- 1Virology Laboratory, Basil Hetzel Institute for Translational Health Research, Discipline of Surgery, University of Adelaide, Adelaide, SA, Australia
- 2Molecular Mucosal Vaccine Immunology Group, The John Curtin School of Medical Research, The Australian National University, Canberra, ACT, Australia
- 3Viral Immunology Systems Program, The Kirby Institute, The University of New South Wales, Sydney, NSW, Australia
Human immunodeficiency virus (HIV)-1 and hepatitis C virus (HCV) are major contributors to the global disease burden with many experts recognizing the requirement of an effective vaccine to bring a durable end to these viral epidemics. The most promising vaccine candidates that have advanced into pre-clinical models and the clinic to eliminate or provide protection against these chronic viruses are viral vectors [e.g., recombinant cytomegalovirus, Adenovirus, and modified vaccinia Ankara (MVA)]. This raises the question, is there a need to develop DNA vaccines against HIV-1 and HCV? Since the initial study from Wolff and colleagues which showed that DNA represents a vector that can be used to express transgenes durably in vivo, DNA has been regularly evaluated as a vaccine vector albeit with limited success in large animal models and humans. However, several recent studies in Phase I-IIb trials showed that vaccination of patients with recombinant DNA represents a feasible therapeutic intervention to even cure cervical cancer, highlighting the potential of using DNA for human vaccinations. In this review, we will discuss the limitations and the strategies of using DNA as a vector to develop prophylactic T cell-mediated vaccines against HIV-1 and HCV. In particular, we focus on potential strategies exploiting DNA vectors to elicit protective localized CD8+ T cell immunity in the liver for HCV and in the cervicovaginal mucosa for HIV-1 as localized immunity will be an important, if not critical component, of an efficacious vaccine against these viral infections.
Introduction
Human immunodeficiency virus (HIV)-1 and hepatitis C virus (HCV) are significant contributors to the global disease burden with ~36.9 million people living with HIV-1 and at least 71 million people persistently infected with HCV (WHO, 2017; UNAIDS, 2018). Anti-retroviral therapy (ART) and direct acting anti-virals (DAAs) have contributed significantly to prolonging the lifespan and curing of HIV-1- and HCV-infected individuals, respectively (Cihlar and Fordyce, 2016; Zhang, 2016), but the annual HIV-1 and HCV incidences are still rising by millions. Furthermore, only 17 million (<50%) people have access to ART (Cihlar and Fordyce, 2016) and only 20% of patients are diagnosed for HCV (WHO, 2017). Additional issues involving drug resistance, reactogenicity associated with life-long ART and the lack of universal access to testing and cost-subsidized therapies minimize the ability of effective anti-viral drugs to end the HIV-1 and HCV epidemics. Thus, there is an urgent need to develop effective prophylactic vaccines to control the number of new infections and reduce the burden of supplying ART and DAA therapies to patients (Shin, 2016; Stone et al., 2016).
HIV-1 and HCV are rapidly mutating RNA viruses that exhibit considerable genetic diversity (nine subtypes in the major group of HIV-1 (German Advisory Committee Blood SAoPTbB., 2016) and 8 genotypes (gt1-8) of HCV which include at least 67 subtypes (Borgia et al., 2018) making immunity that develops during natural infection mostly ineffective. The lack of immune correlates of protection and convenient animal models permissive to infection make vaccine design and testing extremely challenging, and have also contributed to the fact that there is still no licensed vaccine for either HIV-1 or HCV (Wang et al., 2015; Bailey et al., 2019). HIV-1 and HCV co-infections represent an additional obstacle (Platt et al., 2016) although a recent clinical study suggests that co-administration of HIV-1 and HCV vaccines in humans can elicit robust HIV-1- and HCV-specific T cell responses without perturbing the immunodominance hierarchies of T cells responding against the vaccine encoded HIV-1 or HCV antigens (Hartnell et al., 2018).
DNA vaccines have been investigated for nearly three decades and are essentially bacteria-derived plasmids genetically engineered to encode immunogens under the control of promoters that facilitate robust expression of DNA in mammalian cells to induce adaptive immunity (Ferraro et al., 2011). DNA vaccines are inexpensive, easily constructed, stable at room temperature, replication defective in transfected mammalian cells and have minimum side effects which simplifies handling and distribution such that even developing countries can benefit from DNA vaccines (Jorritsma et al., 2016). Furthermore, plasmid DNA can be more easily used in multi-dose regimens unlike recombinant virus vectors that suffer from anti-vector immunity (Frahm et al., 2012). Recent seminal studies described therapeutic DNA vaccination against human papillomavirus (HPV) which resulted in histological regression and/or eliminated persistent HPV infection and HPV-related cervical lesions (Kim et al., 2014; Trimble et al., 2015). More recently, a DNA vaccine was developed that induced protective neutralizing antibodies (NAb) to Zika virus (ZIKV) in mice (Larocca et al., 2016) and rhesus macaques (Abbink et al., 2017) leading to the development of safe and immunogenic ZIKV DNA vaccines for humans (Tebas et al., 2017; Gaudinski et al., 2018). Thus, the many advantages of using plasmid DNA to develop vaccines and the recent developments of DNA vaccines in eliciting protective immunity in humans and higher animal models warrant further examination as to how DNA vaccines can be harnessed in vaccination regimens to target HIV-1 and HCV.
Immune Targets for HIV-1 and HCV Prophylactic Vaccine Development
It is imperative that vaccines take into account the virus tropism, transmission routes, pathogenesis and immune responses that provide effective resistance against infections to elicit protective immunity against HIV-1 and/or HCV.
It is now established that mucosal tissues, mainly the gentio-rectal tissues and gastrointestinal tract, are the major sites of HIV-1 entry and pathogenesis, respectively (Belyakov and Ahlers, 2012). Induction of robust HIV-specific immune responses at these sites will be necessary to prevent HIV-1 infection or at the very least control viraemia during the acute phase of infection thus reducing the viral set point (McMichael and Koff, 2014) and infection-induced microbial translocation which can result in diversion of immune responses to counteract dysbiosis (Vujkovic-Cvijin et al., 2013). Furthermore, a prophylactic HIV-1 vaccine will likely be delivered using an active immunization strategy and attempt to mimic immune responses reported to be protective in macaques against simian immunodeficiency virus (SIV) and/or provide resistance against natural HIV-1 infections (Pontesilli et al., 1998; Saez-Cirion et al., 2007; Hansen et al., 2011; Haynes et al., 2012; Barouch et al., 2015, 2018; Ackerman et al., 2016; Borducchi et al., 2016). In this regard, the most protective immune responses reported to date involve T cell-mediated immunity (CMI) (Pontesilli et al., 1998; Saez-Cirion et al., 2007; Hansen et al., 2011; Borducchi et al., 2016), polyfunctional antibody responses (Barouch et al., 2015, 2018; Ackerman et al., 2016), antibody-dependent cellular cytotoxicity (Haynes et al., 2012), and broadly neutralizing antibodies (bNAb) (Burton and Hangartner, 2016). Although potent bNAb represent a blueprint for HIV-1 vaccine design, these antibodies are unlikely to be as effective in preventing cell to cell transmission compared to neutralizing cell free virus (Parsons et al., 2017). Consequently, a highly effective prophylactic HIV-1 vaccine will likely also rely on CMI to target highly conserved viral proteins such as Gag and Pol (Rolland et al., 2007) and/or non-neutralizing antibodies to broadly target the virus Envelope to prevent cell-cell transmission of the virus.
