Corrigendum: Transcriptome, Phenotypic, and Virulence Analysis of Streptococcus sanguinis SK36 Wild Type and Its CcpA-Null Derivative (ΔCcpA)
- 1Department of Pathogen Biology, College of Basic Medical Sciences, China Medical University, Shenyang, China
- 2Department of Scientific Research, Peking Union Medical College Hospital (East), Beijing, China
- 3Department of Pathophysiology, College of Basic Medical Science, China Medical University, Shenyang, China
Catabolic control protein (CcpA) is linked to complex carbohydrate utilization and virulence factor in many bacteria species, influences the transcription of target genes by many mechanisms. To characterize the activity and regulatory mechanisms of CcpA in Streptococcus sanguinis, here, we analyzed the transcriptome of Streptococcus sanguinis SK36 and its CcpA-null derivative (ΔCcpA) using RNA-seq. Compared to the regulon of CcpA in SK36 in the RegPrecise database, we found that only minority of differentially expressed genes (DEGs) contained putative catabolite response element (cre) in their regulatory regions, indicating that many genes could have been affected indirectly by the loss of CcpA and analyzing the sequence of the promoter region using prediction tools is not a desirable method to recognize potential target genes of global regulator CcpA. Gene ontology and pathway analysis of DEGs revealed that CcpA exerts an influence predominantly involved in carbon catabolite metabolism and some amino acid catabolite pathways, which has been linked to expression of virulence genes in many pathogens and coordinately regulate the disease progression in vivo studies. However, in some scenarios, differences observed at the transcript level could not reflect the real differences at the protein level. Therefore, to confirm the differences in phenotype and virulence of SK36 and ΔCcpA, we characterized the role of CcpA in the regulation of biofilm development, EPS production and the virulence of Streptococcus sanguinis. Results showed CcpA inactivation impaired biofilm and EPS formation, and CcpA also involved in virulence in rabbit infective endocarditis model. These findings will undoubtedly contribute to investigate the mechanistic links between the global regulator CcpA and the virulence of Streptococcus sanguinis, further broaden our understanding of the relationship between basic metabolic processes and virulence.
Introduction
Infective endocarditis (IE) caused by microorganisms has long been regarded as dependent upon biofilms (Costerton et al., 1999; Douglas, 2003; Parsek and Singh, 2003; Xu et al., 2003). Streptococcus sanguinis was recognized as one of the most common causes of endocarditis, alongside Staphylococcus aureus, Enterococcus spp., and Streptococcus bovis (Vogkou et al., 2016; Zhu et al., 2018). It was well-known that biofilm formation is tightly interconnected with EPS production and the degree of exposure of S. sanguinis surface adhesion molecules for the initial colonization (Flemming and Wingender, 2010). Although various factors related to the virulence of S. sanguinis have been identified, the regulatory mechanisms of colonization and biofilm development are still elusive.
Catabolic control protein (CcpA) is linked to complex carbohydrate utilization and virulence factor production in many bacteria species in response to changes in overall energy levels and amount of carbohydrate as the global regulator of carbon catabolite repression (CCR) (Abranches et al., 2008; Willenborg et al., 2014). For example, CcpA has been reported to influence the expression of diverse virulence factors of S. aureus, Streptococcus mutans, and Enterococcus faecium in response to various kinds and concentrations of carbohydrate (Somarajan et al., 2014; Bischoff et al., 2017; Bauer et al., 2018). In Streptococcus suis, CcpA was found to be indispensable for capsule production in glucose-affluent conditions and virulence-associated gene expression (Willenborg et al., 2011). In Streptococcus pneumoniae, capsule expression was also regulated by RegM/CcpA (Giammarinaro and Paton, 2002). Earlier studies have shown that glucan-producing S. sanguinis was found to be more difficultly cleaned from the circulation than glucan-negative mutants (Parker and Ball, 1976; Ramirez-Ronda, 1978). Pioneering work by Bin Zhu and his coworkers reported that insoluble glucan is the major component of S. sanguinis biofilms (Zhu et al., 2017). Skov Sorensen and his coworkers demonstrated that these polysaccharides are similar to S. pneumonia capsular polysaccharide (CPS) in genetic and antigenic aspect, even they are the equivalent of capsular polysaccharides of pneumococci (Skov Sorensen et al., 2016). Considering that CcpA is widely conserved, and the overall CPS structural similarity that exists between viridans group suggests a common biosynthetic pathway for these molecules (Xu et al., 2003; Yang et al., 2009; Skov Sorensen et al., 2016), which prompted us to characterize the role CcpA plays in the biofilm development, EPS production and virulence of S. sanguinis and further to identify potential targets of global regulator CcpA regulated that contribute to cause IE.
It is well-known that CcpA exerts its regulatory role by binding to a typical consensus site called catabolite response element (cre) in the promotor regions (Weickert and Chambliss, 1990). Recently, a novel mode of regulation of the S. aureus CcpA mediated by Stk1 protein phosphorylation was found (Leiba et al., 2012), and the study by Chen et al. (2019) reported that the pilin genes cluster of S. sanguinis SK36 occurred in a CcpA-dependent manner, although a typical cre is absent in the target region (SSA_2318). Furthermore, some researchers have reported the distinct regulatory role of CcpA in S. sanguinis, may involve in the key metabolic pathways through specific metabolic product (Redanz et al., 2018). All these suggested that there may be other unknown regulation model exists. Consequently, analyzing the sequence of the promoter region using the prediction tools is not a desirable method to recognize the CcpA regulated target genes.
In this study, we analyzed the whole transcriptome of wild-type S. sanguinis SK36 and its CcpA-null derivative (ΔCcpA) using high throughput sequencing technologies (RNA-seq). We not only revealed the potential target genes of CcpA in S. sanguinis and showed that some amino acid catabolic pathways are regulated by CcpA in S. sanguinis, we also characterize the role of CcpA in the regulation of EPS production, biofilm formation and the virulence of S. sanguinis. We found that CcpA inactivation impaired EPS production and biofilm formation in vitro, and CcpA also involved in virulence in a rabbit IE model. These findings will undoubtedly contribute to investigate the mechanistic links between the global regulator CcpA and the virulence of S. sanguinis, further broaden our understanding of the relationship between basic metabolic processes and virulence.
Materials and Methods
Bacterial Strains and Culture Conditions
S. sanguinis strains were obtained from Zheng et al. (2011), including SK36, the CcpA mutant (ΔCcpA), and the complement of the ΔCcpA (CcpA+). We grew strains as static cultures at 37°C in brain heart infusion (BHI; Difco, Sparks, MD) or on BHI agar plates in an anaerobic chamber (90% N2, 5% CO2, 5% H2). All the strains grew well and reached the same plateau though CcpA mutant showed a decreased growth. So, we collected the cells at the mid-exponential phase (A 600 = 0.5).When required for selection, antibiotics were added to the medium as follows: spectinomycin at 500 μg/ml and erythromycin at 2 μg/ml for S. sanguinis.
