- 1Centre des Sciences du Goût et de l'Alimentation, AgroSup Dijon, CNRS, INRAE, University of Bourgogne Franche-Comté, Eye and Nutrition Research Group, Dijon, France
- 2Micalis Institute, INRAE, AgroParisTech, University Paris-Saclay, Jouy-en-Josas, France
- 3Inserm U1231 “Lipids, Nutrition, Cancer”, Lipidomic Platform, University of Bourgogne Franche-Comté, Dijon, France
- 4Centre des Sciences du Goût et de l'Alimentation, AgroSup Dijon, CNRS, INRAE, University of Bourgogne Franche-Comté, ChemoSens Platform, Dijon, France
- 5Department of Ophthalmology, University Hospital, Dijon, France
Understanding the molecular mechanisms underlying the changes observed during aging is a prerequisite to design strategies to prevent age-related diseases. Aging is associated with metabolic changes, including alteration in the brain lipid metabolism. These alterations may contribute to the development of pathophysiological conditions. Modifications in the gut microbiota composition are also observed during aging. As communication axes exist between the gut microbiota and the brain and knowing that microbiota influences the host metabolism, we speculated on whether age-associated modifications in the gut microbiota could be involved in the lipid changes observed in aging brain. For that purpose, germ-free mice were colonized by the fecal microbiota of young or old donor mice. Lipid classes and fatty acid profiles were determined in the brain (cortex), plasma and liver by thin-layer chromatography on silica gel-coated quartz rods and gas chromatography. Gut colonization by microbiota of old mice resulted in a significant increase in total monounsaturated fatty acids (MUFA) and a significant decrease in the relative amounts of cholesterol and total polyunsaturated fatty acids (PUFA) in the cortex. Among the eight most represented fatty acids in the cortex, the relative abundances of five (C18:1n-9, C22:6n-3, C20:4n-6, C18:1n-7, and C20:1n-9) were significantly altered in mice inoculated with an aged microbiota. Liquid chromatography analyses revealed that the relative abundance of major species among phosphatidyl and plasmenylcholine (PC 16:0/18:1), phosphatidyl and plasmenylethanolamine (PE 18:0/22:6), lysophosphatidylethanolamine (LPE 22:6) and sphingomyelins (SM d18:1/18:0) were significantly altered in the cortex of mice colonized by the microbiota obtained from aged donors. Transplantation of microbiota from old mice also modified the lipid class and fatty acid content in the liver. Finally, we found that the expression of several genes involved in MUFA and PUFA synthesis (Scd1, Fads1, Fads2, Elovl2, and Elovl5) was dysregulated in mice inoculated with an aged microbiota. In conclusion, our data suggest that changes in gut microbiota that are associated with aging can impact brain and liver lipid metabolisms. Lipid changes induced by an aged microbiota recapitulate some features of aging, thus pointing out the potential role of microbiota alterations in the age-related degradation of the health status.
Introduction
Aging leads to cell degeneration and deterioration of organ structure and function on an irreversible and gradual manner. These conditions make aging a primary risk factor for major human disorders including cancers, metabolic and neurodegenerative diseases (Kennedy et al., 2014; Zierer et al., 2015). Aging is a complex process that involves both, genetic and environmental factors, notably changes in the gut microbiota that is getting more attention.
The term gut microbiota refers to the complex microbial communities that consist of bacteria, fungi, archea and viruses that inhabit the gastrointestinal tract. The resident gut microbiota and its host maintain a mutually beneficial relationship. A healthy host offers a stable environment and nutrients to the microbiota. In turn, by producing bioactive metabolites, gut microbes contribute to maintain homeostasis and ensure healthiness for the host. At the gut level, microbiota fulfills several functions that include intestinal development as well as immunomodulatory, metabolic and protective functions (Jandhyala et al., 2015; Adak and Khan, 2019). It also influences the physiological functioning of distant organs through several routes including neural, endocrine, immune, and metabolic pathways. This is particularly well documented regarding the gut-brain axis (Dinan and Cryan, 2017; Ma et al., 2019). Although it is relatively stable throughout adult-life, the composition of the gut microbiota changes in elderly (Lozupone et al., 2012; O'Toole and Jeffery,2018). These modifications (gain or loss of community members or changes in their relative abundance), termed dysbiosis, can impact the metabolic activity of the microbiota, with consequences on its mutualistic relationship with the host. Indeed, microbiota dysbiosis is associated with the pathogenesis of age-related diseases, including metabolic diseases, as well as cancers, neurodegenerative and psychiatric disorders (Alvarez-Mercado et al., 2019; Ding et al., 2019).
Lipid homeostasis is crucial for host physiology and health. Indeed, lipids are essential components of biological membranes, they constitute energy sources and, as being signaling molecules, they also take part in the regulation of cell metabolism and homeostasis. Changes in lipid metabolism have been reported during aging and in age-related diseases, and the light has been shed on connections between lipid composition of tissues and longevity in human and model organisms (Rappley et al., 2009; Schroeder and Brunet, 2015; de Diego et al., 2019). The intestinal microbiota plays a critical role in protein, carbohydrate and lipid metabolism. Evidence coming from comparisons between germ-free and conventionally raised rodents indicates that the presence of microbes in the gut has an impact on the lipid composition of metabolically important organs such as adipose tissue and liver, but also lipid-rich organs such as the retina (Backhed et al., 2004, 2007; Oresic et al., 2009; Rabot et al., 2010; Velagapudi et al., 2010). The composition of the gut microbiota also influences lipids in organs. Microbial dysbiosis are associated with metabolic diseases such as obesity and diabetes, and the use of prebiotics or probiotic bacteria was shown to ameliorate metabolic syndrome at least by acting on lipid metabolism (Yang and Kweon, 2016; He and Shi, 2017). Regulation of the lipid metabolism by gut microbes takes place at the gut level but also in distant organs. The microbiota regulates lipid metabolization and absorption at the level of the intestinal mucosa (Martinez-Guryn et al., 2018). In addition to that, gut microbes can modify hepatic de novo lipogenesis by modulating expression of genes involved in lipid metabolism and by generating short chain fatty acids (SCFA) that are substrates for hepatic lipogenesis (Singh et al., 2015; Kindt et al., 2018).
Within human tissues, brain is one displaying the highest content in lipids, and diversity of lipid classes and molecular species (Han, 2007). Modifications of the brain lipid composition have been described in aged healthy and unhealthy brain (Rappley et al., 2009; Naudi et al., 2015). Considering the crucial roles played by gut microbes in the regulation of lipid metabolism and the existence of a gut-brain axis, we sought to evaluate whether changes occurring in the gut microbiota during aging would impact brain lipids. We inoculated germ-free mice with the microbiota of young or old conventionally raised mice. We found that animals colonized with an aged microbiota displayed alterations of lipid profiles in the cortex and the liver. They were characterized by a decrease in the cholesterol and polyunsaturated fatty acids (PUFA) levels, while the monounsaturated fatty acid (MUFA) levels were increased. Moreover, we showed that changes in the gut microbiota that are associated with aging induce the generation of MUFA and has an impact on PUFA elongation and unsaturation, by modifying the expression levels of several genes (Scd1, Fads1, Fads2, Elovl2, and Elovl5) encoding enzymes involved in fatty acid biosynthesis.
Materials and Methods
Animals
Germ-free C3H/HeN males were raised under germ-free conditions in isolators in the germ-free animal facility at the Anaxem platform. The bedding was sterile wood shavings. Mice were given free access to autoclaved tap water and a γ-irradiated (45 kGy) standard diet (R03; Scientific Animal Food and Engineering, Augy, France).
Microbiota Transplantation
At 8 weeks of age, germ-free C3H/HeN mice were moved to two experimental isolators for fecal microbiota transplantation (1 isolator for mice that received the microbiota of young mice (n = 7) and 1 isolator for mice that received the microbiota of old mice (n = 8). Germ-free C3H/HeN mice were inoculated with 200 μL of fresh fecal sample in PBS solution of young or old mice, by oral gavage. The fecal microbiota of young mice was obtained from a pool of feces of three C57Bl/6JRj mice at 4 weeks of age (Janvier Labs, Le Genest-Saint-Isle, France). The fecal microbiota of old mice was obtained from feces of three C57Bl/6JRj mice at 24 months of age (Janvier Labs). Collected feces were weighted and diluted in sterile phosphate buffer saline (100 mg of feces/mL).
Mice were kept in experimental isolators throughout the experiment and were sacrificed 60 days after inoculation.
Fecal Microbiota Analysis by 16S rRNA Gene Sequencing
All the following experiments were performed by Biomnigene (www.biomnigene.fr, Besançon, France). DNA was extracted from about 90 mg of frozen mouse fecal samples using the E.Z.N.A Stool DNA kit (Omega Bio-tek, Norcross, GA, USA) following the manufacturer's instructions. The V3-V4 hyper-variable region of the 16S rRNA gene was amplified by using the following primers: forward primer CCTACGGGNGGCWGCAG and reverse primer GGACTACHVGGGTWTCTAAT, in which bases indexes were incorporated to perform multiplexing. The PCR reactions were performed using 10 ng of fecal DNA, 0.4 μM primers, 200 μM dNTP, and 1X of PrimeSTAR® Max DNA Polymerase (Takara Bio Europe, Saint-Germain-en-Laye, France). Amplifications were carried out using the following program: 1 cycle at 98°C for 5 min, followed by 37 cycles at 98°C for 10 s, 58°C for 15 s, 72°C for 5 s, and finishing with a step at 72°C for 1 min. The PCR products were analyzed on Qiaxcel Cartridge (QIAGEN, Courtaboeuf, France) to verify the amplification. For the preparation of the libraries, the concentration of the PCR products was determined by fluorometric assay on Qubit™ 4.0 and the samples were grouped in an equimolar manner. The pool of PCR products was then purified by electrophoresis on PippinHT using a 1.5% agarose cassette (Sage Sciences, Beverly, MA, USA). The sequencing of V3-V4 amplicons was carried out on MiSeq Illumina in 2X251 bp by using the Illumina MiSeq Reagent Kit v2 (500 cycles; Illumina, San Diego, CA, USA).
16S rDNA Gene Sequences and Statistical Analysis
Sequences were analyzed using the FROGS pipeline to obtain the OTU (Operational Taxonomic Units or phylotypes) abundance table. The successive steps involved de-noising and clustering of the sequences into OTUs using SWARM; chimera removal using VSEARCH; taxonomic affiliation for each OTU using both RDP Classifier and NCBI Blast+ on Silva132 16S. Statistical analyses were performed using “R” language and environment version 3.2.3. β-diversity (UniFrac and weighted UniFrac dissimilarity) measurement and analysis of the differences in OTUs between samples were performed using the add-on package “Phyloseq” (Mcmurdie and Holmes, 2013). Differences in the microbial communities between groups were evaluated using constrained analysis of principal coordinates and permutational multivariate ANOVA. Statistical differences between groups for individual OTU abundance was calculated using the Mann-Whitney test with Benjamini-Hochberg false discovery rate correction. Statistical significance was set at p < 0.05.
Lipid Class Distributions
Total lipids were extracted from plasma, livers and brains following the Folch's procedure (Folch et al., 1957). The distribution of lipids into different classes [phospholipids (PL), triglycerides (TG), diglycerides (DG), free fatty acids (FFA), free cholesterol (Chol), and cholesteryl esters (CE)] was determined using a combination of thin-layer chromatography on silica gel-coated quartz rods and flame ionization detection (Iatroscan® system, Iatron, Tokyo, Japan), according to Ackman's technique (Acar et al., 2009). The values obtained for each compound were corrected according to their response factor using specific calibration curves. Data were reported as a percentage relative to total lipids in the sample (considered as 100%).
