- 1Laboratory of Microbial Biotechnology, Institute of Fundamental Medicine and Biology, Kazan (Volga region) Federal University, Kazan, Russia
- 2Laboratory of Extreme Biology, Institute of Fundamental Medicine and Biology, Kazan (Volga region) Federal University, Kazan, Russia
Morganella morganii is an opportunistic bacterial pathogen shown to cause a wide range of clinical and community-acquired infections. This study was aimed at sequencing and comparing the genomes of three M. morganii strains isolated from the urine samples of patients with community-acquired urinary tract infections. Draft genome sequencing was conducted using the Illumina HiSeq platform. The genomes of MM 1, MM 4, and MM 190 strains have a size of 3.82–3.97 Mb and a GC content of 50.9–51%. Protein-coding sequences (CDS) represent 96.1% of the genomes, RNAs are encoded by 2.7% of genes and pseudogenes account for 1.2% of the genomes. The pan-genome containes 4,038 CDS, of which 3,279 represent core genes. Six to ten prophages and 21–33 genomic islands were identified in the genomes of MM 1, MM 4, and MM 190. More than 30 genes encode capsular biosynthesis proteins, an average of 60 genes encode motility and chemotaxis proteins, and about 70 genes are associated with fimbrial biogenesis and adhesion. We determined that all strains contained urease gene cluster ureABCEFGD and had a urease activity. Both MM 4 and MM 190 strains are capable of hemolysis and their activity correlates well with a cytotoxicity level on T-24 bladder carcinoma cells. These activities were associated with expression of RTX toxin gene hlyA, which was introduced into the genomes by a phage similar to Salmonella phage 118970_sal4.
Introduction
Morganella morganii is a gram-negative bacterium, which is a common inhabitant of the environment and intestinal tracts of humans, mammals, and reptiles (Nakao et al., 2013; Lin et al., 2015). It is also an important opportunistic pathogen, which causes a wide range of clinical and community-acquired infections (Liu et al., 2016).
Genus Morganella, similar to genera Proteus and Providencia, belongs to the Proteeae tribe (O'Hara et al., 2000), and includes species such as M. morganii and M. psychrotolerans (Emborg et al., 2006). According to the modern classification, Morganella is a type genus of a novel Morganellaceae family. This family consists of the following 8 genera: Arsenophonus, Cosenzaea, Moellerella, Morganella, Photorhabdus, Proteus, Providencia, and Xenorhabdus (Adeolu et al., 2016).
In spite of its relatively rare occurrence in clinical isolates, M. morganii is a frequent cause of urinary tract infections (UTIs), septicemia, and wound infections (Chen et al., 2012). M. morganii has occasionally been associated with pathologies of diverse localizations such as a brain abscess (Abdalla et al., 2006; Patil et al., 2012), liver abscess (Hakyemez et al., 2012; Ponte and Costa, 2015), chorioamnionitis (Liu et al., 2016), peritonitis (Atalay et al., 2010; Tsai et al., 2013), pericarditis (Nakao et al., 2013), septic arthritis (Schonwetter and Orson, 1988), rhabdomyolysis (Imataki and Uemura, 2017), necrotizing fasciitis following snakebites (Mao et al., 2016; Tsai et al., 2017), bilateral keratitis (Zhang et al., 2017), neonatal sulfhemoglobinemia (Murphy et al., 2015), and even non-clostridial gas gangrene (Ghosh et al., 2009). Erlanger et al. (2017) reported that M. morganii bacteremia cases are more commonly accompanied by complications or by fatal consequences in comparison to Escherichia coli.
UTIs are one of the most socially significant diseases. About 60% of women are estimated to acquire UTI at least once in their lifetime (Hilbert et al., 2012; Lüthje and Brauner, 2014). Although the main causative agents of UTIs are E. coli, Klebsiella pneumoniae, Staphylococcus saprophyticus, and Enterococcus spp. (Foxman, 2010; Flores-Mireles et al., 2015; Waller et al., 2018), M. morganii is often isolated from patients with long-term urinary catheters (Stickler, 2008, 2014). It is known that uropathogens cause infections by synthesizing a complex of virulence factors: different toxins and proteases (Hilbert et al., 2012; Welch, 2016), type I fimbriae and P-fimbriae (Kalita et al., 2014), flagella (Wiles et al., 2008), siderophores (Lüthje and Brauner, 2014), ureases (Mobley and Warren, 1987) etc. The majority of virulence factors from uropathogenic E. coli (UPEC) are encoded by different pathogenicity islands (PAI) (Hannan et al., 2008). Among them is a pore-forming toxin α-hemolysin (HlyA), which is expressed by more than 50% of UPEC strains and plays an important role in pathogenesis of cystitis and pyelonephritis (Ristow and Welch, 2016). HlyA is encoded by hlyCABD operon, which can be located on the chromosome or plasmids (Oloomi and Bouzari, 2008). α-hemolysin activity leads to ATP release and lysis of erythrocytes, lymphocytes, leukocytes, epithelial and endothelial cells of human, and other mammals (Skals et al., 2014; Chenal et al., 2015). For example, this toxin promotes NLRP3 inflammasome-mediated IL-1β release and death of human macrophages (MV Murthy et al., 2018). Although HlyA has been well-studied, some aspects of its mechanism of action and function in vivo presently remain unclear (Ristow and Welch, 2016). In addition, it was recently reported that about 60% of secreted HlyA can be associated to outer membrane vesicles, which was 10 fold more active than purified free toxin (Herlax et al., 2010; Thomas and Wigneshweraraj, 2014). It is known that more than 50% of clinical isolates of M. morganii have a hemolytic activity (Gołuszko et al., 1988; Kim et al., 2007). In 1987, Koronakis et al. (1987) reported that the genetic determinant encoding M. morganii secreted hemolysin was related to hlyA of E. coli. M. morganii hemolysin possesses similar properties to α-hemolysin, being calcium-dependent, having similar structure and function, and causing death of erythrocytes and polymorphonuclear leucocytes (Senior and Hughes, 1988; Eberspächer et al., 1990). Moreover, infection by hemolytic M. morganii strains has been proven to be lethal for mice (Emödy et al., 1982) and immunosuppressed humans (Kim et al., 2007). Consequently, secreted hemolysin remains a major virulence factor of M. morganii.
Numerous virulence-related genes have been identified in the annotated genomes of different M. morganii strains (Chen et al., 2012; Liu et al., 2016). As of January 2019, 55 sequenced genomes of M. morganii have been deposited in the Genome database, 11 of which were fully sequenced. The size of the sequenced M. morganii genomes averages at 3.99 Mb, with a GC content varying from 50.1 to 51.4%. However, to date, a detailed genome analysis has been carried out for only a few strains of M. morganii (KT, F675, and INSRALV892a) (Chen et al., 2012; Olaitan et al., 2014; Jones-Dias et al., 2016). Notwithstanding, none of them were isolated from urine.
Understanding the molecular characteristics of M. morganii will facilitate identification of subtle differences in the genome and pathogenicity characteristics. Here we report the genome sequences and comparative genome analysis of three M. morganii strains isolated from urine in UTI cases, exhibiting variances in hemolytic properties. Comparative genomic analyses which included major genome features, genomic similarities and differences among isolates, determination of the pan- and core genomes, characterization of functional gene categories using the cluster of orthologous genes (COGs) classification, and prediction of genomic islands, virulence factors, and prophages were also performed.
Materials and Methods
Isolation and Cultivation of Bacterial Strains
The M. morganii strains (MM 1, MM 4, MM 190) were isolated from the urine of a 59-year-old man, 35-year-old woman, and a 3-year-old boy, respectively, who had community-acquired urinary tract infections. Samples for MM 1 and MM 4 were collected in October 2014, whereas that of MM 190 was taken in June 2015 by the Clinical Diagnostic Laboratory Biomed (Kazan, Republic of Tatarstan, Russia). Identification of the strains was performed based on microbiological tests and mass spectrometry of protein profiles on MALDI Bio Typer (Bruker Daltonik, Germany). The Score Value >2.5 was observed in all cases, indicating a highly probable species identification. Ethical approval was not required as clinical isolates were collected and stored as part of routine clinical care.
For bacterial cultivation, LB (Lysogeny broth and Lysogeny agar) medium (1% tryptone, 0.5% yeast extract, 0.5% NaCl, pH 8.5) was used. Bacteria were grown at 37°C with aeration (Shaker Braun, Germany). The absorbance of bacterial cultures was measured at 590 nm using XMark Microplate Spectrophotometer (BioRad, Singapore).
Hemolysis Assay
The hemolytic activity was investigated on blood agar containing 5% human erythrocytes. Plates were incubated for 24 h at 37°C. The hemolytic activity was evaluated by measuring the diameter of clear zones around the colonies, and the specific type of hemolysis (α, β, or γ) was determined by the morphology of the hemolysis zone.
To assess the accumulation of hemolysins in the medium, a 2% suspension of human erythrocytes in 0.85% NaCl was used (Senior and Hughes, 1988). LB medium was inoculated by an overnight culture to attain a 1% bacterial suspension, and incubated with aeration at 37°C. Every hour, 500 μl of culture were pelleted by centrifugation (5 min, 8,000 rpm) and the supernatant was collected in a clean tube. Next, the bacterial culture fluid was mixed with a 2% suspension of washed human erythrocytes (with 20 mM CaCl2) in a ratio of 9:1. Following a 30 min incubation at 37°C, samples were centrifuged at 11,600 rpm for 1 min to pellet the cells. Release of hemoglobin was determined by measuring absorbance of supernatant at 540 nm. Hemolysis level was calculated by the formula below as previously described (Rattanama et al., 2012):
As negative and positive controls, a fresh culture medium without bacteria and distilled water were used, respectively.
