dissemination was determined by CFUs recovered from these organs. The data presented were pooled from three independent experiments. Mean ± SEM. *p < 0.05. Two-way ANOVA with Sidak's multiple comparisons test.
Murine SIGNR1 is involved in interactions between UPEC strains and host cells in vitro. To test whether SIGNR1 is functionally essential during infection with UPEC, we infected SIGNR1−/− and WT mice with cystitis isolate UTI89. After the initial inoculation with 108 CFUs of bacteria, bacterial titers in urine and bladder, as well as histopathological scores, were measured to evaluate the host response to UPEC infection. The SIGNR1−/− mice had higher bacterial titers in urine by day 5 compared to the levels in WT mice (Figure 5A). All the infected WT mice cleared the bacteria within 10 days post-infection, evidenced by sterile urine (Figure 5A). A small population of bacteria could persist in the bladder of mice within or underneath the superficial bladder epithelium, although the urine of these mice is sterile (Chan et al., 2013).
Figure 5. SIGNR1−/− mice had higher bacterial titers in the urine and bladder than WT mice. (A) Urine bacterial titers of SIGNR1−/− and WT mice (N = 10 mice/group) time course over 2 weeks post-infection with 108 CFUs of UTI89. Each point represents the value from an individual mouse and the data presents mean ± SEM. (B) Hemocytometer counts of polymorphonuclear leukocyte (PMN) levels in the urine of mice 24 h post infection. (C) Immunofluorescence of bladders from WT and SIGNR1−/− mice (N = 3 mice/group) at 24 h post-infection. Bladder sections were stained for mouse SIGNR1 (red) and UPEC (green) by related monoclonal antibodies, with nuclei counterstained with bis-benzimide (blue). “L” denotes bladder lumen; dashed line indicates approximate location of epithelial surface; arrowheads point to bacterial colonization in the right panel. Scale bars approximate 100 μm in length. Data are representative of three independent experiments. (D) Bladder bacterial titers of SIGNR1−/− and WT mice (N = 6/group) time course over 3 weeks post-infection with 108 CFUs of UTI89. Each point represents the value from an individual mouse. The data presented were pooled from three independent experiments. Mean ± SEM. *p < 0.05; **p < 0.01; ***p < 0.001. Two-way ANOVA with Sidak's multiple comparisons test (A.B,D).
The innate inflammatory response, involving neutrophil recruitment, is activated upon UPEC infection and can resolve the acute phase of bacterial infection (Haraoka et al., 1999). We examined the PMN levels in the urine of infected mice. The urine PMN level in SIGNR1−/− mice was higher than that of WT mice (Figure 5B). Bladders harvested from SIGNR1−/− mice had a higher bacterial burden than those of WT mice at 24 h post-infection (Figure 5C), and no bacteria were detected in mock infection of these mice (Figure S5). Bacterial titers in bladders were also evaluated to determine the persistence of UPEC infections. The UPEC persisted longer in bladders of SIGNR1−/− mice than in those of WT mice. The WT mice exhibited complete clearance of bacteria in the bladder by 14 days post-infection, and SIGNR1−/− mice reached carrier state by 21 days (Figure 5D).
Histopathological analysis of bladder tissue was performed at different time points on a random subset of mice. Both WT and SIGNR1−/− mice exhibited robust inflammatory responses, including neutrophil infiltration accompanied by edema, urothelial exfoliation, and crypt abscess (Figure 6A). Bladders from SIGNR1−/− demonstrated lesions of inflammation with more abundant neutrophil infiltration and crypt abscess than those observed in WT mice throughout the experimental period (Figure 6A). Moreover, WT mice appeared to resolve the acute phase of infection by 2 weeks post-infection. The inflammatory scores obtained from histopathological analysis showed that SIGNR1−/− mice suffered more severe inflammatory lesions than WT mice did (Figure 6B). The observations from Masson' trichrome staining revealed epithelial lesions in the bladders of infected mice by 3 weeks post-infection. Urothelial hyperplasia and remodeling were observed in bladders of SIGNR1−/− mice, while WT mouse bladders showed almost normal structure (Figure 6C). These results indicate that the mice lacking SIGNR1 have an impaired ability to clear bacteria.
Figure 6. Absence of SIGNR1 increases susceptibility to cystitis caused by UTI89. (A) Infected SIGNR1−/− and WT mice (N = 8 mice/group) were sacrificed at days 1, 7, and 14 post-infection. Paraffin-embedded, fixed bladder sections were stained with hematoxylin and eosin (HE) and examined by light microscopy. “L” denotes bladder lumen; the black triangles point to exfoliation of epithelial cells; white triangles indicates abscess area. Scale bars ~100 μm in length. (B) Hematoxylin and eosin staining of bladder sections from 7 days post-infection mice. Sections were scored from 0 to 5 to quantify the severity of inflammatory lesions, horizontal bars indicate median values **p < 0.01. One-way ANOVA with Holm-Sidak's multiple comparisons test. (C) Masson' trichrome staining of bladder sections of 21 days post infection mice (N = 5 mice/group). The black boxes in upper panels correspond to the higher magnification images in lower panels. Scale bars ~100 μm in length. The black arrow indicates collagen deposition and epithelial remodeling. “L” denotes bladder lumen.