Fatty Acid Composition
Total lipids were transmethylated using boron trifluoride in methanol according to Morrison and Smith (Morrison and Smith, 1964). Fatty acid methyl esters (FAMEs) were subsequently extracted with hexane and analyzed on a GC Trace 1310 (Thermo Scientific, Les Ulis, France) gas chromatograph (Palo Alto, CA, USA) using a CPSIL-88 column (100 ×0.25 mm i.d., film thickness 0.20 μm; Varian, Les Ulis, France) equipped with a flame ionization detector. Hydrogen was used as carrier gas (inlet pressure 210 kPa). The oven temperature was held at 60°C for 5 min, increased to 165°C at 15°C/min and held for 1 min, and then to 225°C at 2°C/min and finally held at 225°C for 17 min. The injector and the detector were maintained at 250°C. FAMEs were identified by comparison with commercial and synthetic standards. The data were processed using the ChromQuest software (Thermo Scientific) and reported as a percentage relative to total fatty acids (considered as 100%).
Characterization of Phospholipid Species
The Orbitrap Fusion™ Tribrid mass spectrometer (Thermo Scientific, USA) was used for high-resolution analyses in order to identify phospholipid molecular species in mouse brains. This instrument was equipped with an EASY-MAX NG™ Ion Source (Heated Electrospray Ionization H-ESI) and was controlled by Xcalibur™ 4.1 software. Positive and negative ions were monitored alternatively by switching polarity approach with a spray voltage set to 3,500 V in positive and negative ion modes. The Orbitrap mass analyzer was employed to obtain all mass spectra in full scan mode with the normal mass range and a resolution set value m/z 240,000 amu. A dynamic exclusion filter was applied with an exclusion duration of 15 s and a mass tolerance of 5 ppm. For tandem mass spectrometry (MS/MS) analyses, data-dependent mode was used for the characterization of PL species. Precursor isolation was performed in the Quadrupole analyzer with an isolation window of m/z 1.6 amu. Higher-energy Collisional Dissociation (HCD) was employed for the fragmentation of PL with an optimized collisional energy of 30 eV and a stepped collision energy of 5%. The linear ion trap was used to acquire spectra for fragment ions in data-dependent mode. The Automatic Gain control Target was set to 2.104 with a maximum injection time of 50 ms. The identification of all PL species was performed, using the data of high accuracy and the information collected from fragmentation spectra, with the help of the LipidSearch™ software and the LIPID MAPS® database.
Individual Species of Phospholipid Analysis
High performance liquid chromatography (HPLC) was performed on an Agilent 1,200 equipped with an autosampler, a binary pump and a column oven. During analysis, the column (ZorBAX EclipsePlus C18 −2.1 × 100 mm, 1.8 μm, Agilent Technologies, Les Ulis, France) was maintained at 55°C. The mobile phases consisted of water/methanol (60/40 v/v) 10 mM ammonium acetate, 1 mM acetic acid (A) and of IPA/methanol (90/10) 10 mM ammonium acetate, 1 mM acetic acid (B). The linear gradient was as follows: 40% B for 1 min, up to 95% B in 15 min and maintained at 95 % for 1 min. Column was equilibrated with 40% B for 3 min before each injection. The flow rate was 0.25 mL/min. The autosampler temperature was set at 5°C. One to five microliters of sample were injected onto the HPLC system.
The HPLC system was coupled on-line to a 6,460 triple quadrupole mass spectrometer (MS2) equipped with Jet Stream electrospray ionization source (Agilent Technologies). Source parameters were set-up as follows: temperature set at 325°C, nebulizer gas (nitrogen) pressure 20 psi and flow rate 10 L/min, sheath gas (nitrogen) flow and temperature 11 L/min and 300°C respectively. The capillary and Nozzle voltages were adjusted to 3,500 and 1,000 V, respectively. Quantitation of phosphatidylcholines was achieved in SRM positive mode ([M+1]+ → 184.1; fragmentor 160 V, Collision energy 20 V). Quantitation of phosphatidylethanolamines was achieved in SRM positive mode ([M+1]+ → [M-140]+; fragmentor 136 V, Collision energy 12 V). Quantitation of phosphatidylinositols was achieved in SRM negative mode ([M-1]- → 241 (inositol); fragmentor 150 V, Collision energy 30 V). Relative quantitation of phospholipids was performed according to the area under curve of each species calculated with MassHunter Workstation Software (version B09, Agilent Technologies).
Gene Expression
Total RNA was isolated from livers and brains using TRIzol reagent (Invitrogen, Thermo Fisher Scientific, Illkirch-Graffenstaden, France). cDNA was synthesized using High capacity cDNA Reverse transcription kit (Applied Biosystems, Thermo Fisher Scientific). Real time PCR was performed using C1000 Thermal cycler (Bio-Rad, Marnes-la-Coquette, France) in a reaction mixture of cDNA, iTaq Universal SYBR Green Supermix (Bio-Rad) and mouse-specific oligonucleotides (Supplementary Table 1). Results were normalized to the housekeeping Hprt gene.
Statistical Analyses
Data are presented as the mean ± standard error of the mean (SEM). Statistical analyses were performed with GraphPad Prism software (GraphPad Software Inc., San Diego, CA, USA). The non-parametric Mann and Whitney test was used to compare results between groups. The p-values ≤ 0.05 were considered as statistically significant.
Results
Characterization of the Microbiota Implanted in Germ-Free Mice After Transfer of Fecal Microbiota Coming From Young and Aged Donor Mice
Fecal samples freshly obtained from young or old mice were inoculated into germ-free recipient mice. Sixty days after the transfer, feces were recovered to characterize the microbiota. Based on Unifrac distance metrics, we observed that all the mice that have received microbiota from a same type of donors (young vs. old), clustered together (Figure 1A). This was confirmed with the constrained analysis of the principal coordinates and permutational multivariate ANOVA that showed significant differences between the 2 groups of animals (p < 0.001). Alpha-diversity was analyzed by calculating the Chao1 (estimated community richness) and Shannon's (estimated diversity that takes into account both richness and evenness) index. No significant differences were observed between mice that received microbiota from young donor mice and those that received microbiota from old donor mice (data not shown). However, analysis of relative abundances revealed that the amount of several bacterial genus differs between the two groups of mice in the Bacteroidetes, Firmicutes and Proteobacteria phyla. The proportions of Ruminococcaceae, Acetatifactor, Lachnospira, Lachnospiraceae, Peptococcus, and Escherichia-Shigella were significantly increased and those of Eubacterium ventriosum, Eubacterium xynalophilum, Christensenellaceae, Rikenellaceae, and Candidatus arthromitus were significantly decreased in the fecal microbiota of mice inoculated with the aged microbiota compared to those that received the young microbiota (Figure 1B). These results indicate that, 60 days after the inoculation, the composition of the microbiota in mice transplanted with the microbiota of old donors is distinct from that of mice that received a young microbiota.
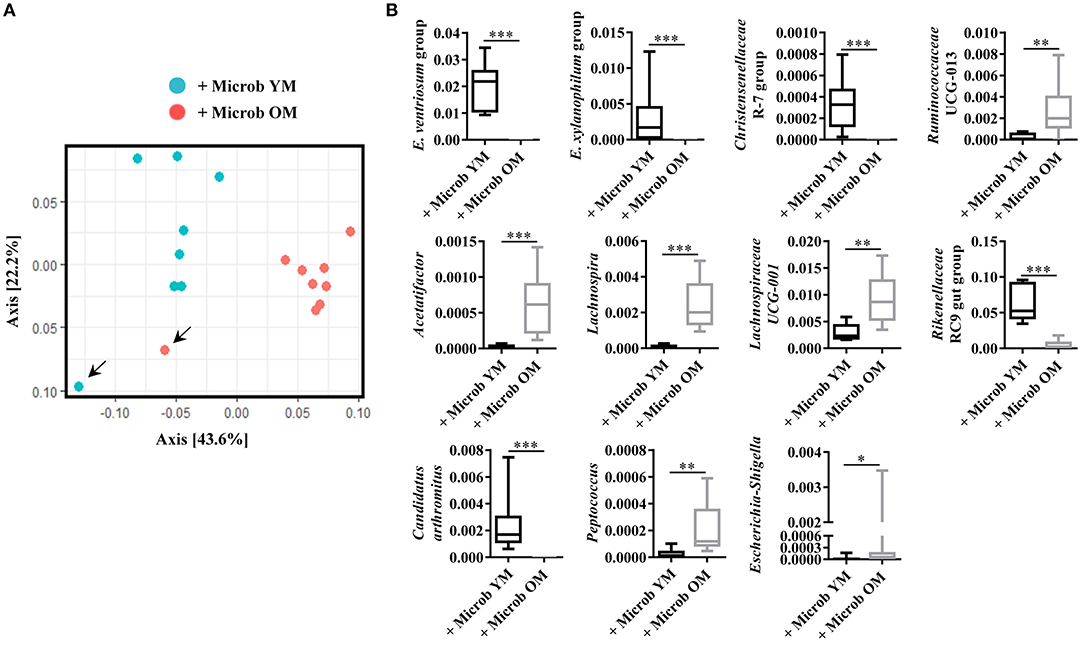
Figure 1. Microbiota analysis in recipient germ-free mice. (A) Composition of the fecal microbiota analyzed by Unifrac distance. Each dot represents one mouse. Blue dots represent fecal microbiota from germ-free mice (n = 7) inoculated with feces of conventionally raised young mice (+ Microb YM) and red dots represent fecal microbiota of germ-free mice (n = 8) inoculated with feces from conventionally raised old mice (+ Microb OM). Arrows indicate donors. (B) Relative abundance in percentage of genus significantly modified in fecal microbiota of mice inoculated with feces from young mice and of mice inoculated with feces from old mice. E. ventriosum group: [Eubacterium] ventriosum group, and E. xylanophilum group: [Eubacterium] xylanophilum group. Comparisons were done between the two groups of mice (+ Microb YM and + Microb OM). *p < 0.05, **p < 0.01 and ***p < 0.001.
Gut Microbiota Has an Impact on the Cortex Content in Cholesterol and Phospholipids
In order to investigate whether changes in the gut microbiota modulate the lipid content of the cortex, we performed several series of lipid analyses. Within tissue lipid classes, the amount of triglycerides (TG) was unchanged by the microbiota (Figure 2A). However, a significant increase in the amount of total phospholipids and a significant decrease in cholesterol were observed in mice transplanted with an aged microbiota compared to those inoculated with a young microbiota (2.7 ± 0.3% and 2.3 ± 0.3%, respectively) (Figure 2A). As cholesterol in the adult brain is primarily supplied by de novo synthesis, we analyzed the expression levels of the Hmgcs1 gene, which encodes the hydroxymethylglutaryl-CoA synthase (HMG-CoA synthase), the enzyme catalyzing the reaction in which acetyl-CoA condenses with acetoacetyl-CoA to form HMG-CoA, and Hmgcr gene, which encodes the HMG-CoA reductase, the enzyme responsible for converting HMG-CoA to mevalonate, a rate-limiting step in cholesterol synthesis (Zhang and Liu, 2015). No significant change in the mRNA levels of Hmgcr or Hmgcs1 was observed (Figures 4A,B). These data suggest that changes in the gut microbiota composition that are associated with aging modify cholesterol content of the cortex by a mechanism other than the one involving the HMGCR and HMGCS enzymes.