Unlike HIV-1 which has a relatively broad tropism, HCV is a bloodborne virus that primarily infects and replicates in hepatocytes. In primary hepatitis C infection, ~25% of patients naturally clear the virus and although reinfection occurs in many individuals (Grebely et al., 2012), it is evident that repeated infection is associated with a reduced magnitude and duration of viraemia, and a greater likelihood of clearance (Sacks-Davis et al., 2015). Thus, characterizing and eliciting the naturally-protective immune responses during primary infection and reinfection provide a rational path for the design of a prophylactic HCV vaccine (Grebely et al., 2012). The immune responses that correlate best with natural protection include robust and broad CMI to conserved HCV non-structural (NS) proteins (NS3, NS4, and NS5) (Smyk-Pearson et al., 2008; Baumert et al., 2014) and NAb targeting conserved regions of the viral envelope (E1E2) proteins (Houghton, 2011; Osburn et al., 2014; Bailey et al., 2017). Although CMI will not prevent infection, clinical data suggest that T cell responses could prevent the development of persistent infection in individuals who naturally clear the virus, which is an acceptable outcome given that primary infection is often asymptomatic and not associated with severe disease outcomes (Baumert et al., 2014). After two decades of unsuccessful pre-clinical studies and Phase I HCV vaccine trials, the current lead prophylactic candidate is in an NIH-sponsored Phase IIb, placebo-controlled trial (ClinicalTrials.gov Identifier: NCT01436357) in high risk people who inject drugs (PWID) (Swadling et al., 2014). The candidate vaccination regimen being tested utilizes a chimpanzee adenovirus (ChAd) prime and a modified vaccinia Ankara (MVA) boost to elicit systemic T cell immunity to gt1 NS antigens (Swadling et al., 2014). However, it is not clear if this vaccination can induce robust intrahepatic T cell immunity and sufficient multi-genotypic immunity to result in significant protection in vaccinated individuals especially given the increased prevalence of multiple genotypes in HCV endemic regions.
T Cell-Mediated DNA Vaccines Against HIV-1 and HCV in the Clinic
DNA vaccines against HCV have been routinely tested in small and large animals including non-human primates (Latimer et al., 2014; Gummow et al., 2015; Grubor-Bauk et al., 2016; Wijesundara et al., 2018). Some candidates have also progressed in phase I/II clinical trials, but none have progressed to a large-scale efficacy trial in humans. A promising DNA vaccine that included a cocktail of four plasmids with each plasmid encoding codon optimized NS3/4A, NS4B, NS5A, or NS5B sequences from gt1a/b virus was used to prime/boost vaccinate macaques by electroporation (Latimer et al., 2014). In this study, the vaccine induced CD4+ and CD8+ T cells against each of the NS proteins encoded in the DNA cocktail which has resulted in the testing of the DNA cocktail in a phase I clinical trial (ClinicalTrials.gov Identifier: NCT02027116) although the results are yet to be disclosed.
A DNA vaccine has been tested for therapeutic vaccination against HCV. 12 hepatitis C patients suffering from chronic disease received three doses of a DNA vaccine encoding codon optimized NS3/4A from gt1a virus via electroporation on the deltoid muscle which induced NS3-specific CMI and a transient decrease in viral RNA levels (Weiland et al., 2013). The vaccine was also tested in eight patients who received interferon and ribavirin treatment of which six patients were completely cured of the infection (Weiland et al., 2013). Thus, DNA vaccines could be exploited in therapeutic settings against HCV, but this is unlikely to occur in the future given the success of using DAA to cure hepatitis C patients.
DNA vaccines against HIV-1 have been tested in different pre-clinical models and some have been tested in phase I/II clinical trials (Okuda et al., 1997; Cafaro et al., 2001; Tomusange et al., 2016). The first human clinical trial of a DNA vaccine, encoding env and rev genes, against HIV-1 was conducted in 1998 (MacGregor et al., 1998). Following vaccination of HIV-1 positive, treatment naïve individuals, no significant changes were observed in CD4+ and CD8+ T cell responses as well as in plasma HIV RNA. In another phase I clinical trial a DNA vaccine that encoded env and rev was shown to induce CD4+ T cell and poor CD8+ T cells responses in HIV-1 seronegative individuals (MacGregor et al., 2002). Similarly, low CD8+ T cell responses were observed in another phase I clinical trial following prime/boost vaccination with a DNA vaccine that encoded gag and pol genes (Tavel et al., 2007). More robust HIV-specific T cell responses have been elicited when DNA vaccines are used to prime and recombinant viral vectors are used to boost immune responses (Kibuuka et al., 2010; Bakari et al., 2011; Churchyard et al., 2011; Hayton et al., 2014; Moyo et al., 2017). However, prime/boost vaccinations with DNA vaccines alone can be optimized to elicit robust immune responses in humans against HIV-1. For instance, a retrospective study evaluating the immunogenicity of 10 HIV-1 DNA vaccine trials that used DNA vaccines in the absence of viral vectors or adjuvants suggest that the use of DNA delivery devices (e.g., electroporators and biojectors), and increasing the number of vaccine doses and dosage could more reproducibly elicit CD4+ and CD8+ T cell responses (Jin et al., 2015).
The main limitation associated with DNA vaccines is their inability to induce long-term immune responses following a single or a few vaccinations (Abbink et al., 2017). Furthermore, DNA vaccines are poorly effective and not well-optimized in eliciting immunity in the liver, gut or genito-rectal mucosa which warrant further refinements of DNA-based vaccination regimens in order to elicit durable protection against HIV-1 and/or HCV.
The Potential of Tissue-Resident Memory T Cells For Controlling HIV-1 and HCV Infections
Since the initial discovery of highly cytotoxic memory T cells residing in tissues (Masopust et al., 2001), several studies have shown that CD8+ tissue-resident memory T (TRM) cells residing in the female reproductive tract, the gut, the lung and the liver form a formidable frontline defense against various pathogen infections (Mueller and Mackay, 2016; Rosato et al., 2017). The protective role of CD8+ TRM cells is primarily due to their ability to (1) maintain a stable and durable population following their formation in tissues even in the absence of cognate antigen encounter following their formation (Gebhardt et al., 2009; MacKay et al., 2012; Beura et al., 2018; Park et al., 2018), and (2) produce anti-viral cytokines and/or exert cytotoxic functions to reduce the number of pathogen-infected cells and to recruit other immune cells (e.g., circulating memory T cells) rapidly to the site of infection (Schenkel et al., 2013; Muruganandah et al., 2018; Park et al., 2018). Furthermore, CD8+ TRM cells respond more rapidly, produce greater amounts of anti-viral/cytotoxic molecules (i.e., in the liver) and appear to be crucial for protection against liver tropic pathogens and pathogens exposed in the vagina and the female reproductive tract compared to circulating memory T cells (Cuburu et al., 2012, 2015; Shin and Iwasaki, 2012; Fernandez-Ruiz et al., 2016; Beura et al., 2018). The greater frequency of intrahepatic CD8+ TRM cells (CD69+ CD103+) amongst the total CD8+ T cell population correlated with partial control of viraemia in Hepatitis B Virus (HBV)-infected patients (Pallett et al., 2017), providing further encouragement that intrahepatic HCV-specific CD8+ TRM cells will likely be protective against HCV.