RNA Isolation and Sequencing
RNA was isolated using the KANGWEI Ultrapure RNA Kit (CWBIO, China) and the isolated RNA was subjected to DNase I (Promega, Beijing, China) treatment and purified with the TIANGEN RNAclean kit (TIANGEN, China). The clean RNA samples were aliquoted into two tubes and frozed at −80°C for further RT-PCR or RNA-Seq processing. The Ribo-Zero TM Magnetic Kit (Gram-Positive Bacteria) was used to remove ribosomal RNA (rRNA) and enrich the mRNA to compensate for the low-input samples. The average RNA Integrity number (RIN) was 8, and the average RNA yield was 100 ng/μl. The library preparation, sequencing, and initial quality check were performed by Berry Genomics Corporation, Genomics and Bioinformatics Service, China (http://www.berrygenomics.com/tech-services/illumina). Samples were then sequenced using the Illumina HiseqTM 2500 Next Generation Sequencer at Berry Genomics Corporation, China. Three independent experiments of each group were performed and sequenced. The resulting sequences were then aligned to the reference genome of strain SK36 (GenBank Accession: NC_009009) in order to create a transcriptome map using EDGE-pro. Gene quantification was calculated by the reads per kilobase per million mapped reads method (RPKM), using RSEM (V1.2.15) software.
Differentially Expressed Genes (DEGs) Analysis
Differences in gene expression profiles were performed using EdgeR statistics including a Benjamini and Hochberg false discovery rate correction. Each group had three biological replicates. False Discovery Rate (FDR) < 0.05 and |log2RPKM ratios (ΔCcpA/WT)|>1 were taken as the thresholds to ascertain the significance of differences in gene expression. To further investigate the DEGs, these DEGs were clustered and presented as heat maps.
Quantitative Real-Time PCR (qRT-PCR)
SSA-1575, SSA-2379, SSA-0016, and SSA-0391, randomly selected DEGs, were performed qRT-PCR to validate the results of RNA-seq. cDNA was synthesized from 2 μg of RNA using the SuperScript II reverse transcriptase (Invitrogen) followed the manufacturer's recommendation. The primer sequences are listed in Table 1. The gyrA gene was used as reference gene for calculation of the relative target gene expression using the 2−ΔΔCt method. All qPCR results are presented as ratios of the ΔCcpA/wild type levels relative to gyrA transcripts. The experiments were repeated three times independently, and three replicates were involved in each sample. Data were analyzed with GraphPad Prism (version 5.0) software using Student's t-test (P-value below 0.05 was considered statistical significance).
Gene Ontology (GO) and Pathway Analysis
To characterize the GO terms, including molecular functions, biological processes, cellular components, and functional pathways of DEGs, significantly enriched GO terms was analyzed by a hypergeometric test, based on “GO Term Finder” (Boyle et al., 2004; Lang et al., 2015). All the DEGs were mapped to the terms in KO (KEGG Orthology) identifier (Kanehisa et al., 2012; Zeng et al., 2013) using KOBAS 2.0 to identify which pathways the significant DEGs belonged to.
Biofilm Development and Quantification
Biofilm formation was measured as reported previously (Zheng et al., 2012), however, some procedures were modified. Briefly, SK36, ΔCcpA, and CcpA+ overnight grown cultures were diluted 1:60 in fresh BHI media supplemented with 0.2% sucrose and inoculated into microtiter plates(96-well cell culture plates; Thermo Fisher scientific), then anaerobically grown as static cultures for 48 h at 37°C to form biofilms. After removing the residual medium and air-drying the microtiter dish, the residual cells were resuspended in the well with 90% ethanol and then transferred to a new-flat bottomed microtiter dish with 100 mL of ethanol where it was measured at 570 nm using a microplate reader. Data were analyzed with GraphPad Prism (version 5.0) software. Statistical significance was indicated when the P-value was below 0.05.
Detection of EPS-Producing Phenotype of the Strains by the Congo Red Agar (CRA) Plate Test
CRA was prepared by adding 0.8 g of Congo red and 50 g of sucrose to 1 L of brain heart infusion agar as described previously (Mathur et al., 2006; Zheng et al., 2012). SK36, ΔCcpA, and CcpA+ overnight cultures grown in BHI medium were streaked on CRA plates and anaerobically cultured for 21 h. Then, plates were scanned with an HP scanner or photographed with a digital camera. The interaction of the direct dye Congo red with intact β-D-glucans (one molecule of Congo red is bound to six D-glucose residues of the D-glucan chain) provides the basis for a rapid and sensitive assay system for detection of EPS production of bacteria (Ogawa and Hatano, 1978).
Rabbit Model of IE
In this study, to study the role of global regulator CcpA in the virulence of S. sanguinis, we used the rabbit endocarditis model. The experiment was performed using a modification of transaortic catheterization models of endocarditis as previously described (Fan et al., 2012) and was reviewed and approved by the IACUC of the China Medical University Laboratory Animal Center. Adult Rex rabbits (2–3 kg; obtained from China Medical University Laboratory Animal Center) were used. Twenty-four hours after the positioning of the catheter, a total of 44 rabbits were assigned randomly to the following treatment groups: A, SK36 (n = 12); B, ΔCcpA (n = 12); C, CcpA+(n = 12); D, control (n = 8). Viable S. sanguinis (~109 CFU) or PBS was injected intravenously via the marginal ear vein. SK36, ΔCcpA, or CcpA+ strains were collected at cell densities (A600 = 0.63) and then washed and resuspended with PBS to the desired cell density as inoculated organisms. Twenty-four hours after injection, 200 μl of arterial blood was extracted from the ear of each animal to be quantitatively cultured in duplicate on BHI plates to determine the number of bacteria present in the blood. Three days after injection, the animals were euthanized. At autopsy, the proper positioning of the catheter was verified. Vegetations were excised, weighed, and homogenized in PBS before being quantitatively cultured in duplicate on BHI plates after serial dilutions in BHI broth. After 24 h incubation at 37°C, the colonies growing on BHI plates were expressed as log10 CFU. Bacterial load from vegetations was expressed by its mean value standard error (SE), and comparisons between groups were performed using one-way analysis of variance (ANOVA). The correlation between CFU and vegetation mass were assessed using Prism (GraphPad Software, La Jolla, CA).
Results
Reads Obtaining and Differentially Expressed Genes (DEGs) Analysis
In this report, we characterized the transcriptional profile of ΔCcpA mutant by analyzing the RNA-Seq data. After performing quality control, we obtained an average of over 20 million clean reads from each group (n = 3). The first group (ΔCcpA) was composed of 7.4, 6.8, and 7.0 million reads and the second group (SK36 WT) contained 7.1, 6.9, and 7.5 million reads. Overall, 76–82% of reads were mapped to the SK36 genome by using EDGE-pro. The saturation curves showed that the sequencing became saturated and the gene coverage indicated adequate sequencing depth (Figures S1–S3 in Supplementary File 1). Data were deposited with the Sequence Read Archive (SRA) at the National Center for Biotechnology Institute (accession PRJNA564466). Differentially expressed genes (DEGs) analysis using EdgeR statistics revealed that the deletion of CcpA in S. sanguinis significantly down-regulated 85 unigenes expression and up-regulated 84 unigenes expression of identified 897 unigenes (Supplementary File 2) compared to wild type SK36 (Table 2), according to the standard of a significant difference in expression levels. When compared the differentially expressed genes (DEGs) to the regulon of CcpA in S. sanguinis SK36 in the RegPrecise database (http://regprecise.lbl.gov, a web resource for collection, visualization and analysis of transcriptional regulons reconstructed by comparative genomics, Supplementary File 3), as a result, 29 of the 84 up-regulated DEGs, and 5 of the 85 down-regulation of DEGs were in the list of the regulon of CcpA (Table 2). To further investigate the nature of the DEGs, we performed Hierarchical clustering analysis and heatmap of the 173 DEGs with the smallest q-values using a Pearson correlation distance metric. The triplicates were analyzed in each group (Figure 1A).
