- National Centre for Cell Science, Pune, India
Leishmania parasites possess an exceptional oxidant and chemical defense mechanism, involving a very unique small molecular weight thiol, trypanothione (T[SH]2), that helps the parasite to manage its survival inside the host macrophage. The reduced state of T[SH]2 is maintained by NADPH-dependent trypanothione reductase (TryR) by recycling trypanothione disulfide (TS2). Along with its most important role as central reductant, T[SH]2 have also been assumed to regulate the activation of iron-sulfur cluster proteins (Fe/S). Fe/S clusters are versatile cofactors of various proteins and execute a much broader range of essential biological processes viz., TCA cycle, redox homeostasis, etc. Although, several Fe/S cluster proteins and their roles have been identified in Leishmania, some of the components of how T[SH]2 is involved in the regulation of Fe/S proteins remains to be explored. In pursuit of this aim, a systems biology approach was undertaken to get an insight into the overall picture to unravel how T[SH]2 synthesis and reduction is linked with the regulation of Fe/S cluster proteins and controls the redox homeostasis at a larger scale. In the current study, we constructed an in silico kinetic model of T[SH]2 metabolism. T[SH]2 reduction reaction was introduced with a perturbation in the form of its inhibition to predict the overall behavior of the model. The main control of reaction fluxes were exerted by TryR reaction rate that affected almost all the important reactions in the model. It was observed that the model was more sensitive to the perturbation introduced in TryR reaction, 5 to 6-fold. Furthermore, due to inhibition, the T[SH]2 synthesis rate was observed to be gradually decreased by 8 to 14-fold. This has also caused an elevated level of free radicals which apparently affected the activation of Fe/S cluster proteins. The present kinetic model has demonstrated the importance of T[SH]2 in leishmanial cellular redox metabolism. Hence, we suggest that, by designing highly potent and specific inhibitors of TryR enzyme, inhibition of T[SH]2 reduction and overall inhibition of most of the downstream pathways including Fe/S protein activation reactions, can be accomplished.
Introduction
Cutaneous leishmaniasis (CL), the most common form of leishmaniasis, has always been neglected as a major public health problem due to its non-fatality. The severity of the disease is in terms of disfigurement and residual scars. The causative agent of CL, a protozoan parasite, Leishmania major has a digenetic lifecycle and lives in two hosts, sandfly and human, in the form of flagellated promastigotes and non-flagellated amastigotes, respectively.
Inside the mammalian host, the parasite lives in the lethal enzymatic environment of macrophage cells, where they have to deal with the macrophage generated oxidative stress to survive. Remarkably, their survival is contributed by a very unique redox metabolism that the parasite has evolved with. The defense machinery of the parasite involves one main central unusual thiol reductant, trypanothione (N1,N8-bis-glutathionylspermidine; T[SH]2) (Fairlamb and Cerami, 1985; Fairlamb et al., 1985). T[SH]2 is synthesized by a bifunctional trypanothione synthetase (TryS) that covalently attaches two molecules of glutathione (GSH) onto one molecule of spermidine (Spd) in a two-step process. T[SH]2 plays a pivotal role in carrying out a number of many important cellular functions, such as detoxification of H2O2 and metals, drug resistance (e.g., antimonials) (Borst and Ouellette, 1995; Mukhopadhyay et al., 1996; Wyllie et al., 2004) and defense against chemical and oxidant stress, maintaining the redox balance by protein disulfide reduction and indirect synthesis of deoxyribonucleotide (Fairlamb and Cerami, 1985; Krauth-Siegel and Lüdemann, 1996; Flohe et al., 1999; Dormeyer et al., 2001). Further, the reduced state of T[SH]2 is maintained by NADPH-dependent trypanothione reductase (TryR), a unique dimeric flavoenzyme, which recycles trypanothione disulfide (TS2) back to T[SH]2 (Krauth-Siegel et al., 1987; Fairlamb et al., 1989; Nogoceke et al., 1997; Fairlamb, 1999; Montemartini et al., 2000; Hofmann et al., 2001; Flohé et al., 2002).
Previous studies have demonstrated the essentiality of TryR for parasite survival and due to its non-existence in mammals, it has been validated as an attractive therapeutic target (Dumas et al., 1997; Tovar et al., 1998a,b; Krieger et al., 2000; Krauth-Siegel et al., 2003; Krauth-Siegel and Comini, 2008; Holloway et al., 2009). The functional analog enzyme for TryR in a mammalian host is glutathione reductase (GR) (Krauth-Siegel and Inhoff, 2003; Krauth-Siegel et al., 2003). Although, TryR and human GR have similar catalytic mechanisms, they are specific to their respective disulfide substrates (Marsh and Bradley, 1997). Numerous crystallographic studies revealed that TryR remains active in a homodimeric form (Shames et al., 1986; Krauth-Siegel et al., 1987; Baiocco et al., 2013) and the catalytic site is contributed from both the subunits forming two regions, the NADP site (N-site) and the active site (G-site). Interestingly, their crossed complexes, TryR-GSSG and GR-T(S)2 were found non-catalytic (Shames et al., 1986; Krauth-Siegel et al., 1987). Moreover, the binding site in TryR is wider and more hydrophobic exhibiting an overall negative charge (Kuriyan et al., 1991; Hunter et al., 1992; Stoll et al., 1997). All these features raise the possibility to inhibit TR selectively without affecting the host's machinery.
Other than these two enzymes, a thioredoxin (Trx) like tryparedoxin (TXN) (Qi and Grishin, 2005), an oxidoreductase, is also the main component of the leishmanial redox system that helps in the activation of many important enzymes through electron shuttling from T[SH]2 (Nogoceke et al., 1997; Dormeyer et al., 2001). Glutaredoxins (Grxs) are other ubiquitous small thiol-disulfide oxidoreductases that play crucial roles in the redox homeostasis of the cell by participating in a large number of biological processes involving cellular redox and iron sulfur (Fe/S) metabolism. In mammals, Grxs are GSH dependent, however in trypanosomatids it was found that T[SH]2 is the main reducing agent for leishmanial Grxs (Figure 1) (Ceylan et al., 2010).