Despite HIV-1 and HCV being highly mutable with a complex and evolving quasispecies, several studies have revealed that only one or few variants, referred to as transmitted/founder (T/F) viruses, establish infection following transmission reflecting a strong genetic bottleneck (Bull et al., 2011; Joseph et al., 2015). T/F viruses will be exposed in the genito-rectal mucosa (i.e., the vagina and the rectum) during the vast majority (>80%) of HIV transmission and in the liver during HCV transmission. Thus, eliciting HIV- and HCV-specific CD8+ TRM cells in the genito-rectal mucosa and the liver, respectively, following vaccination is also an attractive strategy to circumvent issues associated with viral diversity and eliminate these viruses shortly after transmission/exposure. Several vaccine vectors such as radiation attenuated sporozoites (RAS), protein loaded nanoparticles (NP), adenovirus (Ad) vectors, adeno-associated virus (AAV), and HPV pseudovirus (HPV PsV) have been developed to elicit localized protection and in some instances elicit CD8+ TRM cells in the liver or the vagina (Figure 1) (Cuburu et al., 2012, 2015, 2018; Fernandez-Ruiz et al., 2016; Ishizuka et al., 2016; Gola et al., 2018). This provides hope that a vaccine to elicit intravaginal or intrahepatic CD8+ TRM cells can be developed to potentially provide protection against HIV-1 or HCV, respectively.
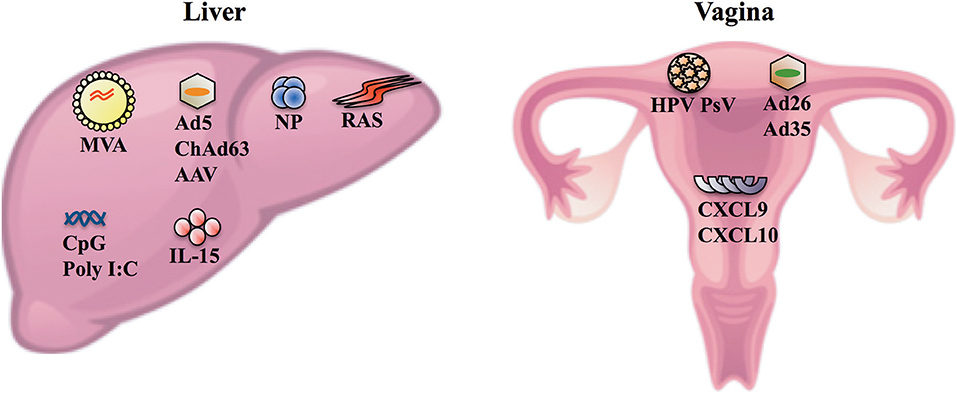
Figure 1. Vaccine vectors and agents that can be used to elicit and/or enhance the formation of CD8+ TRM cells in the liver and the vagina. Following intravenous delivery, MVA, Ad serotype 5 (Ad5), ChAd serotype 63 (ChAd63), AAV, NP, and RAS can enter hepatocytes and/or cells surrounding the hepatic tissues (e.g., Kupffer cells) (Nganou-Makamdop et al., 2012; Tay et al., 2014; Gola et al., 2018). Intravenous delivery of these vectors in multi-dose prime/trap (Fernandez-Ruiz et al., 2016; Olsen et al., 2018), prime/target (Gola et al., 2018) or homologous prime/boost (Ishizuka et al., 2016) regimens elicits protection following P. berghei sporozoite challenge in mice or controlled P. falciparum infections in humans, and elicits intrahepatic CD8+ TRM cells (Fernandez-Ruiz et al., 2016; Ishizuka et al., 2016; Gola et al., 2018). The prime/trap approaches involve priming CD8+ T cells systemically using antibodies that deliver a peptide antigen to cross-presenting dendritic cells (Fernandez-Ruiz et al., 2016) or using gene gun delivery of DNA encoding the cognate antigen (Olsen et al., 2018). The primed CD8+ T cells are then recruited to the liver and differentiate into TRM cells (i.e., trapped) following intravenous delivery of AAV (Fernandez-Ruiz et al., 2016) or RAS (Olsen et al., 2018) that enter cells in the liver and express the relevant cognate antigen which are recognized by the primed CD8+ T cells. The prime/target approach is an adaptation of the prime/trap approach and essentially involves priming naïve CD8+ T cells with Ad5 or ChAd63 vaccine vector delivered via the intramuscular route and recruiting the primed CD8+ T cells to the liver following intravenous delivery of Ad5, NP, MVA, or ChAd63 vaccine vector (Gola et al., 2018). The same study showed that intravenous delivery was more efficient than intramuscular delivery of vaccine vectors to elicit high numbers of intrahepatic CD8+ TRM cells and protection against P. berghei sporozoite challenge in the prime/target approach (Gola et al., 2018). IL-15 appears to be crucial for activated CD8+ T cells to differentiate into TRM cells in the liver and inflammatory signals (e.g., CpG and Poly I:C) can enhance the formation of intrahepatic CD8+ TRM cells in vivo (Holz et al., 2018). In the vagina, intravaginal delivery of HPV PsV, and more recently Ad26 and Ad35 have been shown to transduce cervicovaginal epithelial cells and elicit HPV-specific CD8+ TRM cells in the cervicovaginal mucosa (Cuburu et al., 2012, 2015, 2018). In the absence of a vaccine vector, topical application of CXCL9 and CXCL10 in the vagina can be used to recruit/pull CXCR3+ effector CD8+ T cells into the vagina which subsequently differentiate into cervicovaginal TRM cells (Shin and Iwasaki, 2012).
A recent study suggests that strategies that can induce interleukin (IL)-15 and/or inflammation in the liver can be effective in recruiting circulating effector CD8+ T cells to differentiate into CD8+ TRM cells in the liver (Holz et al., 2018). Systemic immunization strategies that promote up-regulation of gut homing molecules such as α4β7 on antigen-primed CD8+ T cells in secondary lymphoid organs can be efficiently recruited to establish residency in the gut (Masopust et al., 2010). Although these studies and others suggest that local antigen deposition and antigen encounter are not essential to elicit CD8+ TRM cells, it is evident that this process leads to the formation of greatest densities of CD8+ TRM cells especially at sites such as the liver and the vagina (Cuburu et al., 2012; Fernandez-Ruiz et al., 2016; Davies et al., 2017). Intravenous delivery of vaccine vectors appears to be most efficient route to facilitate local, intrahepatic expression of vaccine encoded antigens (Nganou-Makamdop et al., 2012; Tay et al., 2014; Gola et al., 2018), to elicit high numbers of intrahepatic CD8+ TRM cells, and protection against hepatotropic pathogens compared to intradermal and intramuscular vaccine delivery routes (Figure 1) (Epstein et al., 2011; Fernandez-Ruiz et al., 2016; Ishizuka et al., 2016; Gola et al., 2018). In the vagina, several studies suggest that intravaginal delivery of vaccine vectors [HPV PsV, and Ad serotypes 26 (Ad26) and 35 (Ad35)] is the most efficient route to express vaccine-encoded antigens in vaginal tissues and elicit cervicovaginal CD8+ TRM cells (Figure 1) (Cuburu et al., 2015, 2018; Fernandez-Ruiz et al., 2016). Furthermore, topical application of chemokine ligands (CXCL9 and CXCL10) in the vagina have been reported to “pull” systemically primed effector CD8+ T cells into the vagina and allow these cells to differentiate into CD8+ TRM cells (Shin and Iwasaki, 2012).