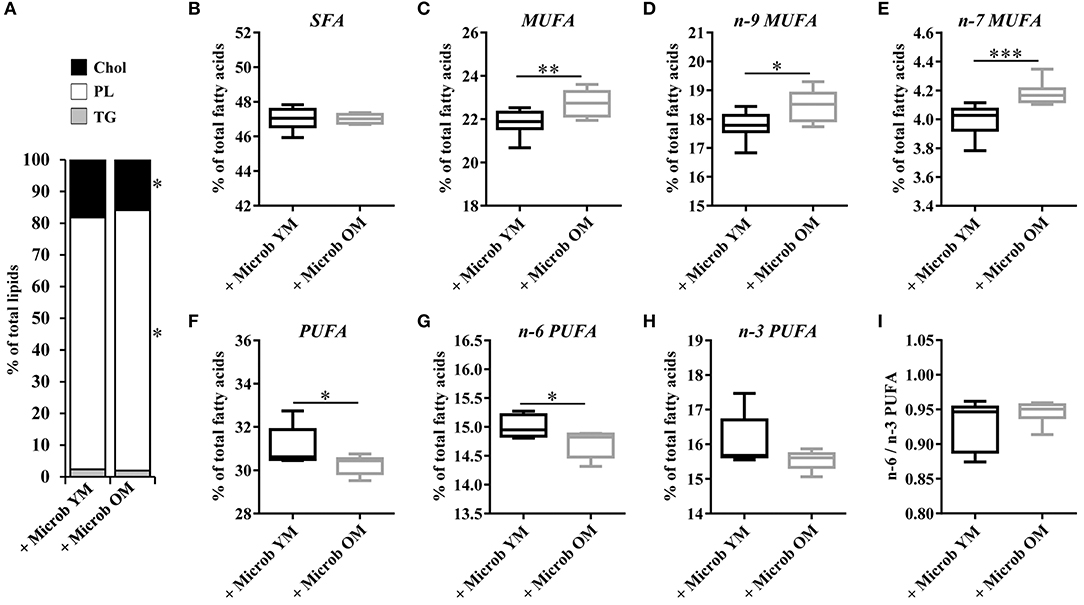
Figure 2. Relative abundance of lipid classes and ester-linked fatty acids in the cortex of mice harboring different age-related microbiota. Lipids were extracted from the cortex of mice inoculated with fecal microbiota of young mice (+ Microb YM) or old mice (+ Microb OM). (A) Relative abundance of lipid classes. Results are expressed as the percentages of cholesterol (Chol), phospholipids (PL), and triglycerides (TG) relative to total lipids, defined as 100%. (B–H) Relative abundance of ester-linked fatty acids. The results correspond to the quantification of fatty acid methyl esters derivatives (FAME) by GC-FID. They are expressed as the percentages of total (B) saturated fatty acids (SFA), (C) monounsaturated fatty acids (MUFA), (D) omega-9 (n-9) MUFA, (E) omega-7 (n-7) MUFA, (F) polyunsaturated fatty acids (PUFA), (G) omega-6 (n-6) PUFA and (H) omega-3 (n-3) PUFA relative to total fatty acids, defined as 100%. (I) Ratio of n-6/n-3 PUFA. (B–I) All data are presented as mean ± SEM. n = 7 for the group of mice inoculated with the microbiota of young mice and n = 8 for the group of mice inoculated with the microbiota of old mice. Comparisons were done between the two groups of mice (+ Microb YM and + Microb OM). *p < 0.05, **p < 0.01 and ***p < 0.001.
Fatty Acid Composition of the Cortex Is Modulated by the Gut Microbiota
Effect of age-related microbiota on the fatty acid composition of the cortex was investigated by GC-FID analyses. The amount of each fatty acid was determined relative to total fatty acids. Regardless of the inoculated microbiota origin, no changes were observed in the abundance of saturated fatty acids (SFA) (Figures 2B, 3 and Supplementary Table 2). However, a significant increase in the amount of total monounsaturated fatty acids (MUFA) was measured in the cortex of mice inoculated with the aged microbiota compared to those of mice with the young microbiota (22.7 ± 0.2% vs. 21.8 ± 0.2% of total fatty acids, respectively) (Figure 2C). Interestingly, two series of MUFA, namely the omega-7 (n-7) and omega-9 (n-9) series, were significantly altered by the age-related changes in gut microbiota (Figures 2D,E, 3 and Supplementary Table 2). Among the 8 most quantitatively represented fatty acids that we detected in the mouse cortex, 3 of them were MUFA (C18:1n-9, C18:1n-7, and C20:1n-9) and their abundances were significantly increased in mice inoculated with the aged microbiota compared to those transplanted with the young one (Figure 3B).
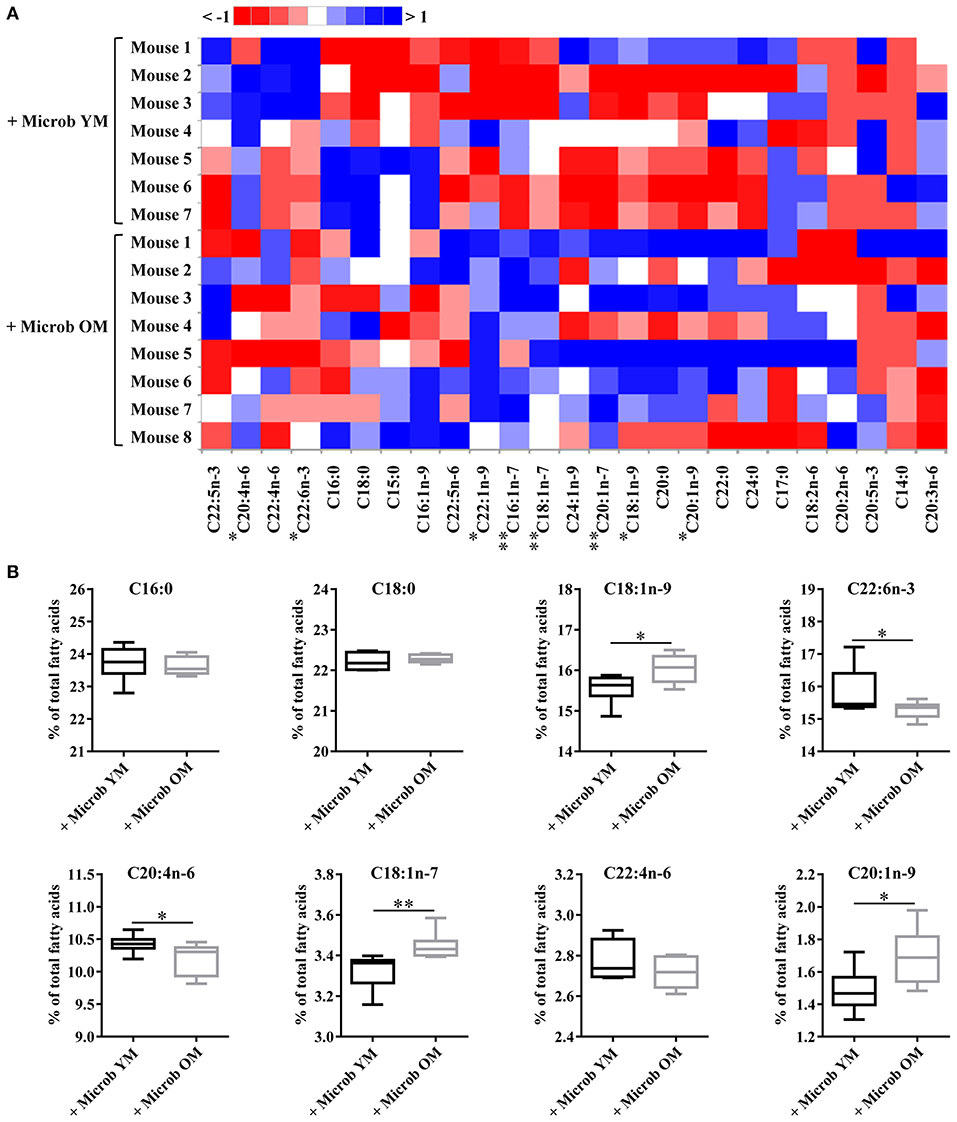
Figure 3. Ester-linked fatty acid profile in the cortex of mice inoculated with different age-related microbiota. Lipids were extracted from the cortex of mice inoculated with fecal microbiota of young mice (+ Microb YM) or old mice (+ Microb OM). The results correspond to quantification of fatty acid methyl esters (FAME) derivatives by GC-FID. The percentage of each fatty acid relative to that of total fatty acids (100%) was determined. (A) Heat map showing the abundance of each fatty acid relative to total fatty acids, defined as 100% (B) Relative abundance of the eight most abundant fatty acids quantified in the brain. All data are presented as mean ± SEM. n = 7 for the group of mice inoculated with the microbiota of young mice and n = 8 for the group of mice inoculated with the microbiota of old mice. Comparisons were done between the two groups of mice (+ Microb YM and + Microb OM). *p < 0.05 and **p < 0.01.
Our data also revealed that microbiota modulates the amount of tissue polyunsaturated fatty acids (PUFA) (Supplementary Table 2). A significantly reduced amount of total PUFA was observed in mice inoculated with the microbiota of old mice compared to those transplanted with young microbiota (30.2 ± 0.2% and 31.2 ± 0.3%, respectively) (Figure 2F). Among them, both n-6 and n-3 series were impacted by the microbiota, but only total n-6 were significantly altered (Figures 2G,H). Particularly, the levels of the most represented PUFA in the brain, arachidonic acid (C20:4n-6 or AA) and docosahexaenoic acid (C22:6n-3 or DHA), were significantly reduced in mice transplanted with an aged microbiota (Figure 3B). The ratio of omega-6 PUFA to omega-3 PUFA remained unchanged (Figure 2I).
With the exception of the essential fatty acids, the linoleic acid (C18:2n-6) and alpha-linolenic acid (C18:3n-3) that are only provided through diet, brain fatty acids can be synthesized by de-novo lipogenesis in brain cells (Tracey et al., 2018). Therefore, we investigated whether the modifications in the content of cortex MUFA and PUFA were the result of dysregulations in the expression of enzymes involved in fatty acid biosynthesis in this tissue. We found that the expression levels of enzymes involved in MUFA biosynthesis (Scd1) and PUFA unsaturation (Fads1 and Fads2) and elongation (Elovl2 and Elovl5) were not impacted by the composition of the gut microbiota (Figure 4).
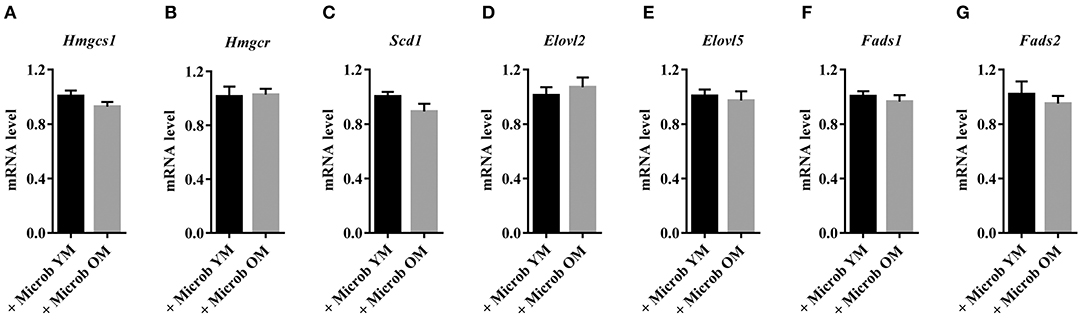
Figure 4. Cortical expression of enzymes involved in cholesterol synthesis in mice harboring age-dependent microbiota. (A,B) Cortical expression of genes encoding enzymes involved in the biosynthesis of cholesterol: (A) hydroxymethylglutaryl-CoA synthase (Hmgcs1) and (B) 3-hydroxy-3-methylglutaryl-CoA reductase (Hmgcr). (C–G) Cortical expression of genes encoding enzymes involved in the biosynthesis of fatty acids: (C) acyl-CoA desaturase 1 (Scd1), (D,E) elongation of very long chain fatty acids proteins 2 and 5 (Elovl2 and Elovl5), (F) acyl-CoA (8-3)-desaturase (Fads1) and (G) acyl-CoA 6-desaturase (Fads2). The levels of mRNA were normalized to Hprt mRNA level for calculation of the relative levels of transcripts. mRNA levels are illustrated as fold change. All data are presented as mean ± SEM. n = 7 for the group of mice inoculated with the microbiota of young mice and n = 8 for the group of mice inoculated with the microbiota of old mice.
Altogether, these data show that age-related changes in gut microbiota promote profound modifications of the fatty acid profile in the cortex.