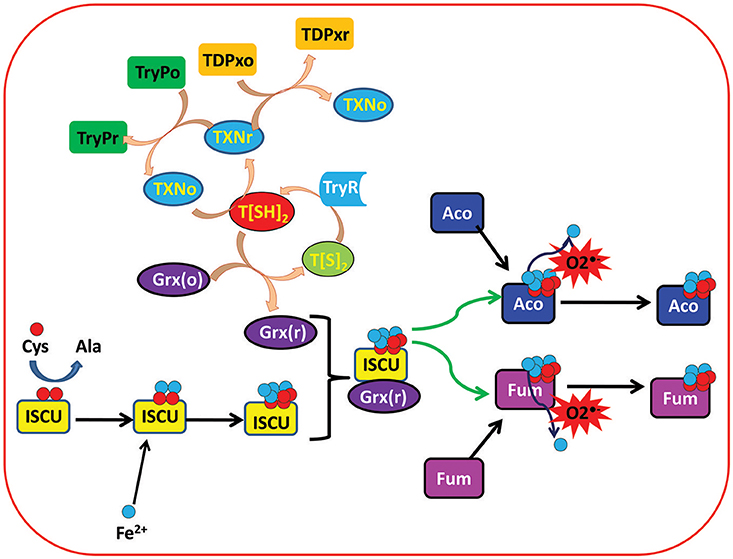
Figure 1. Depicting the important role of trypanothione (T[SH]2) in regulation of ISC proteins in Leishmania parasite. Trypanothione disulfide (T[S]2) is reduced to Trypanothione (T[SH]2) with the help of Trypanothione reductase (TryR) enzyme. Reduced trypanothione helps in reduction of different enzymes and proteins including Tryparedoxins (TXN), Tryparedoxin Peroxidases (TryP), Tryparedoxin-dependent Peroxidases (TDPx), and Glutaredoxins (Grx). On the other hand, Iron sulfur cluster unit (ISCU) obtains sulfur (S) and iron (Fe2+) from cysteine and extracellular environment, respectively, and binds with reduced Grx to form Grx(r)-ISCU[4Fe-4S] complex. Further, Grx(r)-ISCU[4Fe-4S] activates ISC proteins (Aconitase and Fumarase) by transferring its [4Fe-4S] unit to these proteins/enzymes. Under oxidative stress conditions, free radicals like Superoxide free radicals () reacts with activated ISC proteins/enzymes and leaches out one Fe+3 ion. That results in inactivation of the proteins/enzymes.
Structurally, on the basis of the presence of a number of cysteine residues in the conserved motif Grxs are classified as: dithiol Grxs (2-C-Grx) containing a CXXC active site and monothiol Grxs (1-C-Grx) with CXXS motif. 2-C-Grxs play an important role in catalyzing the deglutathionylation of protein disulfides, whereas this function is lacking in 1-C-Grxs (Rahlfs et al., 2001; Tamarit et al., 2003; Deponte et al., 2005; Fernandes et al., 2005; Filser et al., 2008; Johansson et al., 2011). Recently, Fe/S clusters (iron sulfur cluster; ISC) binding ability of Grxs has been identified in trypanosomes including Leishmania (Fernandes et al., 2005; Fladvad et al., 2005; Berndt et al., 2007; Picciocchi et al., 2007; Comini et al., 2008; Iwema et al., 2009; Luo et al., 2010; Johansson et al., 2011; Yeung et al., 2011). This ISC binding ability of both the Grxs is not yet utilized in redox metabolism. These generally play an important role in the regulation of their oxidoreductase activity or to facilitate ISC transfer to other acceptor proteins for the biogenesis of Fe/S proteins. ISC are simple protein cofactors with iron-sulfur moieties and are involved in many biological processes (TCA cycle, etc.). To date, four ISC protein systems have been reported including nitrogen fixing (NIF), ISC, cytosolic iron sulfur protein assembly (CIA), and sulfur mobilization (Lill, 2009).
In yeast, Grx3/4 are reported to be involved in iron homeostasis and play an important role as iron sensors in which they are involved in modulation of Fe/S mediated interactions of Grxs with nuclear transcription factors (Li et al., 2009; Mühlenhoff et al., 2010). However, since, trypanosomes are almost lacking transcription factors, it seems highly unlikely for Grxs to play a similar role in these parasites (Clayton and Shapira, 2007).
T[SH]2 being the central efficient reducing agent of Grxs, and its indirect involvement in the activation/biogenesis of ISCs, new possibilities of exploring the iron homeostasis and its relation to redox metabolism have opened new insights for unexplored roles of TryR (Figure 1).
Due to unacceptable levels of drug resistance and high toxicity, there are increased demands to explore new drugs and drug targets against CL. From the above discussion, it is perceivable that the redox metabolism of Leishmania is an interesting choice of study due to many enzymes of principal importance. Several of them are already being investigated as potential drug targets from a metabolic as well as a druggability point of view (Flohé, 2012; Olin-Sandoval et al., 2012). Nowadays, systems biology approaches (an integration of mathematical methods and computational approaches) are being utilized to study systems dynamics of complex redox metabolic pathways in many organisms, such as, Escherichia coli, Saccharomyces cerevisiae (Beiting and Roos, 2007; Toledano et al., 2007; Nielsen and Jewett, 2008; Pillay et al., 2013). Redox systems have been characterized from systems biology perspective using top-down and bottom-up approaches. Moreover, -omics data is also being integrated to construct metabolic kinetic models to explore the complex mechanisms (Pillay et al., 2013; Go et al., 2014).
In the present work, we have tried to elucidate the underlying mechanism of how T[SH]2 directly or indirectly affects the cellular redox metabolism of the parasite by playing a role in activation and influencing the ISC protein biogenesis. The complexity of multi-enzymatic network has led us to construct a kinetic model of ISC protein biogenesis-trypanothione-Grxs relationship. Comprehensive analysis of the models has presented interesting insights related to the redox biology of the parasite.
Materials and Methods
Kinetic Modeling and Computer Simulation
All the models were constructed in Cell Designer and exported as Systems biology Markup language (SMBL) file. These SBML files were imported in COPASI version 4.19.140 (Hoops et al., 2006), a simulator for biochemical networks (Figures 2, 3). Each reaction was assigned by appropriate kinetic laws and kept as an irreversible reaction, for uniformity in the model (Table 1, Tables S1, S2). Non-enzymatic reactions were assigned with mass action kinetics, while for enzymatic reactions, Michaelis-Menten rate law; Hill kinetics and Ping-pong kinetics, as applicable, were assigned. The concentrations in the model are in micromolar (μM), and the time unit is in seconds. Fluxes of individual reactions within the model are in μM/s. All the kinetic parameters were obtained (or calculated) from published literature except the hypothetical value of inhibition constant (Ki) for TryR. After model construction, time course simulation and other analyses were performed.
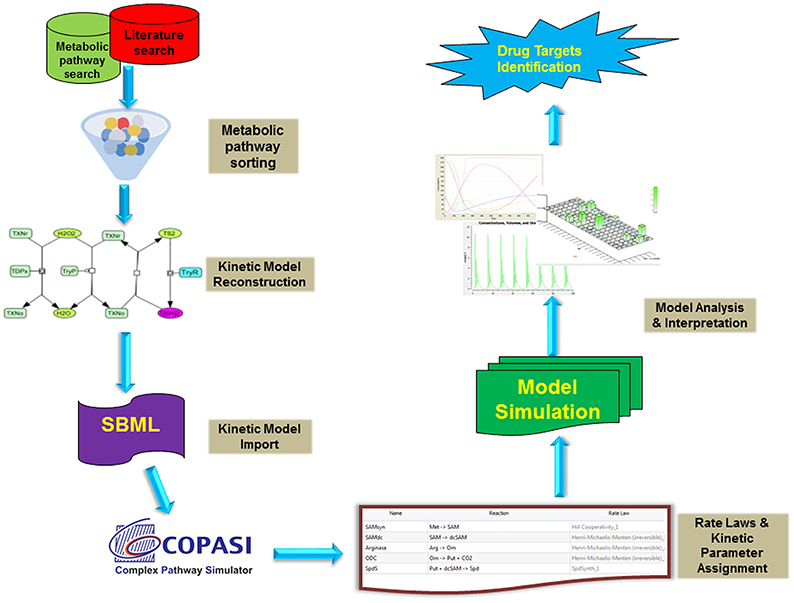
Figure 2. Typical workflow strategies adopted for the kinetic modeling and analysis. Briefly, the metabolic reaction data were retrieved from various databases (LeishCyc, KEGG, etc.) and literature. These pathways were then reconstructed in Cell Designer and exported as SBML model. Further, The SBML model was imported into COPASI and assigned with proper rate laws and parameters followed by its time course kinetic simulation. The model was analyzed and interpreted to propose imported drug targets.