To better understand the underlying differences in inflammatory response to UPEC infection in the SIGNR1−/− and WT mice, we studied the cytokine profile of bladder tissues in both mice groups during E. coli infection at the expected time frame for immune response. As expected, a strong proinflammatory response, demonstrated by drastic cytokine expression, including IL-6 and TNF-α, was observed early upon infection (Figures 7A,B). Interestingly, a major inhibitor of proinflammatory immune responses, IL-10, exhibited high levels of expression parallel to the expression levels of proinflammatory cytokines (Figure 7C). However, we found that there were no differences between the SIGNR1−/− and WT mice in the local IL-10 response, nor in the levels of IL-6 and TNF-α. The IL-10 level in SIGNR1−/− mice was slightly lower than that of WT mice, indicating that severe inflammation of SIGNR1−/− may partly be related to IL-10 expression. Based on this cytokine profile, we could not determine a role for SIGNR1 in the induction of cytokine production to modulate the inflammatory response and the concomitant cellular infiltration upon infection with UPEC.
Figure 7. No differences in cytokine IL6, TNF-α, or IL10 production in bladders of SIGNR1−/− or WT mice upon UPEC infection. Bladders of WT and SIGNR1−/− mice (N = 5 mice/group) at 6 and 24 h post-infection with PBS or 108 CFUs of UTI89 were harvested for cytokine (A) IL6, (B) TNF-α, and (C) IL10 assays in ELISA. The data presented were pooled from three independent experiments. Mean ± SEM. ns, no significance. One-way ANOVA with Holm-Sidak's multiple comparisons test.
Urinary tract infections (UTIs) caused by uropathogenic E. coli are characterized by a high incidence of recurrence. About half of recurrent UTIs are attributed to the same UPEC strain that caused the initial infection (Harrabi, 2012; Silverman et al., 2013), indicating that individuals with UTIs fail to mount an efficient immune response that protects against subsequent infections in the urinary tract. However, the molecular mechanism of how UPEC cause persistent infection remains largely unclear. In a recent study, we demonstrated that S. Typhimurium targets DC-SIGN (CD209s) expressed by APCs to promote host dissemination and infection in an animal model, which may provide an explanation of a persistent infection, such as carried by “Typhoid Mary.” The identification of the molecular mechanism of persistent bacterial infection, such as Helicobacter pylori-associated chronic gastritis and Mycobacterium tuberculosis-associated tuberculosis, would facilitate treatment of infections. These intracellular pathogens all share the ability to interact with CD209s for attachment and invasion of host cells (Tailleux et al., 2003; Driessen et al., 2009; Carroll et al., 2010; Miszczyk et al., 2012; Ye et al., 2019). Moreover, studies by Helaine et al. demonstrated that S. typhimurium could form intracellular persisters in macrophages that serve as reservoirs of bacterial persistence. In an UTI model, macrophages comprise nearly 40% of all APCs in the bladder immune cell compartment of mice (Mora-Bau et al., 2015), suggesting that macrophages may play important roles in local immune surveillance. Phagocytosis is a prominent feature of the macrophages' antibacterial host response and is mediated by pattern recognition receptors (PRRs). Hosts can counteract invading bacterial pathogens utilizing their defensive weapons of APCs, including DCs and macrophages. The interactions between host macrophages and uropathogens are considered crucial to initiate an efficient immune response that defends against invading bacteria in UTIs (Mora-Bau et al., 2015; Owusu-Boaitey et al., 2016; Lacerda Mariano and Ingersoll, 2018).
This study demonstrates that human DC-SIGN and murine SIGNR1 are receptors for recognition and phagocytosis of UPEC strains by CD209s-expressing macrophages and transfectants. The UPEC-CD209s interaction can be significantly inhibited by the addition of an anti-CD209s antibody, indicating that CD209s are receptors for UPEC. However, the mSIGNR1- or hDC-SIGN-mediated interactions was only limitedly inhibited by mannan in this study, which shows discrepancy to our results in previous studies (Zhang et al., 2006; Ye et al., 2019). Two out of four clinical UPEC isolates were rough strains without O-antigen, but showed no differences in their abilities to invade host cells, suggesting that the UPEC-CD209s interaction was LPS core-independent. Interestingly, after transfection with O-antigen-expressing plasmid, the UPEC strain UTI89 exhibited significantly decreased phagocytosis by CD209-expressing transfectants, indicating that the over-expression of O-antigen may shield the interface of the UPEC-CD209s interaction. The type 1 pilus-associated adhesin FimH is the major facilitator of UPEC entry into host cells, and has been reported to bind a wide range of mannose-containing receptors (Leusch et al., 1991; Kukkonen et al., 1993; Eto et al., 2007; Mossman et al., 2008; Ielasi et al., 2016). We therefore examined the possible binding of FimH to mSIGNR1 with the recombinant FimH protein. The result indicates that the FimH can inhibit interactions between the UTI89 strain and CHO/CHO-mSIGNR1 cells; however, the FimH could bind to both CHO and CHO-mSIGNR1 cells, suggesting that the FimH may not specific ligand for the SIGNR1 receptor (Figure S6). Additionally, the sialic acids of polysaccharide capsules play crucial roles in bacterial resistance to phagocytosis and serum killing, as well as the formation of IBCs (Buckles et al., 2009; Anderson et al., 2010; Goller and Seed, 2010). Given the affinity of CD209s to carbohydrate chains exposed on several Gram-negative pathogens, further studies are needed to specify the ligands on UPEC.