Cytotoxicity Assay
Human bladder carcinoma T-24 cells obtained from the Russian Cell Culture Collection (Institute of Cytology, St. Petersburg, Russia) were used as target cells for cytotoxicity assay. T-24 cells were seeded into 12-well tissue culture plates at 2 × 105 cells per well in α-MEM medium with L-glutamine and without antibiotics (Biolot, Russia) containing 10% fetal bovine serum (Hyclone, USA). Cells were grown for 24 h at 37°C in 5% CO2. Overnight bacterial culture was pelleted, resuspended in a fresh α-MEM and added to T-24 cells to a multiplicity of infection (MOI) of about 50 bacteria per cell. Plates were incubated for 2 h at 37°C in 5% CO2, then α-MEM with bacteria were removed, wells were washed twice with PBS (Sigma-Aldrich, USA) and stained by 0.2% trypan blue for 5 min. Cytotoxicity of M. morganii strains was estimated by the number of the dead cells on an inverted microscope Axio Vert A1 (Carl Zeiss, Germany).
Genome Sequencing
Genomic DNA of M. morganii strains MM 1, MM 4, and MM 190 was extracted by phenol chloroform method (Wright et al., 2017). The quantity and quality control of extracted DNA was evaluated using a NanoPhotometer P 300 (Implen, Germany) and by electrophoresis on a 1% agarose gel, respectively. DNA fragmentation was carried out using Covaris S220 (duty factor-5%, cycles per burst-200, times-105 s). Library construction was performed with a NEBNext Ultra II DNA Library Prep Kit (New England Biolabs). To check the quality of DNA fragmentation and DNA library preparation, a 2100 Bioanalyzer (Agilent Technologies, USA) and a High Sensitivity DNA kit (Agilent Technologies, USA) were used. Genome sequencing was carried out at KFU-RIKEN Laboratory (Kazan Federal University, Kazan, Republic of Tatarstan, Russia) on Illumina HiSeq2500 platform (Illumina, USA) with a 251-bp paired-end library.
Genome Assembly, Annotation and Comparative Analysis
The raw reads of the genomes were analyzed by FastQC. To remove low-quality reads, Trimmomatic (v. 0.36) was used (Bolger et al., 2014). Assembly of processed reads was performed by SPAdes (v. 3.10.0.) (Bankevich et al., 2012), and the quality of the assembly was evaluated by Quast (Gurevich et al., 2013). To improve the quality of assembly, all reads <500 bp long and <2.0 coverage were eliminated. Codes used to pre-process and assemble the genomes can be found in Supplementary Material.
The genomes were annotated using the NCBI Prokaryotic Genomes Annotation Pipeline (Angiuoli et al., 2008). For functional annotation RAST (annotation scheme: ClassicRAST) was used (Aziz et al., 2008). Search for closely related strains was carried out using JSpeciesWS (Richter et al., 2016). To align the draft genomes relative to the reference, MeDuSa scaffolder was used (Bosi et al., 2015). Genome sequences circular comparison was performed by BRIG (Alikhan et al., 2011), BLAST tools were used for sequence alignment. To calculate the pan-genome and core-genome, and Venn diagram construction, EDGAR 2.0 was used (Blom et al., 2016). Genome loci were analyzed using ASAP (Glasner et al., 2003), Easyfig (Sullivan et al., 2011), and MAUVE (Darling et al., 2004). Phage regions were identified by PHASTER (Arndt et al., 2016) and analyzed using Virus-Host DB (Mihara et al., 2016). Pathogenicity islands were found by IslandViewer4 (Bertelli et al., 2017). GC content of distinct sequences was calculated using GC Content Calculator (https://jamiemcg.github.io/bioinf/gc.html).
Nucleotide Sequence Accession Numbers
The draft genome sequences of M. morganii strains were deposited in the DDBJ/EMBL/GenBank under the accession numbers QUOO00000000, QPLM00000000, and QMKL00000000 for MM 1, MM 4, and MM 190, respectively. The versions described in this paper are versions QUOO01000000, QPLM01000000, and QMKL01000000. Additionally, the raw reads were deposited in the SRA database under the BioProject accession numbers PRJNA484881, PRJNA482068, and PRJNA478302 for MM 1, MM 4, and MM 190, respectively.
Results and Discussion
General Features of M. morganii Strains Genomes
As a result of genome sequencing, a total of 2,765,361, 2,968,804, and 2,933,802 reads for M. morganii MM 1, MM 4, and MM 190 were generated. These reads have been cleaned and assembled into 21, 36, and 63 scaffolds for MM 1, MM 4, and MM 190, respectively (Table 1).
The genomes were annotated using the NCBI Prokaryotic Genomes Annotation Pipeline (Table 2). The three M. morganii strains have a genome size of 3.82–3.97 Mb with an average GC content of 51%, which correlates well with properties of other annotated M. morganii genomes (https://www.ncbi.nlm.nih.gov/genome/genomes/10874?). About 4,000 genes were identified in the genomes, 96.1% of them were the protein-coding sequences (CDSs), 2.7% encoded RNAs, and 1.2% represented pseudogenes. We have determined that the genome size of M. morganii MM 190 was the largest among the three strains. In MM 190, we documented 3,968,545 bp-long genome with a GC content of 50.9%, containing 3,907 predicted genes. Of them, 3,745 were identified as protein-coding sequences, while 33, 75, and 4 genes encoded rRNAs, tRNAs, and noncoding RNAs, respectively. In addition, 50 genes were found to be pseudogenes. In contrast, MM 1 and MM 4 genomes consisted of 3,882,881 bp and 3,815,979 bp, respectively, with a GC content of 51.0% for both. 3,726 and 3,685 genes were annotated for MM 1 and MM 4, of which 3,587 and 3,548 were identified as CDSs, 74 and 73 as tRNA genes, 40 and 45 as pseudogenes, whereas 21 and 15 coded for rRNAs.
Comparative genome analysis of many bacterial species have demonstrated significant intra-species genome variabilities (Tettelin et al., 2008). Thus, to obtain a general assessment of the gene repertoire for studied species, it is essential to calculate the pan-genome which represents the total number of non-redundant genes, as well as core genome, which includes genes shared by all strains and encodes proteins necessary for basic biological functions. Using the EDGAR platform, we found that the pan-genome of M. morganii isolates comprises 4,038 genes. The core genome of MM 1, MM 4, and MM 190 strains contained a total of 3,279 protein-coding genes (Figure 1). MM 1 shared 40 and 45 genes with MM 190 and MM 4, respectively. In contrast, MM 190 and MM 4 had 179 common sequences. 221, 30, and 244 unique genes were identified in the genomes of MM 1, MM 4, and MM 190, majority of which encoded hypothetical and phage-associated proteins. We likewise calculated the pan- and core-genome of the studied strains and eleven M. morganii strains with whole-genome sequences, which had been deposited in the Genome database as of January 2019 (Supplementary Table 1, Supplementary Figure 1). The 14 genomes of M. morganii have a pan-genome consisting of 7,152 CDS and a core-genome containing 2,826 protein-coding genes.
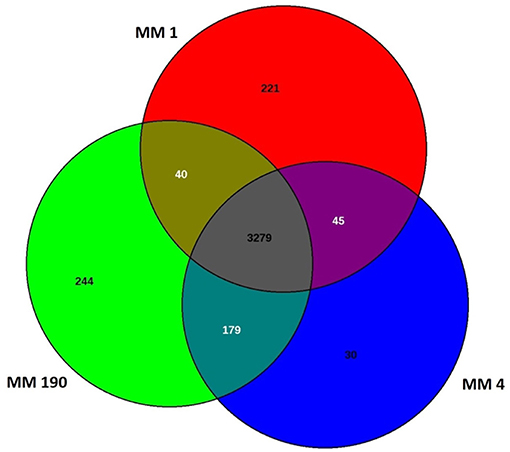
Figure 1. Venn diagram showing the number of shared and unique protein-coding sequences in identified M. morganii strains.
Genome Neighbor Identification
To find the closest genomes of M. morganii MM 1, MM 4, and MM 190 strains, we considered 11 fully sequenced genomes of M. morganii, which were described at the previous section (Figure 2). Using JSpeciesWS, we determined that M. morganii FDAARGOS_63 (CP026046) was the closest neighbor of MM 1 strain, since they shared a 98.98% similarity. Conversely, MM 4 (99.92%) and MM 190 (99.91%) showed maximum homology with the M. morganii FDAARGOS_172 strain (CP014026). We likewise discovered that M. morganii KT (CP004345), which had been used as a reference strain in most previous studies, had 98.58, 99.88, and 99.89% identity with MM 1, MM 4, and MM 190, respectively. It should be noted that, while M. morganii FDAARGOS_172 was also obtained from urine of patient with UTI, similar to the strains we identified, the isolation source of M. morganii KT was human blood (Chen et al., 2012). However, M. morganii KT was the closest neighbor for the studied strains, when they were analyzed as a whole. Therefore, M. morganii KT was used as a reference in the subsequent genome analysis.