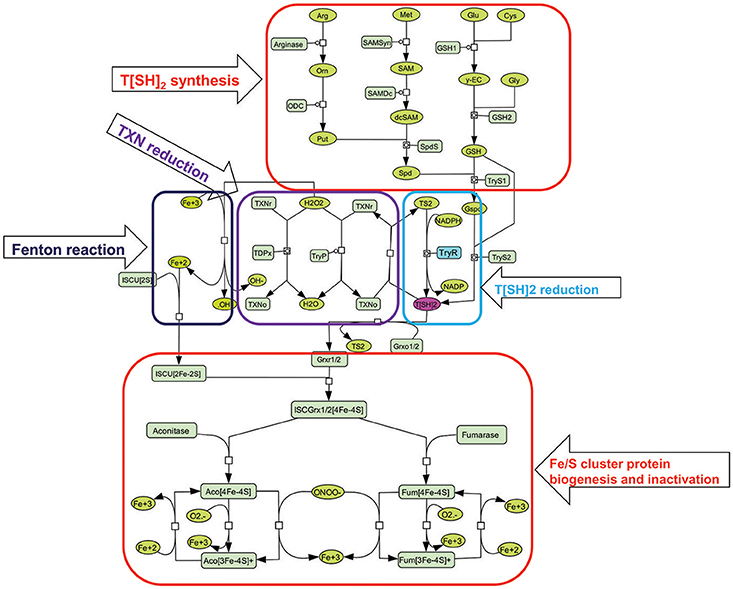
Figure 3. Illustration of kinetic model used for constructing perturbed models. T[SH]2 is synthesized from different pathways involving key amino acids [arginine (arg), methionine (met), glutamate (glu), cysteine (cys), glycine (gly)] (Trypanothione (T[SH]2) synthesis). It is also obtained from the reduction of Trypanothione disulfide (T[S]2) with the help of Trypanothione reductase (TryR) enzyme (T[SH]2 reduction). Furthermore, T[SH]2 reduces Tryparedoxins (TXN) that is, further, utilized to neutralize hydrogen peroxide (H2O2) in Tryparedoxin Peroxidases (TryP) or Tryparedoxin-dependent Peroxidases (TDPx) mediated catalyzed reaction (Tryparedoxin (TXN) reduction). This reaction releases free hydroxyl (OH−) ion. OH− is then converted to free hydroxyl radical (•OH) ions through Fenton reaction involving Fe3+ ion. On the other hand, Iron sulfur cluster unit (ISCU [4Fe-4S]) is formed (Figure 2), and binds with reduced Grx to form Grx(r)-ISCU[4Fe-4S] complex. Further, Grx(r)-ISCU[4Fe-4S] activates ISC proteins (Aconitase and Fumarase) by transferring its [4Fe-4S] unit to these enzymes. Under oxidative stress conditions, free radicals like Superoxide free radicals () and peroxynitrite (ONOO−) reacts with activated aconitase[4Fe-4S] and fumarase[4Fe-4S] and inactivates them by removing one Fe+3 ion.
Parameter Scanning and Sensitivity Analysis
In order to observe the effect of different parameters and concentrations on the model, parameter scanning and sensitivity analysis were performed. The reconstructed network was numerically simulated using Deterministic LSODA ODE solver (Hindmarsh, 1983) for defining the mathematical framework of the model.
Result and Discussion
Once inside the host macrophage, the survival of leishmanial parasite is mainly contributed by a unique redox metabolism that involves one main low molecular weight thiol molecule, T[SH]2 (Fairlamb and Cerami, 1992; Flohe et al., 1999; Dormeyer et al., 2001), that is synthesized by TryS enzyme. However, after performing its function as a main reductant of many enzymes, proteins and other small molecules, it is converted to its oxidized state, TS2. To balance between the T[SH]2- TS2 states, TS2 is reduced by an important enzyme, TryR. As mentioned earlier, T[SH]2 plays various important roles in a number of processes, such as, regulation of thiol redox balance, etc. Other than these, activation and regulation of Fe/S cluster proteins is another essential task in which T[SH]2 has played an important role (Fairlamb and Cerami, 1992; Flohe et al., 1999; Dormeyer et al., 2001) (Figures 1, 3). In order to understand the role of T[SH]2, a systems biology approach was adopted through which we tried to get an overall insight of how redox homeostasis is maintained in Leishmania.
Kinetic Model Reconstruction
In our models, we have introduced reactions for the synthesis of T[SH]2 via arginine, glycine, glutamate and methionine. The demand for T[SH]2 were included in the form of reduction reactions of Grx1/2, TXN, TDPx, and TryP. Grx1/2 is further known to activate other Fe/S cluster proteins (Clayton and Shapira, 2007). In Leishmania, there are many regulatory proteins/enzymes which are activated only after the assembly of Fe/S cluster in their respective binding scaffold. Aconitase and Fumarase are such ISC enzymes containing a cubane [4Fe-4S]2+ cluster. These enzymes were taken into account because of their well-established roles in energy metabolism and electron transport chain (ETC) mechanism. We assume, any kind of perturbation in T[SH]2 synthesis or reduction might directly affect their activation. Hence, to connect T[SH]2 with Fe/S cluster proteins, activation reactions of aconitase and fumarase were added as an important contribution from T[SH]2 pathway. Thus, the kinetic model for redox metabolism of Leishmania parasite was constructed using the metabolic simulator COPASI (Hoops et al., 2006) (http://www.copasi.org) by applying previously determined rate constants under physiological conditions for substrates of the pathway enzymes (Figure 2). All reactions were considered irreversible and the models were simulated using the LSODA algorithm. The details of the kinetic models are discussed in the sections below.
Basal Models
Model 1: This basal model has a total of 22 reactions, with 22 kinetic laws, 56 parameters, and 38 components (metabolites) (Figure 3; Table 2). In this model, reactions related to T[SH]2 synthesis pathway were included. Further, the demand reactions for T[SH]2 were added in the form of Grx1/2, TDPx, TryP, and TXN reduction. The model was further extended and included with Fe/S cluster proteins activation through ISCU-Grx1/2 mediated activators.