Can We Exploit DNA Vaccines to Elicit Tissue-Resident Memory T Cells for Protection Against HIV-1 or HCV?
There has been much research and progress made to improve the immunogenicity of DNA vaccines with respect to the choice of adjuvants, route of vaccine delivery, codon optimization of genes, method of delivery (e.g., electroporation and gene gun), etc. These aspects have been reviewed extensively elsewhere (Nagata et al., 1999; Garmory et al., 2003; Jechlinger, 2006; Vanniasinkam et al., 2006; Jorritsma et al., 2016) and the resulting refinements have led to DNA vaccines being more effectively exploited for use in Phase I and II clinical trials especially in the context of cancer (Kim et al., 2014; Trimble et al., 2015). However, vast majority of the studies including those progressing to the clinic have delivered DNA vaccines using intradermal or intramuscular routes. These routes may not be as effective compared to intravenous route to elicit intrahepatic CD8+ TRM cells or the intravaginal route to elicit cervicovaginal CD8+ TRM cells (Figure 1).
As mentioned above, it is important that a vaccination regimen designed to elicit CD8+ TRM cells facilitate local antigen presentation to naïve and antigen experienced precursors of CD8+ TRM cells, which is best achieved by the local expression of vaccine-encoded antigens and/or promoting local inflammation (Figure 1). Manual massaging (Liu et al., 2004), hydrodynamic injections (Yu et al., 2014), and liposome complexes (Kawakami et al., 2000) are some commonly used techniques to transfect hepatocytes in vivo following intravenous delivery of DNA. The expression of vaccine-encoded antigens in hepatocytes is a common hallmark of studies that have elicited intrahepatic CD8+ TRM cells (Fernandez-Ruiz et al., 2016; Ishizuka et al., 2016), but none of these delivery strategies have led to a licensed vaccine for use in humans. Furthermore, it not known whether any of these strategies can elicit intrahepatic CD8+ TRM cells in humans mainly owing to the difficulties of isolating liver biopsies in healthy patients although fine needle aspirates may be used to less invasively sample liver-resident T cells (Gill et al., 2018a,b) and the lack of biomarkers (i.e., in the blood) that can accurately predict the formation of CD8+ TRM cells in the liver and other tissues/organs. In the vagina, a proof of concept study has shown that DNA can be expressed following submucosal intravaginal delivery of DNA in mice (Sun et al., 2015). However, the same study reported poorly immunogenic responses in mice and noted that electroporation was required to improve the immunogenicity of intravaginally delivered DNA which could be difficult to exploit in humans.
DNA can be used as a vector to prime high numbers of circulating antigen-specific T cells (Gummow et al., 2015; Wijesundara et al., 2018) which can then be recruited to the liver using vectors that efficiently enter cells in the hepatic tissues or the vagina using chemokine ligands or vectors that transduce vaginal epithelial cells (Figure 1). Furthermore, given the poor transfection efficiency and immunogenicity of DNA when delivered into the vagina or the liver, it is more feasible to exploit DNA as an immune priming agent in a vaccination regimen to elicit HIV-1- or HCV-specific CD8+ TRM cells in the vagina or the liver, respectively. Furthermore, analogous strategies using protein-based T cell priming agents in prime/pull (Shin and Iwasaki, 2012), prime/trap (Fernandez-Ruiz et al., 2016), or prime/target (Gola et al., 2018) regimens have been used to elicit protective cervicovaginal or intrahepatic CD8+ TRM cells. A caveat in this case is to determine whether the primed T cells express adequate levels of chemokine receptors (e.g., CXCR3 and CXCR6) necessary to home to the liver (Sato et al., 2005; Tse et al., 2014; Gola et al., 2018; Olsen et al., 2018) or the vagina (Shin and Iwasaki, 2012) following DNA immunization. Even if not obligatory, the expression of the relevant homing receptors could be required to ensure that high densities of primed CD8+ T cells are recruited to the cervicovaginal mucosa or the liver following introduction of a vaccine vector or an agent (Figure 1) to facilitate the formation of CD8+ TRM cells. Several studies have shown that the number of CD8+ TRM cells is a crucial parameter that dictates the collective ability of these cells to confer protection against pathogens exposed in the skin, liver, or the vagina with greater numbers favoring protective outcomes (Cuburu et al., 2012; Shin and Iwasaki, 2012; Fernandez-Ruiz et al., 2016; Park et al., 2018).
Concluding Remarks
DNA has recently re-emerged as an effective vaccination platform in humans, but its use in developing a T cell-based vaccine will likely rely on its ability to be exploited in a regimen that can elicit robust immunity in the vagina and the gut in the context of HIV-1, or the liver in the context of HCV. In this regard, we have highlighted the importance of eliciting cervicovaginal or intrahepatic CD8+ TRM cells against these viruses and also reviewed strategies as well as caveats associated with using DNA to elicit localized CD8+ TRM cells as a frontline defense against HIV-1 and HCV.
Author Contributions
DW and ZM conceived the initial drafts of the manuscript. BG-B, MM, AS, CR, RB, AL, and EG revised many parts of the manuscript and contributed to finalize the manuscript.
Funding
The following grants have supported the work conducted in our laboratories and cited in the manuscript: From the National Health and Medical Research Council (NHMRC): grants APP1026293 (EG), APP525431 (CR), APP543139 (EG), and APP543143 (EG). From the Australian Centre for HIV and Hepatitis Virology Research, CR and EG have received an EOI grant. From The Hospital Research Foundation (THRF) and the Channel 7 Children's Research Foundation, DW received a project grant.
Conflict of Interest Statement
The authors declare that the research was conducted in the absence of any commercial or financial relationships that could be construed as a potential conflict of interest.
Acknowledgments
THRF have provided early career fellowships for DW and AS. AL is supported by a Fellowship from the NHMRC (NHMRC; No. 1043067).