Identification of Lipid Species Modified in the Cortex by Age-Associated Changes in the Gut Microbiota
Because cortex fatty acids are almost exclusively esterified on phospholipids, and since phospholipid subclasses play differential roles in brain metabolism (Farooqui et al., 2000; Tracey et al., 2018), we wanted to find out which phospholipids were involved in the changes we observed in the fatty acid composition of the cortex. HPLC-MS2 analyses revealed the presence of 41 species of phosphatidyl and plasmenylcholine (PC and PlsC), 80 species of phosphatidyl, and plasmenylethanolamine (PE and PlsE), 14 species of phosphatidyl and plasmenylinositol (PI and PlsI) and 15 species of sphingolipids, which were all sphingomyelins (SM) in the cortex (Supplementary Tables 3, 4). We compared, for each phospholipid class, the relative abundance of lipid species based on the fatty acid (SFA, MUFA, PUFA, C20:4, or C22:6) esterified at the sn-2 position (Table 1). Although the cortex content in total SFA was not modified by age-associated changes in the gut microbiota composition, we observed several alterations in the distribution of SFA among phospholipid classes and at the species level. On one hand, mice inoculated with the microbiota of old mice had a very slight but significant increase in the amount of PlsE with SFA at the sn-2 position compared to those inoculated with the microbiota of young mice (0.80 ± 0.01% and 0.76 ± 0.01%, respectively) (Table 1). These changes affected the following PlsE species: PlsE (18:1p/18:0), PlsE (16:0p/20:0), PlsE (18:1p/20:0) and PlsE (18:0p/20:0) (Supplementary Table 3). On the other hand, SM with SFA were significantly decreased by 1.2 ± 0.4% in the cortex of mice inoculated with an aged microbiota compared to those inoculated with a young microbiota (Table 1). These microbiota-dependent modifications affected the SM (d18:1/17:0) and SM (d18:1/18:0) species, the latter representing around 50% of the total SM species (Supplementary Table 4).
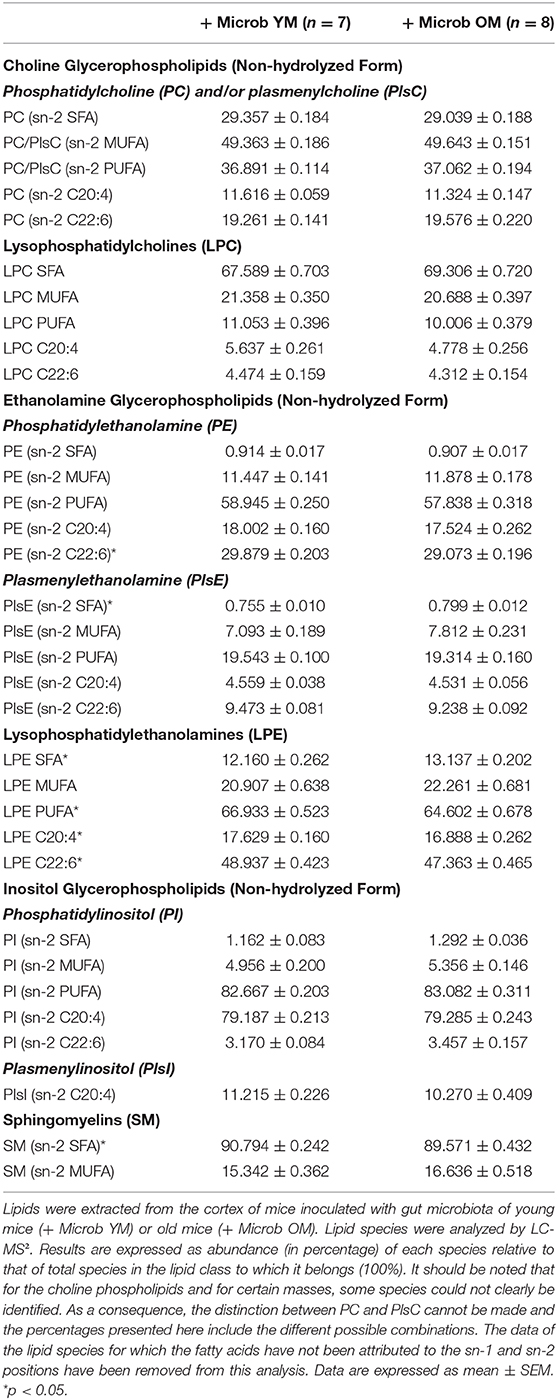
Table 1. Relative amounts of lipid species in the cortex depending on the lipid class and the family of fatty acids esterified at the sn-2 position.
In addition to that, we observed that the amount of PE with C22:6 was significantly decreased by 0.8% ± 0.2% in the cortex of mice inoculated with the microbiota of old mice compared to those inoculated with the microbiota of young mice (Table 1). These changes were highlighted particularly by alterations in the PE (20:0/22:6) and PE (18:0/22:6) species, the latter representing about 20% of the total PE and PlsE species and 50% of the PE and PlsE species esterified to C22:6n-3 (Supplementary Table 3).
Among PC/PlsC and PI/PlsI classes, comparison of lipid species abundance based on the fatty acid (SFA, MUFA, PUFA, C20:4, or C22:6) esterified at the sn-2 position showed no significant difference between the two groups of mice (Table 1). However, at the level of lipid species we observed a slight but significant decrease of 0.4 ± 0.2% in the amount of PC (16:0/18:1). This species has a MUFA esterified at the sn-2 position and represents 22.5 ± 0.1% of the PC and PlsC in the cortex of mice inoculated with an aged microbiota (Supplementary Table 3). The amounts of 5 others PC species were significantly modified between the two groups of mice but, altogether, they represent <0.1% of the PC and PlsC (Supplementary Table 3).
Lysophospholipids are phospholipids in which one of the fatty acyl chains has been removed and replaced with a hydroxyl group. They can reflect a degradation of phospholipids in tissues. Eight species of lysophosphatidylcholines (LPC) and 5 species of lysophosphatidylethanolamines (LPE) were detected (Supplementary Table 3). Comparison of the abundance of lysophospholipid individual species revealed several changes but only among LPE series (Table 1). A significant increase in the amount of LPE esterified to SFA and a significant decrease in LPE with PUFA (including LPE C20:4 and LPE C22:6) were observed in the brain of mice harboring the microbiota of old mice compared to those with the microbiota of young mice (Table 1 and Supplementary Table 3).
These data show that changes in the gut microbiota composition associated with aging has an impact on the relative abundance of cortex individual phospholipid species, and particularly on PC/PlsC, PE/PlsE, LPE, and SM.
Plasma Lipid Composition Is Altered in Mice Inoculated With the Microbiota of Old Mice
Fatty acids are taken up into the brain from circulating blood by mechanisms that still remain under debate (Katz et al., 2007; Bruce et al., 2017). Our data showed that the relative amounts of plasma cholesteryl ester, cholesterol, triglycerides, and phospholipids were not modified by changes in the gut microbiota (Figure 5A). However, mice inoculated with microbiota of old mice exhibited significantly increased amounts of plasma diglycerides (0.9 ± 0.3% vs. 0.0 ± 0.0%) and free fatty acids (1.3 ± 0.2% vs. 0.5% ± 0.2%) compared to mice inoculated with the microbiota of young mice, suggesting a partial hydrolysis of blood triglycerides (Figure 5A). These results suggest that the plasma lipid composition is modified by an aged microbiota.
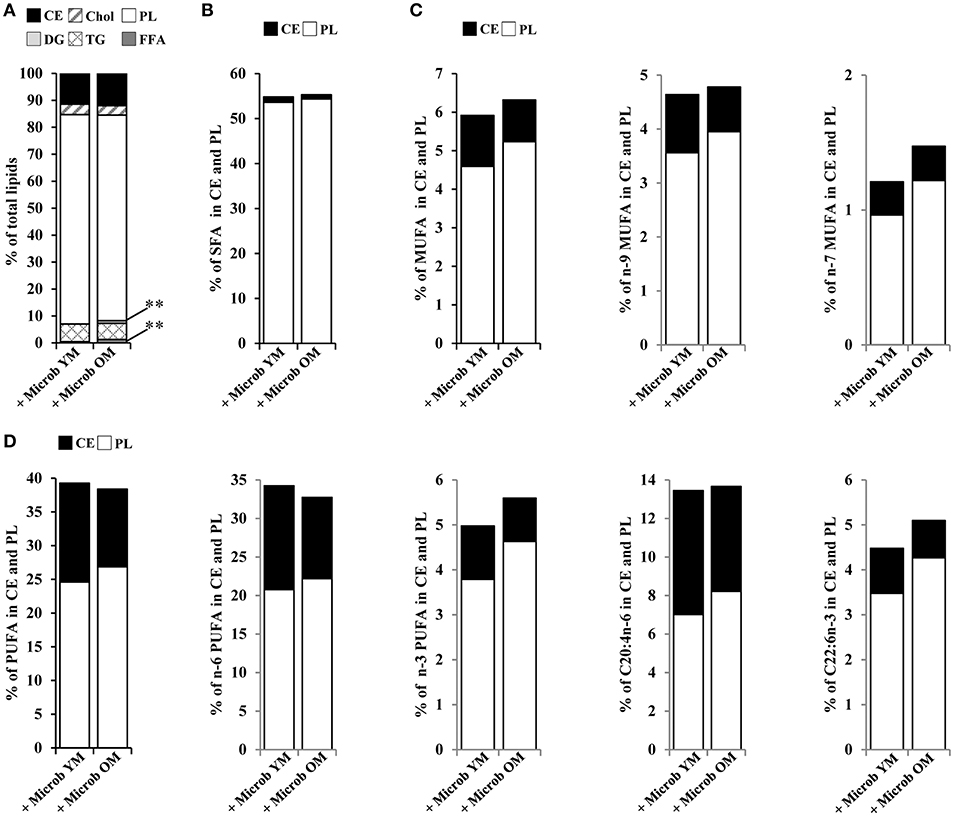
Figure 5. Plasma lipid and fatty acid profile in mice inoculated with different age-related microbiota. Lipids were extracted from the plasma of mice inoculated with fecal microbiota of young mice (+ Microb YM) or old mice (+ Microb OM). (A) Relative abundance of lipid classes. Results are expressed as the percentages of cholesteryl esters (CE), cholesterol (Chol), phospholipids (PL), diglycerides (DG), triglycerides (TG), and free fatty acids (FFA) relative to total lipids, defined as 100%. (B–D) Total lipid extract was separated on silica gel. CE and PL were extracted and their fatty acid profiles were determined by GC-FID. To express the results, we took into account the relative proportions of CE and PL measured in the total lipid extracts. Results are expressed as percentages of (B) total saturated fatty acids (SFA), (C) monounsaturated fatty acids (MUFA), and (D) polyunsaturated fatty acids (PUFA) on CE and PL in total lipid extracts, defined as 100%. All data are presented as mean. n = 7 for the group of mice inoculated with the microbiota of young mice and n = 7 for the group of mice inoculated with the microbiota of old mice. **p < 0.01.
By GC-FID, we evaluated more specifically the variations in the distribution of fatty acids on cholesteryl esters and phospholipids (Figures 5B–D). No significant difference in the amounts of fatty acid series or DHA and AA was observed between the groups of mice that have received different age-related microbiota. However, not being significant and limited to cholesteryl esters and phospholipids, these data do not allow us to conclude on an effect due to changes in the composition of the intestinal microbiota on the fatty acid content of the plasma.
Age-Related Changes of Gut Microbiota Modulate Hepatic Lipogenesis
We investigated whether alterations in the cortex lipid composition occurring in mice that received the microbiota of old donor mice could be the consequence of an altered hepatic lipid metabolism. Our data revealed profound modifications of the liver lipid composition in mice inoculated with the microbiota of old mice comparatively to those inoculated with the microbiota of young mice (Figure 6A). These changes were characterized by significant decreases in the amounts of cholesterol (3.2 ± 0.2% vs. 4.5 ± 0.3%) and phospholipids (62.2 ± 2.4% vs. 77.0 ± 2.9%), balanced by a significant increase in the amount of triglycerides (30.7 ± 2.7% vs. 15.0 ± 3.0%). The analysis of the hepatic fatty acid composition by GC-FID also showed remarkable microbiota-dependent changes (Figure 6). The amount of SFA was significantly reduced (by 4.0 ± 0.3%) and that of MUFA was significantly increased (by 6.0 ± 1.5%) in mice with a microbiota of old mice compared to those with a young microbiota (Figures 6B–D). Regarding PUFA, the amount of n-6 fatty acids was unchanged whereas that of the n-3 fatty acids was significantly decreased in mice with an aged microbiota (10.6 ± 0.3% vs. 11.8 ± 0.3%), resulting in a significant increase in the n-6 PUFA to n-3 PUFA ratio (Figures 6E–G).