Model 2: Another basal model, Model 2, was constructed from Model 1 by connecting it to free radicals' mediated effect on Fe/S proteins. It has been studied that several oxidants such as, hydrogen peroxide, superoxide free radical, and peroxynitrite can inactivate ISC proteins by leaching out one labile iron from the cluster giving [3Fe-4S]+ cluster protein. Superoxide free radicals () and peroxynitrite (ONOO−) were shown to be particularly reactive with aconitase [4Fe-4S]2+ and fumarase [4Fe-4S]2+ (Castro et al., 1994; Crack et al., 2014). Rate constants at which ONOO− and inactivates aconitase are 1.4 × 105 M−1s−1 and 3.5 × 106 M−1s−1, respectively (Castro et al., 1994). Hence, reactions including ONOO− and mediated inactivation of aconitase and fumarase were added to Model 1. Further, considering the increased level of free iron after ISC inactivation, fenton reaction was also included in the model (Figure 3, Table 1, Tables S1–S3). As a result, Model 2 consists of a total of 29 reactions with 64 kinetic parameters and 45 metabolites.
Perturbed Models
Model 3 and Model 4: Modified models were constructed from Model 2 by adding perturbation at TryR reaction in the T[SH]2 reduction pathway. A hypothetical inhibitor was used as perturbation whose inhibition rate constant was kept ten times lower than the Km of T[SH]2 as per the experimentally determined range (Table S4). To check the effect of TryR inhibition on downstream reactions, competitive and non-competitive inhibition rate laws were assigned to construct Models 3 and 4. These models were consisting of 29 reactions with 64 parameters and 46 components (Tables 1, 2).
Time Course Simulation and Sensitivity Analysis of Kinetic Models
Time course simulation of basal models for a certain time period showed maximum production of T[SH]2 (Figure 4A), that saturated at an early time point in the model. The obtained concentration of T[SH]2 was further used to observe how the increasing T[SH]2 concentration can affect the level of Fe/S cluster protein activation. Parameter scanning of up to a 10-fold increased T[SH]2 concentration against Fe/S cluster proteins in model 1 demonstrated greater effect on Fe/S cluster proteins. The increased concentration of T[SH]2 has direct effect on Fe/S proteins, aconitase, and fumarase whose synthesis was gradually increased with the T[SH]2 level (Figure 4B) suggesting T[SH]2 mediated direct control on the activation of Fe/S proteins.
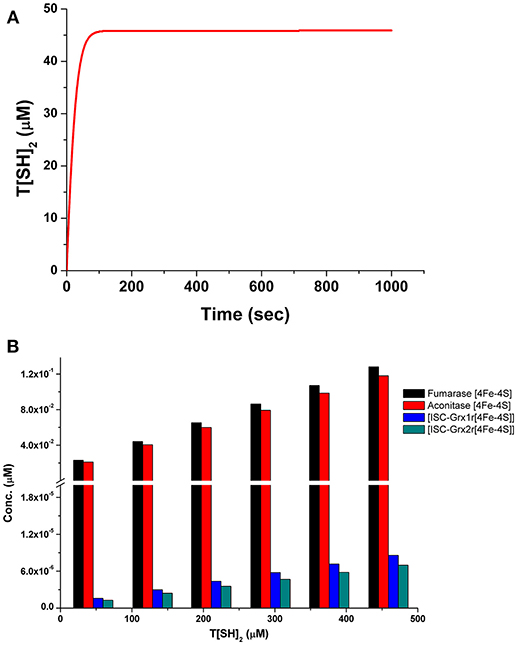
Figure 4. (A) Prediction of T[SH]2 concentration through time course simulation in basal kinetic model; (B) Prediction of the effect of increased concentration of T[SH]2 against Fe/S cluster proteins in basal model showing the gradual increase in their concentration.
Comparison of T[SH]2 synthesis rates among the models revealed that the synthesis rate was higher in model 1 and model 2 as compared to model 3 and model 4. The rate was gradually decreased, by 8 and 14-folds, in models 3 and 4, respectively, due to perturbed TryR catalyzed reaction (Figure 5A). Similarly, T[SH]2 concentrations were also found to be greatly affected due to the perturbation in models 3 and 4 (Figure 5B). Furthermore, other components of the models were also found to be influenced by inhibition applied in respective models (Figure 5C). Through our kinetic models, we were able to predict the effect of inhibition perturbation introduced in the TryR reaction. However, in vitro and in vivo validation of the same will provide a deeper understanding of the inhibition kinetics. A lot of literature related to the inhibition study of TryR enzyme has already been made available. These studies have already been able to demonstrate the effect of various inhibitors and their inhibition mechanisms against TryR enzyme (Jones et al., 2010; Beig et al., 2015; Saccoliti et al., 2017; Turcano et al., 2018). A previously published kinetic model in T. cruzi provided greater insights on the T[SH]2 synthesis and reduction pathways (Olin-Sandoval et al., 2012). They showed the significantly important roles of TryR and other enzymes under oxidative stress conditions and suggested the importance of specificity of the inhibitor against TryR. Through our models along with the previously published inhibition studies, we also suggest that if a TryR specific inhibitor is designed, it could greatly affect the activity of TryR as well as its downstream pathways because of the valuable role of T[SH]2.
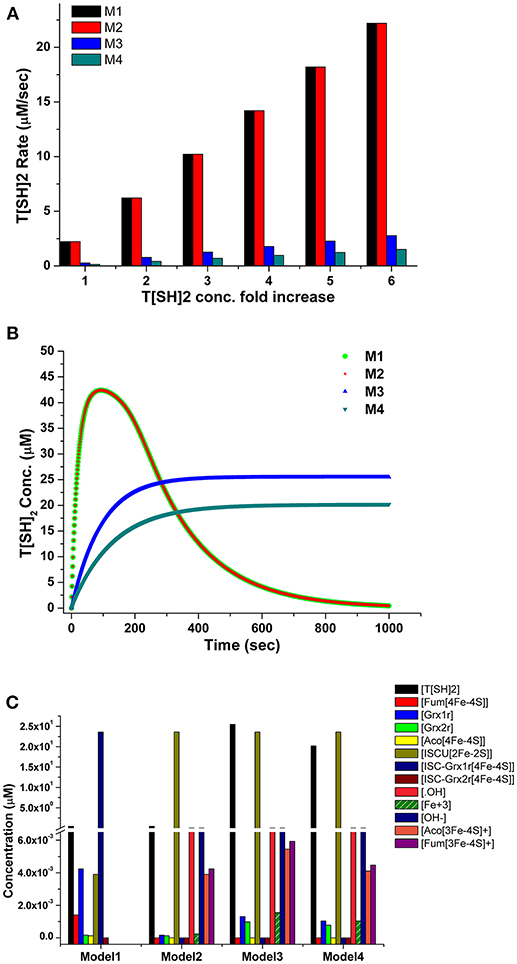
Figure 5. (A) Comparison of T[SH]2 production rates in four models with respect to the increased concentration of T[SH]2. (B) Comparison of T[SH]2 concentration in four models suggesting the decrease in its level in M3 and M4 models due to applied inhibition kinetics. (C) Concentrations of all components were compared in four models showed the level of inactivated aconitase and fumarase and free OH ions was increased.