SIGNR1 (CD209b) is a mouse homolog of the human DC-SIGN receptor that is reported to interact with several bacterial species (Tailleux et al., 2003; Lanoue et al., 2004; Yang et al., 2019; Ye et al., 2019), but its functional role in vivo in UTIs has not been well-defined. We examined SIGNR1-deficient (SIGNR1−/−) mice in a UTI model. We demonstrate that in SIGNR1−/− mice, the bacterial burden in the bladder and the intensity of inflammatory responses, were significantly higher than those of WT mice over time post-infection. The difference in the inflammatory response upon UPEC infection between SIGNR1−/− and WT mice is not due to changes in the expression levels of IL-6, TNF-α, or IL-10, but is consistent with the observed reduction of the phagocytic ability of SIGNR1−/− macrophages. Nevertheless, it appears that the IL-10 level in SIGNR1−/− mice was slightly lower than that of WT mice, suggesting that severe inflammation of SIGNR1−/− may partly be related to IL-10, which is characterized as an immunosuppressive cytokine.
The C-type lectin receptors (CLRs) are involved in modulatory processes of immunity by cross-talk with other PRRs, such as Toll-like receptors (TLRs). Studies by Geijtenbeek et al. showed that the binding of DC-SIGN with ManLAM derived from Mycobacterium tuberculosis affects intracellular signaling pathways through communication with TLRs, thus modulating cytokine production involved in inflammatory responses (Geijtenbeek et al., 2003; Gringhuis et al., 2007, 2009). Because of its broad specificity for mannose and/or fucose-terminated glycan, DC-SIGN can mediate the interaction between host cells and numerous pathogens (Ehlers, 2010; Miszczyk et al., 2012; He et al., 2019; Ye et al., 2019). In our recent work, the SIGNR1−/− mice were more susceptible to Y. pestis infection compared to WT mice (Yang et al., 2019), and this difference may reflect the multifunctional properties of this receptor in the host immune system. The complement system plays a significant role in hosts' anti-infection processes. Kang et al. found involvement of SIGNR1 in the complement fixation pathway by interaction with C1q (Kang et al., 2006). Overall, experiments with knockout mice are required to better understand the in vivo function of SIGNR1. However, there are potential limitations of this approach to study bacteria-host cell interactions, and this strategy may only work if SIGNR1 is the only, or the main receptor for pathogens. Unfortunately, many pathogens do not depend on only one receptor in their interactions with host cells.
In summary, this is the first demonstration that human DC-SIGN and mouse SIGNR1 are cellular receptors for recognition and phagocytosis of uropathogenic E. coli by host cells. Murine SIGNR1 contributes to clearance of UPEC in an UTI model. However, the specific UPEC molecules that bind to CD209s were not identified. We also observed that over-expression of O-antigen significantly inhibited the UPEC-CD209s interactions. Further investigations are needed to determine the corresponding UPEC ligands, as we did previously for the identification of the carbohydrate LPS core from many Gram-negative bacteria (Klena et al., 2005; Zhang et al., 2006, 2008; Yang et al., 2015, 2019; He et al., 2019; Ye et al., 2019). Several studies showed that in vivo targeting of CD209s drastically enhances antigen presentation as well as persistent CD8+/CD4+ T-cell-mediated protective immunity against intracellular pathogens (Singh et al., 2009; Unger et al., 2012; Hesse et al., 2013; Schetters et al., 2018). Thus, given the protective role of SIGNR1 in UTIs, the interaction between UPEC and CD209s should be targeted in treatment strategies to prevent persistent UTIs.
The datasets generated for this study are available on request to the corresponding author.
The studies involving human participants were reviewed and approved by Medical Ethics Committee of Tongji Hospital, Tongji Medical College, Huazhong University of Science and Technology. The patients/participants provided their written informed consent to participate in this study. The animal study was reviewed and approved by Institutional Animal Care and Use Committees and Institutional Review Board of Tongji Hospital, Tongji Medical College, Huazhong University of Science and Technology.
TC, HCa, and YZ designed and conceived the experiments. YZ, SZha, YH, and BW performed the experiments. YZ, YH, CY, YX, QL, and WL analyzed the data. YL, LJ, and SZhu contributed reagents, materials, and analytical tools. YZ, HCh, WC, ZS, and TC wrote and reviewed the manuscript.
This work was supported by grants from the National Natural Science Foundation of China (NSFC 81271780 and 81471915) and by two local grants from Tongji Hospital, Tongji Medical College to Tie Chen.
The authors declare that the research was conducted in the absence of any commercial or financial relationships that could be construed as a potential conflict of interest.
