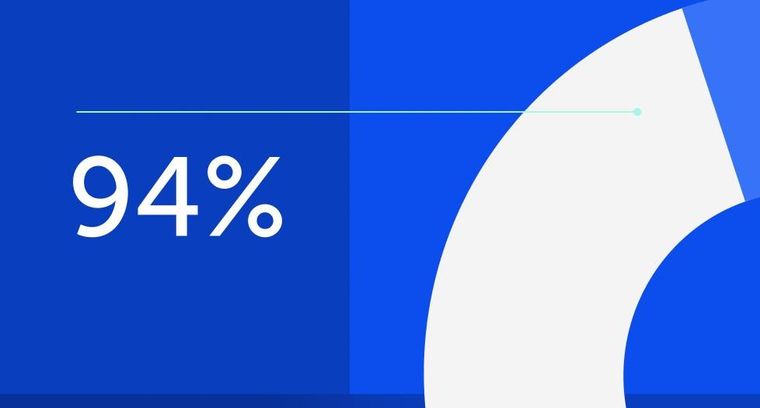
94% of researchers rate our articles as excellent or good
Learn more about the work of our research integrity team to safeguard the quality of each article we publish.
Find out more
REVIEW article
Front. Chem., 10 September 2020
Sec. Nanoscience
Volume 8 - 2020 | https://doi.org/10.3389/fchem.2020.00818
This article is part of the Research TopicNanozymes: From Rational Design to Biomedical ApplicationsView all 14 articles
Nano-sized iron sulfides have attracted intense research interest due to the variety of their types, structures, and physicochemical properties. In particular, nano-sized iron sulfides exhibit enzyme-like activity by mimicking natural enzymes that depend on an iron-sulfur cluster as cofactor, extending their potential for applications in biomedicine. The present review principally summarizes the synthesis, properties and applications in biomedical fields, demonstrating that nano-sized iron sulfides have considerable potential for improving human health and quality of life.
With the development of nanotechnology (Li et al., 2019), nanomaterials have become a major resource for the development of novel therapeutic medicines and technologies designed to improve human health and the quality of life (Zhang and Webster, 2009; Esmaeili et al., 2020; Wang et al., 2020). In particular, due to their multiple functionality and excellent biocompatibility, iron-based nanomaterials are frequently used in the biomedical field, such as bioseparation, biosensors, magnetic resonance imaging (MRI), tumor hyperthermia, and drug delivery (Chen and Gu, 2017). In addition, recent studies have revealed that these nanomaterials have intrinsic enzyme-like properties (Gao et al., 2007; Xie et al., 2012; Xu et al., 2018), an important form of nanozyme representing a new generation of artificial enzyme (Wei and Wang, 2013; Dong et al., 2019; Liang and Yan, 2019). Currently, the majority of iron-based nanomaterials are iron oxide which possess excellent supraparamagnetic properties, with catalytic activity mimicking that of oxido-reductases, including peroxidase, catalase, superoxide dismutase, and oxidase (Gao et al., 2007; Liang and Yan, 2019). However, iron sulfide nanomaterials have not been comprehensively studied or used in the biomedical fields. Since O and S are congeneric elements, iron sulfide demonstrates similar physiochemical properties as iron oxide (Fu et al., 2019). In addition, the phases of iron sulfide in nature include mackinawite (FeS), pyrrhotite (Fe1−xS), pyrite (FeS2), and greigite (Fe3S4), etc., which exhibit more variability than iron oxide containing only Fe2O3 and Fe3O4. The band gap in iron sulfide is smaller than that of iron oxide, leading to the former having more appropriate electron transfer and conductivity (Wadia et al., 2009; Jin et al., 2017; Zhang et al., 2018). Importantly, iron-sulfur clusters are important cofactors in many enzymes which serve as active centers for electron transfer in catalytic processes and respiratory chain reactions (Qi and Cowan, 2011). Therefore, it is anticipated that iron sulfide nanomaterials will display multiple functionalities and they have great potential in biomedical applications. Herein, we will summarize the types, synthesis and properties of iron sulfide nanomaterials and emphasize their applications in biomedical and medical fields. This will provide a comprehensive understanding of iron sulfide nanomaterials and illustrate their considerable potential as novel multifunctional biomaterials in biomedical applications.