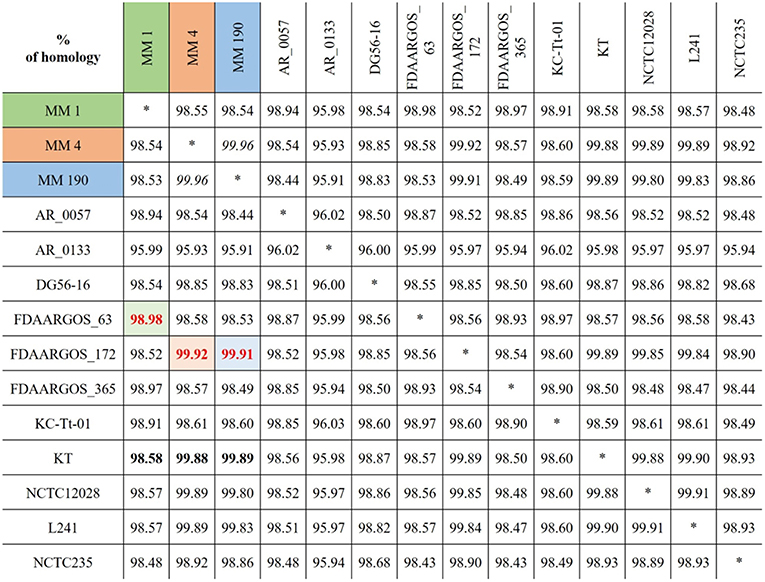
Figure 2. Alignment results for the genomes of identified M. morganii strains using JSpeciesWS (January, 2019).
We next performed multiple genome alignment of the M. morganii strains in relation to M. morganii KT using MAUVE and BRIG (Figures 3A,B). MAUVE analysis determined that all genomes shared high content of homologous regions, however, certain loci peculiar to the M. morganii KT genome were also identified (Figure 3A). Circular-comparison of M. morganii genomes by BRIG showed that MM 1, MM 4, and MM 190 contained similar regions, which differed from the KT strain (Figure 3B). Thus, it is possible that MM 1, MM 4, and MM 190 could have a similar origin.
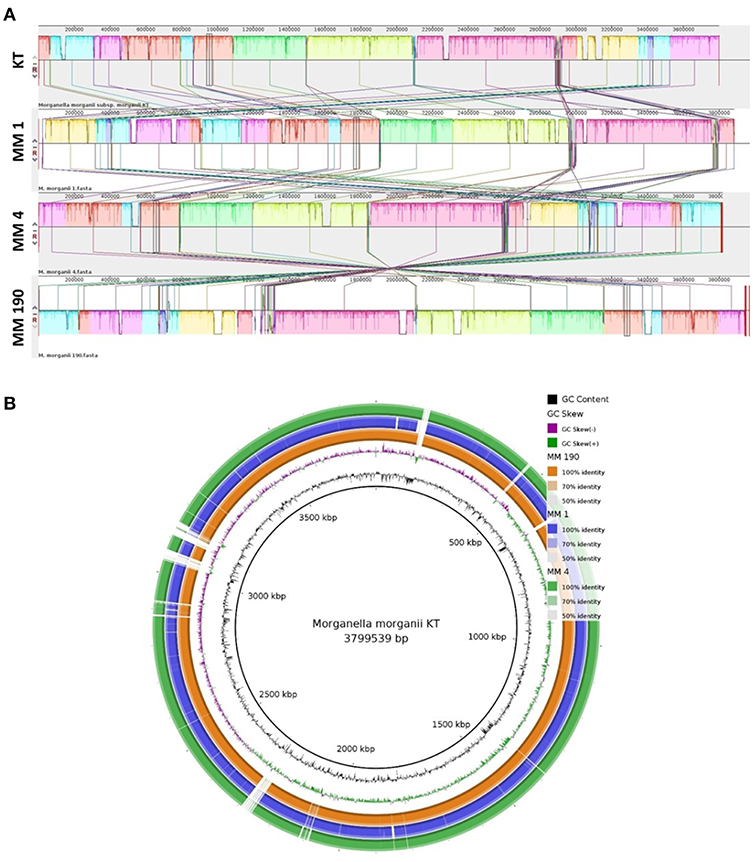
Figure 3. Multiple genome alignment of the genomes of M. morganii MM 1, MM 4, and MM 190 strains. The genome of M. morganii KT was used as a reference. (A) Genome alignment by MAUVE. The highly homologous regions are indicated by identical colors. Each block represents a similarity profile of the genome sequence. Height of profile indicates the genomic conservation level inside each block. White areas specify the unique sequences of the genome. (B) Circular genomic maps of M. morganii strains obtained using BRIG. Color saturation indicates the homology rate, blanks show the absence of similarity.
Functional Annotation of Genomes
Coding Sequences
For detailed analysis of the genomes, the RAST annotation server was used. We found that about 40% of genes annotated by this server do not belong to any of the subsystems. In our instance, RAST found 3,580 coding sequences and 84 RNA genes in the genome of M. morganii MM 1, and 57% of annotated sequences belonged to subsystems (Supplementary Figure 2). We identified 3,529 CDSs and 83 RNA genes in M. morganii MM 4 genome and 3,711 CDSs and 84 RNA genes in M. morganii MM 190 genome using this server. Respectively, 57 and 55% of these genes belong to subsystem categories.
RAST revealed that the three annotated M. morganii genomes contain virulence-related genes corresponding to the following categories: “cell wall and capsule” (163–172 genes); “regulation and cell signaling” (108–110 genes), including “quorum sensing and biofilm formation” (9 genes) and “regulation of virulence” (9 genes); “motility and chemotaxis” (56 genes); “iron acquisition and metabolism” (9–19 genes); and “virulence, disease and defense” (78–79 genes). MM 4 and MM 190 strains had the same number of genes under the categories above. This correlates well with genome alignment results because they have a 99.96% of genome identity (Figure 2). We detected that RAST annotated fewer genes in comparison with NCBI tools. Hence, we used the results of both RAST and the Prokaryotic Genomes Annotation Pipeline for the identification of all virulence-related genes in the M. morganii genomes (Figure 4; Supplementary Table 3). Notwithstanding, there were no significant differences between the gene number of the strains.
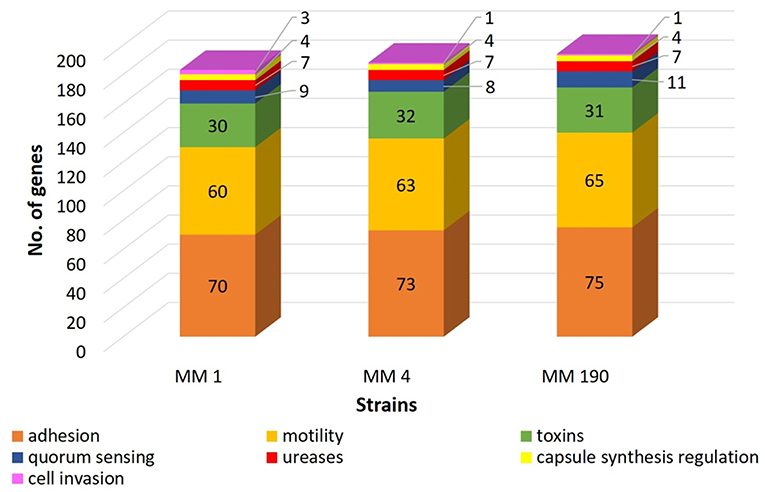
Figure 4. A comparison of the number of virulence-related genes in genomes of uropathogenic M. morganii strains obtained from annotation results of RAST and NCBI.
Phage Regions and Pathogenicity Islands
A large quantity of pathogenic bacteria contain intact or degenerated prophage regions in their genomes (Casjens, 2003; Davies et al., 2016) majority of which code for different virulence factors (Brüssow et al., 2004). Prophage diversity is caused by high frequency of recombination with other phages, mobile elements, and genomic DNA of host bacteria. This flexibility also leads to divergence of bacterial strains within species. Thus, a large part of strain-specific DNA are associated with prophage sequences (Casjens, 2003; Zubair et al., 2015). Both intact and incomplete prophages can impart important biological properties of their host. Consequently, to fully understand bacterial genomes, the identification and analysis of their prophages are important.
Prophage sequences diversity in different M. morganii strains was investigated. Using PHASTER, we identified 7, 6, and 10 prophages in the genomes of MM 1, MM 4, and MM 190 strains, respectively (Supplementary Table 2). M. morganii MM 1 genome contains 4 intact prophages similar to mEp460 (49.7 Kb), phiO18P (34.5 Kb), HK446 (41.3 Kb), and vB_SosS_Oslo (24.5 Kb). The same number of intact prophages was determined in the genome of M. morganii MM 4. However, they showed resemblance with Gifsy (17.3 Kb), phiO18P (33.8 Kb), cdtl (19.2 Kb), and 118970_sal3 (12 Kb). Finally, M. morganii MM 190 has five intact prophages, which showed similarities with the following: Fels1 (35.3 Kb), ST64B (14.8 Kb), ENT47670 (45.2 Kb), 118970_sal3 (19 Kb), and HK446 (7.7 Kb). All genomes contain incomplete prophage homologous to Burkholderia cenocepacia phage BcepB1A (NC_005886, Summer et al., 2006). MM 1 and MM 4 contained intact prophage similar to Aeromonas virus phiO18P (NC_009542, Beilstein and Dreiseikelmann, 2008), whereas in the genomes of MM 1 and MM 190 an intact prophage possessing a 70% identity with Enterobacteria phage HK446 (NC_019714) was identified. Two prophage homologous to Salmonella phages 118970_sal4 (NC_030919) and 118970_sal3 (NC_031940, Paradiso et al., 2016) were found to be common for MM 4 and MM 190. M. morganii MM 190 had duplicated 118970_sal3 prophages, though one of them was incomplete. In addition, insertions of 118970_sal4 contained hlyCABD operon encoding an α-hemolysin homolog.