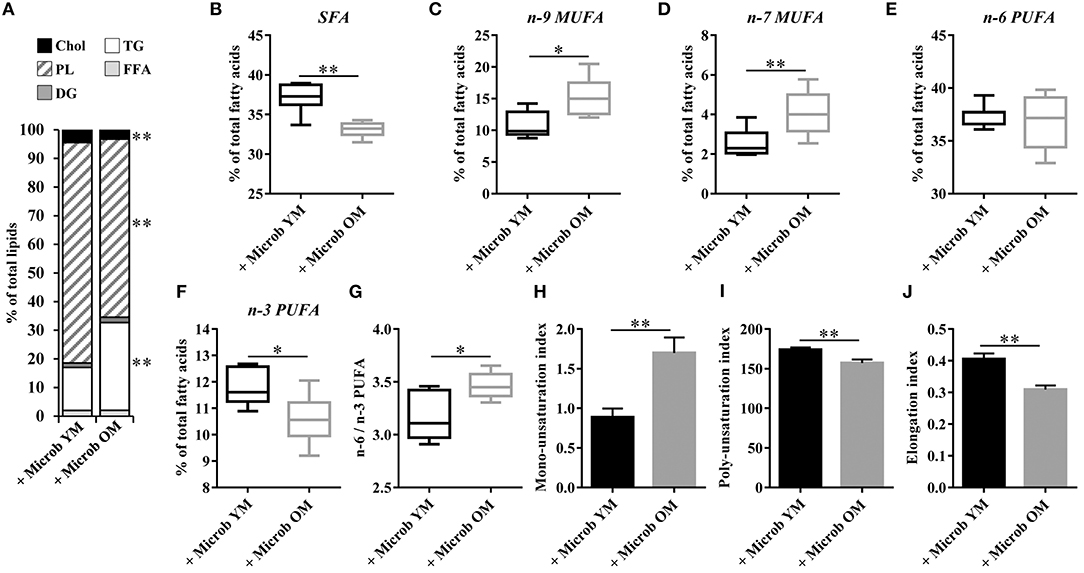
Figure 6. Relative abundance of lipid classes and ester-linked fatty acids in the liver of mice inoculated with different age-related microbiota. Lipids were extracted from the liver of mice inoculated with fecal microbiota of young mice (+ Microb YM) or old mice (+ Microb OM). (A) Relative abundance of lipid classes. Results are expressed as the percentages of cholesterol (Chol), phospholipids (PL), diglycerides (DG), triglycerides (TG), and free fatty acids (FFA) relative to total lipids, defined as 100%. (B–J) The results presented were obtained from the analysis of the quantification data of fatty acid methyl esters (FAME) derivatives by GC-FID. Results are expressed as the percentages of total (B) saturated fatty acids (SFA), (C) omega-9 (n-9) monounsaturated fatty acids (MUFA), (D) omega-7 (n-7) MUFA, (E) omega-6 (n-6) polyunsaturated fatty acids (PUFA), and (F) omega-3 (n-3) PUFA relative to total fatty acids, defined as 100%. (G) Ratio of n-6/n-3 PUFA. (H) Mono-unsaturation index corresponding to the sum of the ratios “products” (C16:1n-7 and C16:1n-9)/“precursor” (C16) and “products” (C18:1n-7 and C18:1n-9)/“precursor” (C18). (I) Poly-unsaturation index corresponding to the sum of the amounts of each fatty acid that had more than on carbon-carbon double bond multiplied by its unsaturated bond number. (J) Elongation index corresponding to the ratio of fatty acids with 20-carbons and more/fatty acids with 16- and 18-carbons. All data are presented as mean ± SEM. n = 7 for the group of mice inoculated with the microbiota of young mice and n = 8 for the group of mice inoculated with the microbiota of old mice. Comparisons were done between the two groups of mice (+ Microb YM and + Microb OM). *p < 0.05 and **p < 0.01.
We next investigated whether changes in the hepatic lipid content observed in mice inoculated with the microbiota of old donors could be due to an altered expression of genes involved in lipogenesis. Hepatic expression of genes encoding enzymes involved in the biosynthesis of cholesterol (Acat1, Acat2, Hmgcs1, and Hmgcr), di- and triglycerides (Plpp1, Plpp2, Plpp3, and Dgat2) and fatty acids (Fasn, Scd1, Fads1, Fads2, Elovl2, and Elovl5), and in the regulation of lipid metabolism (Srebp1c and Srebp2) was measured (Figure 7). No significant modification of the transcript levels of genes involved in cholesterol, di- and triglycerides biosynthesis and in the regulation of hepatic lipid metabolism was observed (Figures 7A,B,D). However, we observed significant decreases in the expression of Fads1 and Fads2 (by 28.8 ± 5.8% and 16.3 ± 5.5%, respectively) in mice that have received the microbiota of old mice compared to those inoculated with the microbiota of young mice (Figure 7C). This result was in accordance with the significant decrease in the poly-unsaturation index observed in mice inoculated with an aged microbiota (Figure 6I).
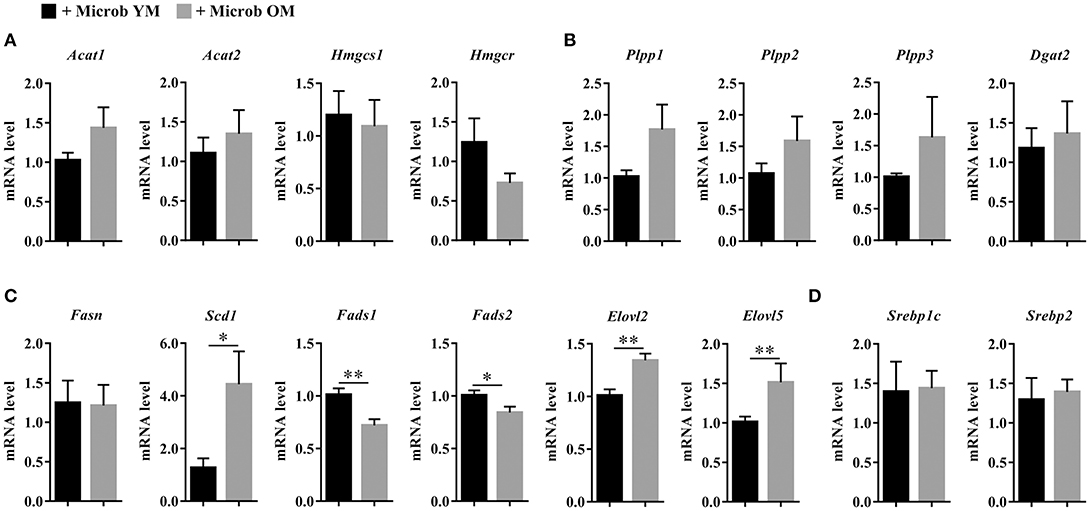
Figure 7. Expression of hepatic genes involved in lipid biosynthesis. (A) Hepatic expression of genes encoding enzymes involved in the biosynthesis of cholesterol: acetyl-CoA acetyltransferase 1 and 2 (Acat1 and Acat2), hydroxymethylglutaryl-CoA synthase (Hmgcs1) and 3-hydroxy-3-methylglutaryl-CoA reductase (Hmgcr). (B) Hepatic expression of genes encoding enzymes involved in the biosynthesis of di- and triglycerides: phospholipid phosphatases 1, 2, and 3 (Plpp1, Plpp2 and Plpp3) and diacylglycerol O-acyltransferase 2 (Dgat2). (C) Hepatic expression of genes encoding enzymes involved in the biosynthesis of fatty acids: fatty acid synthase (Fasn), acyl-CoA desaturase 1 (Scd1), acyl-CoA (8-3)-desaturase (Fads1), acyl-CoA 6-desaturase (Fads2), and elongation of very long chain fatty acids proteins 2 and 5 (Elovl2 and Elovl5). (D) Hepatic expression of genes encoding enzymes involved in the regulation of lipid synthesis: sterol regulatory element-binding proteins 1 (variant 3) and 2 (Srebp1c and Srebp2). The levels of mRNA were normalized to Hprt mRNA level for calculation of the relative levels of transcripts. mRNA levels are illustrated as fold change. All data are presented as mean ± SEM. n = 7 for the group of mice inoculated with the microbiota of young mice and n = 8 for the group of mice inoculated with the microbiota of old mice. Comparisons were done between the two groups of mice (+ Microb YM and + Microb OM). *p < 0.05 and **p < 0.01.
In addition, the level of Scd1 mRNA was significantly increased by 3.5 in mice harboring an aged microbiota compared to those with a young microbiota (Figure 7C). SCD1 (stearoyl-CoA desaturase 1) is the rate limiting enzyme that catalyzes the biosynthesis of MUFA. In accordance with Scd1 mRNA level, we observed an increase in the mono-unsaturation index in mice inoculated with the microbiota of old mice compared to those inoculated with the microbiota of young mice (Figure 6H). The level of Elovl2 and Elovl5 mRNA were also significantly increased by 1.3 and 1.5, respectively, in mice harboring an aged microbiota compared to those with a young microbiota (Figure 7C). ELOVL2 (elongation of very long chain fatty acids protein 2) and ELOVL5 catalyze the reactions of the long-chain fatty acids elongation cycle. However, these results were unexpected since we observed a significant decrease in the hepatic elongation index in mice inoculated with the microbiota of old mice suggesting a decreased amount of long-chain fatty acids (Figure 6J).
Taken together, these results show that age-associated changes in microbiota promote a reprograming of genes related to MUFA biosynthesis and PUFA elongation and desaturation in the liver.
Discussion
In humans, modifications in the gut microbiota during aging have been reported and a large number of studies suggest that these changes could be a major factor that can tip the balance between healthy and non-healthy aging. Since aging is associated with dysregulations in lipid metabolism, we investigated whether changes in the gut microbiota that are associated with aging can alter host lipid homeostasis.
Aging is a complex process affecting host physiological, genomic, metabolic, and immunological functions (Lopez-Otin et al., 2013). The dialogue between host and microbiota is bi-directional and alterations of the host can also shape the gut microbiota (Goodrich et al., 2014; Davenport, 2016; Kolde et al., 2018). In addition, in humans a large number of environmental factors such as the place of residence (hospital, residential care setting or home) or the diet can influence the composition of the gut microbiota. For that matter, multiple factors should be taken into account when studying the relationship of the gut microbiota with the host (O'Toole and Jeffery, 2015; Garcia-Pena et al., 2017). The germ-free mouse model offers a good opportunity to study the impact of gut microbes on host physiology by overcoming these variables. In order to study the impact of age-related changes of microbiota on lipid homeostasis, we transferred the fecal microbiota of young vs. old donor mice into germ-free mice. The composition of the fecal microbiota recovered in mice after 2 months of colonization was analyzed by 16S rRNA gene sequencing, a powerful tool widely used to explore the composition of bacterial communities (Rapin et al., 2017). We showed that microbiota were different between the two groups of mice, with that of mice inoculated with fecal microbiota of old donors recapitulating some features described in the elderly. This includes an increase in the relative abundance of Escherichia-Shigella, a bacterial genus associated with inflammation, and a decrease in Christensenellaceae, a bacterial family associated with longevity (Biagi et al., 2016; Kumar et al., 2016). However, it should be noticed that the 16S rRNA sequencing method has some limitations and gives little information about the whole genetic, functional, and metabolic capabilities of the microbiota (Rapin et al., 2017).