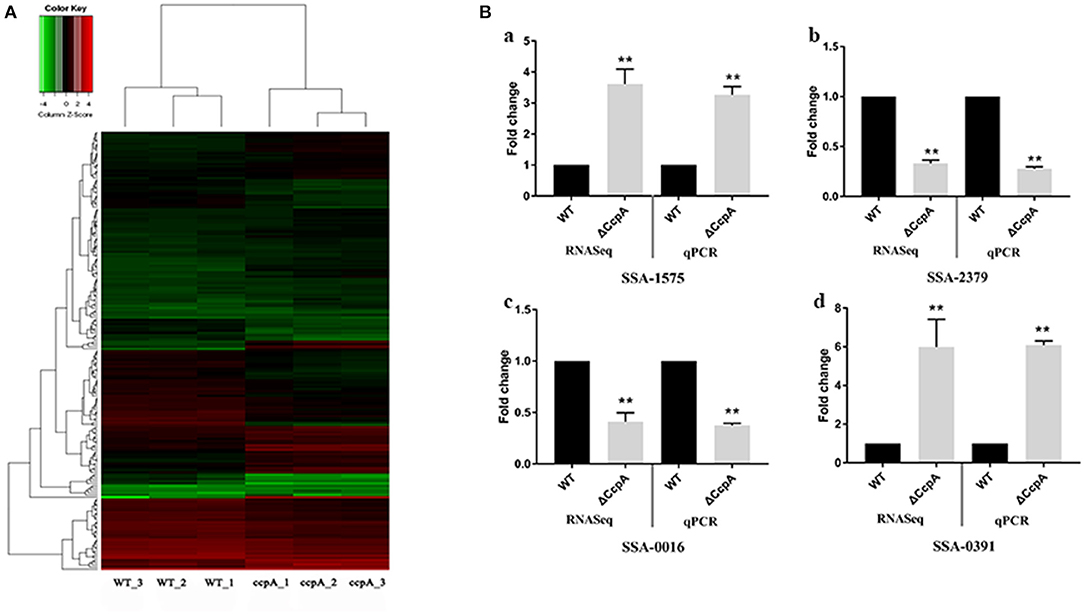
Figure 1. Analysis and validation of DEGs obtained by the RNA-Seq experiments. (A) Hierarchical clustering analysis and heatmap of the 195 DEGs with the smallest q-values. Three biological replicates of each group were analyzed separately. (B) qRT-PCR validation of selected DEGs, with expression level in ΔCcpA mutant normalized to the SK36 wild type. The transcript levels of (a) SSAA-1575, (b) SSA-2379, (c) SSA-0016, and (d) SSA-0391 of ΔCcpA mutant were detected by qRT-PCR. The data presented are averages and standard deviations of three independent experiments with similar results, triplicate in each experiment. **Indicates the significant difference at P < 0.01 compared to the SK36.
Confirmation of RNA-Seq Results by qRT-PCR
To validate the DEGs observed by the RNA-Seq experiments, we randomly selected four DEGs (SSA-1575, SSA-2379, SSA-0016, and SSA-0391) to examine the transcript levels by qRT-PCR. The results of qRT-PCR matched those of the RNA-seq: the expression of SSA-1575, SSA-2379, SSA-0016, and SSA-0391 in ΔCcpA mutant were 3.27-, 0.28-, 0.37-, 6.08-fold compared to wild type SK36, respectively (Figure 1B). The consistent results revealed that the DEGs obtained by RNA-Seq data is reliable and efficient.
Gene Ontology (GO) and Pathway Analysis of DEGs
Then, to characterize DEGs in functional groups and identify pathways that were significantly regulated by S. sanguinis CcpA, we performed GO terms and pathway analyses. Of the 85-down regulated DEGs, 56 DEGs could be assigned to a significant GO classification. Of the 84-up regulated DEGs, 74 DEGs could be assigned to a significant GO classification (Figure 2, Supplementary File 4). These results suggested that DEGs predominately involved in basic metabolic processes. Then KEGG pathway enrichment analysis was performed to identify pathways that regulated by CcpA, among the 85 down-regulated and 84 up-regulated DEGs, 27 and 48 DEGs were mapped to 24 and 33 KEGG pathways, respectively (Supplementary File 5). Seven pathways namely-“Pyruvate metabolism,” “Butanoate metabolism,” “Taurine and hypotaurine metabolism,” “Propanoate metabolism,” “Phenylalanine, tyrosine, and tryptophan biosynthesis,” “Carbon fixation pathways in prokaryotes,” and “Phosphotransferase system (PTS)” were the most significant (P < 0.05) pathway represented by the up-regulated DEGs, while the down-regulated DEGs predominantly involved in the “Arginine and proline metabolism,” “Biosynthesis of amino acids,” and “2-Oxocarboxylic acid metabolism” pathway (Figure 3).
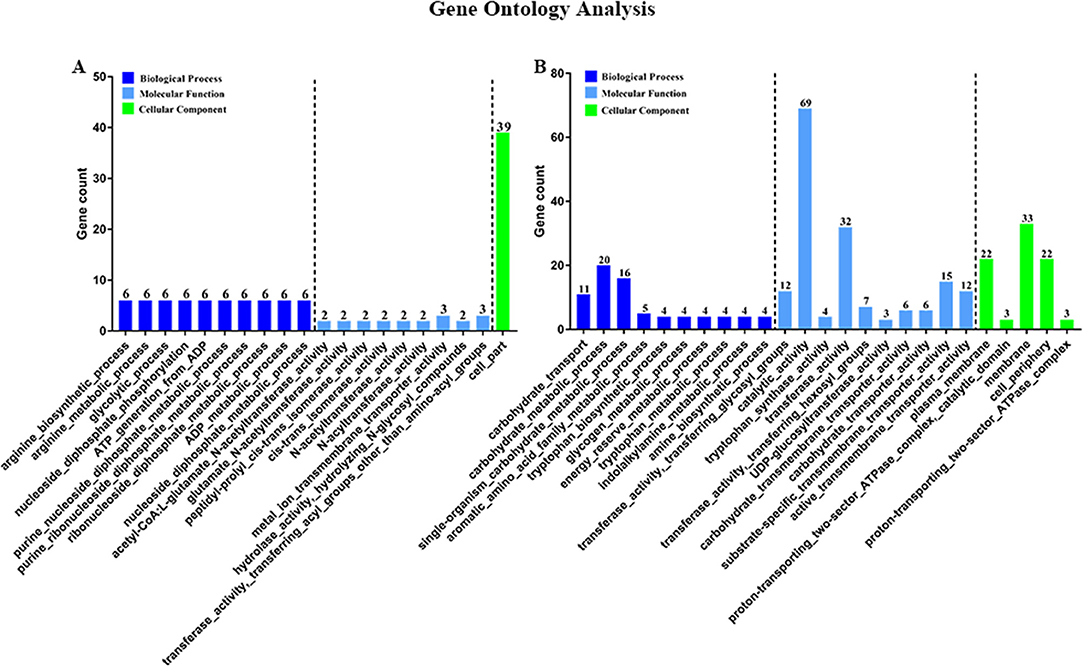
Figure 2. Gene Ontology (GO) classification of DEGs. Genes were annotated in three categories: biological process, molecular function, and cellular component. Y-axis represents the gene counts of a specific category of DEGs within that main category. X-axis represents the top 10 significant (p < 0.05) enrichment pathway Term (If less than 10,it represents all enrichment pathway Terms). (A,B) Represent CcpA case vs. WT ctrl down-regulated genes GO Term and CcpA case vs. WT ctrl up-regulated genes GO Term, respectively.