Thus, the strains contain 6-10 prophages (16 unique types of prophages for three genomes), which represent 5.4, 3.1, and 6.2% of the MM 1, MM 4, and MM 190 genomes, respectively. This is typical for enterobacteria. For instance, the E. coli strain K-12 contains 9 prophages which account for 3.6% of its genome (Wang et al., 2010). 5 ± 3 prophage regions have been identified in the genomes of different serovars of Salmonella enterica (Mottawea et al., 2018), although some of them contained up to 15 prophages per genome. This is also true for other M. morganii strains. For example, M. morganii F675 had 9 prophages (6 of them were intact), comprising 7.3% of the total genome sequence (Olaitan et al., 2014). M. morganii INSRALV892a contained 10 prophage regions, of which four complete prophages showed the high similarity with Enterobacteria phages SfV and mEp235 (Jones-Dias et al., 2016). Finally, M. morganii KT had 2 intact and 12 incomplete prophages, representing 7% of its genome sequence, which were also found in the genomes of Providencia rustigianii, Providencia alcalifaciens, Proteus mirabilis, and 14 other entrobacteria species (Chen et al., 2012).
Pathogenicity islands (PAIs) are DNA sequences of 10–200 Kb in size, which are widespread among bacterial pathogens and carry a number of virulence-related genes (Hacker and Kaper, 2000). In many cases the base composition of PAIs differs from host genome, allowing one to trace the origin of these elements (Schmidt and Hensel, 2004). PAIs are often located next to tRNA genes and flanked by direct repeats (Hacker and Kaper, 2000). tRNA genes are known as insertion sites for foreign DNA acquired by transformation, transduction, and conjugation (Gal-Mor and Finlay, 2006). Thus, PAIs can distribute the virulence-associated genes by horizontal gene transfer (Hochhut et al., 2006). It is known that colonization of urinary tract by UPEC strains is mediated by the expression of PAI-encoded virulence factors (Schmidt and Hensel, 2004). For example, pore-fornimg toxin α-hemolysin, which is active against majority of eukaryotic cells, is encoded by PAI (Javadi et al., 2017). Using IslandViewer4, we determined 25, 21, and 33 genomic islands (GIs) ranging from 4.2 to 34.9 Kb in the genomes of MM 1, MM 4, and MM 190, respectively. It was shown that these GIs encoded phage-associated proteins (transposase, recombinase, integrase etc.) and some toxins, such as type II toxin-antitoxin system toxins and HlyA family RTX toxin. Moreover, GIs from MM 1 include genes responsible for flagellar biosynthesis, conjugation, and arsenical resistance.
Hemolysins
Pore-forming toxins in particular hemolysins have a cytotoxic activity against broad range of cells from different species (Ristow and Welch, 2016; Lu et al., 2018). Lin et al. (1999) reported that V. cholerae strains express RTX toxin, which leads to the destruction of HEp-2 cells monolayer after 1 h of incubation. M. morganii MM 4 and M. morganii MM 190 were capable of β-hemolysis and their hemolytic activity was maximal at 2 h of growth (Figures 5A,B), whereas MM 190 strain showed 2 times higher hemolytic activity than MM 4. We likewise investigated the influence of M. morganii strains on T-24 bladder carcinoma cells monolayer. Some dead cells (stained blue) were detected in wells with MM 190 following 1 h of incubation, while 100% cell death occurred after 2 h of cultivation with the same strain (Figure 5C). MM 4 strain triggered the detachment of T-24 cells from substrate, whereas MM 1 demonstrated low cytotoxicity. These data correlate well with the hemolytic activity level of the investigated strains, suggesting a major role of secreted hemolysins from M. morganii in the destruction of urothelial cells.
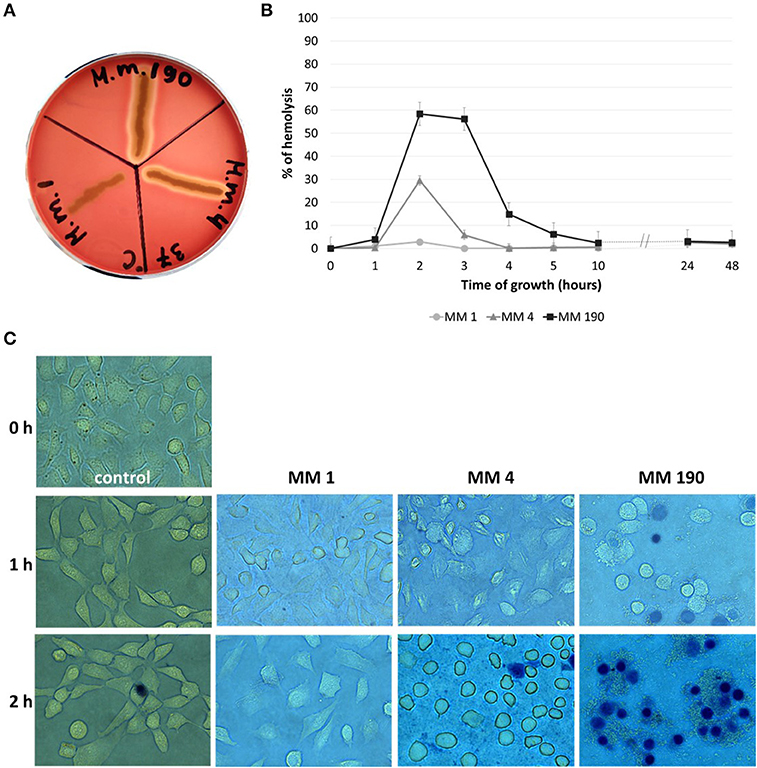
Figure 5. The hemolytic properties and cytotoxicity of uropathogenic M. morganii strains. (A) Evaluation of the hemolytic properties of M. morganii strains on 5% blood agar and (B) comparative analysis of hemolytic activity in 2% erythrocyte suspension during 48 h of growth. Hemolysis level in distilled water was taken as 100%. (C) Determination of the influence of M. morganii strains on T-24 bladder carcinoma cells monolayer using inverted microscopy (magnification−63x, trypan blue staining). Control–cell culture without bacteria.
Previously, we identified hlyA and hpmA hemolysin genes in the genomes of the M. morganii strains using PCR amplification (Minnullina et al., 2016). hpmA was common for all analyzed strains, but hlyA gene encoding RTX toxin was found only in MM 4 and MM 190 genomes (Table 3). It was determined that hpmA genes from the strains have a 97–100% identity to M. morganii FDAARGOS_172 and M. morganii KT sequences. hlyA genes from MM 4 and MM 190 showed 100% homology with M. morganii FDAARGOS_172 RTX toxin hemolysin A gene, even though homologous sequences were not identified in the genome of M. morganii KT.
Homologs of hpmA and hpmB genes encoding calcium-independent hemolysin and hemolysin activator protein in P. mirabilis (Fraser et al., 2002) were previously identified in M. morganii KT and M. morganii F675 genomes (Chen et al., 2012; Olaitan et al., 2014). The genomes of all strains (MM 1, MM 4, MM 190) contained intact hpmBA loci encoding hemolysin activation protein and filamentous hemagglutinin N-terminal domain-containing protein. Blastx-analysis confirmed that translated nucleotide sequences of hpmA from MM 1, MM 4, and MM 190 only have a 38% identity with the amino acid sequence of Proteus mirabilis (M30186). Notwithstanding, this sequence was not determined in Providencia genus.
Analogous to M. morganii KT isolated from blood sample, the hlyA gene homologous to α-hemolysin gene of E. coli was not detected in the genome of the non-hemolytic M. morganii MM 1. Genome analysis showed that only MM 4 and MM 190 strains contained intact hlyCABD operon having a 77% sequence homology with uropathogenic E. coli 536 (CP000247) (Figure 6). Translated nucleotide sequences of the RTX toxin genes had a 79% identity to amino acid sequence of Proteus columbae (WP_100158705). It is known that secreted hemolysin from M. morganii to a strong extent shares identity with the α-hemolysin of E. coli, showing maximal activity at the early growth hours (Gołuszko et al., 1988; Senior and Hughes, 1988). Thus, hemolytic properties of M. morganii MM 4 and M. morganii MM 190 are likely associated with expression of the hlyA gene. Oloomi and Bouzari (2008) reported that some strains of E. coli containing α-hemolysin gene did not show hemolytic properties on blood agar. Accordingly, hemolytic activity level can be associated with expression features of hlyA gene in different strains, since MM 4 and MM 190 hold a 100% identity in the hlyCABD operon.
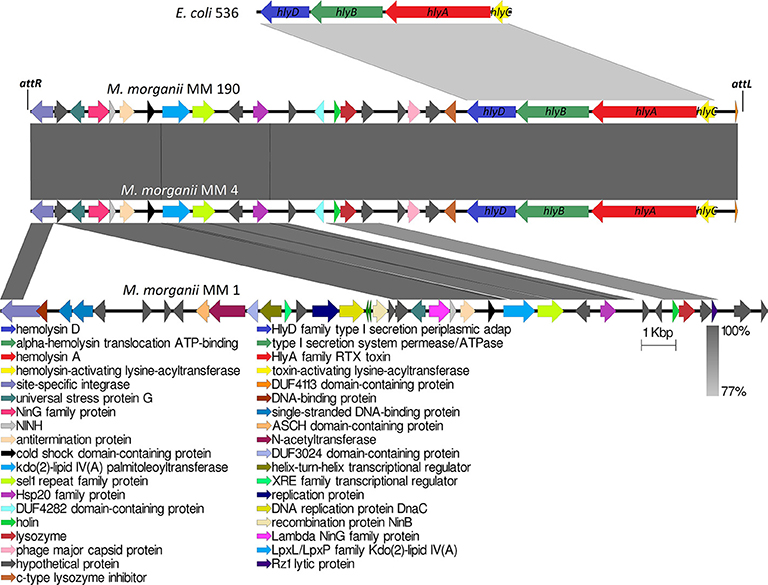
Figure 6. Multiple alignment of genome loci containing hlyCABD operon from uropathogenic M. morganii strains and E. coli 536. attL and attR indicate the attachment sites of 118970_sal4 phage.