The brain tissue is characterized by its high content in lipids. In fact, lipids constitute approximately half of the brain dry weight and mainly consist of phospholipids, cholesterol and sphingolipids. Because of their high diversity and due to their hydrophobic properties, lipids play crucial roles in maintaining the structure and the functions of the brain. Alteration in the brain lipid metabolism is associated with many neurological disorders (Adibhatla and Hatcher, 2007). Cholesterol is essential for neuronal physiology (Zhang and Liu, 2015). During aging, its amount decreases in the cortex, and defects in cholesterol metabolism were reported to be associated with neurodegenerative diseases and cognitive deficits related to aging (Soderberg et al., 1990; Svennerholm et al., 1994; Zhang and Liu, 2015). Interestingly, our results reproduced this cholesterol alteration in the cortex of mice with the microbiota of old mice in comparison with the mice inoculated with the microbiota of young donor mice. As we have also observed a decrease in hepatic cholesterol amount, one can hypothesize that the decrease in brain cholesterol could be a consequence of decreased cholesterol availability in the bloodstream. But, such a hypothesis does not seem very plausible since, due to the blood brain barrier brain cholesterol is primarily produced through de novo synthesis (Dietschy and Turley, 2004). Another hypothesis could have been an alteration in the cholesterol biosynthesis pathway. However, we did not observe any modification in the expression of the rate-limiting enzyme in cholesterol synthesis HMGCR in the cortex of mice inoculated with the microbiota of old mice compared to those inoculated with the microbiota of young mice. To explain the decrease in cholesterol in mice with an aged microbiota we finally hypothesize that expression of cholesterol-removing enzymes could be enhanced (Martin et al., 2010; Nes, 2011). This hypothesis is supported by studies showing increased amount of cholesterol-24-hydroxylase enzyme (CYP46A1)—the major enzyme involved in cholesterol removal in neuronal tissues—in brains of old humans and rodents compared to young ones (Lund et al., 1999; Martin et al., 2008; Perovic et al., 2009).
Brain contains high amounts of phospholipids (glycerophospholipids and sphingomyelins) (O'Brien and Sampson, 1965; Soderberg et al., 1990). Glycerophospholipids are enriched in PUFA, particularly in docosahexaenoic acid (DHA, C22:6n-3) and arachidonic acid (AA, C20:4n-6) whereas sphingomyelins are composed of fatty acids with low levels of unsaturation. These lipids are structurally and functionally important cell constituents. Besides providing structural integrity to neuronal cell membranes for the proper functioning of membrane-bound enzymes or transport systems, they play many others important functions such as being reservoirs of signaling messengers and antioxidant molecules (Farooqui et al., 2000; Tracey et al., 2018). Any changes in the phospholipid content, whether in the distribution of different sub-classes or in their structure, could then have profound consequences on cell function. In this study, we showed that intestinal colonization by a microbiota from old donor mice compared to that of young donor mice profoundly modifies the structure of brain phospholipids by changing their fatty acid composition. Among the changes observed, there was an increased amount of MUFA belonging to the n-7 and n-9 series, mainly C18:1n-9, C18:1n-7, and C20:1n-9, which are part of the 8 most represented fatty acids in the brain. These changes were balanced by a decreased brain content in PUFA, and particularly in C20:4n-6 (arachidonic acid, AA) and C22:6n-3 (docosahexaenoic acid, DHA), the two major PUFAs in neurons. Interestingly, similar age-associated changes in fatty acid composition have already been reported in rat and human cortical tissues (Bowen et al., 1973; Favrelere et al., 2000; Giusto et al., 2002; Little et al., 2007; McNamara et al., 2008).
Ethanolamine and choline glycerophospholipids (PE and PC) are the two most represented classes of phospholipids in the brain, notably in cortical tissue (Sastry, 1985; Little et al., 2007). Our lipidomic data suggest that an aged microbiota is associated with changes in MUFA content in PE and PUFA content in PE and LPE. Increased amounts of PE esterified with C18:1n-9 and C20:1n-9 and/or decreased amount of PE esterified with C20:4n-6 or C22:6n-3 have previously been reported in the cortex of aged rats and macaques (Favrelere et al., 2000; Little et al., 2007; Latour et al., 2013; Lam et al., 2016). However, other authors did not observe any age-related modification of these PE species in old rat cortex (Letondor et al., 2014). Interestingly, and as already reported by Little et al. we show in our study a decrease in PE (18:0/22:6), which is the most represented species (about 20%) among all ethanolamine phospholipids (Little et al., 2007). Modifications among sphingomyelin species have also occurred in the cortex of mice harboring the microbiota of old donors compared to those that have received the microbiota of young donors. They affected two of the three major species of SM. The amount of SM (d18:1/18:0) that represents about half of the total SM species was decreased and this was balanced by SM (d18:1/24:1) that represents about 8% of the SM species and whose amount was increased in mice with an aged microbiota compared to those with a young one. Increase in SM (C24:1) has already been described in the cortex of old macaques and rats (Giusto et al., 1992; Lam et al., 2016).
The mechanisms of fatty acid uptake by the brain are not yet fully understood (Qi et al., 2002; Katz et al., 2007). Some specificities have been documented depending on the fatty acid and this explains, in part, the unique lipid profile of the brain, characterized by an enrichment in PUFA, and particularly in DHA and AA (Gazzah et al., 1994; Contreras et al., 2000; Rosenberger et al., 2010; Chen et al., 2013). Uptake and transport of fatty acids will also depend on their form (non-esterified or esterified), and on the lipid entities on which they are esterified. Identifying the fatty acids esterified on cholesterol and phospholipids in plasma did not allow us to clearly establish a link between circulating fatty acids and the brain fatty acid profile in mice harboring age-dependent microbiota. In this study, we have observed non-significant variations in the fatty acid composition of plasma CE and PL. Plasma total CE and PL originate from low-density lipoproteins (LDL) and high-density lipoproteins (HDL) making that the analysis of fatty acid composition of whole plasma CEs and whole plasma PLs considers LDL and HDL lipoproteins together. The variability and the non-significant tendencies we observed might then be the result of variations of the fatty acid composition in only one of these lipoprotein classes. One can hypothesize that targeting lipids of isolated LDL and/or HDL lipoproteins is likely to underline differences in the fatty acid composition between animals receiving an aged microbiota vs. a young microbiota, and then reinforce the hypothesis of defective liver biosynthesis and consequences on nervous tissues through blood transport. Further studies are needed to verify this hypothesis. In addition, we have observed an increase in the amount of FFA and DG in plasma of mice colonized by an aged microbiota. Increase of plasma FFA level have already been reported in metabolic and immune-related diseases and some data support a link between microbial dysbiosis and altered serum FFA levels (Boden, 2008; Rodriguez-Carrio et al., 2017). Beyond the influence that they could have on the fatty acid composition of the brain, attention should be paid to plasma FFA since they are likely to be degradation products of TG and could constitute systemic mediators modulating inflammation and metabolism, two conditions related to aging (Pararasa et al., 2015; Suiter et al., 2018).
Our data show that changes in microbiota influence the relative proportion of lipid classes in the liver. Indeed, mice that received the microbiota of old donors displayed elevated amount of TG that was balanced by reduced amount of phospholipids and cholesterol compared to mice inoculated with the microbiota of young mice. We further analyzed the expression of several genes encoding enzymes involved in the biogenesis of TG and cholesterol, but we did not observe any alteration. A hypothesize to explain the decrease in hepatic cholesterol in mice inoculated with the microbiota of old donors could be an enhanced conversion of cholesterol to bile acids. Indeed, bile acids are synthesized from cholesterol by hepatocytes and gut microbiota is involved in the regulation of bile acid synthesis, in particular by modulating the hepatic expression of the cholesterol 7α-hydroxylase (CYP7A1) (Sayin et al., 2013).
Moreover, the fatty acid profile observed in the liver was almost entirely similar to that of the brain. Except for SFA, whose amount was unchanged in the brain and decreased in the liver, transfer of an aged microbiota to mice induced an increase in the level of MUFA and a decrease in the level of n-3 PUFA compared to mice harboring a young microbiota. Accordingly, the mono-unsaturation index was augmented and the poly-unsaturation index was reduced in mice with an aged microbiota. Several studies support the role of gut microbes as potent regulators of hepatic lipid metabolism. Indeed, comparison of hepatic fatty acid metabolism between germ-free and conventionally raised specific-pathogen-free mice has shown that gut microbes impact the MUFA content and PUFA elongation by modifying Scd1 and Elovl5 expression, respectively (Kindt et al., 2018). In addition, alteration of the expression level of genes involved in hepatic lipid metabolism (Srebp1c, Acc, Fas, Scd1, Acat2, Dgat1, Dgat2, Hmgcs, and Hmgcr) has been reported in TLR5-deficient mice (Singh et al., 2015). In our study, we showed that modifications of the hepatic content in MUFA and PUFA were associated with the dysregulated expression of genes involved in fatty acid biosynthesis (upregulation of Scd1, and downregulation of Fads1, and Fads2 gene expression). Unexpectedly, we observed an increase in the expression level of Elovl2 and Elovl5 genes that encode elongases in the liver of mice inoculated with the microbiota of old mice while the elongation index was decreased. Although these results seem inconsistent, similar data has already been described by others. Indeed, Wang et al. observed an increase in MUFA abundance associated with a decrease in PUFA abundance in the liver of obese mice while the expression of Elovl5, Elovl6, and Scd1 were upregulated (Wang et al., 2006). The facilitation of hepatic elongation and desaturation processes involving ELOVL5 and SCD1 could contribute to and explain the increased production of hepatic MUFA.
Our study was conducted in male mice. However, studies in human and animal models have shown that sex dimorphism in lipid metabolism exists and that the phospholipid and fatty acid compositions of several tissues, including those of plasma, brain and liver, differ according to the gender (Childs et al., 2010; Slater-Jefferies et al., 2010; Varlamov et al., 2014; Rodriguez-Navas et al., 2016). Thus, it would be interesting to evaluate in another study whether an aged microbiota would induce in recipient female germ free mice similar changes in the fatty acid and phospholipid contents than those observed in males.
Metabolites generated by an aged microbiota and that promote the remodeling of lipid and fatty acid contents in the brain and in the liver remain to be identified. SCFA may constitute good candidates as they have been described to influence hepatic lipogenesis in addition to the part they take in the communication pathways between the gut and the brain (De Vadder et al., 2014; Singh et al., 2015; Dinan and Cryan, 2017; Kindt et al., 2018). In our study, alteration in the cortex lipid content due to gut colonization by an aged microbiota seems to reflect those observed in the liver, the hub of lipid metabolism. Identifying and acting on the microbial mediators involved in the dysregulation of hepatic lipogenesis might help develop strategies to influence brain lipid composition and prevent age-related lipid modifications of this tissue.
In conclusion, this study provides evidence that gut microbiota should also be considered as an important contributor to lipid changes occurring in the brain during aging. Changes in the gut microbiota related to aging is associated with modifications in lipid classes (in particular in reducing cholesterol amounts) and in fatty acid profile (in decreasing PUFA and among them DHA, and in increasing MUFA content) in the cortex. Such alterations have been described as features of cerebral aging. Given the crucial role played by these lipids in neuronal membrane dynamics but also in cell signalization, these microbiota-driven alterations of lipids could have profound impact on brain physiology.
Alterations of the hepatic lipid profile and fatty acid synthesis were also shown in response to gut colonization by an aged microbiota. This hepatic phenotype could be one of the drivers of the modification occurring in the brain but may also impact the metabolic activity of others organs. This point needs further studies.
Altogether, our results support the idea that manipulation of the microbiota holds promise as an innovative strategy to influence the development of comorbidities associated with aging.
Data Availability Statement
All datasets generated for this study are included in the article/Supplementary Material.
Ethics Statement
Experiments on animals were performed at the Anaxem platform (Micalis Institute, INRA, Jouy-en-Josas, France). The Anaxem facilities are accredited by the French “Direction Départementale de la Protection des Populations (DDPP78),” accreditation number A78-322-6. All procedures involving animal experimentation were carried out according to the European guidelines for the care and use of laboratory animals and were approved by the “French Ministère de l'Enseignement Supérieur et de la Recherche” (authorization number 3441-2016010614307552).