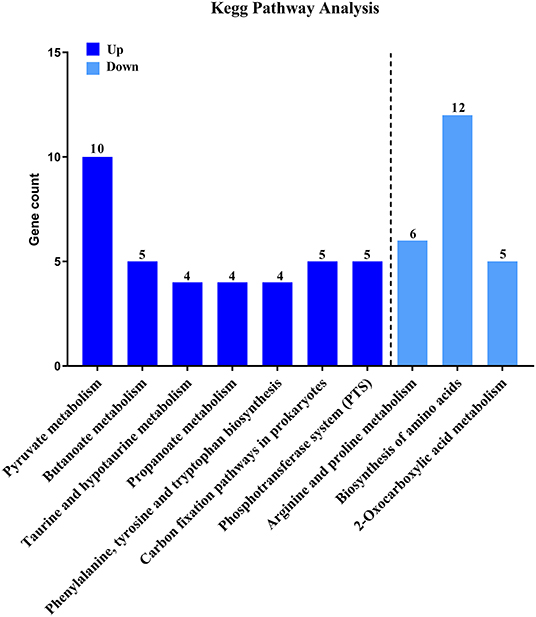
Figure 3. KEGG pathway analysis of DEGs. Here represents CcpA case vs. WT ctrl significantly (P < 0.05) up-regulated genes pathway enrichment and CcpA case vs. WT ctrl significantly (P < 0.05) down-regulated genes pathway enrichment, respectively.
ΔCcpA Mutant Shows Decreased Formation of Biofilm
Regarding of the importance of biofilm formation in infective endocarditis (Moser et al., 2017), we further explored the potential role of CcpA in the formation of biofilm. We observed biofilm development in vitro by a microtiter plate assay. As expected, quantitative analysis of biofilm production of SK36, ΔCcpA, and CcpA+ grown on the microtiter plate surface indicated that ΔCcpA showed weakened biofilm-forming ability compared to SK36 and CcpA+ (Figure 4).
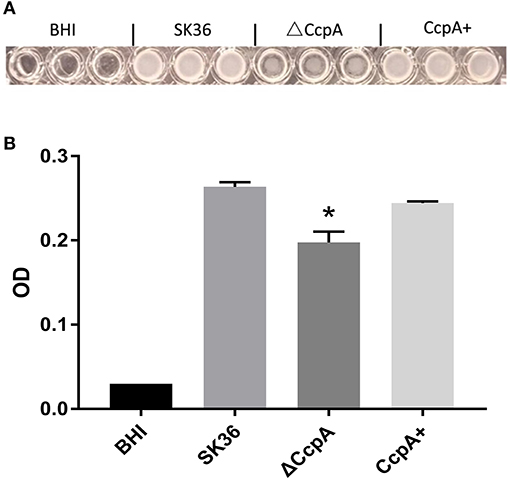
Figure 4. The effect of CcpA on the formation of biofilm. SK36, ΔCcpA, and CcpA+ strains cultures were inoculated into microtiter plates and anaerobically grown as static cultures for 48 h at 37°C to form biofilms. (A) Representative of microtiter plate wells from each experiment showing the respective biofilm formation of each S. sanguinis strain. (B) Quantitative analysis of biofilm production measuring at 570 nm using a microplate reader. The data presented are averages and standard deviations of three independent experiments with similar results, triplicate in each experiment. *Indicates the significant difference at P < 0.05 compared to the SK36.
ΔCcpA Shows Impaired EPS Production
To investigate the role that CcpA plays in EPS production in S. sanguinis, the Congo red agar (CRA) plate test was performed. The direct analysis of the colonies formed on CRA plate allows the recognition of EPS-producing strains (Teather and Wood, 1982; Freeman et al., 1989). Results showed that there was an obvious distinction in the appearance of ΔCcpA compared to SK36. ΔCcpA produced pink colonies, whereas SK36 and CcpA + formed black colonies, suggesting that CcpA is required for EPS production in S. sanguinis (Figure 5).
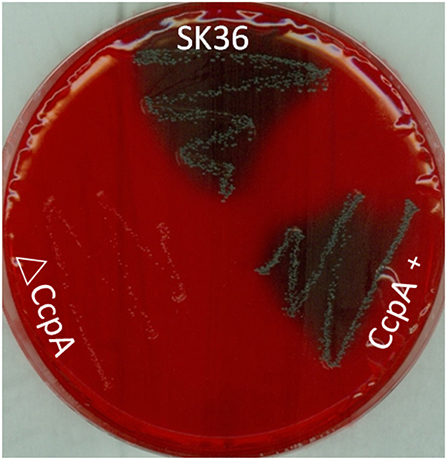
Figure 5. Detection of EPS production by CRA plate test. SK36, ΔCcpA, and CcpA+strains were inoculated on the CRA plates. Through the different color of colonies formed on the solid medium to recognize the EPS-producing strains (characterized by black colonies on the red agar) and non-EPS-producing strains (pink/red colored colonies).
CcpA Contributes to the Virulence of S. sanguinis in IE
Some studies demonstrated that disruptions in biofilm formation result in attenuation of virulence in some streptococcal species (Shenoy et al., 2017). However, the relationship between S. sanguinis biofilm formation and its pathogenicity in endocarditis was controversy, previous works have shown that there was no correlation between biofilm formation in vitro and virulence in vivo for S. sanguinis (Ge et al., 2008; Zhu et al., 2018; Baker et al., 2019). We then further probe the role CcpA plays in virulence of S. sanguinis in a rabbit infective endocarditis model. In this study, rabbits were inoculated with 1 × 109 CFU of SK36, ΔCcpA, or CcpA+ strains by intravenous. Twenty-four hours after inoculation, bacteria recovered from the blood ranged from 102 to 103 CFU per 1 ml of blood. The counts of ΔCcpA were obviously fewer than SK36 and CcpA+, P < 0.01 (Figure 6A). Three days after injection, the vegetation masses from the control group were barely observable (mean = 0.032 g), while the resulting vegetations from SK36, ΔCcpA, and CcpA+ were apparent in macroscopic lesions, the means of the vegetation masses were 0.194, 0.126, and 0.195 g, respectively, there was a significant difference between ΔCcpA with SK36 (P < 0.01; Figure 6B). Bacterial load from the vegetations per rabbit varied from 107 to 1010 CFU, and the bacterial load of ΔCcpA was reduced compared to SK36 and CcpA+ (Figure 6C). Furthermore, the bacterial load and vegetation masses were significantly correlated, R2 = 0.420, N = 36 (Figure 6D). According to this data, CcpA seems to be involved in the virulence of SK36 and CcpA+ strains.