References
Abbink, P., Larocca, R. A., Visitsunthorn, K., Boyd, M., De La Barrera, R. A., Gromowski, G. D., et al. (2017). Durability and correlates of vaccine protection against Zika virus in rhesus monkeys. Sci. Transl. Med. 9:eaao4163. doi: 10.1126/scitranslmed.aao4163
Ackerman, M. E., Mikhailova, A., Brown, E. P., Dowell, K. G., Walker, B. D., Bailey-Kellogg, C., et al. (2016). Polyfunctional HIV-specific antibody responses are associated with spontaneous HIV control. PLoS Pathog. 12:e1005315. doi: 10.1371/journal.ppat.1005315
Bailey, J. R., Barnes, E., and Cox, A. L. (2019). Approaches, progress, and challenges to hepatitis C vaccine development. Gastroenterology 156, 418–4430. doi: 10.1053/j.gastro.2018.08.060
Bailey, J. R., Flyak, A. I., Cohen, V. J., Li, H., Wasilewski, L. N., Snider, A. E., et al. (2017). Broadly neutralizing antibodies with few somatic mutations and hepatitis C virus clearance. JCI Insight 2:e92872. doi: 10.1172/jci.insight.92872
Bakari, M., Aboud, S., Nilsson, C., Francis, J., Buma, D., Moshiro, C., et al. (2011). Broad and potent immune responses to a low dose intradermal HIV-1 DNA boosted with HIV-1 recombinant MVA among healthy adults in Tanzania. Vaccine 29, 8417–8428. doi: 10.1016/j.vaccine.2011.08.001
Barouch, D. H., Alter, G., Broge, T., Linde, C., Ackerman, M. E., Brown, E. P., et al. (2015). Protective efficacy of adenovirus/protein vaccines against SIV challenges in rhesus monkeys. Science 349, 320–324. doi: 10.1126/science.aab3886
Barouch, D. H., Tomaka, F. L., Wegmann, F., Stieh, D. J., Alter, G., Robb, M. L., et al. (2018). Evaluation of a mosaic HIV-1 vaccine in a multicentre, randomised, double-blind, placebo-controlled, phase 1/2a clinical trial (APPROACH) and in rhesus monkeys (NHP 13-19). Lancet 392, 232–243. doi: 10.1016/S0140-6736(18)31364-3
Baumert, T. F., Fauvelle, C., Chen, D. Y., and Lauer, G. M. (2014). A prophylactic hepatitis C virus vaccine: a distant peak still worth climbing. J. Hepatol. 61(1 Suppl.), S34–S44. doi: 10.1016/j.jhep.2014.09.009
Belyakov, I. M., and Ahlers, J. D. (2012). Mucosal immunity and HIV-1 infection: applications for mucosal AIDS vaccine development. Curr. Top. Microbiol. Immunol. 354:157–179. doi: 10.1007/82_2010_119
Beura, L. K., Mitchell, J. S., Thompson, E. A., Schenkel, J. M., Mohammed, J., Wijeyesinghe, S., et al. (2018). Intravital mucosal imaging of CD8(+) resident memory T cells shows tissue-autonomous recall responses that amplify secondary memory. Nat. Immunol. 19, 173–182. doi: 10.1038/s41590-017-0029-3
Borducchi, E. N., Cabral, C., Stephenson, K. E., Liu, J., Abbink, P., Ng'ang'a, D., et al. (2016). Ad26/MVA therapeutic vaccination with TLR7 stimulation in SIV-infected rhesus monkeys. Nature 540, 284–287. doi: 10.1038/nature20583
Borgia, S. M., Hedskog, C., Parhy, B., Hyland, R. H., Stamm, L. M., Brainard, D. M., et al. (2018). Identification of a novel hepatitis C virus genotype from Punjab, India: expanding classification of hepatitis C virus into 8 genotypes. J. Infect. Dis. 218, 1722–1729. doi: 10.1093/infdis/jiy401
Bull, R. A., Luciani, F., McElroy, K., Gaudieri, S., Pham, S. T., Chopra, A., et al. (2011). Sequential bottlenecks drive viral evolution in early acute hepatitis C virus infection. PLoS Pathog. 7:e1002243. doi: 10.1371/journal.ppat.1002243
Burton, D. R., and Hangartner, L. (2016). Broadly neutralizing antibodies to HIV and their role in vaccine design. Annu. Rev. Immunol. 34, 635–659. doi: 10.1146/annurev-immunol-041015-055515
Cafaro, A., Titti, F., Fracasso, C., Maggiorella, M. T., Baroncelli, S., Caputo, A., et al. (2001). Vaccination with DNA containing tat coding sequences and unmethylated CpG motifs protects cynomolgus monkeys upon infection with simian/human immunodeficiency virus (SHIV89.6P). Vaccine 19, 2862–2877. doi: 10.1016/S0264-410X(01)00002-0
Churchyard, G. J., Morgan, C., Adams, E., Hural, J., Graham, B. S., Moodie, Z., et al. (2011). A phase IIA randomized clinical trial of a multiclade HIV-1 DNA prime followed by a multiclade rAd5 HIV-1 vaccine boost in healthy adults (HVTN204). PLoS ONE 6:e21225. doi: 10.1371/journal.pone.0021225
Cihlar, T., and Fordyce, M. (2016). Current status and prospects of HIV treatment. Curr. Opin. Virol. 18:50–56. doi: 10.1016/j.coviro.2016.03.004
Cuburu, N., Graham, B. S., Buck, C. B., Kines, R. C., Pang, Y. Y., Day, P. M., et al. (2012). Intravaginal immunization with HPV vectors induces tissue-resident CD8+ T cell responses. J. Clin. Invest. 122, 4606–4620. doi: 10.1172/jci63287
Cuburu, N., Khan, S., Thompson, C. D., Kim, R., Vellinga, J., Zahn, R., et al. (2018). Adenovirus vector-based prime-boost vaccination via heterologous routes induces cervicovaginal CD8(+) T cell responses against HPV16 oncoproteins. Int. J. Cancer 142, 1467–1479. doi: 10.1002/ijc.31166
Cuburu, N., Wang, K., Goodman, K. N., Pang, Y. Y., Thompson, C. D., Lowy, D. R., et al. (2015). Topical herpes simplex virus 2 (HSV-2) vaccination with human papillomavirus vectors expressing gB/gD ectodomains induces genital-tissue-resident memory CD8+ T cells and reduces genital disease and viral shedding after HSV-2 challenge. J. Virol. 89, 83–96. doi: 10.1128/jvi.02380-14
Davies, B., Prier, J. E., Jones, C. M., Gebhardt, T., Carbone, F. R., Mackay, L. K. (2017). Cutting edge: tissue-resident memory T cells generated by multiple immunizations or localized deposition provide enhanced immunity. J. Immunol. 198, 2233–2237. doi: 10.4049/jimmunol.1601367
Epstein, J. E., Tewari, K., Lyke, K. E., Sim, B. K., Billingsley, P. F., Laurens, M. B., et al. (2011). Live attenuated malaria vaccine designed to protect through hepatic CD8(+) T cell immunity. Science 334, 475–480. doi: 10.1126/science.1211548
Fernandez-Ruiz, D., Ng, W. Y., Holz, L. E., Ma, J. Z., Zaid, A., Wong, Y. C., et al. (2016). Liver-resident memory CD8(+) T cells form a front-line defense against malaria liver-stage infection. Immunity 45, 889–902. doi: 10.1016/j.immuni.2016.08.011
Ferraro, B., Morrow, M. P., Hutnick, N. A., Shin, T. H., Lucke, C. E., and Weiner, D. B. (2011). Clinical applications of DNA vaccines: current progress. Clin. Infect. Dis. 53, 296–302. doi: 10.1093/cid/cir334
Frahm, N., DeCamp, A. C., Friedrich, D. P., Carter, D. K., Defawe, O. D., Kublin, J. G., et al. (2012). Human adenovirus-specific T cells modulate HIV-specific T cell responses to an Ad5-vectored HIV-1 vaccine. J. Clin. Invest. 122, 359–367. doi: 10.1172/jci60202
Garmory, H. S., Brown, K. A., and Titball, R. W. (2003). DNA vaccines: improving expression of antigens. Genet. Vaccines Ther. 1:2. doi: 10.1186/1479-0556-1-2