Author Contributions
M-AB, PL, and NA designed the experiments. FC performed inoculation of germ-free mice with fecal microbiota. FC and MA collected the samples. CC and PL analyzed the data from microbiota sequencing. MA and BB performed gene expression analyses. SG and LM performed lipid class profiles and fatty acid analysis by gas chromatography. J-PP, SC, and OB performed brain lipid quantification by HPLC-MS2. MA, AB, NA, and M-AB analyzed the data and prepared the figures and tables. MA and M-AB wrote the manuscript. All authors reviewed the final version of the manuscript.
Funding
This work was supported by grants from Agence Nationale de la Recherche [ANR-11-LABX-0021-01]; INRA, the Conseil Régional Bourgogne, Franche-Comté [PARI grant]; and the FEDER (European Funding for Regional Economical Development); Fondation de France/Fondation de l'oeil.
Conflict of Interest
AB is consultant for Aerie, Allergan, Bausch & Lomb, Santen, and Théa Pharma.
The remaining authors declare that the research was conducted in the absence of any commercial or financial relationships that could be construed as a potential conflict of interest.
Supplementary Material
The Supplementary Material for this article can be found online at: https://www.frontiersin.org/articles/10.3389/fcimb.2019.00444/full#supplementary-material
References
Acar, N., Berdeaux, O., Juaneda, P., Gregoire, S., Cabaret, S., Joffre, C., et al. (2009). Red blood cell plasmalogens and docosahexaenoic acid are independently reduced in primary open-angle glaucoma. Exp. Eye Res. 89, 840–853. doi: 10.1016/j.exer.2009.07.008
Adak, A., and Khan, M. R. (2019). An insight into gut microbiota and its functionalities. Cell Mol. Life Sci. 76, 473–493. doi: 10.1007/s00018-018-2943-4
Adibhatla, R. M., and Hatcher, J. F. (2007). Role of lipids in brain injury and diseases. Fut. Lipidol 2, 403–422. doi: 10.2217/17460875.2.4.403
Alvarez-Mercado, A. I., Navarro-Oliveros, M., Robles-Sanchez, C., Plaza-Diaz, J., Saez-Lara, M. J., Munoz-Quezada, S., et al. (2019). Microbial population changes and their relationship with human health and disease. Microorganisms 7:68. doi: 10.3390/microorganisms7030068
Backhed, F., Ding, H., Wang, T., Hooper, L. V., Koh, G. Y., Nagy, A., et al. (2004). The gut microbiota as an environmental factor that regulates fat storage. Proc. Natl. Acad. Sci. U.S.A. 101, 15718–15723. doi: 10.1073/pnas.0407076101
Backhed, F., Manchester, J. K., Semenkovich, C. F., and Gordon, J. I. (2007). Mechanisms underlying the resistance to diet-induced obesity in germ-free mice. Proc. Natl. Acad. Sci. U.S.A. 104, 979–984. doi: 10.1073/pnas.0605374104
Biagi, E., Franceschi, C., Rampelli, S., Severgnini, M., Ostan, R., Turroni, S., et al. (2016). Gut microbiota and extreme longevity. Curr. Biol. 26, 1480–1485. doi: 10.1016/j.cub.2016.04.016
Boden, G. (2008). Obesity and free fatty acids. Endocrinol. Metab. Clin. North Am. 37, 635–646, viii–ix. doi: 10.1016/j.ecl.2008.06.007
Bowen, D. M., Smith, C. B., and Davison, A. N. (1973). Molecular changes in senile dementia. Brain 96, 849–856. doi: 10.1093/brain/96.4.849
Bruce, K. D., Zsombok, A., and Eckel, R. H. (2017). Lipid processing in the brain: a key regulator of systemic metabolism. Front. Endocrinol. 8:60. doi: 10.3389/fendo.2017.00060
Chen, C. T., Domenichiello, A. F., Trepanier, M. O., Liu, Z., Masoodi, M., and Bazinet, R. P. (2013). The low levels of eicosapentaenoic acid in rat brain phospholipids are maintained via multiple redundant mechanisms. J. Lipid Res. 54, 2410–2422. doi: 10.1194/jlr.M038505
Childs, C. E., Romeu-Nadal, M., Burdge, G. C., and Calder, P. C. (2010). The polyunsaturated fatty acid composition of hepatic and plasma lipids differ by both sex and dietary fat intake in rats. J. Nutr. 140, 245–250. doi: 10.3945/jn.109.115691
Contreras, M. A., Greiner, R. S., Chang, M. C., Myers, C. S., Salem, N. Jr., and Rapoport, S. I. (2000). Nutritional deprivation of alpha-linolenic acid decreases but does not abolish turnover and availability of unacylated docosahexaenoic acid and docosahexaenoyl-CoA in rat brain. J. Neurochem. 75, 2392–2400. doi: 10.1046/j.1471-4159.2000.0752392.x
Davenport, E. R. (2016). Elucidating the role of the host genome in shaping microbiome composition. Gut Microbes 7, 178–184. doi: 10.1080/19490976.2016.1155022
de Diego, I., Peleg, S., and Fuchs, B. (2019). The role of lipids in aging-related metabolic changes. Chem. Phys. Lipids 222, 59–69. doi: 10.1016/j.chemphyslip.2019.05.005
De Vadder, F., Kovatcheva-Datchary, P., Goncalves, D., Vinera, J., Zitoun, C., Duchampt, A., et al. (2014). Microbiota-generated metabolites promote metabolic benefits via gut-brain neural circuits. Cell 156, 84–96. doi: 10.1016/j.cell.2013.12.016
Dietschy, J. M., and Turley, S. D. (2004). Thematic review series: brain Lipids. cholesterol metabolism in the central nervous system during early development and in the mature animal. J. Lipid Res. 45, 1375–1397. doi: 10.1194/jlr.R400004-JLR200
Dinan, T. G., and Cryan, J. F. (2017). The Microbiome-gut-brain axis in health and disease. Gastroenterol. Clin. North Am. 46, 77–89. doi: 10.1016/j.gtc.2016.09.007
Ding, R. X., Goh, W. R., Wu, R. N., Yue, X. Q., Luo, X., Khine, W. W. T., et al. (2019). Revisit gut microbiota and its impact on human health and disease. J. Food Drug Anal. 27, 623–631. doi: 10.1016/j.jfda.2018.12.012
Farooqui, A. A., Horrocks, L. A., and Farooqui, T. (2000). Glycerophospholipids in brain: their metabolism, incorporation into membranes, functions, and involvement in neurological disorders. Chem. Phys. Lipids 106, 1–29. doi: 10.1016/S0009-3084(00)00128-6
Favrelere, S., Stadelmann-Ingrand, S., Huguet, F., De Javel, D., Piriou, A., Tallineau, C., et al. (2000). Age-related changes in ethanolamine glycerophospholipid fatty acid levels in rat frontal cortex and hippocampus. Neurobiol. Aging 21, 653–660. doi: 10.1016/S0197-4580(00)00170-6
Folch, J., Lees, M., and Sloane Stanley, G. H. (1957). A simple method for the isolation and purification of total lipides from animal tissues. J. Biol. Chem. 226, 497–509.
Garcia-Pena, C., Alvarez-Cisneros, T., Quiroz-Baez, R., and Friedland, R. P. (2017). Microbiota and aging. a review and commentary. Arch. Med. Res. 48, 681–689. doi: 10.1016/j.arcmed.2017.11.005
Gazzah, N., Gharib, A., Bobillier, P., Lagarde, M., and Sarda, N. (1994). Evidence for brain docosahexaenoate recycling in the free-moving adult rat: implications for measurement of phospholipid synthesis. Neurosci. Lett. 177, 103–106. doi: 10.1016/0304-3940(94)90055-8
Giusto, N. M., Roque, M. E., and Ilincheta De Boschero, M. G. (1992). Effects of aging on the content, composition and synthesis of sphingomyelin in the central nervous system. Lipids 27, 835–839. doi: 10.1007/BF02535859
Giusto, N. M., Salvador, G. A., Castagnet, P. I., Pasquare, S. J., and Ilincheta De Boschero, M. G. (2002). Age-associated changes in central nervous system glycerolipid composition and metabolism. Neurochem. Res. 27, 1513–1523. doi: 10.1023/A:1021604623208
Goodrich, J. K., Waters, J. L., Poole, A. C., Sutter, J. L., Koren, O., Blekhman, R., et al. (2014). Human genetics shape the gut microbiome. Cell 159, 789–799. doi: 10.1016/j.cell.2014.09.053
Han, X. (2007). Neurolipidomics: challenges and developments. Front. Biosci. 12, 2601–2615. doi: 10.2741/2258
He, M., and Shi, B. (2017). Gut microbiota as a potential target of metabolic syndrome: the role of probiotics and prebiotics. Cell Biosci. 7:54. doi: 10.1186/s13578-017-0183-1
Jandhyala, S. M., Talukdar, R., Subramanyam, C., Vuyyuru, H., Sasikala, M., and Nageshwar Reddy, D. (2015). Role of the normal gut microbiota. World J. Gastroenterol. 21, 8787–8803. doi: 10.3748/wjg.v21.i29.8787
Katz, R., Hamilton, J. A., Pownall, H. J., Deckelbaum, R. J., Hillard, C. J., Leboeuf, R. C., et al. (2007). Brain uptake and utilization of fatty acids, lipids & lipoproteins: recommendations for future research. J. Mol. Neurosci. 33, 146–150. doi: 10.1007/s12031-007-0059-7
Kennedy, B. K., Berger, S. L., Brunet, A., Campisi, J., Cuervo, A. M., Epel, E. S., et al. (2014). Geroscience: linking aging to chronic disease. Cell 159, 709–713. doi: 10.1016/j.cell.2014.10.039
Kindt, A., Liebisch, G., Clavel, T., Haller, D., Hormannsperger, G., Yoon, H., et al. (2018). The gut microbiota promotes hepatic fatty acid desaturation and elongation in mice. Nat. Commun. 9:3760. doi: 10.1038/s41467-018-05767-4
Kolde, R., Franzosa, E. A., Rahnavard, G., Hall, A. B., Vlamakis, H., Stevens, C., et al. (2018). Host genetic variation and its microbiome interactions within the human microbiome project. Genome Med. 10:6. doi: 10.1186/s13073-018-0515-8
Kumar, M., Babaei, P., Ji, B., and Nielsen, J. (2016). Human gut microbiota and healthy aging: recent developments and future prospective. Nutr. Healthy Aging 4, 3–16. doi: 10.3233/NHA-150002
Lam, S. M., Chua, G. H., Li, X. J., Su, B., and Shui, G. (2016). Biological relevance of fatty acyl heterogeneity to the neural membrane dynamics of rhesus macaques during normative aging. Oncotarget 7, 55970–55989. doi: 10.18632/oncotarget.11190
Latour, A., Grintal, B., Champeil-Potokar, G., Hennebelle, M., Lavialle, M., Dutar, P., et al. (2013). Omega-3 fatty acids deficiency aggravates glutamatergic synapse and astroglial aging in the rat hippocampal CA1. Aging Cell 12, 76–84. doi: 10.1111/acel.12026
Letondor, A., Buaud, B., Vaysse, C., Fonseca, L., Herrouin, C., Servat, B., et al. (2014). Erythrocyte DHA level as a biomarker of DHA status in specific brain regions of n-3 long-chain PUFA-supplemented aged rats. Br. J. Nutr. 112, 1805–1818. doi: 10.1017/S0007114514002529
Little, S. J., Lynch, M. A., Manku, M., and Nicolaou, A. (2007). Docosahexaenoic acid-induced changes in phospholipids in cortex of young and aged rats: a lipidomic analysis. Prostaglandins Leukot. Essent. Fatty Acids 77, 155–162. doi: 10.1016/j.plefa.2007.08.009
Lopez-Otin, C., Blasco, M. A., Partridge, L., Serrano, M., and Kroemer, G. (2013). The hallmarks of aging. Cell 153, 1194–1217. doi: 10.1016/j.cell.2013.05.039
Lozupone, C. A., Stombaugh, J. I., Gordon, J. I., Jansson, J. K., and Knight, R. (2012). Diversity, stability and resilience of the human gut microbiota. Nature 489, 220–230. doi: 10.1038/nature11550
Lund, E. G., Guileyardo, J. M., and Russell, D. W. (1999). cDNA cloning of cholesterol 24-hydroxylase, a mediator of cholesterol homeostasis in the brain. Proc. Natl. Acad. Sci. U.S.A. 96, 7238–7243. doi: 10.1073/pnas.96.13.7238
Ma, Q., Xing, C., Long, W., Wang, H. Y., Liu, Q., and Wang, R. F. (2019). Impact of microbiota on central nervous system and neurological diseases: the gut-brain axis. J. Neuroinflam. 16:53. doi: 10.1186/s12974-019-1434-3
Martin, M., Dotti, C. G., and Ledesma, M. D. (2010). Brain cholesterol in normal and pathological aging. Biochim. Biophys. Acta. 1801, 934–944. doi: 10.1016/j.bbalip.2010.03.011
Martin, M. G., Perga, S., Trovo, L., Rasola, A., Holm, P., Rantamaki, T., et al. (2008). Cholesterol loss enhances TrkB signaling in hippocampal neurons aging in vitro. Mol. Biol. Cell 19, 2101–2112. doi: 10.1091/mbc.e07-09-0897
Martinez-Guryn, K., Hubert, N., Frazier, K., Urlass, S., Musch, M. W., Ojeda, P., et al. (2018). Small Intestine microbiota regulate host digestive and absorptive adaptive responses to dietary lipids. Cell Host Microbe 23, 458–469. doi: 10.1016/j.chom.2018.03.011
Mcmurdie, P. J., and Holmes, S. (2013). phyloseq: an R package for reproducible interactive analysis and graphics of microbiome census data. PLoS ONE 8:e61217. doi: 10.1371/journal.pone.0061217
McNamara, R. K., Liu, Y., Jandacek, R., Rider, T., and Tso, P. (2008). The aging human orbitofrontal cortex: decreasing polyunsaturated fatty acid composition and associated increases in lipogenic gene expression and stearoyl-CoA desaturase activity. Prostaglandins Leukot. Essent. Fatty Acids 78, 293–304. doi: 10.1016/j.plefa.2008.04.001
Morrison, W. R., and Smith, L. M. (1964). Preparation of fatty acid methyl esters and dimethylacetals from lipids with boron fluoride–methanol. J. Lipid Res. 5, 600–608.