Solid phases of iron sulfides principally comprise FeS (mackinawite), Fe1−xS (pyrrhotite), FeS2p (pyrite), FeS2m (marcasite), Fe3S4 (greigite), and Fe9S11 (smythite). The content of iron within a biomaterial therefore influences its phase, shape, and physical and chemical properties. FeS naturally has a tetragonal structure, with each iron atom coordinated to four sulfurs. For Fe1−xS, a monoclinic hexagonal is present. FeS2p forms stable iron (II) disulfides with cubic structures. FeS2m differs from FeS2p as an orthorhombic metastable iron (II) disulfide, whilst Fe3S4 is a cubic metastable Fe (II) Fe (III) sulfide. Hexagonal Fe9S11 is related to the Fe1−xS phase (Rickard and Luther, 2007).
Reported crystal structures of iron sulfide are displayed in Figure 1 (Fleet, 1971; Argueta-Figueroa et al., 2017). FeS possesses a tetragonal layered structure in which the iron atoms are linked through tetrahedral coordination to four equidistant sulfur atoms. A single iron atom is coordinated to four equidistant sulfur atoms. The distance of Fe-Fe is 2.5967 Å. In addition, Fe-Fe bonding is substantial in FeS. To assess the effects of van der Waals forces resulting from the S atoms, sheets including Fe are stacked along the C-axis. The spacing of these layers is 5 Å. The structure of Fe2S2 is closed to FeS. The structure of FeS2 is similar to that of NaCl in which S2− is located at the center of a cube. The cubic structure has a low symmetry. In addition, FeS2 exhibits chirality through absorbed organic molecules. Fe3S4 has an inverse spinel structure in which 8 Fe atoms are located at the tetrahedral A-sites and 16 Fe atoms are located at the B-sites of the octahedron. The unit cell of Fe3S4 is 9.876 Å. In addition, the cubic structure of Fe3S4 forms a closely packed array of S molecules linked by smaller Fe units (Figure 1D). It has been established that the Fe7S8 structure is a hexagonal supercell (Fleet, 1971). The viable distribution of vacancy sites ideal for the base structure of NiAs was observed to describe the structure of Fe9S10 (Elliot, 2010).
Figure 1. Crystal structure of iron sulfide. (A) FeS. (B) FeS2. (C) Fe2S2. (D) Fe3S4. Reproduced with permission from Rickard and Luther (2007). Copyright 2007, American Chemical Society.
Nano-sized iron sulfide encompasses a range of iron and sulfur compounds. Firstly, the range of chemical and biological methods for their production are discussed. In addition, the most reported synthesized methods for creating different phases of iron sulfide are presented in Table 1.
Thermal decomposition is the most commonly-used hydrothermal reaction for iron sulfide production. The typical solvothermal synthesis method of nFeS firstly involves the dissolution of FeCl3·6H2O in 40 mL of ethylene glycol. NaOAc and organosulfur compounds (allyl methyl sulfide, diallyl sulfide, diallyl trisulfide, diallyl disulfide, cysteine, cystine, glutathione (GSH), or methionine) are then added under continuous and vigorous stirring. The system was then sonicated for 10 min and transferred to a Teflon-lined stainless steel autoclave. The mixture was reacted at 200°C for 12 h and precipitates washed three times with ethanol and water. Finally, the products were dried at 60°C for 3 h (Xu et al., 2018). For FeS2, the single source molecular precursor Fe3+ diethyl dithiophosphate forms an aqueous solution through the reaction of FeCl3 and (C2H5O)2P(S)SNH4, with hexadecyltrimethylammonium bromide (CTAB) added as a surfactant and reacted with a single precursor [(C2H5O)2P(S)S]3Fe (Wadia et al., 2009). For FeS, FeCl3·6H2O was dissolved in ultrapure water with ethanolamine and thiourea added to the solution. After stirring for 25 min, the mixture was added to a Teflon lined autoclave and reacted at 180°C for 12 h. The synthesis of Fe3S4 differs from that of FeS. FeCl3·6H2O, ethylene glycol, thiourea and H2O2 were mixed and reacted at 180°C for 18 h in the presence of the capping agent polyvinyl pyrrolidone (PVP) to prevent excessive growth and aggregation of the nanoparticles (NPs) (Moore et al., 2019). Fe1−xS single crystals were then synthesized through a hydrothermal method by the addition of K0.8Fe1.6S2 crystals, Fe powder, NaOH, and thiourea in deionized water, which was reacted at 120°C for 3–4 days (Guo et al., 2017). Ionic liquids that form extended hydrogen bond systems were then used to form higher structures in the base of the hydrothermal process, as reported by Zheng and colleagues when changing the structure of Fe3S4 (Ma et al., 2010). Generally speaking, the product obtained by the hydrothermal method has better dispersibility and controllability, but iron oxide impurities can also appear during the synthesis of iron sulfide. Meanwhile, multiphase iron sulfide appears to occur easily, as assessed by X-ray diffraction (XRD) patterns for hydrothermally-synthesized samples.