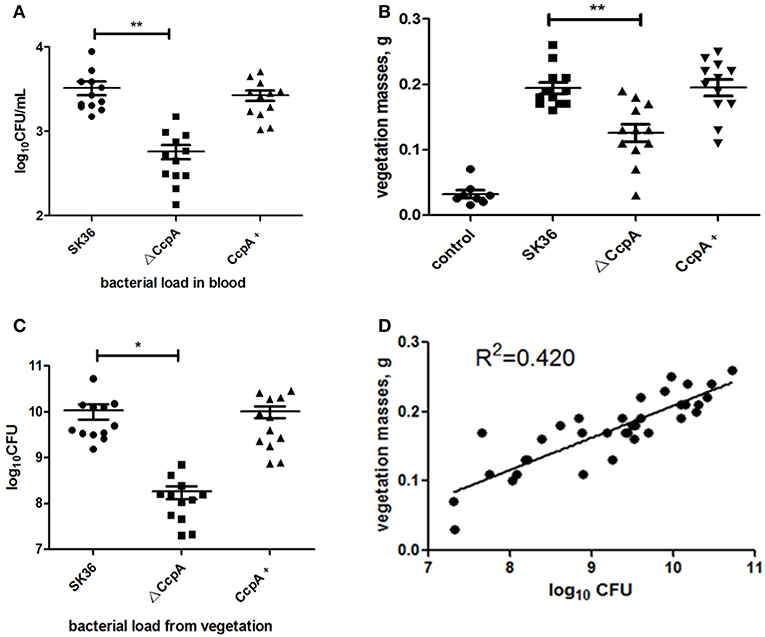
Figure 6. CcpA affects bacterial load and vegetation weight in a S. sanguinis rabbit IE model. (A) Bacterial loads in blood of the rabbit IE model were enumerated as log10 total CFU 24 h after infection. (B) Vegetation masses from the valves of each rabbit IE model were weighed 3 days after infection. (C) Bacterial loads in vegetations of the rabbit IE model were enumerated as log10 total CFU. The data presented are mean value standard error (SE). *,**Indicate the significant difference at P < 0.05 and <0.01, respectively compared to the SK36. (D) Plot represents the correlation between the vegetation bacterial load (total CFU) and vegetation mass. R2 = 0.420 (n = 36) indicated that there was a correlation between them.
Discussion
CcpA as one of the global regulators, influences the transcription of target genes by many mechanisms, but our understanding of CcpA activity and the mechanism by which CcpA exerts its regulation in S. sanguinis are limited. Here, we analyzed the transcriptional profile of S. sanguinis SK36 wild-type and its CcpA-null derivative (ΔCcpA) by RNA-seq and provided a global view of potential targets that regulator CcpA regulated. We found that 169 unigenes were significantly differentially expressed when deleted CcpA in S. sanguinis (down-regulated 85 unigenes and up-regulated 84 unigenes expression) comparing to wild type SK36 (Table 2). When we compared the DEGs of ΔCcpA mutant with Regulon of CcpA in S. sanguinis SK36 in the RegPrecise database (the Regprecise search of the S. sanguinis SK36 genome yielded 133 genes in 66 operons with potential cre-sites detected in their promoter regions), found 33 operons (60 genes) of the 66 predicted cre sites (133genes) (Supplementary File 1) that matched the 173 genes differentially expressed, 28 of the 66 predicted operons by Regprecise were not among the genes found to be differentially expressed in a CcpA mutant, and 5 of 66 the operons (SSA_1127, SSA_0219 to SSA_0224, SSA_0267, SSA_0395, SSA_1065) were not detected in this study. Here we provide the complete list of Regulon of CcpA in S. sanguinis SK36 and the genes found to be differentially expressed in a CcpA mutant (Supplementary File 3). This means that less than half of the differentially expressed genes have a cre site and may therefore be directly regulated by CcpA. In agreement with data from a previous study of S. mutans (Zeng et al., 2013) in which about half of the operons predicted by Regprecise were not among the genes found to be differentially expressed in a CcpA mutant. When referred to the literature, we also have known that only 38 of the 226 genes (17%) in S. aureus and 76 of the 124 genes (61%) in group A streptococcus (GAS) regulated by CcpA present a predicted cre in the beginning of an operon (Kinkel and McIver, 2008; Seidl et al., 2009), all these support our data. Therefore, the regulatory mechanism of CcpA is complicated and analyzing the sequence of the promoter region using prediction tools is not a desirable method to recognize potential target genes of global regulator CcpA.
Although RNA-seq is an efficient method for determining transcriptional variations between biotypes, however, in some scenarios, differences observed at the transcript level are not seen at final protein expression, reflecting the importance of post-transcriptional and post-translational influence on the protein level. Therefore, to characterize the activity of CcpA in S. sanguinis is necessary. When analyzed the down regulated DEGs, we found a subset of genes, argB, argC, argG, argH, and argJ, all of these arg genes were associated with arginine biosynthesis. Many studies have reported the association between the arginine metabolism and biofilm development in S. sanguinis (Zhu et al., 2017; Nascimento et al., 2019), Streptococcus gordonii (Robinson et al., 2018), S. aureus and S. mutans (Zhu et al., 2007; Sharma et al., 2014; Huang et al., 2017). Furthermore, another subset of regulated DEGs, comD, comEC, and comX related to the genetic competence system, can also influence biofilm formation which have been reported in S. mutans (Li et al., 2001; Aspiras et al., 2004; Perry et al., 2009). When we performed GO terms and pathway analyses Kyoto Encyclopedia of Genes and Genomes (KEGG) pathway analyzes, we found that seven pathways were the most significant pathway represented by the up-regulated DEGs, the down-regulated DEGs predominantly involved in the “Arginine and proline metabolism,” “Biosynthesis of amino acids,” and “2-Oxocarboxylic acid metabolism” pathway, providing that CcpA not only regulated carbon catabolite metabolism, but also involved in some amino acid catabolite pathways. As we all known, many pathogens have been shown that specific metabolic pathways are associated with expression of virulence genes and coordinately regulate the disease progression in vivo studies (Somarajan et al., 2014; Bauer et al., 2018; Valdes et al., 2018). Thus, we were encouraged to investigate a possible role for CcpA in biofilm formation of S. sanguinis. As expected, by microtiter plate assay, we observed that CcpA inactivation impaired biofilm formation.