The Supplementary Material for this article can be found online at: https://www.frontiersin.org/articles/10.3389/fcimb.2019.00457/full#supplementary-material
Abraham, S. N., and Miao, Y. (2015). The nature of immune responses to urinary tract infections. Nat. Rev. Immunol. 15, 655–663. doi: 10.1038/nri3887
Anderson, G. G., Goller, C. C., Justice, S., Hultgren, S. J., and Seed, P. C. (2010). Polysaccharide capsule and sialic acid-mediated regulation promote biofilm-like intracellular bacterial communities during cystitis. Infect. Immun. 78, 963–975. doi: 10.1128/IAI.00925-09
Anderson, G. G., Palermo, J. J., Schilling, J. D., Roth, R., Heuser, J., and Hultgren, S. J. (2003). Intracellular bacterial biofilm-like pods in urinary tract infections. Science 301, 105–107. doi: 10.1126/science.1084550
Billips, B. K., Forrestal, S. G., Rycyk, M. T., Johnson, J. R., Klumpp, D. J., and Schaeffer, A. J. (2007). Modulation of host innate immune response in the bladder by uropathogenic Escherichia coli. Infect. Immun. 75, 5353–5360. doi: 10.1128/IAI.00922-07
Bokil, N. J., Totsika, M., Carey, A. J., Stacey, K. J., Hancock, V., Saunders, B. M., et al. (2011). Intramacrophage survival of uropathogenic Escherichia coli: differences between diverse clinical isolates and between mouse and human macrophages. Immunobiology 216, 1164–1171. doi: 10.1016/j.imbio.2011.05.011
Buckles, E. L., Wang, X., Lane, M. C., Lockatell, C. V., Johnson, D. E., Rasko, D. A., et al. (2009). Role of the K2 capsule in Escherichia coli urinary tract infection and serum resistance. J. Infect. Dis. 199, 1689–1697. doi: 10.1086/598524
Burns, S. M., and Hull, S. I. (1998). Comparison of loss of serum resistance by defined lipopolysaccharide mutants and an acapsular mutant of uropathogenic Escherichia coli O75:K5. Infect. Immun. 66, 4244–4253.
Cai, W., Cai, X., Yang, Y., Yan, S., and Zhang, H. (2017). Transcriptional control of dual transporters involved in alpha-ketoglutarate utilization reveals their distinct roles in uropathogenic Escherichia coli. Front. Microbiol. 8:275. doi: 10.3389/fmicb.2017.00275
Carey, A. J., Sullivan, M. J., Duell, B. L., Crossman, D. K., Chattopadhyay, D., Brooks, A. J., et al. (2016). Uropathogenic Escherichia coli engages CD14-dependent signaling to enable bladder-macrophage-dependent control of acute urinary tract infection. J. Infect. Dis. 213, 659–668. doi: 10.1093/infdis/jiv424
Carroll, M. V., Sim, R. B., Bigi, F., Jakel, A., Antrobus, R., and Mitchell, D. A. (2010). Identification of four novel DC-SIGN ligands on Mycobacterium bovis BCG. Protein Cell 1, 859–870. doi: 10.1007/s13238-010-0101-3
Chan, C. Y., St John, A. L., and Abraham, S. N. (2013). Mast cell interleukin-10 drives localized tolerance in chronic bladder infection. Immunity 38, 349–359. doi: 10.1016/j.immuni.2012.10.019
Chen, T., Belland, R. J., Wilson, J., and Swanson, J. (1995). Adherence of pilus- Opa+ gonococci to epithelial cells in vitro involves heparan sulfate. J. Exp. Med. 182, 511–517. doi: 10.1084/jem.182.2.511
Chen, T., Bolland, S., Chen, I., Parker, J., Pantelic, M., Grunert, F., et al. (2001). The CGM1a (CEACAM3/CD66d)-mediated phagocytic pathway of Neisseria gonorrhoeae expressing opacity proteins is also the pathway to cell death. J. Biol. Chem. 276, 17413–17419. doi: 10.1074/jbc.M010609200
Chen, T., Grunert, F., Medina-Marino, A., and Gotschlich, E. C. (1997). Several carcinoembryonic antigens (CD66) serve as receptors for gonococcal opacity proteins. J. Exp. Med. 185, 1557–1564. doi: 10.1084/jem.185.9.1557
Cortes, G., Borrell, N., de Astorza, B., Gomez, C., Sauleda, J., and Alberti, S. (2002). Molecular analysis of the contribution of the capsular polysaccharide and the lipopolysaccharide O side chain to the virulence of Klebsiella pneumoniae in a murine model of pneumonia. Infect. Immun. 70, 2583–2590. doi: 10.1128/IAI.70.5.2583-2590.2002