It is worth mentioning here that, in 4Fe-4S cluster, only three of the iron atoms are attached to the protein, but the fourth iron interacts only with the sulfur of Fe/S cluster. This iron has a free coordination site that helps in its participation in substrate binding (Emptage et al., 1983; Robbins and Stout, 1989a,b). Henceforth, it is assumed that, when a free radical reacts with the Fe/S cluster protein/enzyme, it, perhaps, sequesters out this free iron from the cluster that subsequently converts the active [4Fe-4S] protein to an inactive [3Fe-4S] cluster protein. In our model, the introduction of ONOO− and ions has caused increased production of inactive [3Fe-4S] clusters from active aconitase[4Fe-4S] and fumarase[4Fe-4S] enzymes by releasing Fe3+ into the system. This Fe3+ ion directly increased the rate of Fenton reaction resulting in enhanced production of Fe2+, •OH and OH−. It was observed that the formation of ISCU [2Fe-2S] was possibly increased due to the higher level of accumulation of Fe2+ released from fenton reaction (Figure 5C).
The robustness i.e., the ability to cope with the kinetic perturbations to maintain the performance, of the kinetic model was confirmed by varying the values of kinetic parameters of the TryR reaction (VmaxT[SH]2, KmT[S]2 and KmNADPH) (Table 3). Our results showed negligible variation in the concentration of T[SH]2 suggesting the robust nature of the model. A similar result in terms of robustness was obtained previously in the kinetic modeling study in Trypanosoma cruzi (Olin-Sandoval et al., 2012). To see the effect of TryR inhibition introduced in the basal kinetic model with radicals (M2), a sensitivity analysis of the pathways was performed. For this purpose, the TryR reaction parameter Vmax was selected to observe its influence on the reaction flux of all other reactions and a comparison was made among the three models (M2, M3, and M4). In all models, only ISCU (Iron sulfur cluster unit) activation was not affected by TryR reaction. It was observed that, the perturbed models with TryR reaction inhibition were highly sensitive to the reaction rate of TryR (Figure 6). Two downstream reactions, TDPx (neutralization of H2O2) and fenton reaction were found to be greatly sensitive to the rate of TryR reaction (Figures 6B,C). Although all reaction fluxes were found to be sensitive to T[SH]2 reduction rate including its own reaction flux, a 5 to 6-fold increase in sensitivity score was noted in all cases. Reactions related to Fe/S proteins activation, aconitase[4Fe-4S] and fumarase[4Fe-4S] via reduced Grx1/2 as well as their inactivation via free radicals were influenced at a greater rate suggesting direct influence of TryR on downstream reactions. These observations suggest that Fe/S proteins are directly connected to T[SH]2 level and a slight change in this would lead to the reduced level of activated Grx1/2, thereby, the activation of Fe/S proteins would be disturbed. Therefore, T[SH]2 emerged out as the most critical component of the redox metabolism and Fe/S cluster protein homeostasis in leishmania. The kinetic model built by our group is submitted to BioModel database (MODEL1811300001; Data Sheet 1) Chelliah et al. (2015).
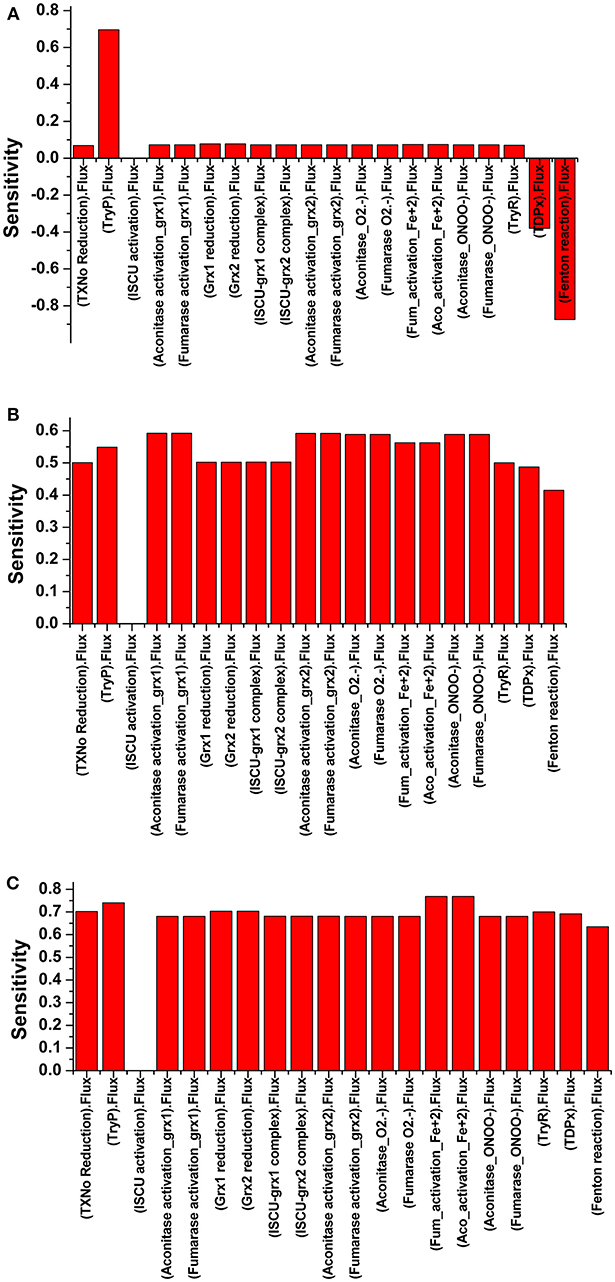
Figure 6. Illustration of Sensitivity analysis of (A) Model 2; (B) Model 3; and (C) Model 4. For sensitivity analyses in three models, VmaxTryR was used to see its effect on the flux of other reactions. Analyses shows that there was nil effect on ISCU activation (A), in contrast to the perturbed models with TryR reaction inhibition where all reactions except ISCU activation were found to be highly sensitive (B,C).
Conclusion
In a nutshell, our kinetic models have demonstrated the importance of T[SH]2 in leishmanial cellular redox metabolism. Introducing the perturbation at the TryR reaction, our analyses suggests that the inhibition of TryR enzyme might be an important check point for disturbing the parasite's survival inside the host macrophages. By designing novel potent inhibitors against the TryR enzyme, inhibition of T[SH]2 reduction and thereby, the perturbation of activation and regulation of ISC proteins can be achieved. However, to prove this hypothesis, present kinetic models needs to be refined in order to reproduce longer oxidative stress conditions. Moreover, proper inhibition reactions should be incorporated in order to check which inhibition conditions suit the best to perturb the whole downstream metabolites production. This will require determination of experimental parameters solely in case of Leishmania major.
Author Contributions
AK, NC, and SS contributed in the planning and experimentation of the study. AK and NC contributed in kinetic modeling. AK, NC, and SS participated in analyzing the results and writing of the manuscript.
Conflict of Interest Statement
The authors declare that the research was conducted in the absence of any commercial or financial relationships that could be construed as a potential conflict of interest.
Acknowledgments
AK acknowledges his UGC-JRF from University of Grants Commission, New Delhi. NC acknowledges her fellowship from DST-SERB NPDF (PDF/2016/001346) from Department of Science and Technology, Ministry of Science and Technology, Government of India. The authors would also like to thank the Director, National Centre for Cell Science (NCCS) for supporting the Bioinformatics and High Performance Computing Facility at NCCS, Pune, India.