Recently, some researchers demonstrated that the arginine biosynthetic genes, especially argB gene, mutation reduced polysaccharide production, resulting in the formation of a fragile biofilm in S. sanguinis (Zhu et al., 2017). Exopolysaccharides (EPS) are the primary part of the biofilm matrix, and EPS absence results in a biofilm deficient in some bacterial species (Munro and Macrina, 1993; Yang et al., 2009). However, when we looked into the DEGs, we found that the GtfP, responsible for glucan synthesis in S. sanguinis, is not in the list of the down regulated DEGs. When referred to the literature, we found that inactivating the GtfP gene did a marked reduction in the amount of water-soluble glucans in the culture supernatant, but not in the amount of water-insoluble glucans expressed on the bacterial cell surface (Yoshida et al., 2014), while the insoluble glucan is the major component of S. sanguinis biofilms reported by Bin Zhu and his coworkers (Zhu et al., 2017). Therefore, it is still necessary to characterize the role of CcpA play in EPS production in S. sanguinis. To our surprise, the result of Congo red agar (CRA) plate test showed that CcpA is indeed required for EPS production in S. sanguinis.
Some studies demonstrated that disruptions in biofilm formation result in attenuation of virulence in some streptococcal species (Shenoy et al., 2017). However, the relationship between S. sanguinis biofilm formation and its pathogenicity in endocarditis was controversy, previous works have shown that there was no correlation between biofilm formation in vitro and virulence in vivo for S. sanguinis (Ge et al., 2008; Zhu et al., 2018; Baker et al., 2019), which prompt us to further probe the role CcpA plays in virulence of S. sanguinis. BLASTP analysis of the down regulated DEGs, indicated that SSA_0684 encode fibril-like structure subunit FibA and SSA_0829 (srpA), encode platelet-binding glycoprotein, which mediates the binding of S. sanguinis to human platelets (Loukachevitch et al., 2016); SSA_0391 (spxB), encode pyruvate oxidase, involved in survival of S. sanguinis in human blood (Sumioka et al., 2017), all of these genes correlate with virulence for S. sanguinis SK36. Thus, we characterized the role of CcpA plays in virulence of S. sanguinis in a rabbit infective endocarditis model. The results showed that vegetation masses and the bacterial load from the blood and vegetations of ΔCcpA group were reduced compared to SK36 and CcpA+. In this study, we present data showing that CcpA is a global regulator involved in many metabolic processes and related to virulence, these findings will undoubtedly contribute to investigate the mechanistic links between the global regulator CcpA and the virulence of S. sanguinis.
Regarding the data from the previous report, CcpA was expressed in wild-type SK36 at different growth phases at a similar level (Chen C. et al., 2019), combined with the growth profiles of CcpA mutant, we selected the mid-logarithmic phase for transcriptome analysis, encouraged by previous studies (Lu et al., 2018; Chen C. et al., 2019). Even though we noticed that some gene expression was influenced by growth phase, for example, previous work has shown that a larger amount of SSA_2315 protein was detected from cultures at late log phase than from cultures at early log phase, suggesting that the expression of SSA_2315 was influenced by growth phase, thus missing some genes is unescapable. Maybe, that is why the SSA_2315 was not detected in this study. Furthermore, several phenotypes, including biofilm and virulence, were shown to be affected by growth phase, therefore, we still could not exclude the contribution of the slow growth of CcpA mutants to affect the phenotypes. This concern was encouraged by a study of Streptococcus pyogenes (Paluscio et al., 2018) in which they proposed the pathogen temporal regulation mode that growth/damage balance can be actively controlled by the pathogen and implicate CcpA as a master regulator of this relationship.
Data Availability Statement
Data were deposited with the Sequence Read Archive (SRA) at the National Center for Biotechnology Institute (accession PRJNA564466).
Ethics Statement
The animal study was reviewed and approved by the IACUC of the China Medical University Laboratory Animal Center.
Author Contributions
LZ and MS designed the study. YB, MS, AW, and MX were responsible for the acquisition of data. LS and LZ analyzed the experimental data. YB and LZ were the major contributors in drafting and revising the manuscript. All authors read and approved the final manuscript.
Funding
This work was supported by the National Natural Science Foundation of China (No. 81170211) from LZ.
Conflict of Interest
The authors declare that the research was conducted in the absence of any commercial or financial relationships that could be construed as a potential conflict of interest.
Supplementary Material
The Supplementary Material for this article can be found online at: https://www.frontiersin.org/articles/10.3389/fcimb.2019.00411/full#supplementary-material
References
Abranches, J., Nascimento, M. M., Zeng, L., Browngardt, C. M., Wen, Z. T., Rivera, M. F., et al. (2008). CcpA regulates central metabolism and virulence gene expression in Streptococcus mutans. J. Bacteriol. 190, 2340–2349. doi: 10.1128/JB.01237-07
Aspiras, M. B., Ellen, R. P., and Cvitkovitch, D. G. (2004). ComX activity of Streptococcus mutans growing in biofilms. FEMS Microbiol. Lett. 238, 167–174. doi: 10.1016/j.femsle.2004.07.032
Baker, S. P., Nulton, T. J., and Kitten, T. (2019). Genomic, phenotypic, and virulence analysis of Streptococcus sanguinis oral and infective-endocarditis isolates. Infect. Immun. 87:e00703–18. doi: 10.1128/IAI.00703-18
Bauer, R., Mauerer, S., and Spellerberg, B. (2018). Regulation of the beta-hemolysin gene cluster of Streptococcus anginosus by CcpA. Sci. Rep. 8:9028. doi: 10.1038/s41598-018-27334-z
Bischoff, M., Wonnenberg, B., Nippe, N., Nyffenegger-Jann, N. J., Voss, M., Beisswenger, C., et al. (2017). CcpA affects infectivity of Staphylococcus aureus in a hyperglycemic environment. Front. Cell Infect. Microbiol. 7:172. doi: 10.3389/fcimb.2017.00172
Boyle, E. I., Weng, S., Gollub, J., Jin, H., Botstein, D., Cherry, J., et al. (2004). GO::TermFinder–open source software for accessing gene ontology information and finding significantly enriched Gene Ontology terms associated with a list of genes. Bioinformatics 20, 3710–3715. doi: 10.1093/bioinformatics/bth456
Chen, C., Wang, L., Lu, Y., Yu, H., and Tian, H. (2019). Comparative transcriptional analysis of Lactobacillus plantarum and its ccpA-knockout mutant under galactooligosaccharides and glucose conditions. Front. Microbiol. 10:1584. doi: 10.3389/fmicb.2019.01584
Chen, Y. M., Chiang, Y. C., Tseng, T. Y., Wu, H., Chen, Y. Y., Wu, C. H., et al. (2019). Molecular and functional analysis of the type IV pilus gene cluster in Streptococcus sanguinis SK36.” Appl. Environ. Microbiol. 85:e02788–18. doi: 10.1128/AEM.02788-18
Costerton, J. W., Stewart, P. S., and Greenberg, E. P. (1999). Bacterial biofilms: a common cause of persistent infections. Science 284, 1318–1322. doi: 10.1126/science.284.5418.1318
Douglas, L. J. (2003). Candida biofilms and their role in infection. Trends Microbiol. 11, 30–36. doi: 10.1016/S0966-842X(02)00002-1