Phage-mediated horizontal transfer of virulence-related genes is a major pathway of bacterial evolution (Javadi et al., 2017). In this connection, toxins have gained special attention among virulence factors in bacteria (Fortier and Sekulovic, 2013). A typical example is the botulinum neurotoxin (type C1), one of the most powerful toxins encoded by phage CEβ (Eklund et al., 1971). We observed that hlyCABD operons are inserted into genomes of M. morganii MM 4 and M. morganii MM 190 with phage sequences similar to Salmonella phage 118970_sal4 (dsDNA viruses, Caudovirales family, Figure 6). These prophages were incomplete and showed the same length (20.9 Kb) and GC content (39.3%). Meanwhile, the GC content of MM 4 and MM 190 genomes averaged at about 51%. 118970_sal4 prophage of length 42.4 Kb is well-known among Salmonella Heidelberg isolates. For instance, 92.3% of 196 isolates of S. Heidelberg contained this prophage in their genomes (Mottawea et al., 2018). However, neither the Salmonella spp. nor the phage 118970_sal4 genomes contained sequences homologous to RTX toxin operon.
Koronakis et al. (1987) suggested that hlyCABD locus of E. coli could have been imported from the Proteus genus since the GC content of its operon is about 39% (similar to genomic DNA of Proteus spp.) in contrast to that of the E. coli genome, having a 50% GC. hlyCABD loci from MM 4 and MM 190 strains have a GC content of 36.7% and are integrated into PAIs size of 11.3 Kb containing 9 genes. Furthermore, hlyCABD operon of E. coli 536 integrated to PAI (Hochhut et al., 2006), possesses a GC content of 40.2% in comparison with the whole genome (GC = 50.5%). It is known that many of UPEC strains contain hlyCABD operon integrated to PAIs, such as PAI-I, PAI-II, PAI-IV, and PAI-V. A classic illustration can be seen in E. coli C5, in which this sequence was located in PAI-I, which is inserted into the leuX loci (Javadi et al., 2017). In this connection, we suggest that M. morganii strains MM 4 and MM 190 could have a common source of RTX toxin operon with E. coli 536. Thus, our data for the first time suggest the possible transfer of hlyCABD operon into M. morganii genome by phage.
Ureases
Urease is a major virulence factor of uropathogenic microorganisms (Jones and Mobley, 1987), whose activity often leads to urinary stone formation (Mobley and Warren, 1987). We have investigated whether MM 1, MM 4, and MM 190 strains were capable of urease synthesis. The urease gene cluster ureABCEFGD was determined in their genomes in following loci: DYH52_RS16945-DYH52_RS16975 for MM 1, DVJ80_RS14270-DVJ80_RS14300 for MM 4, and DQ401_RS15300-DQ401_RS15330 for MM 190. Interestingly, the ureR regulatory gene was not identified in any of the genomes as well as in other M. morganii strains (Chen et al., 2012; Olaitan et al., 2014). It has been shown that the ureABCEFGD locus is conservative within the genus, the sequences show a 99–100% homology with each other and 99% with M. morganii KT and M. morganii FDAARGOS_172. Blast-analysis determined that the closest neighbors of the strains using ureABCEFGD locus among Proteeae tribe were Providencia stuartii FDAARGOS_145 (CP014024) and Proteus vulgaris FDAARGOS_556 (CP033736) with homology rates of 73 and 64%, respectively. However, in the Proteus genus, the ureD gene is located on the top of the operon, unlike in Morganella and Providencia (Figure 7).
Cell Capsule and Virulence Genes Regulation
Capsular polysaccharides (CPS) allow pathogenic bacteria to avoid the host immune response, mediate biofilm formation and survive in adverse conditions (Willis and Whitfield, 2013). Thirty-five genes were identified for M. morganii MM 1, MM 4, and MM 190 strains fitting the “capsular and extracellular polysaccharides” subcategory by the RAST server. We found that the strains contain four genes responsible for capsule synthesis regulation: rcsB, rcsC, rcsD, and rcsF (Supplementary Table 3). The Rcs phosphorelay two-component pathway initially was described as capsule and colanic acid synthesis regulator in E. coli (Stout, 1994). However, it was later discovered that this system plays an important role in the regulation of pathogenicity and biofilm formation (Huang et al., 2006). The Rcs phosphorelay is distributed among representatives of Enterobacterales order and consists of three proteins, which constitute the signaling pathway: the phosphotranspherase RcsD, the response regulator RcsB, and the hybrid kinase RcsC (Clarke, 2010). In E. coli, these proteins are encoded by genes which are located in convergent operons rcsDB and rcsC (Huang et al., 2006). Rcs-system is involved in the regulation of genes responsible for the synthesis of LPS, fimbriae, flagella, group 1 capsule, colanic acid, and other components of bacterial surface structures, which affect the virulence of pathogenic bacteria (Clarke, 2010; Pannen et al., 2016). rcsDBC sequences of MM 4 and MM 190 had a homology level of 100% to each other, and 99% with MM 1, KT, and FDAARGOS_172 strains. M. morganii strains exhibit different similarities with representatives of the Proteeae tribe relative to this locus. In particular, the RcsD, RcsB, and RcsC loci respectively showed 42, 91, and 51% amino acid sequence identity to P. mirabilis PmBC1123. Loci containing rcsB, rcsC, and rcsD genes were highly conserved among the other enterobacteria (Figure 8).
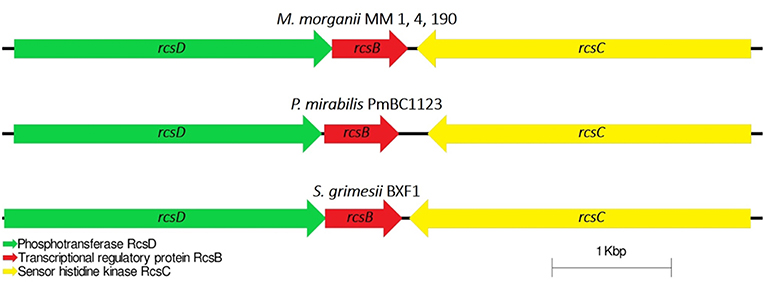
Figure 8. Comparative analysis of the loci of capsular synthesis regulating genes in M. morganii, P. mirabilis, and Serratia grimesii. rcsF gene is located distally.
Motility and Adhesion
Expression of flagella enables UPEC to spread from the bladder to the kidneys (Wiles et al., 2008). Several reports indicated that chemotaxis may be involved in the development of diseases caused by enterobacteria (Olsen et al., 2013; Matilla and Krell, 2018). Wuichet and Zhulin (2010) reported that 54% of 450 bacterial genomes analyzed in their study contain genes associated with chemosensory pathways. Chemoreceptor genes, which have a common origin with flagellar motor and signaling genes, represent about 14 units per genome. For instance, Borrelia burgdorferi chemoreceptor genes comprised 5–6% of the corresponding genome (Lacal et al., 2010; Charon et al., 2012). We identified more than 60 genes associated with motility and chemotaxis in the genomes of M. morganii MM 1, MM 4, and MM 190 strains (Supplementary Table 3). Among them, there are genes encoding structural components of flagellum and basal body (fli, flg, mot), type III secretion system proteins (ysc), transcriptional regulators (flh), and chemotaxis-related genes (che). Flagella and chemotaxis-associated genes were located between the following loci: DYH52_RS12265-DYH52_RS12675 (MM 1), DVJ80_RS00620-DVJ80_RS00920 (MM 4), and DQ401_RS04175-DQ401_RS04475 (MM 190).
It is known that type I fimbriae allow uropathogens to colonize bladder epithelium, while mannose-resistance (MR) fimbriae or P-fimbriae promote adhesion to kidney epithelial cells (Kalita et al., 2014; Lüthje and Brauner, 2014). Seventy, Seventy-three, and Seventy-five fimbrial biogenesis and adhesion-related genes were determined in the genomes of MM 1, MM 4, and MM 190, respectively (Supplementary Table 3). Several duplicated loci containing fim operon encoding type I fimbriae were found in all genomes. Besides them, we detected a large mrf gene cluster similar to mrp locus of P. mirabilis (Meslet-Cladiere et al., 2004) and pap genes involved in MR-fimbriae expression, pil genes responsible for type IV fimbriae synthesis, and stf genes encoding fimbrial assembly proteins. These data confirm that the M. morganii strains may be important causative agents of UTIs.
Conclusions
In this study we present the first detailed genome analysis of urine isolates of M. morganii. MM 1, MM 4, and MM 190 strains differed by the number of phage-associated genes. We determined that cytotoxicity and hemolytic activity of MM 4 and MM 190 strains were associated with expression of RTX toxin gene, which belongs to the genome island inserted into the genomes by a phage similar to the Salmonella phage 118970_sal4. Thus, the results of this study could be an important information source for investigating the pathogenesis of uropathogenic M. morganii.
Data Availability
The datasets generated for this study can be found in DDBJ/EMBL/GenBank, QUOO00000000, QPLM00000000, QMKL00000000.