Supplementary Material
The Supplementary Material for this article can be found online at: https://www.frontiersin.org/articles/10.3389/fcimb.2019.00015/full#supplementary-material
Abbreviations
Arg, Arginine; Met, Methionine; Glu, Glutamate; Cys, Cysteine; Orn, Ornithine; Put, Putrescine; SAM, S-adenosylmethionine; dcSAM, S-adenosylmethioninamine; γEC, γ-L-glutamyl-L-cysteine; Spd, Spermidine; Gly, Glycine; GSH, Glutathione; T[SH]2, Trypanothione; Gspd, Glutathionylspermidine; TS2, Trypanothione disulfide; TXNo/r, Tryparedoxin oxidized/reduced; Grx, Glutaredoxin; ISCU, Iron sulfur cluster unit; Aco, Aconitase; Fum, Fumarase; SAMSyn, S-adnosylmethionine Synthase; SAMDc, S-adnosylmethionine decarboxylase; γECS, γEC synthetase; GS, Glutathione synthetase; TryS, Trypanothione synthetase; TryR, Trypanothione reductase; TryP, Trypaoredoxin peroxidase; ODC, Ornithine decarboxylase; SpdS, Spermidine synthase; TDPx, Glutathione peroxidase-like tryparedoxine; NADP+, Nicotinamide adenine dinucleotide phosphate; ISC, Iron sulfur cluster; CIA, Cytosolic iron sulfur protein assembly; GR, Glutathione reductase.
References
Baiocco, P., Poce, G., Alfonso, S., Cocozza, M., Porretta, G. C., Colotti, G., et al. (2013). Inhibition of Leishmania infantum trypanothione reductase by azole-based compounds: a comparative analysis with its physiological substrate by X-ray crystallography. ChemMedChem 7, 1175–1183. doi: 10.1002/cmdc.201300176
Beig, M., Oellien, F., Garoff, L., Noack, S., Krauth-Siegel, R. L., and Selzer, P. M. (2015). Trypanothione reductase: a target protein for a combined in vitro and in silico screening approach. PLoS Negl. Trop. Dis. 9:e0003773. doi: 10.1371/journal.pntd.0003773
Beiting, D. P., and Roos, D. S. (2007). A systems biological view of intracellular pathogens. Immunol. Rev. 240, 117–128. doi: 10.1111/j.1600-065X.2010.00998.x
Berndt, C., Hudemann, C., Hanschmann, E. M., Axelsson, R., Holmgren, A., and Lillig, C. H. (2007). How does iron–sulfur cluster coordination regulate the activity of human glutaredoxin 2. Antioxid. Redox Signal. 9, 151–157. doi: 10.1089/ars.2007.9.151
Borst, P., and Ouellette, M. (1995). New mechanisms of drug resistance in parasitic protozoa. Annu. Rev. Microbiol. 49, 427–460. doi: 10.1146/annurev.mi.49.100195.002235
Castro, L., Rodriguez, M., and Radi, R. (1994). Marianela Rodriguez, and Rafael Radi. Aconitase is readily inactivated by peroxynitrite, but not by its precursor, nitric oxide. J. Biol. Chem. 269, 29409–29415.
Ceylan, S., Seidel, V., Ziebart, N., Berndt, C., Dirdjaja, N., and Krauth-Siegel, R. L. (2010). The dithiol glutaredoxins of African trypanosomes have distinct roles and are closely linked to the unique trypanothione metabolism. J. Biol. Chem. 285, 35224–35237. doi: 10.1074/jbc.M110.165860
Chelliah, V., Juty, N., Ajmera, I., Ali, R., Dumousseau, M., Glont, M., et al. (2015). BioModels: ten-year anniversary. Nucleic Acids Res. 43, D542–D548. doi: 10.1093/nar/gku1181
Clayton, C., and Shapira, M. (2007). Post-transcriptional regulation of gene expression in trypanosomes and leishmanias. Mol. Biochem. Parasitol. 156, 93–101. doi: 10.1016/j.molbiopara.2007.07.007
Comini, M. A., Rettig, J., Dirdjaja, N., Hanschmann, E. M., Berndt, C., and Krauth-Siegel, R. L. (2008). Monothiol glutaredoxin-1 is an essential iron-sulfur protein in the mitochondrion of African trypanosomes. J. Biol. Chem. 283, 27785–27798. doi: 10.1074/jbc.M802010200
Crack, J. C., Stapleton, M. R., Green, J., Thomson, A. J., and Le Brun, N. E. (2014). Influence of association state and DNA binding on the O2-reactivity of [4Fe-4S] fumarate and nitrate reduction (FNR) regulator. Biochem. J. 463, 83–92. doi: 10.1042/BJ20140169
Deponte, M., Becker, K., and Rahlfs, S. (2005). Plasmodium falciparum glutaredoxin-like proteins. Biol. Chem. 386, 33–40. doi: 10.1515/BC.2005.005
Dormeyer, M., Reckenfelderbäumer, N., Ludemann, H., and Krauth-Siegel, R. L. (2001). Trypanothione-dependent synthesis of deoxyribonucleotides by Trypanosoma brucei ribonucleotide reductase. J. Biol. Chem. 276, 10602–10606. doi: 10.1074/jbc.M010352200
Dumas, C., Ouellette, M., Tovar, J., Cunningham, M. L., Fairlamb, A. H., Tamar, S., et al. (1997). Disruption of the trypanothione reductase gene of Leishmania decreases its ability to survive oxidative stress in macrophages. EMBO J. 16, 2590–2598. doi: 10.1093/emboj/16.10.2590
Emptage, M. H., Dreyers, J. L., Kennedy, M. C., and Beinert, H. (1983). Optical and EPR characterization of different species of active and inactive aconitase. J. Biol. Chem. 258, 11106–11111.
Fairlamb, A. H. (1999). Future prospects for the chemotherapy of Chagas' disease. Medicina (B Aires). 59, 179–187.
Fairlamb, A. H., Blackburn, P., Ulrich, P., Chait, B. T., and Cerami, A. (1985). Trypanothione: a novel bis (glutathionyl) spermidine cofactor for glutathione reductase in trypanosomatids. Science 227, 1485–1487. doi: 10.1126/science.3883489
Fairlamb, A. H., and Cerami, A. (1985). Identification of a novel, thiol-containing co-factor essential for glutathione reductase enzyme activity in trypanosomatids. Mol. Biochem. Parasitol. 14, 187–198. doi: 10.1016/0166-6851(85)90037-4
Fairlamb, A. H., and Cerami, A. (1992). Metabolism and functions of trypanothione in the Kinetoplastida. Annu. Rev. Microbiol. 46, 695–729. doi: 10.1146/annurev.mi.46.100192.003403
Fairlamb, A. H., Henderson, G. B., and Cerami, A. (1989). Trypanothione is the primary target for arsenical drugs against African trypanosomes. Proc. Natl. Acad. Sci. U.S.A. 86, 2607–2611.