Fan, J., Zhang, Y., Chuang-Smith, O. N., Frank, K. L., Guenther, B. D., Kern, M., et al. (2012). Ecto-5'-nucleotidase: a candidate virulence factor in Streptococcus sanguinis experimental endocarditis. PLoS ONE 7:e38059. doi: 10.1371/journal.pone.0038059
Flemming, H. C., and Wingender, J. (2010). The biofilm matrix. Nat. Rev. Microbiol. 8, 623–633. doi: 10.1038/nrmicro2415
Freeman, D. J., Falkiner, F. R., and Keane, C. T. (1989). New method for detecting slime production by coagulase negative staphylococci. J. Clin. Pathol. 42, 872–874. doi: 10.1136/jcp.42.8.872
Ge, X., Kitten, T., Chen, Z., Lee, S. P., Munro, C., and Xu, P. (2008). Identification of Streptococcus sanguinis genes required for biofilm formation and examination of their role in endocarditis virulence. Infect. Immun. 76, 2551–2559. doi: 10.1128/IAI.00338-08
Giammarinaro, P., and Paton, J. C. (2002). Role of RegM, a homologue of the catabolite repressor protein CcpA, in the virulence of Streptococcus pneumoniae. Infect. Immun. 70, 5454–5461. doi: 10.1128/IAI.70.10.5454-5461.2002
Huang, X., Zhang, K., Deng, M., Exterkate, R. A. M., Liu, C., Zhou, X., et al. (2017). Effect of arginine on the growth and biofilm formation of oral bacteria. Arch. Oral Biol. 82, 256–262. doi: 10.1016/j.archoralbio.2017.06.026
Kanehisa, M., Goto, S., Sato, Y., Furumichi, M., and Tanabe, M. (2012). KEGG for integration and interpretation of large-scale molecular data sets. Nucleic Acids Res. 40, D109–D114. doi: 10.1093/nar/gkr988
Kinkel, T. L., and McIver, K., S. (2008). CcpA-mediated repression of streptolysin S expression and virulence in the group A streptococcus. Infect. Immun. 76, 3451–3463. doi: 10.1128/IAI.00343-08
Lang, X., Wan, Z., Pan, Y., Wang, X., Wang, X., Bu, Z., et al. (2015). Investigation into the role of catabolite control protein A in the metabolic regulation of Streptococcus suis serotype 2 using gene expression profile analysis. Exp. Ther. Med. 10, 127–132. doi: 10.3892/etm.2015.2470
Leiba, J., Hartmann, T., Cluzel, M. E., Cohen-Gonsaud, M., Delolme, F., Bischoff, M., et al. (2012). A novel mode of regulation of the Staphylococcus aureus catabolite control protein A (CcpA) mediated by Stk1 protein phosphorylation. J. Biol. Chem. 287, 43607–43619. doi: 10.1074/jbc.M112.418913
Li, Y. H., Lau, P. C., Lee, J. H., Ellen, R., and Cvitkovitch, D. G. (2001). Natural genetic transformation of Streptococcus mutans growing in biofilms. J. Bacteriol. 183, 897–908. doi: 10.1128/JB.183.3.897-908.2001
Loukachevitch, L. V., Bensing, B. A., Yu, H., Zeng, J., Chen, X., Sullam, P. M., et al. (2016). Structures of the Streptococcus sanguinis SrpA binding region with human sialoglycans suggest features of the physiological ligand. Biochem. 55, 5927–5937. doi: 10.1021/acs.biochem.6b00704
Lu, Y., Song, S., Tian, H., Yu, H., Zhao, J., and Chen, C. (2018). Functional analysis of the role of CcpA in Lactobacillus plantarum grown on fructooligosaccharides or glucose: a transcriptomic perspective. Microb. Cell Fact. 17:201. doi: 10.1186/s12934-018-1050-4
Mathur, T., Singhal, S., Khan, S., Upadhyay, D. J., Fatma, T., and Rattan, A. (2006). Detection of biofilm formation among the clinical isolates of Staphylococci: an evaluation of three different screening methods. Indian J. Med. Microbiol. 24, 25–29. doi: 10.4103/0255-0857.19890
Moser, C., Pedersen, H. T., Lerche, C. J., Kolpen, M., Line, L., et al. (2017). Biofilms and host response - helpful or harmful. APMIS 125, 320–338. doi: 10.1111/apm.12674
Munro, C. L., and Macrina, F. L. (1993). Sucrose-derived exopolysaccharides of Streptococcus mutans V403 contribute to infectivity in endocarditis. Mol. Microbiol. 8, 133–142. doi: 10.1111/j.1365-2958.1993.tb01210.x
Nascimento, M. M., Alvarez, A. J., Huang, X., Hanway, S., Perry, S., Luce, A., et al. (2019). Arginine metabolism in supragingival oral biofilms as a potential predictor of caries risk. JDR Clin. Trans. Res. 4, 262–270. doi: 10.1177/2380084419834234
Ogawa, K., and Hatano, M. (1978). Circular dichroism of the complex of a (1-3)-β-D-glucan with Congo Red. Carbohydr. Res. 527–535. doi: 10.1016/S0008-6215(00)84144-0
Paluscio, E., Watson, M. E. Jr, and Caparon, M. G. (2018). CcpA coordinates growth/damage balance for Streptococcus pyogenes pathogenesis. Sci. Rep. 8:14254. doi: 10.1038/s41598-018-32558-0
Parker, M. T., and Ball, L. C. (1976). Streptococci and aerococci associated with systemic infection in man. J. Med. Microbiol. 9, 275–302. doi: 10.1099/00222615-9-3-275
Parsek, M. R., and Singh, P. K. (2003). Bacterial biofilms: an emerging link to disease pathogenesis. Annu. Rev. Microbiol. 57, 677–701. doi: 10.1146/annurev.micro.57.030502.090720
Perry, J. A., Cvitkovitch, D. G., and Levesque, C. M. (2009). Cell death in Streptococcus mutans biofilms: a link between CSP and extracellular DNA. FEMS Microbiol. Lett. 299, 261–266. doi: 10.1111/j.1574-6968.2009.01758.x
Ramirez-Ronda, C. H. (1978). Adherence of glucan-positive and glucan-negative streptococcal strains to normal and damaged heart valves. J. Clin. Invest. 62, 805–814. doi: 10.1172/JCI109192
Redanz, S., Masilamani, R., Cullin, N., Giacaman, R. A., Merritt, J., and Kreth, J. (2018). Distinct regulatory role of carbon catabolite protein A (CcpA) in oral streptococcal spxB expression. J. Bacteriol. 200:e00619–17. doi: 10.1128/JB.00619-17
Robinson, J. C., Rostami, N., Casement, J., Vollmer, W., Rickard, A. H., and Jakubovics, N. S. (2018). ArcR modulates biofilm formation in the dental plaque colonizer Streptococcus gordonii. Mol. Oral Microbiol. 33, 143–154. doi: 10.1111/omi.12207
Seidl, K., Muller, S., Francois, P., Kriebitzsch, C., Schrenzel, J., Engelmann, S., et al. (2009). Effect of a glucose impulse on the CcpA regulon in Staphylococcus aureus. BMC Microbiol. 9:95. doi: 10.1186/1471-2180-9-95
Sharma, S., Lavender, S., Woo, J., Guo, L., Shi, W., Kilpatrick-Liverman, L., et al. (2014). Nanoscale characterization of effect of L-arginine on Streptococcus mutans biofilm adhesion by atomic force microscopy. Microbiology 160(Pt 7), 1466–1473. doi: 10.1099/mic.0.075267-0
Shenoy, A. T., Brissac, T., Gilley, R. P., Kumar, N., Wang, Y., Gonzalez-Juarbe, N., et al. (2017). Streptococcus pneumoniae in the heart subvert the host response through biofilm-mediated resident macrophage killing. PLoS Pathog. 13:e1006582. doi: 10.1371/journal.ppat.1006582
Skov Sorensen, U. B., Yao, K., Yang, Y., Tettelin, H., and Kilian, M. (2016). Capsular polysaccharide expression in commensal streptococcus species: genetic and antigenic similarities to Streptococcus pneumoniae. MBio 7:e01844–16. doi: 10.1128/mBio.01844-16
Somarajan, S. R., Roh, J. H., Singh, K. V., Weinstock, G., and Murray, B. E. (2014). CcpA is important for growth and virulence of Enterococcus faecium. Infect. Immun. 82, 3580–3587. doi: 10.1128/IAI.01911-14
Sumioka, R., Nakata, M., Okahashi, N., Li, Y., Wada, S., Yamaguchi, M., et al. (2017). Streptococcus sanguinis induces neutrophil cell death by production of hydrogen peroxide. PLoS One 12:e0172223. doi: 10.1371/journal.pone.0172223
Teather, R. M., and Wood, P. J. (1982). Use of Congo red-polysaccharide interactions in enumeration and characterization of cellulolytic bacteria from the bovine rumen. Appl. Environ. Microbiol. 43, 777–780.