Gaudinski, M. R., Houser, K. V., Morabito, K. M., Hu, Z., Yamshchikov, G., Rothwell, R. S., et al. (2018). Safety, tolerability, and immunogenicity of two Zika virus DNA vaccine candidates in healthy adults: randomised, open-label, phase 1 clinical trials. Lancet 391, 552–562. doi: 10.1016/S0140-6736(17)33105-7
Gebhardt, T., Wakim, L. M., Eidsmo, L., Reading, P. C., Heath, W. R., and Carbone, F. R. (2009). Memory T cells in nonlymphoid tissue that provide enhanced local immunity during infection with herpes simplex virus. Nat. Immunol. 10, 524–530. doi: 10.1038/ni.1718
German Advisory Committee Blood SAoPTbB. (2016). Human Immunodeficiency Virus (HIV). Transfus. Med. Hemother. 43, 203–222. doi: 10.1159/000445852
Gill, U. S., Pallett, L. J., Kennedy, P. T. F., and Maini, M. K. (2018a). Liver sampling: a vital window into HBV pathogenesis on the path to functional cure. Gut 67, 767–775. doi: 10.1136/gutjnl-2017-314873
Gill, U. S., Pallett, L. J., Thomas, N., Burton, A. R., Patel, A. A., Yona, S., et al. (2018b). Fine needle aspirates comprehensively sample intrahepatic immunity. Gut. 1-11 doi: 10.1136/gutjnl-2018-317071
Gola, A., Silman, D., Walters, A. A., Sridhar, S., Uderhardt, S., Salman, A. M., et al. (2018). Prime and target immunization protects against liver-stage malaria in mice. Sci. Transl. Med. 10:eaap9128. doi: 10.1126/scitranslmed.aap9128
Grebely, J., Prins, M., Hellard, M., Cox, A. L., Osburn, W. O., Lauer, G., et al. (2012). Hepatitis C virus clearance, reinfection, and persistence, with insights from studies of injecting drug users: towards a vaccine. Lancet Infect. Dis. 12, 408–414. doi: 10.1016/s1473-3099(12)70010-5
Grubor-Bauk, B., Yu, W., Wijesundara, D., Gummow, J., Garrod, T., Brennan, A. J., et al. (2016). Intradermal delivery of DNA encoding HCV NS3 and perforin elicits robust cell-mediated immunity in mice and pigs. Gene Ther. 23, 26–37. doi: 10.1038/gt.2015.86
Gummow, J., Li, Y., Yu, W., Garrod, T., Wijesundara, D., Brennan, A. J., et al. (2015). A multiantigenic DNA vaccine that induces broad hepatitis C virus-specific T-cell responses in mice. J. Virol. 89, 7991–8002. doi: 10.1128/jvi.00803-15
Hansen, S. G., Ford, J. C., Lewis, M. S., Ventura, A. B., Hughes, C. M., Coyne-Johnson, L., et al. (2011). Profound early control of highly pathogenic SIV by an effector memory T-cell vaccine. Nature 473, 523–527. doi: 10.1038/nature10003
Hartnell, F., Brown, A., Capone, S., Kopycinski, J., Bliss, C., Makvandi-Nejad, S., et al. (2018). A novel vaccine strategy employing serologically different chimpanzee adenoviral vectors for the prevention of HIV-1 and HCV coinfection. Front. Immunol. 9:3175. doi: 10.3389/fimmu.2018.03175
Haynes, B. F., Gilbert, P. B., McElrath, M. J., Zolla-Pazner, S., Tomaras, G. D., Alam, S. M., et al. (2012). Immune-correlates analysis of an HIV-1 vaccine efficacy trial. N. Engl. J. Med. 366, 1275–1286. doi: 10.1056/NEJMoa1113425
Hayton, E. J., Rose, A., Ibrahimsa, U., Del Sorbo, M., Capone, S., Crook, A., et al. (2014). Safety and tolerability of conserved region vaccines vectored by plasmid DNA, simian adenovirus and modified vaccinia virus ankara administered to human immunodeficiency virus type 1-uninfected adults in a randomized, single-blind phase I trial. PLoS ONE 9:e101591. doi: 10.1371/journal.pone.0101591
Holz, L. E., Prier, J. E., Freestone, D., Steiner, T. M., English, K., Johnson, D. N., et al. (2018). CD8(+) T cell activation leads to constitutive formation of liver tissue-resident memory T cells that seed a large and flexible niche in the liver. Cell Rep. 25, 68–79 e4. doi: 10.1016/j.celrep.2018.08.094
Houghton, M. (2011). Prospects for prophylactic and therapeutic vaccines against the hepatitis C viruses. Immunol. Rev. 239, 99–108. doi: 10.1111/j.1600-065X.2010.00977.x
Ishizuka, A. S., Lyke, K. E., DeZure, A., Berry, A. A., Richie, T. L., Mendoza, F. H., et al. (2016). Protection against malaria at 1 year and immune correlates following PfSPZ vaccination. Nat. Med. 22, 614–623. doi: 10.1038/nm.4110
Jechlinger, W. (2006). Optimization and delivery of plasmid DNA for vaccination. Expert Rev. Vaccines 5, 803–825. doi: 10.1586/14760584.5.6.803
Jin, X., Morgan, C., Yu, X., DeRosa, S., Tomaras, G. D., Montefiori, D. C., et al. (2015). Multiple factors affect immunogenicity of DNA plasmid HIV vaccines in human clinical trials. Vaccine 33, 2347–2353. doi: 10.1016/j.vaccine.2015.03.036
Jorritsma, S. H. T., Gowans, E. J., Grubor-Bauk, B., and Wijesundara, D. K. (2016). Delivery methods to increase cellular uptake and immunogenicity of DNA vaccines. Vaccine 34, 5488–5494. doi: 10.1016/j.vaccine.2016.09.062
Joseph, S. B., Swanstrom, R., Kashuba, A. D., and Cohen, M. S. (2015). Bottlenecks in HIV-1 transmission: insights from the study of founder viruses. Nat. Rev. Microbiol. 13, 414–425. doi: 10.1038/nrmicro3471
Kawakami, S., Fumoto, S., Nishikawa, M., Yamashita, F., and Hashida, M. (2000). In vivo gene delivery to the liver using novel galactosylated cationic liposomes. Pharm. Res. 17, 306–313. doi: 10.1023/A:1007501122611
Kibuuka, H., Kimutai, R., Maboko, L., Sawe, F., Schunk, M. S., Kroidl, A., et al. (2010). A Phase I/II study of a multiclade HIV-1 DNA plasmid prime and recombinant adenovirus-type 5 boost vaccine in HIV uninfected East Africans (RV 172). J. Infect. Dis. 201, 600–607. doi: 10.1086/650299
Kim, T. J., Jin, H. T., Hur, S. Y., Yang, H. G., Seo, Y. B., Hong, S. R., et al. (2014). Clearance of persistent HPV infection and cervical lesion by therapeutic DNA vaccine in CIN3 patients. Nat. Commun. 5:5317. doi: 10.1038/ncomms6317
Larocca, R. A., Abbink, P., Peron, J. P., Zanotto, P. M., Iampietro, M. J., Badamchi-Zadeh, A., et al. (2016). Vaccine protection against Zika virus from Brazil. Nature 536, 474–478. doi: 10.1038/nature18952
Latimer, B., Toporovski, R., Yan, J., Pankhong, P., Morrow, M. P., Khan, A. S., et al. (2014). Strong HCV NS3/4a, NS4b, NS5a, NS5b-specific cellular immune responses induced in Rhesus macaques by a novel HCV genotype 1a/1b consensus DNA vaccine. Hum. Vaccin. Immunother. 10, 2357–2365. doi: 10.4161/hv.29590
Liu, F., Lei, J., Vollmer, R., and Huang, L. (2004). Mechanism of liver gene transfer by mechanical massage. Mol. Ther. 9, 452–7. doi: 10.1016/j.ymthe.2003.12.003
MacGregor, R. R., Boyer, J. D., Ugen, K. E., Lacy, K. E., Gluckman, S. J., Bagarazzi, M. L., et al. (1998). First human trial of a DNA-based vaccine for treatment of human immunodeficiency virus type 1 infection: safety and host response. J. Infect. Dis. 178, 92–100.