Fernandes, A. P., Fladvad, M., Berndt, C., Andrésen, C., Lillig, C. H., Neubauer, P., et al. (2005). A novel monothiol glutaredoxin (Grx4) from Escherichia coli can serve as a substrate for thioredoxin reductase. J. Biol. Chem. 280, 24544–24552. doi: 10.1074/jbc.M500678200
Filser, M., Comini, M. A., Molina-Navarro, M. M., Dirdjaja, N., Herrero, E., and Krauth-Siegel, R. L. (2008). Cloning, functional analysis, and mitochondrial localization of Trypanosoma brucei monothiol glutaredoxin-1. Biol. Chem. 389, 21–32. doi: 10.1515/BC.2007.147
Fladvad, M., Bellanda, M., Fernandes, A. P., Mammi, S., Vlamis-Gardikas, A., Holmgren, A., et al. (2005). Molecular mapping of functionalities in the solution structure of reduced Grx4, a monothiol glutaredoxin from Escherichia coli. J. Biol. Chem. 280, 24553–24561. doi: 10.1074/jbc.M500679200
Flohé, L. (2012). The trypanothione system and the opportunities it offers to create drugs for the neglected kinetoplast diseases. Biotechnol. Adv. 30, 294–301. doi: 10.1016/j.biotechadv.2011.05.012
Flohé, L., Budde, H., Bruns, K., Castro, H., Clos, J., Hofmann, B., et al. (2002). Tryparedoxin peroxidase of Leishmania donovani: molecular cloning, heterologous expression, specificity, and catalytic mechanism. Arch. Biochem. Biophys. 397, 324–335. doi: 10.1006/abbi.2001.2688
Flohe, L., Hecht, H. J., and Steinert, P. (1999). Glutathione and trypanothione in parasitic hydroperoxide metabolism. Free Radic. Biol. Med. 27, 966–984. doi: 10.1016/S0891-5849(99)00172-0
Go, Y. M., Roede, J. R., Orr, M., Liang, Y., and Jones, D. P. (2014). Integrated redox proteomics and metabolomics of mitochondria to identify mechanisms of cd toxicity. Toxicol. Sci. 139, 59–73. doi: 10.1093/toxsci/kfu018
Hofmann, B., Budde, H., Bruns, K., Guerrero, S. A., Kalisz, H. M., Menge, U., et al. (2001). Structures of tryparedoxins revealing interaction with trypanothione. Biol. Chem. 382, 459–471. doi: 10.1515/BC.2001.056
Holloway, G. A., Charman, W. N., Fairlamb, A. H., Brun, R., Kaiser, M., Kostewicz, E., et al. (2009). Trypanothione reductase high-throughput screening campaign identifies novel classes of inhibitors with antiparasitic activity. Antimicrob. Agents Chemother. 53, 2824–2833. doi: 10.1128/AAC.01568-08
Hoops, S., Sahle, S., Gauges, R., Lee, C., Pahle, J., Simus, N., et al. (2006). COPASI-a complex pathway simulator. Bioinformatics 22, 3067–3074. doi: 10.1093/bioinformatics/btl485
Hunter, W. N., Bailey, S., Habash, J., Harrop, S. J., Helliwell, J. R., Aboagye-Kwarteng, T., et al. (1992). Active site of trypanothione reductase: a target for rational drug design. J. Mol. Biol. 227, 322–333. doi: 10.1016/0022-2836(92)90701-K
Iwema, T., Picciocchi, A., Traore, D. A., Ferrer, J. L., Chauvat, F., and Jacquamet, L. (2009). Structural basis for delivery of the intact [Fe2S2] cluster by monothiol glutaredoxin. Biochemistry 48, 6041–6043. doi: 10.1021/bi900440m
Johansson, C., Roos, A. K., Montano, S. J., Sengupta, R., Filippakopoulos, P., Guo, K., et al. (2011). The crystal structure of human GLRX5: iron–sulfur cluster co-ordination, tetrameric assembly and monomer activity. Biochem. J. 433, 303–311. doi: 10.1042/BJ20101286
Jones, D. C., Ariza, A., Chow, W. H. A., Oza, S. L., and Fairlamb, A. H. (2010). Comparative structural, kinetic and inhibitor studies of Trypanosoma brucei trypanothione reductase with T. cruzi. Mol. Biochem. Parasitol. 169, 12–19. doi: 10.1016/j.molbiopara.2009.09.002
Krauth-Siegel, R. L., and Comini, M. A. (2008). Redox control in trypanosomatids, parasitic protozoa with trypanothione-based thiol metabolism. Biochim. Biophys. Acta 1780, 1236–1248. doi: 10.1016/j.bbagen.2008.03.006
Krauth-Siegel, R. L., Enders, B., Henderson, G. B., Fairlamb, A. H., and Schirmer, R. H. (1987). Trypanothione reductase from Trypanosoma cruzi: purification and characterization of the crystalline enzyme. Eur. J. Biochem. 164, 123–128. doi: 10.1111/j.1432-1033.1987.tb11002.x
Krauth-Siegel, R. L., and Inhoff, O. (2003). Parasite-specific trypanothione reductase as a drug target molecule. Parasitol. Res. 90, S77–S85. doi: 10.1007/s00436-002-0771-8
Krauth-Siegel, R. L., and Lüdemann, H. (1996). Reduction of dehydroascorbate by trypanothione. Mol. Biochem. Parasitol. 80, 203–208.
Krauth-Siegel, R. L., Meiering, S. K., and Schmidt, H. (2003). The parasite-specific trypanothione metabolism of Trypanosoma and Leishmania. Biol. Chem. 384, 539–549. doi: 10.1515/BC.2003.062
Krieger, S., Schwarz, W., Ariyanayagam, M. R., Fairlamb, A. H., Krauth-Siegel, R. L., and Clayton, C. (2000). Trypanosomes lacking trypanothione reductase are avirulent and show increased sensitivity to oxidative stress. Mol. Microbiol. 35, 542–552. doi: 10.1046/j.1365-2958.2000.01721.x
Kuriyan, J., Kong, X. P., Krishna, T. S. R., Sweet, R. M., Murgolo, N. J., Field, H., et al. (1991). X-ray structure of trypanothione reductase from Crithidia fasciculata at 2.4-Å resolution. Proc. Natl. Acad. Sci. U S A. 88, 8764–8768.
Li, H., Mapolelo, D. T., Dingra, N. N., Naik, S. G., Lees, N. S., Hoffman, B. M., et al. (2009). The yeast iron regulatory proteins Grx3/4 and Fra2 form heterodimeric complexes containing a [2Fe-2S] cluster with cysteinyl and histidyl ligation. Biochemistry 48, 9569–9581. doi: 10.1021/bi901182w
Lill, R. (2009). Function and biogenesis of iron–sulphur proteins. Nature 460, 831–838. doi: 10.1038/nature08301
Luo, M., Jiang, Y. L., Ma, X. X., Tang, Y. J., He, Y. X., Yu, J., et al. (2010). Structural and biochemical characterization of yeast monothiol glutaredoxin Grx6. J. Mol. Biol. 398, 614–622. doi: 10.1016/j.jmb.2010.03.029
Marsh, I. R., and Bradley, M. (1997). Substrate specificity of trypanothione reductase. Eur. J. Biochem. 243, 690–694. doi: 10.1111/j.1432-1033.1997.00690.x
Montemartini, M., Nogoceke, E., Gommel, D. U., Singh, M., Kalisz, H. M., Steinert, P., et al. (2000). Tryparedoxin and tryparedoxin peroxidase. Biofactors 11, 71–72. doi: 10.1002/biof.5520110120
Mühlenhoff, U., Molik, S., Godoy, J. R., Uzarska, M. A., Richter, N., Seubert, A., et al. (2010). Cytosolic monothiol glutaredoxins function in intracellular iron sensing and trafficking via their bound iron-sulfur cluster. Cell Metab. 12, 373–385. doi: 10.1016/j.cmet.2010.08.001
Mukhopadhyay, R., Dey, S., Xu, N., Gage, D., Lightbody, J., Ouellette, M., et al. (1996). Trypanothione overproduction and resistance to antimonials and arsenicals in Leishmania. Proc. Natl. Acad. Sci. U.S.A. 93, 10383–10387.