Valdes, K. M., Sundar, G. S., Belew, A. T., Islam, E., El-Sayed, N. M., Le Breton, Y., et al. (2018). Glucose levels alter the Mga virulence regulon in the group A streptococcus. Sci. Rep. 8:4971. doi: 10.1038/s41598-018-23366-7
Vogkou, C. T., Vlachogiannis, N. I., Palaiodimos, L., and Kousoulis, A. A. (2016). The causative agents in infective endocarditis: a systematic review comprising 33,214 cases. Eur. J. Clin. Microbiol. Infect. Dis. 35, 1227–1245. doi: 10.1007/s10096-016-2660-6
Weickert, M. J., and Chambliss, G. H. (1990). Site-directed mutagenesis of a catabolite repression operator sequence in Bacillus subtilis. Proc. Natl. Acad. Sci. U.S.A. 87, 6238–6242. doi: 10.1073/pnas.87.16.6238
Willenborg, J., de Greeff, A., Jarek, M., Valentin-Weigand, P., and Goethe, R. (2014). The CcpA regulon of Streptococcus suis reveals novel insights into the regulation of the streptococcal central carbon metabolism by binding of CcpA to two distinct binding motifs. Mol. Microbiol. 92, 61–83. doi: 10.1111/mmi.12537
Willenborg, J., Fulde, M., de Greeff, A., Rohde, M., Smith, H. E., Valentin-Weigand, P., et al. (2011). Role of glucose and CcpA in capsule expression and virulence of Streptococcus suis. Microbiology 157(Pt 6), 1823–1833. doi: 10.1099/mic.0.046417-0
Xu, D. Q., Thompson, J., and Cisar, J. O. (2003). Genetic loci for coaggregation receptor polysaccharide biosynthesis in Streptococcus gordonii 38. J. Bacteriol. 185, 5419–5430. doi: 10.1128/JB.185.18.5419-5430.2003
Yang, J., Ritchey, M., Yoshida, Y., Bush, C. A., and Cisar, J. (2009). Comparative structural and molecular characterization of ribitol-5-phosphate-containing Streptococcus oralis coaggregation receptor polysaccharides. J. Bacteriol. 191, 1891–1900. doi: 10.1128/JB.01532-08
Yoshida, Y., Konno, H., Nagano, K., Abiko, Y., Nakamura, Y., Tanaka, Y., et al. (2014). The influence of a glucosyltransferase, encoded by gtfP, on biofilm formation by Streptococcus sanguinis in a dual-species model. APMIS 122, 951–960. doi: 10.1111/apm.12238
Zeng, L., Choi, S. C., Danko, C. G., Siepel, A., Stanhope, M. J., and Burne, R. A. (2013). Gene regulation by CcpA and catabolite repression explored by RNA-Seq in Streptococcus mutans. PLoS ONE 8:e60465. doi: 10.1371/journal.pone.0060465
Zheng, L., Chen, Z., Itzek, A., Ashby, M., and Kreth, J. (2011). Catabolite control protein A controls hydrogen peroxide production and cell death in Streptococcus sanguinis. J Bacteriol 193, 516–526. doi: 10.1128/JB.01131-10
Zheng, L., Chen, Z., Itzek, A., Herzberg, M. C., and Kreth, J. (2012). CcpA regulates biofilm formation and competence in Streptococcus gordonii. Mol. Oral Microbiol. 27, 83–94. doi: 10.1111/j.2041-1014.2011.00633.x
Zhu, B., Ge, X., Stone, V., Kong, X., El-Rami, F., Liu, Y., et al. (2017). ciaR impacts biofilm formation by regulating an arginine biosynthesis pathway in Streptococcus sanguinis SK36. Sci. Rep. 7:17183. doi: 10.1038/s41598-017-17383-1
Zhu, B., Macleod, L. C., Kitten, T., and Xu, P. (2018). Streptococcus sanguinis biofilm formation & interaction with oral pathogens. Future Microbiol. 13, 915–932. doi: 10.2217/fmb-2018-0043
Zhu, Y., Weiss, E. C., Otto, M., Fey, P. D., Smeltzer, M. S., and Somerville, G. A. (2007). Staphylococcus aureus biofilm metabolism and the influence of arginine on polysaccharide intercellular adhesin synthesis, biofilm formation, and pathogenesis. Infect. Immun. 75, 4219–4226. doi: 10.1128/IAI.00509-07
Keywords: CcpA, RNA-seq, DEGs, EPS, biofilm, Streptococcus sanguinis
Citation: Bai Y, Shang M, Xu M, Wu A, Sun L and Zheng L (2019) Transcriptome, Phenotypic, and Virulence Analysis of Streptococcus sanguinis SK36 Wild Type and Its CcpA-Null Derivative (ΔCcpA). Front. Cell. Infect. Microbiol. 9:411. doi: 10.3389/fcimb.2019.00411
Received: 12 August 2019; Accepted: 19 November 2019;
Published: 04 December 2019.
Edited by:
Michael J. Federle, University of Illinois at Chicago, United StatesReviewed by:
Lin Zeng, University of Florida, United StatesPing Xu, Virginia Commonwealth University, United States
Copyright © 2019 Bai, Shang, Xu, Wu, Sun and Zheng. This is an open-access article distributed under the terms of the Creative Commons Attribution License (CC BY). The use, distribution or reproduction in other forums is permitted, provided the original author(s) and the copyright owner(s) are credited and that the original publication in this journal is cited, in accordance with accepted academic practice. No use, distribution or reproduction is permitted which does not comply with these terms.
*Correspondence: Lanyan Zheng, bHl6aGVuZyYjeDAwMDQwO2NtdS5lZHUuY24=
†These authors have contributed equally to this work