MacGregor, R. R., Ginsberg, R., Ugen, K. E., Baine, Y., Kang, C. U., Tu, X. M., et al. (2002). T-cell responses induced in normal volunteers immunized with a DNA-based vaccine containing HIV-1 env and rev. AIDS 16, 2137–2143. doi: 10.1097/00002030-200211080-00005
MacKay, L. K., Stock, A. T., Ma, J. Z., Jones, C. M., Kent, S. J., Mueller, S. N., et al. (2012). Long-lived epithelial immunity by tissue-resident memory T (TRM) cells in the absence of persisting local antigen presentation. Proc. Natl. Acad. Sci. U.S.A. 109, 7037–7042. doi: 10.1073/pnas.1202288109
Masopust, D., Choo, D., Vezys, V., Wherry, E. J., Duraiswamy, J., Akondy, R., et al. (2010). Dynamic T cell migration program provides resident memory within intestinal epithelium. J. Exp. Med. 207, 553–564. doi: 10.1084/jem.20090858
Masopust, D., Vezys, V., Marzo, A. L., and Lefrançois, L. (2001). Preferential localization of effector memory cells in nonlymphoid tissue. Science 291, 2413–2417. doi: 10.1126/science.1058867
McMichael, A. J., and Koff, W. C. (2014). Vaccines that stimulate T cell immunity to HIV-1: the next step. Nat. Immunol. 15, 319–322. doi: 10.1038/ni.2844
Moyo, N., Borthwick, N. J., Wee, E. G., Capucci, S., Crook, A., Dorrell, L., et al. (2017). Long-term follow up of human T-cell responses to conserved HIV-1 regions elicited by DNA/simian adenovirus/MVA vaccine regimens. PLoS ONE 12:e0181382. doi: 10.1371/journal.pone.0181382
Mueller, S. N., and Mackay, L. K. (2016). Tissue-resident memory T cells: local specialists in immune defence. Nat. Rev. Immunol. 16, 79–89. doi: 10.1038/nri.2015.3
Muruganandah, V., Sathkumara, H. D., Navarro, S., and Kupz, A. (2018). A systematic review: the role of resident memory T cells in infectious diseases and their relevance for vaccine development. Front. Immunol. 9:1574. doi: 10.3389/fimmu.2018.01574
Nagata, T., Uchijima, M., Yoshida, A., Kawashima, M., and Koide, Y. (1999). Codon optimization effect on translational efficiency of DNA vaccine in mammalian cells: analysis of plasmid DNA encoding a CTL epitope derived from microorganisms. Biochem. Biophys. Res. Commun. 261, 445–451. doi: 10.1006/bbrc.1999.1050
Nganou-Makamdop, K., Ploemen, I., Behet, M., Van Gemert, G. J., Hermsen, C., Roestenberg, M., et al. (2012). Reduced Plasmodium berghei sporozoite liver load associates with low protective efficacy after intradermal immunization. Parasite Immunol. 34, 562–569. doi: 10.1111/pim.12000.x
Okuda, K., Xin, K. O., Tsuji, T., Bukawa, H., Tanaka, S., Koff, W. C., et al. (1997). DNA vaccination followed by macromolecular multicomponent peptide vaccination against HIV-1 induces strong antigen-specific immunity. Vaccine 15, 1049–1056.
Olsen, T. M., Stone, B. C., Chuenchob, V., and Murphy, S. C. (2018). Prime-and-Trap malaria vaccination to generate protective CD8(+) liver-resident memory T cells. J. Immunol. 201, 1984–1993. doi: 10.4049/jimmunol.1800740
Osburn, W. O., Snider, A. E., Wells, B. L., Latanich, R., Bailey, J. R., Thomas, D. L., et al. (2014). Clearance of hepatitis C infection is associated with the early appearance of broad neutralizing antibody responses. Hepatology 59, 2140–2151. doi: 10.1002/hep.27013
Pallett, L. J., Davies, J., Colbeck, E. J., Robertson, F., Hansi, N., Easom, N. J. W., et al. (2017). IL-2(high) tissue-resident T cells in the human liver: sentinels for hepatotropic infection. J. Exp. Med. 214, 1567–1580. doi: 10.1084/jem.20162115
Park, S. L., Zaid, A., Hor, J. L., Christo, S. N., Prier, J. E., Davies, B., et al. (2018). Local proliferation maintains a stable pool of tissue-resident memory T cells after antiviral recall responses. Nat. Immunol. 19, 183–191. doi: 10.1038/s41590-017-0027-5
Parsons, M. S., Lloyd, S. B., Lee, W. S., Kristensen, A. B., Amarasena, T., Center, R. J., et al. (2017). Partial efficacy of a broadly neutralizing antibody against cell-associated SHIV infection. Sci. Transl. Med. 9:eaaf1483. doi: 10.1126/scitranslmed.aaf1483
Platt, L., Easterbrook, P., Gower, E., McDonald, B., Sabin, K., McGowan, C., et al. (2016). Prevalence and burden of HCV co-infection in people living with HIV: a global systematic review and meta-analysis. Lancet Infect. Dis. 16, 797–808. doi: 10.1016/S1473-3099(15)00485-5
Pontesilli, O., Klein, M. R., Kerkhof-Garde, S. R., Pakker, N. G., de Wolf, F., Schuitemaker, H., et al. (1998). Longitudinal analysis of human immunodeficiency virus type 1-specific cytotoxic T lymphocyte responses: a predominant gag-specific response is associated with nonprogressive infection. J. Infect. Dis. 178, 1008–1018.