Nielsen, J., and Jewett, M. C. (2008). Impact of systems biology on metabolic engineering of Saccharomyces cerevisiae. FEMS Yeast Res. 8, 122–131. doi: 10.1111/j.1567-1364.2007.00302.x
Nogoceke, E., Gommel, D. U., Kieß, M., Kalisz, H. M., and Flohé, L. (1997). A unique cascade of oxidoreductases catalyses trypanothione-mediated peroxide metabolism in Crithidia fasciculate. Biol. Chem. 378, 827–836.
Olin-Sandoval, V., González-Chávez, Z., Berzunza-Cruz, M., Martínez, I., Jasso-Chávez, R., Becker, I., et al. (2012). Drug target validation of the trypanothione pathway enzymes through metabolic modelling. FEBS J. 279, 1811–1833. doi: 10.1111/j.1742-4658.2012.08557.x
Picciocchi, A., Saguez, C., Boussac, A., Cassier-Chauvat, C., and Chauvat, F. (2007). CGFS-type monothiol glutaredoxins from the cyanobacterium Synechocystis PCC6803 and other evolutionary distant model organisms possess a glutathione-ligated [2Fe-2S] cluster. Biochemistry 46, 15018–15026. doi: 10.1021/bi7013272
Pillay, C. S., Hofmeyr, J. H., Mashamaite, L. N., and Rohwer, J. M. (2013). From top-down to bottom-up: computational modeling approaches for cellular redoxin networks. Antioxid. Redox Signal. 18, 2075–2086. doi: 10.1089/ars.2012.4771
Qi, Y., and Grishin, N. V. (2005). Structural classification of thioredoxin-like fold proteins. Proteins 58, 376–388. doi: 10.1002/prot.20329
Rahlfs, S., Fischer, M., and Becker, K. (2001). Plasmodium falciparum possesses a classical glutaredoxin and a second, glutaredoxin-like protein with a PICOT homology domain. J. Biol. Chem. 276, 37133–37140. doi: 10.1074/jbc.M105524200
Robbins, A. H., and Stout, C. D. (1989a). Structure of activated aconitase: formation of the [4Fe-4S] cluster in the crystal. Proc. Natl. Acad. Sci. U S A. 86, 3639–3643. doi: 10.1073/pnas.86.10.3639
Saccoliti, F., Angiulli, G., Pupo, G., Pescatori, L., Madia, V. N., Messore, A., et al. (2017). Inhibition of Leishmania infantum trypanothione reductase by diaryl sulfide derivatives. J. Enzyme Inhib. Med. Chem. 32, 304–310. doi: 10.1080/14756366.2016.1250755
Shames, S. L., Fairlamb, A. H., Cerami, A., and Walsh, C. T. (1986). Purification and characterization of trypanothione reductase from Crithidia fasciculata, a new member of the family of disulfide-containing flavoprotein reductases. Biochemistry 25, 3519–3526. doi: 10.1021/bi00360a007
Stoll, V. S., Simpson, S. J., Krauth-Siegel, R. L., Walsh, C. T., and Pai, E. F. (1997). Glutathione reductase turned into trypanothione reductase: structural analysis of an engineered change in substrate specificity. Biochemistry 36, 6437–6447. doi: 10.1021/bi963074p
Tamarit, J., Bellí, G., Cabiscol, E., Herrero, E., and Ros, J. (2003). Biochemical characterization of yeast mitochondrial Grx5 monothiol glutaredoxin. J. Biol. Chem. 278, 25745–25751. doi: 10.1074/jbc.M303477200
Toledano, M. B., Kumar, C., Le Moan, N., Spector, D., and Tacnet, F. (2007). The system biology of thiol redox system in Escherichia coli and yeast: differential functions in oxidative stress, iron metabolism and DNA synthesis. FEBS Lett. 581, 3598–3607. doi: 10.1016/j.febslet.2007.07.002
Tovar, J., Cunningham, M. L., Smith, A. C., Croft, S. L., and Fairlamb, A. H. (1998a). Down-regulation of Leishmania donovani trypanothione reductase by heterologous expression of a trans-dominant mutant homologue: effect on parasite intracellular survival. Proc. Natl. Acad. Sci. U S A. 95, 5311–5316. doi: 10.1073/pnas.95.9.5311
Tovar, J., Wilkinson, S., Mottram, J. C., and Fairlamb, A. H. (1998b). Evidence that trypanothione reductase is an essential enzyme in Leishmania by targeted replacement of the tryA gene locus. Mol. Microbiol. 29, 653–660. doi: 10.1046/j.1365-2958.1998.00968.x
Turcano, L., Torrente, E., Missineo, A., Andreini, M., Gramiccia, M., Di Muccio, T., et al. (2018). Identification and binding mode of a novel Leishmania trypanothione reductase inhibitor from high throughput screening. PLoS Negl. Trop. Dis. 12:e0006969. doi: 10.1371/journal.pntd.0006969
Wyllie, S., Cunningham, M. L., and Fairlamb, A. H. (2004). Dual action of antimonial drugs on thiol redox metabolism in the human pathogen Leishmania donovani. J. Biol. Chem. 279, 39925–39932. doi: 10.1074/jbc.M405635200
Keywords: leishmaniasis, redox metabolism, trypanothione, Fe/S cluster proteins, glutaredoxin, systems biology, kinetic modeling
Citation: Kumar A, Chauhan N and Singh S (2019) Understanding the Cross-Talk of Redox Metabolism and Fe-S Cluster Biogenesis in Leishmania Through Systems Biology Approach. Front. Cell. Infect. Microbiol. 9:15. doi: 10.3389/fcimb.2019.00015
Received: 31 October 2018; Accepted: 17 January 2019;
Published: 04 February 2019.
Edited by:
Tunahan Cakir, Gebze Technical University, TurkeyReviewed by:
Pinar Pir, Babraham Institute (BBSRC), United KingdomMaria Viridiana Olin-Sandoval, Instituto Nacional de Ciencias Médicas y Nutrición Salvador Zubirán (INCMNSZ), Mexico
Copyright © 2019 Kumar, Chauhan and Singh. This is an open-access article distributed under the terms of the Creative Commons Attribution License (CC BY). The use, distribution or reproduction in other forums is permitted, provided the original author(s) and the copyright owner(s) are credited and that the original publication in this journal is cited, in accordance with accepted academic practice. No use, distribution or reproduction is permitted which does not comply with these terms.
*Correspondence: Shailza Singh, shailza_iitd@yahoo.com;singhs@nccs.res.in