Driessen, N. N., Ummels, R., Maaskant, J. J., Gurcha, S. S., Besra, G. S., Ainge, G. D., et al. (2009). Role of phosphatidylinositol mannosides in the interaction between mycobacteria and DC-SIGN. Infect. Immun. 77, 4538–4547. doi: 10.1128/IAI.01256-08
Ehlers, S. (2010). DC-SIGN and mannosylated surface structures of Mycobacterium tuberculosis: a deceptive liaison. Eur. J. Cell Biol. 89, 95–101. doi: 10.1016/j.ejcb.2009.10.004
Engering, A., Van Vliet, S. J., Geijtenbeek, T. B., and Van Kooyk, Y. (2002). Subset of DC-SIGN(+) dendritic cells in human blood transmits HIV-1 to T lymphocytes. Blood 100, 1780–1786. doi: 10.1182/blood-2001-12-0179
Eto, D. S., Jones, T. A., Sundsbak, J. L., and Mulvey, M. A. (2007). Integrin-mediated host cell invasion by type 1-piliated uropathogenic Escherichia coli. PLoS Pathog. 3:e100. doi: 10.1371/journal.ppat.0030100
Foxman, B. (2002). Epidemiology of urinary tract infections: incidence, morbidity, and economic costs. Am J Med. 113 (Suppl. 1A):5s−13s. doi: 10.1016/S0002-9343(02)01054-9
Geijtenbeek, T. B., Kwon, D. S., Torensma, R., van Vliet, S. J., van Duijnhoven, G. C., Middel, J., et al. (2000). DC-SIGN, a dendritic cell-specific HIV-1-binding protein that enhances trans-infection of T cells. Cell 100, 587–597. doi: 10.1016/S0092-8674(00)80694-7
Geijtenbeek, T. B., Van Vliet, S. J., Koppel, E. A., Sanchez-Hernandez, M., Vandenbroucke-Grauls, C. M., Appelmelk, B., et al. (2003). Mycobacteria target DC-SIGN to suppress dendritic cell function. J. Exp. Med. 197, 7–17. doi: 10.1084/jem.20021229
Goller, C. C., and Seed, P. C. (2010). Revisiting the Escherichia coli polysaccharide capsule as a virulence factor during urinary tract infection: contribution to intracellular biofilm development. Virulence 1, 333–337. doi: 10.4161/viru.1.4.12388
Gringhuis, S. I., den Dunnen, J., Litjens, M., van der Vlist, M., and Geijtenbeek, T. B. (2009). Carbohydrate-specific signaling through the DC-SIGN signalosome tailors immunity to Mycobacterium tuberculosis, HIV-1 and Helicobacter pylori. Nat. Immunol. 10, 1081–1088. doi: 10.1038/ni.1778
Gringhuis, S. I., den Dunnen, J., Litjens, M., van Het Hof, B., van Kooyk, Y., and Geijtenbeek, T. B. (2007). C-type lectin DC-SIGN modulates Toll-like receptor signaling via Raf-1 kinase-dependent acetylation of transcription factor NF-kappaB. Immunity 26, 605–616. doi: 10.1016/j.immuni.2007.03.012
Haraoka, M., Hang, L., Frendeus, B., Godaly, G., Burdick, M., Strieter, R., et al. (1999). Neutrophil recruitment and resistance to urinary tract infection. J. Infect. Dis. 180, 1220–1229. doi: 10.1086/315006
Harrabi, H. (2012). Uncomplicated urinary tract infection. N. Engl. J. Med. 367, 185; author reply 185. doi: 10.1056/NEJMc1205507
He, Y. X., Ye, C. L., Zhang, P., Li, Q., Park, C. G., Yang, K., et al. (2019). Yersinia pseudotuberculosis exploits CD209 receptors for promoting host dissemination and infection. Infect. Immun. 87:e00654-18. doi: 10.1128/IAI.00654-18
Helaine, S., Cheverton, A. M., Watson, K. G., Faure, L. M., Matthews, S. A., and Holden, D. W. (2014). Internalization of Salmonella by macrophages induces formation of nonreplicating persisters. Science 343, 204–208. doi: 10.1126/science.1244705
Hesse, C., Ginter, W., Forg, T., Mayer, C. T., Baru, A. M., Arnold-Schrauf, C., et al. (2013). In vivo targeting of human DC-SIGN drastically enhances CD8(+) T-cell-mediated protective immunity. Eur. J. Immunol. 43, 2543–2553. doi: 10.1002/eji.201343429
Hopkins, W. J., Gendron-Fitzpatrick, A., Balish, E., and Uehling, D. T. (1998). Time course and host responses to Escherichia coli urinary tract infection in genetically distinct mouse strains. Infect. Immun. 66, 2798–2802.