Author Contributions
LM conducted the hemolysis and cytotoxicity assays, genomic DNA extraction, genome assembly and comparative analysis, and prepared the manuscript. DP performed the genome annotation and analysis. ES and LS carried out the genome sequencing of all the bacterial isolates. MS was involved in the review and design of the manuscript. AM directed conception and design of the study, wrote sections of the manuscript, and critically revised the final version of the manuscript. All authors contributed to manuscript revision, read and approved the submitted version.
Funding
This work was supported by the Russian Government Program of Competitive Growth of Kazan Federal University.
Conflict of Interest Statement
The authors declare that the research was conducted in the absence of any commercial or financial relationships that could be construed as a potential conflict of interest.
Acknowledgments
Authors would like to specially thank Dr. Eugene Shakirov and Yaw Akosah for critical comments to the manuscript, and Maria Shalavina at the Clinical Diagnostic Laboratory Biomed (Kazan, Republic of Tatarstan, Russia) for strain isolation.
Supplementary Material
The Supplementary Material for this article can be found online at: https://www.frontiersin.org/articles/10.3389/fcimb.2019.00167/full#supplementary-material
References
Abdalla, J., Saad, M., Samnani, I., Lee, P., and Moorman, J. (2006). Central nervous system infection caused by Morganella morganii. Am. J. Med. Sci. 331, 44–47. doi: 10.1097/00000441-200601000-00013
Adeolu, M., Alnajar, S., Naushad, S., and S Gupta, R. (2016). Genome-based phylogeny and taxonomy of the ‘Enterobacteriales’: proposal for Enterobacterales ord. nov. divided into the families Enterobacteriaceae, Erwiniaceae fam. nov., Pectobacteriaceae fam. nov., Yersiniaceae fam. nov., Hafniaceae fam. nov., Morganellaceae fam. nov., and Budviciaceae fam. nov. Int. J. Syst. Evol. Microbiol. 66, 5575–5599. doi: 10.1099/ijsem.0.001485
Alikhan, N. F., Petty, N. K., Ben Zakour, N. L., and Beatson, S. A. (2011). BLAST Ring Image Generator (BRIG): simple prokaryote genome comparisons. BMC Genomics 12:402. doi: 10.1186/1471-2164-12-402
Angiuoli, S.V., Gussman, A., Klimke, W., Cochrane, G., Field, D., Garrity, G., et al. (2008). Toward an online repository of standard operating procedures (SOPs) for (meta)genomic annotation. OMICS 12, 137–141. doi: 10.1089/omi.2008.0017
Arndt, D., Grant, J. R., Marcu, A., Sajed, T., Pon, A., Liang, Y., et al. (2016). PHASTER: a better, faster version of the PHAST phage search tool. Nucleic Acids Res. 44, W16–W21. doi: 10.1093/nar/gkw387
Atalay, H., Güney, I., Solak, Y., and Almaz, E. (2010). First case of CAPD-related peritonitis caused by Morganella morganii. Perit. Dial. Int. 30, 119–121. doi: 10.3747/pdi.2008.00265
Aziz, R.K., Bartels, D., Best, A.A., DeJongh, M., Disz, T., Edwards, R.A., et al. (2008). The RAST server: rapid annotations using subsystems technology. BMC Genomics 9:75. doi: 10.1186/1471-2164-9-75
Bankevich, A., Nurk, S., Antipov, D., Gurevich, A.A., Dvorkin, M., Kulikov, A.S., et al. (2012). Spades: a new genome assembly algorithm and its applications to single-cell sequencing. J. Comput. Biol. 19, 455–477. doi: 10.1089/cmb.2012.0021
Beilstein, F., and Dreiseikelmann, B. (2008). Temperate bacteriophage PhiO18P from an Aeromonas media isolate: characterization and complete genome sequence. Virology 373, 25–29. doi: 10.1016/j.virol.2007.11.016
Bertelli, C., Laird, M. R., Williams, K. P., Lau, B. Y., Hoad, G., et al. (2017). IslandViewer 4: expanded prediction of genomic islands for larger-scale datasets. Nucleic Acids Res. 45, W30–W35. doi: 10.1093/nar/gkx343
Blom, J., Kreis, J., Spänig, S., Juhre, T., Bertelli, C., Ernst, C., et al. (2016). EDGAR 2.0: an enhanced software platform for comparative gene content analyses. Nucleic Acids Res. 44, W22–W28. doi: 10.1093/nar/gkw255
Bolger, A.M., Lohse, M., and Usadel, B. (2014). Trimmomatic: a flexible trimmer for Illumina sequence data. Bioinformatics 30, 2114–2120. doi: 10.1093/bioinformatics/btu170
Bosi, E., Donati, B., Galardini, M., Brunetti, S., Sagot, M. F., Lió, P., et al. (2015). MeDuSa: a multi-draft based scaffolder. Bioinformatics 31, 2443–2451. doi: 10.1093/bioinformatics/btv171
Brüssow, H., Canchaya, C., and Hardt, W. D. (2004). Phages and the evolution of bacterial pathogens: from genomic rearrangements to lysogenic conversion. Microbiol Mol. Biol. Rev. 68, 560–602. doi: 10.1128/MMBR.68.3.560-602.2004
Casjens, S. (2003). Prophages and bacterial genomics: what have we learned so far? Mol. Microbiol. 49, 277–300. doi: 10.1046/j.1365-2958.2003.03580.x
Charon, N. W., Cockburn, A., Li, C., Liu, J., Miller, K. A., Miller, M. R., et al. (2012). The unique paradigm of spirochete motility and chemotaxis. Annu. Rev. Microbiol. 66, 349–370. doi: 10.1146/annurev-micro-092611-150145
Chen, Y.T., Peng, H.L., Shia, W.C., Hsu, F.R., Ken, C.F., Tsao, Y.M., et al. (2012). Whole-genome sequencing and identification of Morganella morganii KT pathogenicity-related genes. BMC Genomics 13(Suppl. 7):S4. doi: 10.1186/1471-2164-13-S7-S4
Chenal, A., Sotomayor-Perez, A. C., and Ladant, D. (2015). “Structure and function of RTX toxins,” in The Comprehensive Sourcebook of Bacterial Protein Toxins, eds. J. Alouf, D. Ladant, and M. R. Popoff (Paris: Elsevier), 677–718. doi: 10.1016/B978-0-12-800188-2.00023-9
Clarke, D. J. (2010). The Rcs phosphorelay: more than just a two-component pathway. Future Microbiol. 5, 1173–1184. doi: 10.2217/fmb.10.83
Darling, A. C., Mau, B., Blattner, F. R., and Perna, N. T. (2004). Mauve: multiple alignment of conserved genomic sequence with rearrangements. Genome Res. 14, 1394–1403. doi: 10.1101/gr.2289704
Davies, E. V., Winstanley, C., Fothergill, J. L., and James, C. E. (2016). The role of temperate bacteriophages in bacterial infection. FEMS Microbiol. Lett. 363:fnw015. doi: 10.1093/femsle/fnw015
Eberspächer, B., Hugo, F., Pohl, M., and Bhakdi, S. (1990). Functional similarity between the haemolysins of Escherichia coli and Morganella morganii. J. Med. Microbiol. 33, 165–170. doi: 10.1099/00222615-33-3-165
Eklund, M. W., Poysky, F. T., Reed, S. M., and Smith, C. A. (1971). Bacteriophage and the toxigenicity of Clostridium botulinum type C. Science 172, 480–482. doi: 10.1126/science.172.3982.480
Emborg, J., Dalgaard, P., and Ahrens, P. (2006). Morganella psychrotolerans sp. nov., a histamine-producing bacterium isolated from various seafoods. Int. J. Syst. Evol. Microbiol. 56, 2473–2479. doi: 10.1099/ijs.0.64357-0
Emödy, L., Vörös, S., and Pál, T. (1982). Alpha-haemolysin, a possible virulence factor in Proteus morganii. FEMS Microbiol. Lett. 13, 329–331. doi: 10.1111/j.1574-6968.1982.tb08282.x
Erlanger, D., Assous, M.V., Wiener-Well, Y., Yinnon, A.M., and Ben-Chetrit, E. (2017). Clinical manifestations, risk factors and prognosis of patients with Morganella morganii sepsis. J. Microbiol. Immunol. Infect. S1684-S1182, 30193–30197. doi: 10.1016/j.jmii.2017.08.010
Flores-Mireles, A. L., Walker, J. N., Caparon, M., and Hultgren, S. J. (2015). Urinary tract infections: epidemiology, mechanisms of infection and treatment options. Nat. Rev. Microbiol. 13, 269–284. doi: 10.1038/nrmicro3432
Fortier, L. C., and Sekulovic, O. (2013). Importance of prophages to evolution and virulence of bacterial pathogens. Virulence 4, 354–365. doi: 10.4161/viru.24498
Foxman, B. (2010). The epidemiology of urinary tract infection. Nat. Rev. Urol. 7, 653–660. doi: 10.1038/nrurol.2010.190
Fraser, G. M., Claret, L., Furness, R., Gupta, S., and Hughes, C. (2002). Swarming-coupled expression of the Proteus mirabilis hpmBA haemolysin operon. Microbiology 148, 2191–2201. doi: 10.1099/00221287-148-7-2191
Gal-Mor, O., and Finlay, B. B. (2006). Pathogenicity islands: a molecular toolbox for bacterial virulence. Cell. Microbiol. 8, 1707–1719. doi: 10.1111/j.1462-5822.2006.00794.x
Ghosh, S., Bal, A.M., Malik, I., and Collier, A. (2009). Fatal Morganella morganii bacteraemia in a diabetic patient with gas gangrene. J. Med. Microbiol. 58, 965–967. doi: 10.1099/jmm.0.008821-0
Glasner, J. D., Liss, P., Plunkett, G., Darling, A., Prasad, T., Rusch, M., et al. (2003). ASAP, a systematic annotation package for community analysis of genomes. Nucleic Acids Res. 31, 147–151. doi: 10.1093/nar/gkg125
Gołuszko, P., Gospodarek, E., and Bugalski, R. (1988). Haemolytic activity of Morganella morganii strains. Acta Microbiol. Pol. 37, 65–71.