Rolland, M., Nickle, D. C., and Mullins, J. I. (2007). HIV-1 group M conserved elements vaccine. PLoS Pathog. 3:e157. doi: 10.1371/journal.ppat.0030157
Rosato, P. C., Beura, L. K., and Masopust, D. (2017). Tissue resident memory T cells and viral immunity. Curr. Opin. Virol. 22, 44–50. doi: 10.1016/j.coviro.2016.11.011
Sacks-Davis, R., Grebely, J., Dore, G. J., Osburn, W., Cox, A. L., Rice, T. M., et al. (2015). Hepatitis C virus reinfection and spontaneous clearance of reinfection–the InC3 study. J. Infect. Dis. 212, 1407–1419. doi: 10.1093/infdis/jiv220
Saez-Cirion, A., Lacabaratz, C., Lambotte, O., Versmisse, P., Urrutia, A., Boufassa, F., et al. (2007). HIV controllers exhibit potent CD8 T cell capacity to suppress HIV infection ex vivo and peculiar cytotoxic T lymphocyte activation phenotype. Proc. Natl. Acad. Sci. U.S.A. 104, 6776–6781. doi: 10.1073/pnas.0611244104
Sato, T., Thorlacius, H., Johnston, B., Staton, T. L., Xiang, W., Littman, D. R., et al. (2005). Role for CXCR6 in recruitment of activated CD8+ lymphocytes to inflamed liver. J. Immunol. 174, 277–283.doi: 10.4049/jimmunol.174.1.277
Schenkel, J. M., Fraser, K. A., Vezys, V., and Masopust, D. (2013). Sensing and alarm function of resident memory CD8(+) T cells. Nat. Immunol. 14, 509–513. doi: 10.1038/ni.2568
Shin, H., and Iwasaki, A. (2012). A vaccine strategy that protects against genital herpes by establishing local memory T cells. Nature 491, 463–467. doi: 10.1038/nature11522
Shin, S. Y. (2016). Recent update in HIV vaccine development. Clin. Exp. Vaccine Res. 5, 6–11. doi: 10.7774/cevr.2016.5.1.6
Smyk-Pearson, S., Tester, I. A., Klarquist, J., Palmer, B. E., Pawlotsky, J. M., Golden-Mason, L., et al. (2008). Spontaneous recovery in acute human hepatitis C virus infection: functional T-cell thresholds and relative importance of CD4 help. J. Virol. 82, 1827–1837. doi: 10.1128/jvi.01581-07
Stone, J., Martin, N. K., Hickman, M., Hellard, M., Scott, N., McBryde, E., et al. (2016). The potential impact of a hepatitis C vaccine for people who inject drugs: is a vaccine needed in the age of direct-acting antivirals? PLoS ONE 11:e0156213. doi: 10.1371/journal.pone.0156213
Sun, Y., Peng, S., Qiu, J., Miao, J., Yang, B., Jeang, J., et al. (2015). Intravaginal HPV DNA vaccination with electroporation induces local CD8+ T-cell immune responses and antitumor effects against cervicovaginal tumors. Gene Ther. 22, 528–535. doi: 10.1038/gt.2015.17
Swadling, L., Capone, S., Antrobus, R. D., Brown, A., Richardson, R., Newell, E. W., et al. (2014). A human vaccine strategy based on chimpanzee adenoviral and MVA vectors that primes, boosts, and sustains functional HCV-specific T cell memory. Sci. Transl. Med. 6:261ra153. doi: 10.1126/scitranslmed.3009185
Tavel, J. A., Martin, J. E., Kelly, G. G., Enama, M. E., Shen, J. M., Gomez, P. L., et al. (2007). Safety and immunogenicity of a Gag-Pol candidate HIV-1 DNA vaccine administered by a needle-free device in HIV-1–seronegative subjects. J. AIDS 44, 601–605. doi: 10.1097/QAI.0b013e3180417cb6
Tay, S. S., Wong, Y. C., McDonald, D. M., Wood, N. A., Roediger, B., Sierro, F., et al. (2014). Antigen expression level threshold tunes the fate of CD8 T cells during primary hepatic immune responses. Proc. Natl. Acad. Sci. U.S.A. 111, E2540–E2549. doi: 10.1073/pnas.1406674111
Tebas, P., Roberts, C. C., Muthumani, K., Reuschel, E. L., Kudchodkar, S. B., Zaidi, F. I., et al. (2017). Safety and immunogenicity of an anti-Zika virus DNA vaccine - preliminary report. N. Engl. J. Med. doi: 10.1056/NEJMoa1708120
Tomusange, K., Wijesundara, D., Gummow, J., Garrod, T., Li, Y., Gray, L., et al. (2016). A HIV-Tat/C4-binding protein chimera encoded by a DNA vaccine is highly immunogenic and contains acute EcoHIV infection in mice. Sci. Rep. 6:29131. doi: 10.1038/srep29131
Trimble, C. L., Morrow, M. P., Kraynyak, K. A., Shen, X., Dallas, M., Yan, J., et al. (2015). Safety, efficacy, and immunogenicity of VGX-3100, a therapeutic synthetic DNA vaccine targeting human papillomavirus 16 and 18 E6 and E7 proteins for cervical intraepithelial neoplasia 2/3: a randomised, double-blind, placebo-controlled phase 2b trial. Lancet 386, 2078–2088. doi: 10.1016/S0140-6736(15)00239-1
Tse, S. W., Radtke, A. J., Espinosa, D. A., Cockburn, I. A., and Zavala, F. (2014). The chemokine receptor CXCR6 is required for the maintenance of liver memory CD8(+) T cells specific for infectious pathogens. J. Infect. Dis. 210, 1508–16. doi: 10.1093/infdis/jiu281
UNAIDS (2018). Miles to Go- Closing Gaps, Breaking Barriers, Righting Injustices. Global AIDS update 2018.
Vanniasinkam, T., Reddy, S. T., and Ertl, H. C. J. (2006). DNA immunization using a non-viral promoter. Virology 344, 412–420. doi: 10.1016/j.virol.2005.08.040
Vujkovic-Cvijin, I., Dunham, R. M., Iwai, S., Maher, M. C., Albright, R. G., Broadhurst, M. J., et al. (2013). Dysbiosis of the gut microbiota is associated with HIV disease progression and tryptophan catabolism. Sci. Transl. Med. 5:193ra91. doi: 10.1126/scitranslmed.3006438
Wang, H. B., Mo, Q. H., and Yang, Z. (2015). HIV vaccine research: the challenge and the way forward. J. Immunol. Res. 2015:503978. doi: 10.1155/2015/503978
Weiland, O., Ahlen, G., Diepolder, H., Jung, M. C., Levander, S., Fons, M., et al. (2013). Therapeutic DNA vaccination using in vivo electroporation followed by standard of care therapy in patients with genotype 1 chronic hepatitis C. Mol. Ther. 21, 1796–1805. doi: 10.1038/mt.2013.119
WHO (2017). Global Hepatitis Report, 2017. Geneva: World Health Organisation. Available online at: https://www.who.int/hepatitis/publications/global-hepatitis-report2017/en/. (accessed November 06, 2018).
Wijesundara, D. K., Gummow, J., Li, Y., Yu, W., Quah, B. J., Ranasinghe, C., et al. (2018). Induction of genotype cross-reactive, hepatitis C virus-specific, cell-mediated immunity in DNA-vaccinated mice. J. Virol. 92:e02133-17. doi: 10.1128/jvi.02133-17
Yu, W., Grubor-Bauk, B., Gargett, T., Garrod, T., and Gowans, E. J. (2014). A novel challenge model to evaluate the efficacy of hepatitis C virus vaccines in mice. Vaccine 32, 3409–3416. doi: 10.1016/j.vaccine.2014.04.014
Keywords: DNA vaccine, hepatitis C, human immunodeficiency virus, HIV/AIDS, HCV, tissue-resident memory, T cell immunity
Citation: Mekonnen ZA, Grubor-Bauk B, Masavuli MG, Shrestha AC, Ranasinghe C, Bull RA, Lloyd AR, Gowans EJ and Wijesundara DK (2019) Toward DNA-Based T-Cell Mediated Vaccines to Target HIV-1 and Hepatitis C Virus: Approaches to Elicit Localized Immunity for Protection. Front. Cell. Infect. Microbiol. 9:91. doi: 10.3389/fcimb.2019.00091
Received: 23 November 2018; Accepted: 14 March 2019;
Published: 03 April 2019.
Edited by:
Alberto Moreno, Emory University School of Medicine, United StatesReviewed by:
Leo J. Swadling, University College London, United KingdomAntonella Folgori, ReiThera Srl, Italy
Copyright © 2019 Mekonnen, Grubor-Bauk, Masavuli, Shrestha, Ranasinghe, Bull, Lloyd, Gowans and Wijesundara. This is an open-access article distributed under the terms of the Creative Commons Attribution License (CC BY). The use, distribution or reproduction in other forums is permitted, provided the original author(s) and the copyright owner(s) are credited and that the original publication in this journal is cited, in accordance with accepted academic practice. No use, distribution or reproduction is permitted which does not comply with these terms.
*Correspondence: Danushka K. Wijesundara, ZGFudXNoa2Eud2lqZXN1bmRhcmFAYWRlbGFpZGUuZWR1LmF1