Horvath, D. J. Jr., Li, B., Casper, T., Partida-Sanchez, S., Hunstad, D. A., Hultgren, S. J., et al. (2011). Morphological plasticity promotes resistance to phagocyte killing of uropathogenic Escherichia coli. Microbes Infect. 13, 426–437. doi: 10.1016/j.micinf.2010.12.004
Ielasi, F. S., Alioscha-Perez, M., Donohue, D., Claes, S., Sahli, H., Schols, D., et al. (2016). Lectin-glycan interaction network-based identification of host receptors of microbial pathogenic adhesins. MBio 7. doi: 10.1128/mBio.00584-16
Isberg, R. R., and Leong, J. M. (1990). Multiple beta 1 chain integrins are receptors for invasin, a protein that promotes bacterial penetration into mammalian cells. Cell 60, 861–871. doi: 10.1016/0092-8674(90)90099-Z
Justice, S. S., Hung, C., Theriot, J. A., Fletcher, D. A., Anderson, G. G., Footer, M. J., et al. (2004). Differentiation and developmental pathways of uropathogenic Escherichia coli in urinary tract pathogenesis. Proc. Natl. Acad. Sci. U.S.A. 101, 1333–1338. doi: 10.1073/pnas.0308125100
Justice, S. S., Hunstad, D. A., Seed, P. C., and Hultgren, S. J. (2006). Filamentation by Escherichia coli subverts innate defenses during urinary tract infection. Proc. Natl. Acad. Sci. U.S.A. 103, 19884–19889. doi: 10.1073/pnas.0606329104
Kang, Y. S., Do, Y., Lee, H. K., Park, S. H., Cheong, C., Lynch, R. M., et al. (2006). A dominant complement fixation pathway for pneumococcal polysaccharides initiated by SIGN-R1 interacting with C1q. Cell 125, 47–58. doi: 10.1016/j.cell.2006.01.046
Kerrn, M. B., Struve, C., Blom, J., Frimodt-Moller, N., and Krogfelt, K. A. (2005). Intracellular persistence of Escherichia coli in urinary bladders from mecillinam-treated mice. J. Antimicrob. Chemother. 55, 383–386. doi: 10.1093/jac/dki002
Klena, J., Zhang, P., Schwartz, O., Hull, S., and Chen, T. (2005). The core lipopolysaccharide of Escherichia coli is a ligand for the dendritic-cell-specific intercellular adhesion molecule nonintegrin CD209 receptor. J. Bacteriol. 187, 1710–1715. doi: 10.1128/JB.187.5.1710-1715.2005
Klena, J. D., Ashford, R. S. II., and Schnaitman, C. A. (1992). Role of Escherichia coli K-12 rfa genes and the rfp gene of Shigella dysenteriae 1 in generation of lipopolysaccharide core heterogeneity and attachment of O antigen. J. Bacteriol. 174, 7297–7307. doi: 10.1128/jb.174.22.7297-7307.1992
Klena, J. D., and Schnaitman, C. A. (1993). Function of the rfb gene cluster and the rfe gene in the synthesis of O antigen by Shigella dysenteriae 1. Mol. Microbiol. 9, 393–402. doi: 10.1111/j.1365-2958.1993.tb01700.x
Klumpp, D. J., Weiser, A. C., Sengupta, S., Forrestal, S. G., Batler, R. A., and Schaeffer, A. J. (2001). Uropathogenic Escherichia coli potentiates type 1 pilus-induced apoptosis by suppressing NF-kappaB. Infect. Immun. 69, 6689–6695. doi: 10.1128/IAI.69.11.6689-6695.2001
Kukkonen, M., Raunio, T., Virkola, R., Lahteenmaki, K., Makela, P. H., Klemm, P., et al. (1993). Basement membrane carbohydrate as a target for bacterial adhesion: binding of type I fimbriae of Salmonella enterica and Escherichia coli to laminin. Mol. Microbiol. 7, 229–237. doi: 10.1111/j.1365-2958.1993.tb01114.x
Lacerda Mariano, L., and Ingersoll, M. A. (2018). Bladder resident macrophages: mucosal sentinels. Cell. Immunol. 330, 136–141. doi: 10.1016/j.cellimm.2018.01.018
Lanoue, A., Clatworthy, M. R., Smith, P., Green, S., Townsend, M. J., Jolin, H. E., et al. (2004). SIGN-R1 contributes to protection against lethal pneumococcal infection in mice. J. Exp. Med. 200, 1383–1393. doi: 10.1084/jem.20040795
Leusch, H. G., Drzeniek, Z., Markos-Pusztai, Z., and Wagener, C. (1991). Binding of Escherichia coli and Salmonella strains to members of the carcinoembryonic antigen family: differential binding inhibition by aromatic alpha-glycosides of mannose. Infect. Immun. 59, 2051–2057.
Loughman, J. A., and Hunstad, D. A. (2011). Attenuation of human neutrophil migration and function by uropathogenic bacteria. Microbes Infect. 13, 555–565. doi: 10.1016/j.micinf.2011.01.017
McDonald, D., Wu, L., Bohks, S. M., KewalRamani, V. N., Unutmaz, D., and Hope, T. J. (2003). Recruitment of HIV and its receptors to dendritic cell-T cell junctions. Science 300, 1295–1297. doi: 10.1126/science.1084238