Gurevich, A., Saveliev, V., Vyahhi, N., and Tesler, G. (2013). QUAST: quality assessment tool for genome assemblies. Bioinformatics 29, 1072–1075. doi: 10.1093/bioinformatics/btt086
Hacker, J., and Kaper, J. B. (2000). Pathogenicity islands and the evolution of microbes. Annu. Rev. Microbiol. 54, 641–679. doi: 10.1146/annurev.micro.54.1.641
Hakyemez, I. N., Sit, M., Aktas, G., Tas, T., Mengeloglu, F.Z., and Kucukbayrak, A. (2012). A case of giant hepatic hydatid cyst infected with Morganella morganii and the literature review. Case Rep. Gastrointest. Med. 2012:591561. doi: 10.1155/2012/591561
Hannan, T. J., Mysorekar, I. U., Chen, S. L., Walker, J. N., Jones, J. M., Pinkner, J. S., et al. (2008). LeuX tRNA-dependent and -independent mechanisms of Escherichia coli pathogenesis in acute cystitis. Mol. Microbiol. 67, 116–128. doi: 10.1111/j.1365-2958.2007.06025.x
Herlax, V., Henning, M. F., Bernasconi, A. M., Goni, F. M., and Bakas, L. (2010). The lytic mechanism of Escherichia coli α-hemolysin associated to outer membrane vesicles. Health. 2, 484–492. doi: 10.4236/health.2010.25072
Hilbert, D.W., Paulish-Miller, T.E., Tan, C.K., Carey, A.J., Ulett, G.C., Mordechai, E., et al. (2012). Clinical Escherichia coli isolates utilize alpha-hemolysin to inhibit in vitro epithelial cytokine production. Microbes Infect. 14, 628–638. doi: 10.1016/j.micinf.2012.01.010
Hochhut, B., Wilde, C., Balling, G., Middendorf, B., Dobrindt, U., Brzuszkiewicz, E., et al. (2006). Role of pathogenicity island-associated integrases in the genome plasticity of uropathogenic Escherichia coli strain 536. Mol. Microbiol. 61, 584–595. doi: 10.1111/j.1365-2958.2006.05255.x
Huang, Y. H., Ferrières, L., and Clarke, D. J. (2006). The role of the Rcs phosphorelay in Enterobacteriaceae. Res. Microbiol. 157, 206–212. doi: 10.1016/j.resmic.2005.11.005
Imataki, O., and Uemura, M. (2017). Fatal rhabdomyolysis caused by Morganella morganii in a patient with multiple myeloma. Intern. Med. 56, 369–371. doi: 10.2169/internalmedicine.56.7252
Javadi, M., Bouzari, S., and Oloomi, M. (2017). “Horizontal gene transfer and the diversity of Escherichia coli,” in Escherichia coli. Recent Advances on Physiology, Pathogenesis and Biotechnological Applications, ed. A. Samie (London: IntechOpen), 317–331. doi: 10.5772/intechopen.68307
Jones, B. D., and Mobley, H. L. (1987). Genetic and biochemical diversity of ureases of Proteus, Providencia, and Morganella species isolated from urinary tract infection. Infect. Immun. 55, 2198–2203.
Jones-Dias, D., Clemente, L., Moura, I. B., Sampaio, D. A., Albuquerque, T., Vieira, L., et al. (2016). Draft genomic analysis of an avian multidrug resistant Morganella morganii isolate carrying qnrD1. Front. Microbiol. 7:1660. doi: 10.3389/fmicb.2016.01660
Kalita, A., Hu, J., and Torres, A.G. (2014). Recent advances in adherence and invasion of pathogenic Escherichia coli. Curr. Opin. Infect. Dis. 27, 459–464. doi: 10.1097/QCO.0000000000000092
Kim, J. H., Cho, C. R., Um, T. H., Rhu, J. Y., Kim, E. S., Jeong, J. W., et al. (2007). Morganella morganii sepsis with massive hemolysis. J. Korean Med. Sci. 22, 1082–1084. doi: 10.3346/jkms.2007.22.6.1082
Koronakis, V., Cross, M., Senior, B., Koronakis, E., and Hughes, C. (1987). The secreted hemolysins of Proteus mirabilis, Proteus vulgaris, and Morganella morganii are genetically related to each other and to the alpha-hemolysin of Escherichia coli. J. Bacteriol. 169, 1509–1515. doi: 10.1128/jb.169.4.1509-1515.1987
Lacal, J., García-Fontana, C., Muñoz-Martínez, F., Ramos, J. L., and Krell, T. (2010). Sensing of environmental signals: classification of chemoreceptors according to the size of their ligand binding regions. Environ. Microbiol. 12, 2873–2884. doi: 10.1111/j.1462-2920.2010.02325.x
Lin, T. Y., Chan, M. C., Yang, Y. S., Lee, Y., Yeh, K. M., Lin, J. C., et al. (2015). Clinical manifestations and prognostic factors of Morganella morganii bacteremia. Eur. J. Clin. Microbiol. Infect. Dis. 34, 231–236. doi: 10.1007/s10096-014-2222-8
Lin, W., Fullner, K. J., Clayton, R., Sexton, J. A., Rogers, M. B., Calia, K. E., et al. (1999). Identification of a Vibrio cholerae RTX toxin gene cluster that is tightly linked to the cholera toxin prophage. Proc. Natl. Acad. Sci. U.S.A. 96, 1071–1076. doi: 10.1073/pnas.96.3.1071
Liu, H., Zhu, J., Hu, Q., and Rao, X. (2016). Morganella morganii, a non-negligent opportunistic pathogen. Int. J. Infect. Dis. 50, 10–17. doi: 10.1016/j.ijid.2016.07.006
Lu, Y., Rafiq, A., Zhang, Z., Aslani, F., Fijak, M., Lei, T., et al. (2018). Uropathogenic Escherichia coli virulence factor hemolysin A causes programmed cell necrosis by altering mitochondrial dynamics. FASEB J. 32, 4107–4120. doi: 10.1096/fj.201700768R
Lüthje, P., and Brauner, A. (2014). Virulence factors of uropathogenic E. coli and their interaction with the host. Adv. Microb. Physiol. 65, 337–372. doi: 10.1016/bs.ampbs.2014.08.006
Mao, Y. C., Liu, P. Y., Hung, D. Z., Lai, W. C., Huang, S. T., Hung, Y. M., et al. (2016). Bacteriology of Naja atra snakebite wound and its implications for antibiotic therapy. Am. J. Trop. Med. Hyg. 94, 1129–1135. doi: 10.4269/ajtmh.15-0667
Matilla, M. A., and Krell, T. (2018). The effect of bacterial chemotaxis on host infection and pathogenicity. FEMS Microbiol. Rev. 42, 40–67 doi: 10.1093/femsre/fux052
Meslet-Cladiere, L. M., Pimenta, A., Duchaud, E., Holland, I. B., and Blight, M. A. (2004). In vivo expression of the mannose-resistant fimbriae of Photorhabdus temperata K122 during insect infection. J. Bacteriol. 186, 611–622. doi: 10.1128/JB.186.3.611-622.2004
Mihara, T., Nishimura, Y., Shimizu, Y., Nishiyama, H., Yoshikawa, G., Uehara, H., et al. (2016). Linking virus genomes with host taxonomy. Viruses 8:66. doi: 10.3390/v8030066
Minnullina, L. F., Al Allak, M. H., Khaitlina, S. Y., Sharipova, M. R., and Mardanova, A. M. (2016). The hemolytic properties of clinical isolates of Morganella morganii. Res. J. Pharm. Biol. Chem. Sci. 7, 1550–1556.
Mobley, H. L., and Warren, J. W. (1987). Urease-positive bacteriuria and obstruction of long-term urinary catheters. J. Clin. Microbiol. 25, 2216–2217.