Naudi, A., Cabre, R., Jove, M., Ayala, V., Gonzalo, H., Portero-Otin, M., et al. (2015). Lipidomics of human brain aging and Alzheimer's disease pathology. Int. Rev. Neurobiol. 122, 133–189. doi: 10.1016/bs.irn.2015.05.008
Nes, W. D. (2011). Biosynthesis of cholesterol and other sterols. Chem. Rev. 111, 6423–6451. doi: 10.1021/cr200021m
O'Brien, J. S., and Sampson, E. L. (1965). Lipid composition of the normal human brain: gray matter, white matter, and myelin. J. Lipid Res. 6, 537–544.
Oresic, M., Seppanen-Laakso, T., Yetukuri, L., Backhed, F., and Hanninen, V. (2009). Gut microbiota affects lens and retinal lipid composition. Exp. Eye Res. 89, 604–607. doi: 10.1016/j.exer.2009.06.018
O'Toole, P. W., and Jeffery, I. B. (2015). Gut microbiota and aging. Science 350, 1214–1215. doi: 10.1126/science.aac8469
O'Toole, P. W., and Jeffery, I. B. (2018). Microbiome-health interactions in older people. Cell Mol. Life Sci. 75, 119–128. doi: 10.1007/s00018-017-2673-z
Pararasa, C., Bailey, C. J., and Griffiths, H. R. (2015). Ageing, adipose tissue, fatty acids and inflammation. Biogerontology 16, 235–248. doi: 10.1007/s10522-014-9536-x
Perovic, M., Mladenovic Djordjevic, A., Smiljanic, K., Tanic, N., Rakic, L., Ruzdijic, S., et al. (2009). Expression of cholesterol homeostasis genes in the brain of the male rat is affected by age and dietary restriction. Biogerontology 10, 735–745. doi: 10.1007/s10522-009-9220-8
Qi, K., Hall, M., and Deckelbaum, R. J. (2002). Long-chain polyunsaturated fatty acid accretion in brain. Curr. Opin. Clin. Nutr. Metab. Care 5, 133–138. doi: 10.1097/00075197-200203000-00003
Rabot, S., Membrez, M., Bruneau, A., Gerard, P., Harach, T., Moser, M., et al. (2010). Germ-free C57BL/6J mice are resistant to high-fat-diet-induced insulin resistance and have altered cholesterol metabolism. FASEB J. 24, 4948–4959. doi: 10.1096/fj.10-164921
Rapin, A., Pattaroni, C., Marsland, B. J., and Harris, N. L. (2017). Microbiota analysis using an illumina MiSeq platform to sequence 16S rRNA Genes. Curr. Protoc. Mouse Biol. 7, 100–129. doi: 10.1002/cpmo.29
Rappley, I., Myers, D. S., Milne, S. B., Ivanova, P. T., Lavoie, M. J., Brown, H. A., et al. (2009). Lipidomic profiling in mouse brain reveals differences between ages and genders, with smaller changes associated with alpha-synuclein genotype. J. Neurochem. 111, 15–25. doi: 10.1111/j.1471-4159.2009.06290.x
Rodriguez-Carrio, J., Lopez, P., Sanchez, B., Gonzalez, S., Gueimonde, M., Margolles, A., et al. (2017). Intestinal dysbiosis is associated with altered short-chain fatty acids and serum-free fatty acids in systemic lupus erythematosus. Front. Immunol. 8:23. doi: 10.3389/fimmu.2017.00023
Rodriguez-Navas, C., Morselli, E., and Clegg, D. J. (2016). Sexually dimorphic brain fatty acid composition in low and high fat diet-fed mice. Mol. Metab. 5, 680–689. doi: 10.1016/j.molmet.2016.06.014
Rosenberger, T. A., Villacreses, N. E., Weis, M. T., and Rapoport, S. I. (2010). Rat brain docosahexaenoic acid metabolism is not altered by a 6-day intracerebral ventricular infusion of bacterial lipopolysaccharide. Neurochem. Int. 56, 501–507. doi: 10.1016/j.neuint.2009.12.010
Sastry, P. S. (1985). Lipids of nervous tissue: composition and metabolism. Prog. Lipid Res. 24, 69–176. doi: 10.1016/0163-7827(85)90011-6
Sayin, S. I., Wahlstrom, A., Felin, J., Jantti, S., Marschall, H. U., Bamberg, K., et al. (2013). Gut microbiota regulates bile acid metabolism by reducing the levels of tauro-beta-muricholic acid, a naturally occurring FXR antagonist. Cell Metab. 17, 225–235. doi: 10.1016/j.cmet.2013.01.003
Schroeder, E. A., and Brunet, A. (2015). Lipid profiles and signals for long life. Trends Endocrinol. Metab. 26, 589–592. doi: 10.1016/j.tem.2015.08.007
Singh, V., Chassaing, B., Zhang, L., San Yeoh, B., Xiao, X., Kumar, M., et al. (2015). Microbiota-dependent hepatic lipogenesis mediated by stearoyl CoA desaturase 1 (SCD1) promotes metabolic syndrome in TLR5-deficient mice. Cell Metab. 22, 983–996. doi: 10.1016/j.cmet.2015.09.028
Slater-Jefferies, J. L., Hoile, S. P., Lillycrop, K. A., Townsend, P. A., Hanson, M. A., and Burdge, G. C. (2010). Effect of sex and dietary fat intake on the fatty acid composition of phospholipids and triacylglycerol in rat heart. Prostaglandins Leukot. Essent. Fatty Acids 83, 219–223. doi: 10.1016/j.plefa.2010.07.006
Soderberg, M., Edlund, C., Kristensson, K., and Dallner, G. (1990). Lipid compositions of different regions of the human brain during aging. J. Neurochem. 54, 415–423. doi: 10.1111/j.1471-4159.1990.tb01889.x
Suiter, C., Singha, S. K., Khalili, R., and Shariat-Madar, Z. (2018). Free fatty acids: circulating contributors of metabolic syndrome. Cardiovasc. Hematol. Agents Med. Chem. 16, 20–34. doi: 10.2174/1871525716666180528100002
Svennerholm, L., Bostrom, K., Jungbjer, B., and Olsson, L. (1994). Membrane lipids of adult human brain: lipid composition of frontal and temporal lobe in subjects of age 20 to 100 years. J. Neurochem. 63, 1802–1811. doi: 10.1046/j.1471-4159.1994.63051802.x
Tracey, T. J., Steyn, F. J., Wolvetang, E. J., and Ngo, S. T. (2018). Neuronal lipid metabolism: multiple pathways driving functional outcomes in health and disease. Front. Mol. Neurosci. 11:10. doi: 10.3389/fnmol.2018.00010
Varlamov, O., Bethea, C. L., and Roberts, C. T. Jr. (2014). Sex-specific differences in lipid and glucose metabolism. Front. Endocrinol. 5:241. doi: 10.3389/fendo.2014.00241
Velagapudi, V. R., Hezaveh, R., Reigstad, C. S., Gopalacharyulu, P., Yetukuri, L., Islam, S., et al. (2010). The gut microbiota modulates host energy and lipid metabolism in mice. J. Lipid Res. 51, 1101–1112. doi: 10.1194/jlr.M002774
Wang, Y., Botolin, D., Xu, J., Christian, B., Mitchell, E., Jayaprakasam, B., et al. (2006). Regulation of hepatic fatty acid elongase and desaturase expression in diabetes and obesity. J. Lipid Res. 47, 2028–2041. doi: 10.1194/jlr.M600177-JLR200
Yang, J. Y., and Kweon, M. N. (2016). The gut microbiota: a key regulator of metabolic diseases. BMB Rep. 49, 536–541. doi: 10.5483/BMBRep.2016.49.10.144
Zhang, J., and Liu, Q. (2015). Cholesterol metabolism and homeostasis in the brain. Protein Cell 6, 254–264. doi: 10.1007/s13238-014-0131-3
Keywords: aging, microbiota, lipid, cholesterol, fatty acid, phospholipid, liver, cortex
Citation: Albouery M, Buteau B, Grégoire S, Cherbuy C, Pais de Barros J-P, Martine L, Chain F, Cabaret S, Berdeaux O, Bron AM, Acar N, Langella P and Bringer M-A (2020) Age-Related Changes in the Gut Microbiota Modify Brain Lipid Composition. Front. Cell. Infect. Microbiol. 9:444. doi: 10.3389/fcimb.2019.00444
Received: 23 October 2019; Accepted: 10 December 2019;
Published: 14 January 2020.
Edited by:
Frederic Antonio Carvalho, INSERM U1107 Douleur et Biophysique Neurosensorielle (Neuro-Dol), FranceReviewed by:
Sanjoy K. Bhattacharya, University of Miami, United StatesMaricela Rodriguez-Cruz, Instituto Mexicano del Seguro Social, Mexico
Copyright © 2020 Albouery, Buteau, Grégoire, Cherbuy, Pais de Barros, Martine, Chain, Cabaret, Berdeaux, Bron, Acar, Langella and Bringer. This is an open-access article distributed under the terms of the Creative Commons Attribution License (CC BY). The use, distribution or reproduction in other forums is permitted, provided the original author(s) and the copyright owner(s) are credited and that the original publication in this journal is cited, in accordance with accepted academic practice. No use, distribution or reproduction is permitted which does not comply with these terms.
*Correspondence: Marie-Agnès Bringer, bWFyaWUtYWduZXMuYnJpbmdlciYjeDAwMDQwO2lucmEuZnI=
†These authors have contributed equally to this work