Miszczyk, E., Rudnicka, K., Moran, A. P., Fol, M., Kowalewicz-Kulbat, M., Druszczynska, M., et al. (2012). Interaction of Helicobacter pylori with C-type lectin dendritic cell-specific ICAM grabbing nonintegrin. J. Biomed. Biotechnol. 2012:206463. doi: 10.1155/2012/206463
Mora-Bau, G., Platt, A. M., van Rooijen, N., Randolph, G. J., Albert, M. L., and Ingersoll, M. A. (2015). Macrophages subvert adaptive immunity to urinary tract infection. PLoS Pathog. 11:e1005044. doi: 10.1371/journal.ppat.1005044
Mossman, K. L., Mian, M. F., Lauzon, N. M., Gyles, C. L., Lichty, B., Mackenzie, R., et al. (2008). Cutting edge: FimH adhesin of type 1 fimbriae is a novel TLR4 ligand. J. Immunol. 181, 6702–6706. doi: 10.4049/jimmunol.181.10.6702
Mulvey, M. A., Lopez-Boado, Y. S., Wilson, C. L., Roth, R., Parks, W. C., Heuser, J., et al. (1998). Induction and evasion of host defenses by type 1-piliated uropathogenic Escherichia coli. Science 282, 1494–1497. doi: 10.1126/science.282.5393.1494
Murray, G. L., Attridge, S. R., and Morona, R. (2003). Regulation of Salmonella typhimurium lipopolysaccharide O antigen chain length is required for virulence; identification of FepE as a second Wzz. Mol. Microbiol. 47, 1395–1406. doi: 10.1046/j.1365-2958.2003.03383.x
Mysorekar, I. U., and Hultgren, S. J. (2006). Mechanisms of uropathogenic Escherichia coli persistence and eradication from the urinary tract. Proc. Natl. Acad. Sci. U.S.A. 103, 14170–14175. doi: 10.1073/pnas.0602136103
O'Brien, V. P., Hannan, T. J., Nielsen, H. V., and Hultgren, S. J. (2016). Drug and vaccine development for the treatment and prevention of urinary tract infections. Microbiol. Spectr. 4. doi: 10.1128/microbiolspec.UTI-0013-2012
Owusu-Boaitey, N., Bauckman, K. A., Zhang, T., and Mysorekar, I. U. (2016). Macrophagic control of the response to uropathogenic E. coli infection by regulation of iron retention in an IL-6-dependent manner. Immun. Inflamm. Dis. 4, 413–426. doi: 10.1002/iid3.123
Oyston, P. C., Prior, J. L., Kiljunen, S., Skurnik, M., Hill, J., and Titball, R. W. (2003). Expression of heterologous O-antigen in Yersinia pestis KIM does not affect virulence by the intravenous route. J Med. Microbiol. 52(Pt 4), 289–294. doi: 10.1099/jmm.0.05044-0
Ronald, A. (2002). The etiology of urinary tract infection: traditional and emerging pathogens. Am. J. Med. 113 (Suppl. 1A), 14s−19s. doi: 10.1016/S0002-9343(02)01055-0
Rycroft, J. A., Gollan, B., Grabe, G. J., Hall, A., Cheverton, A. M., Larrouy-Maumus, G., et al. (2018). Activity of acetyltransferase toxins involved in Salmonella persister formation during macrophage infection. Nat. Commun. 9:1993. doi: 10.1038/s41467-018-04472-6
Sanchez, G. V., Babiker, A., Master, R. N., Luu, T., Mathur, A., and Bordon, J. (2016). Antibiotic resistance among urinary isolates from female outpatients in the United States in 2003 and 2012. Antimicrob. Agents Chemother. 60, 2680–2683. doi: 10.1128/AAC.02897-15
Schaale, K., Peters, K. M., Murthy, A. M., Fritzsche, A. K., Phan, M. D., Totsika, M., et al. (2016). Strain- and host species-specific inflammasome activation, IL-1beta release, and cell death in macrophages infected with uropathogenic Escherichia coli. Mucosal Immunol. 9, 124–136. doi: 10.1038/mi.2015.44
Schetters, S. T. T., Kruijssen, L. J. W., Crommentuijn, M. H. W., Kalay, H., Ochando, J., den Haan, J. M. M., et al. (2018). Mouse DC-SIGN/CD209a as target for antigen delivery and adaptive immunity. Front. Immunol. 9:990. doi: 10.3389/fimmu.2018.00990
Schilling, J. D., Lorenz, R. G., and Hultgren, S. J. (2002). Effect of trimethoprim-sulfamethoxazole on recurrent bacteriuria and bacterial persistence in mice infected with uropathogenic Escherichia coli. Infect. Immun. 70, 7042–7049. doi: 10.1128/IAI.70.12.7042-7049.2002
Schiwon, M., Weisheit, C., Franken, L., Gutweiler, S., Dixit, A., Meyer-Schwesinger, C., et al. (2014). Crosstalk between sentinel and helper macrophages permits neutrophil migration into infected uroepithelium. Cell 156, 456–468. doi: 10.1016/j.cell.2014.01.006
Schnaitman, C. A., and Klena, J. D. (1993). Genetics of lipopolysaccharide biosynthesis in enteric bacteria. Microbiol. Rev. 57, 655–682.