Mottawea, W., Duceppe, M. O., Dupras, A. A., Usongo, V., Jeukens, J., Freschi, L., et al. (2018). Salmonella enterica prophage sequence profiles reflect genome diversity and can be used for high discrimination subtyping. Front. Microbiol. 9:836. doi: 10.3389/fmicb.2018.00836
Murphy, K., Ryan, C., Dempsey, E. M., O'Toole, P. W., Ross, R. P., Stanton, C., et al. (2015). Neonatal sulfhemoglobinemia and hemolytic anemia associated with intestinal Morganella morganii. Pediatrics 136:e1641–5. doi: 10.1542/peds.2015-0996
MV Murthy, A., Phan, M. D., Peters, K. M., Nhu, N. T. K., Welch, R. A., Ulett, G. C., et al. (2018). Regulation of hemolysin in uropathogenic Escherichia coli fine-tunes killing of human macrophages. Virulence 9, 967–980. doi: 10.1080/21505594.2018.1465786
Nakao, T., Yoshida, M., Kanashima, H., and Yamane, T. (2013). Morganella morganii pericarditis in a patient with multiple myeloma. Case Rep. Hematol. 2013:452730. doi: 10.1155/2013/452730
O'Hara, C. M., Brenner, F. W., and Miller, J. M. (2000). Classification, identification, and clinical significance of Proteus, Providencia, and Morganella. Clin. Microbiol. Rev. 13, 534–546. doi: 10.1128/CMR.13.4.534
Olaitan, A. O., Diene, S. M., Gupta, S. K., Adler, A., Assous, M. V., and Rolain, J. M. (2014). Genome analysis of NDM-1 producing Morganella morganii clinical isolate. Expert Rev. Anti Infect. Ther. 12, 1297–1305. doi: 10.1586/14787210.2014.944504
Oloomi, M., and Bouzari, S. (2008). Molecular profile and genetic diversity of cytolethal distending toxin (CDT)-producing Escherichia coli isolates from diarrheal patients. APMIS 116, 125–132. doi: 10.1111/j.1600-0463.2008.00910.x
Olsen, J. E., Hoegh-Andersen, K. H., Casadesús, J., Rosenkranzt, J., Chadfield, M. S., and Thomsen, L. E. (2013). The role of flagella and chemotaxis genes in host pathogen interaction of the host adapted Salmonella enterica serovar Dublin compared to the broad host range serovar S. Typhimurium. BMC Microbiol. 13:67. doi: 10.1186/1471-2180-13-67
Pannen, D., Fabisch, M., Gausling, L., and Schnetz, K. (2016). Interaction of the RcsB response regulator with auxiliary transcription regulators in Escherichia coli. J. Biol. Chem. 291, 2357–2370. doi: 10.1074/jbc.M115.696815
Paradiso, R., Orsini, M., Bolletti Censi, S., Borriello, G., and Galiero, G. (2016). Complete genome sequence of a Myoviridae bacteriophage infecting Salmonella enterica serovar Typhimurium. Genome Announc. 4:e00940–16. doi: 10.1128/genomeA.00940-16
Patil, A. B., Nadagir, S. D., Lakshminarayana, S., and Syeda, F. M. (2012). Morganella morganii, subspecies morganii, biogroup A: an unusual causative pathogen of brain abscess. J. Neurosci. Rural Pract. 3, 370–372. doi: 10.4103/0976-3147.102631
Ponte, A., and Costa, C. (2015). Multiple liver abscesses due to Morganella morganii. Acta Med. Port. 28:539. doi: 10.20344/amp.5584
Rattanama, P., Thompson, J. R., Kongkerd, N., Srinitiwarawong, K., Vuddhakul, V., and Mekalanos, J. J. (2012). Sigma E regulators control hemolytic activity and virulence in a shrimp pathogenic Vibrio harveyi. PLoS ONE 7:e32523. doi: 10.1371/journal.pone.0032523
Richter, M., Rosselló-Móra, R., Oliver Glöckner, F., and Peplies, J. (2016). JSpeciesWS: a web server for prokaryotic species circumscription based on pairwise genome comparison. Bioinformatics 32, 929–931. doi: 10.1093/bioinformatics/btv681
Ristow, L. C., and Welch, R. A. (2016). Hemolysin of uropathogenic Escherichia coli: a cloak or a dagger? Biochim. Biophys. Acta 1858, 538–545. doi: 10.1016/j.bbamem.2015.08.015
Schmidt, H., and Hensel, M. (2004). Pathogenicity islands in bacterial pathogenesis. Clin. Microbiol. Rev. 17, 14–56. doi: 10.1128/CMR.17.1.14-56.2004
Schonwetter, R. S., and Orson, F. M. (1988). Chronic Morganella morganii arthritis in an elderly patient. J. Clin. Microbiol. 26, 1414–1415.
Senior, B. W., and Hughes, C. (1988). Production and properties of haemolysins from clinical isolates of the Proteeae. J. Med. Microbiol. 25, 17–25. doi: 10.1099/00222615-25-1-17
Skals, M., Bjaelde, R. G., Reinholdt, J., Poulsen, K., Vad, B. S., Otzen, D. E., et al. (2014). Bacterial RTX toxins allow acute ATP release from human erythrocytes directly through the toxin pore. J. Biol. Chem. 289, 19098–19109. doi: 10.1074/jbc.M114.571414
Stickler, D. J. (2008). Bacterial biofilms in patients with indwelling urinary catheters. Nat. Clin. Pract. Urol. 5, 598–608. doi: 10.1038/ncpuro1231
Stickler, D. J. (2014). Clinical complications of urinary catheters caused by crystalline biofilms: something needs to be done. J. Intern. Med. 276, 120–129. doi: 10.1111/joim.12220
Stout, V. (1994). Regulation of capsule synthesis includes interactions of the RcsC/RcsB regulatory pair. Res. Microbiol. 145, 389–392. doi: 10.1016/0923-2508(94)90086-8
Sullivan, M. J., Petty, N. K., and Beatson, S. A. (2011). Easyfig: a genome comparison visualizer. Bioinformatics 27, 1009–1010. doi: 10.1093/bioinformatics/btr039
Summer, E. J., Gonzalez, C. F., Bomer, M., Carlile, T., Embry, A., Kucherka, A. M., et al. (2006). Divergence and mosaicism among virulent soil phages of the Burkholderia cepacia complex. J. Bacteriol. 188, 255–268. doi: 10.1128/JB.188.1.255-268.2006
Tettelin, H., Riley, D., Cattuto, C., and Medini, D. (2008). Comparative genomics: the bacterial pan-genome. Curr. Opin. Microbiol. 11, 472–477. doi: 10.1016/j.mib.2008.09.006
Thomas, M. S., and Wigneshweraraj, S. (2014). Regulation of virulence gene expression. Virulence 5, 832–834. doi: 10.1080/21505594.2014.995573
Tsai, M. T., Yeh, J. T., Yang, W. C., and Wu, T. H. (2013). CAPD-related peritonitis caused by Morganella morganii. Perit. Dial. Int. 33, 104–105. doi: 10.3747/pdi.2012.00035
Tsai, Y. H., Hsu, W. H., Huang, K. C., Yu, P. A., Chen, C. L., and Kuo, L. T. (2017). Necrotizing fasciitis following venomous snakebites in a tertiary hospital of southwest Taiwan. Int. J. Infect. Dis. 63, 30–36. doi: 10.1016/j.ijid.2017.08.005
Waller, T. A., Pantin, S. A. L., Yenior, A. L., and Pujalte, G. G. A. (2018). Urinary tract infection antibiotic resistance in the United States. Prim. Care. 45, 455–466. doi: 10.1016/j.pop.2018.05.005
Wang, X., Kim, Y., Ma, Q., Hong, S. H., Pokusaeva, K., Sturino, J. M., et al. (2010). Cryptic prophages help bacteria cope with adverse environments. Nat. Commun. 1:147. doi: 10.1038/ncomms1146
Welch, R. A. (2016). Uropathogenic Escherichia coli-associated exotoxins. Microbiol. Spectr. 4, 1–10 doi: 10.1128/microbiolspec.UTI-0011-2012
Wiles, T. J., Kulesus, R. R., and Mulvey, M. A (2008). Origins and virulence mechanisms of uropathogenic Escherichia coli. Exp. Mol. Pathol. 85, 11–19. doi: 10.1016/j.yexmp.2008.03.007
Willis, L. M., and Whitfield, C. (2013). Structure, biosynthesis, and function of bacterial capsular polysaccharides synthesized by ABC transporter-dependent pathways. Carbohydr. Res. 378, 35–44. doi: 10.1016/j.carres.2013.05.007
Wright, M. H., Adelskov, J., and Greene, A. C. (2017). Bacterial DNA extraction using individual enzymes and phenol/chloroform separation. J. Microbiol. Biol. Educ. 18:18.2.48. doi: 10.1128/jmbe.v18i2.1348
Wuichet, K., and Zhulin, I. B. (2010). Origins and diversification of a complex signal transduction system in prokaryotes. Sci. Signal. 3:ra50. doi: 10.1126/scisignal.2000724
Zhang, B., Pan, F., and Zhu, K. (2017). Bilateral Morganella morganii keratitis in a patient with facial topical corticosteroid-induced rosacea-like dermatitis: a case report. BMC Ophthalmol. 17:106. doi: 10.1186/s12886-017-0504-5
Keywords: Morganella morganii, uropathogens, genome, virulence-related genes, prophages, hemolysins
Citation: Minnullina L, Pudova D, Shagimardanova E, Shigapova L, Sharipova M and Mardanova A (2019) Comparative Genome Analysis of Uropathogenic Morganella morganii Strains. Front. Cell. Infect. Microbiol. 9:167. doi: 10.3389/fcimb.2019.00167
Received: 19 March 2019; Accepted: 03 May 2019;
Published: 22 May 2019.
Edited by:
Vincenzo Scarlato, Department of Pharmacy and Biotechnology, University of Bologna, ItalyReviewed by:
Paolo Sonego, Fondazione Edmund Mach, ItalyShannon Lyn Johnson, Los Alamos National Laboratory (DOE), United States
Copyright © 2019 Minnullina, Pudova, Shagimardanova, Shigapova, Sharipova and Mardanova. This is an open-access article distributed under the terms of the Creative Commons Attribution License (CC BY). The use, distribution or reproduction in other forums is permitted, provided the original author(s) and the copyright owner(s) are credited and that the original publication in this journal is cited, in accordance with accepted academic practice. No use, distribution or reproduction is permitted which does not comply with these terms.
*Correspondence: Leyla Minnullina, c2VxdWVuY2VyMTRAZ21haWwuY29t; TEZNaW5udWxsaW5hQGtwZnUucnU=