Silverman, J. A., Schreiber, H. L. T., Hooton, T. M., and Hultgren, S. J. (2013). From physiology to pharmacy: developments in the pathogenesis and treatment of recurrent urinary tract infections. Curr. Urol. Rep. 14, 448–456. doi: 10.1007/s11934-013-0354-5
Singh, S. K., Stephani, J., Schaefer, M., Kalay, H., Garcia-Vallejo, J. J., den Haan, J., et al. (2009). Targeting glycan modified OVA to murine DC-SIGN transgenic dendritic cells enhances MHC class I and II presentation. Mol. Immunol. 47, 164–174. doi: 10.1016/j.molimm.2009.09.026
Stamm, W. E., and Norrby, S. R. (2001). Urinary tract infections: disease panorama and challenges. J. Infect. Dis. 183 (Suppl. 1), S1–S4. doi: 10.1086/318850
Stocks, C. J., Phan, M. D., Achard, M. E. S., Nhu, N. T. K., Condon, N. D., Gawthorne, J. A., et al. (2019). Uropathogenic Escherichia coli employs both evasion and resistance to subvert innate immune-mediated zinc toxicity for dissemination. Proc. Natl. Acad. Sci. U.S.A. 116, 6341–6350. doi: 10.1073/pnas.1820870116
Tailleux, L., Schwartz, O., Herrmann, J. L., Pivert, E., Jackson, M., Amara, A., et al. (2003). DC-SIGN is the major Mycobacterium tuberculosis receptor on human dendritic cells. J. Exp. Med. 197, 121–127. doi: 10.1084/jem.20021468
Takahara, K., Yashima, Y., Omatsu, Y., Yoshida, H., Kimura, Y., Kang, Y. S., et al. (2004). Functional comparison of the mouse DC-SIGN, SIGNR1, SIGNR3 and Langerin, C-type lectins. Int. Immunol. 16, 819–829. doi: 10.1093/intimm/dxh084
Unger, W. W., van Beelen, A. J., Bruijns, S. C., Joshi, M., Fehres, C. M., van Bloois, L., et al. (2012). Glycan-modified liposomes boost CD4+ and CD8+ T-cell responses by targeting DC-SIGN on dendritic cells. J. Control. Release 160, 88–95. doi: 10.1016/j.jconrel.2012.02.007
Waldhuber, A., Puthia, M., Wieser, A., Cirl, C., Durr, S., Neumann-Pfeifer, S., et al. (2016). Uropathogenic Escherichia coli strain CFT073 disrupts NLRP3 inflammasome activation. J. Clin. Invest. 126, 2425–2436. doi: 10.1172/JCI81916
West, A. P., Brodsky, I. E., Rahner, C., Woo, D. K., Erdjument-Bromage, H., Tempst, P., et al. (2011). TLR signalling augments macrophage bactericidal activity through mitochondrial ROS. Nature 472, 476–480. doi: 10.1038/nature09973
Yang, K., He, Y., Park, C. G., Kang, Y. S., Zhang, P., Han, Y., et al. (2019). Yersinia pestis Interacts With SIGNR1 (CD209b) for Promoting Host Dissemination and Infection. Front. Immunol. 10:96. doi: 10.3389/fimmu.2019.00096
Yang, K., Park, C. G., Cheong, C., Bulgheresi, S., Zhang, S., Zhang, P., et al. (2015). Host Langerin (CD207) is a receptor for Yersinia pestis phagocytosis and promotes dissemination. Immunol. Cell Biol. 93, 815–824. doi: 10.1038/icb.2015.46
Ye, C., Li, Q., Li, X., Park, C. G., He, Y., Zhang, Y., et al. (2019). Salmonella typhimurium interacts with CD209 receptors to promote host dissemination and infection. Infect. Immun. 87:e00100–19. doi: 10.1128/IAI.00100-19
Zhang, P., Skurnik, M., Zhang, S. S., Schwartz, O., Kalyanasundaram, R., Bulgheresi, S., et al. (2008). Human dendritic cell-specific intercellular adhesion molecule-grabbing nonintegrin (CD209) is a receptor for Yersinia pestis that promotes phagocytosis by dendritic cells. Infect. Immun. 76, 2070–2079. doi: 10.1128/IAI.01246-07
Keywords: uropathogenic E. coli, urinary tract infection, macrophage, DC-SIGN, SIGNR1
Citation: Zhang Y, Zhang S, He Y, Sun Z, Cai W, Lv Y, Jiang L, Li Q, Zhu S, Li W, Ye C, Wu B, Xue Y, Chen H, Cai H and Chen T (2020) Murine SIGNR1 (CD209b) Contributes to the Clearance of Uropathogenic Escherichia coli During Urinary Tract Infections. Front. Cell. Infect. Microbiol. 9:457. doi: 10.3389/fcimb.2019.00457
Received: 16 September 2019; Accepted: 16 December 2019;
Published: 10 January 2020.
Edited by:
Matthew S. Francis, Umeå University, SwedenReviewed by:
Keira Melican, Karolinska Institutet (KI), SwedenCopyright © 2020 Zhang, Zhang, He, Sun, Cai, Lv, Jiang, Li, Zhu, Li, Ye, Wu, Xue, Chen, Cai and Chen. This is an open-access article distributed under the terms of the Creative Commons Attribution License (CC BY). The use, distribution or reproduction in other forums is permitted, provided the original author(s) and the copyright owner(s) are credited and that the original publication in this journal is cited, in accordance with accepted academic practice. No use, distribution or reproduction is permitted which does not comply with these terms.
*Correspondence: Hongxiang Chen, aG9uZ3hpYW5nY2hlbkBob3RtYWlsLmNvbQ==; Huahua Cai, Q2xhcmVsdWNreTlAMTI2LmNvbQ==; Tie Chen, Y2hlbnRpZUBodXN0LmVkdS5jbg==; dGllY2hlbjIwMDVAeWFob28uY29t
†These authors have contributed equally to this work
Disclaimer: All claims expressed in this article are solely those of the authors and do not necessarily represent those of their affiliated organizations, or those of the publisher, the editors and the reviewers. Any product that may be evaluated in this article or claim that may be made by its manufacturer is not guaranteed or endorsed by the publisher.
Research integrity at Frontiers
Learn more about the work of our research integrity team to safeguard the quality of each article we publish.