The principal advantages of microwave-assisted methods, compared with conventional heating include its reduced reaction time, smaller particle size distribution, and higher purity. Ethylene glycol is a solvent suitable for microwave-assisted methods owing to its relatively high dipole moment. For FeS2 microspherolites, FeSO4·7H2O, PVP-K30 and S powder in ethylene glycol can be reacted by microwave in an N2 atmosphere (Li M. et al., 2011). Although this emerging technique may be more desirable it should be noted that the aggregation phenomenon does not appear to be improved.
Chemical co-precipitation does not introduce impurities. The operation is performed under mild conditions and is typically synthesized using Fe3S4 methods, in which iron (II) sulfate heptahydrate and sodium sulfide are dissolved in ultrapure deionized water. The solution was then added dropwise to acetic acid to adjust the pH to 3.0, followed by stirring for several minutes. The reaction was prepared under an N2 atmosphere (Chang et al., 2011). In addition, green synthesis was achieved in a continuous stirred-tank reactor (Simeonidis et al., 2016). As previously reported, the conditions of synthesis required by co-precipitation are harsher than those of other methods, and the products obtained may show poor homogeneity.
Chemical synthesis methods using high temperatures have been reported for FeS2. Briefly, iron (II) acetylacetonate (Fe(acac)2), trioctylphosphine oxide (TOPO) and oleyamine (OLA) were mixed and degassed at 110°C for 1 h under a vacuum. The mixture was then rapidly heated to 220°C for 1 h under vigorous magnetic stirring in the presence of nitrogen. Sulfur was then quickly injected into the solution, which was heated to 220°C for 1 h. Once the solution cooled, ethanol was added to the precipitate to develop the FeS2 nanoplates (Bi et al., 2011). Synthesis methods for Fe1−xS and Fe3S4 have also been reported. The rapid injection method has been used to reduce the size of Fe3S4 (Beal et al., 2012). This method of synthesis is highly sensitive to the experimental conditions.
As a convenient and stable synthetic method, Bala and colleagues described specific FeS' sonochemical synthesis. Firstly, sodium sulfide was dissolved in double distilled water. FeSO4·7H2O was then independently dissolved in a solution of double distilled water and polyethylene glycol (1:1). The sodium sulfide solution contained a drop of Triton-X surfactant which was added dropwise to the above solution while being continuously sonicated for 30 min. PVP was then added and the system was mixed via ultrasound for a further 30 min (Ahuja et al., 2019).
Some unusual synthetic chemical methods have been reported. The low temperature synthesis of FeS2 nanoparticles was described in 2014 (Srivastava et al., 2014a). Briefly, FeCl3 and sodium polysulfide (Na2Sx) were mixed in pH 5.6 acetate buffer in an anaerobic environment. The black solution then was reacted in a 90–100°C oil bath for 4 h to produce a grayish FeS2 product. Flux methods were then used to synthesize 1D Fe7S8. The reaction was conducted within a furnace at 750–850°C (Kong et al., 2005). FeS can also be created in a biological system (Mei and Ma, 2013).
The bio-synthesis of iron sulfide using microorganisms is superior for biomedical applications (Li X. et al., 2011). When microorganisms interact with target ions, they are transported into microbial cells to form NPs in the presence of specific enzymes. In addition to the advantages of green synthesis, biological methods improve the biocompatibility of iron sulfide. The particles generated have higher catalytic reactivity and a greater surface area. Previous studies have reported that FeS2, Fe3S4, and FeS NPs can be produced by microorganisms. For the biomineralization of Fe3S4 and FeS2, a magnetotactic bacterium has been described (Mann et al., 1990). In 1995, FeS materials were produced by sulfate-reducing bacteria grown on iron containing substrates (Watson et al., 1995). Bazylinski and colleagues also reported the formation of Fe3S4 using non-cultured magnetotactic bacteria (Lefèvre et al., 2010). Sulfate-reducing bacteria were able to produce Fe1−xS as reported by Charnock and colleagues (Watson et al., 2000). NPs were subsequently formed at the surface by the microorganisms and as such, the porous structure of the iron sulfide NPs failed to prevent normal metabolism. These studies verified the utility of this method for efficient NP production. Chemical biomineralization methods have also been used to synthesize FeS2 and FeS Quantum dots (QDs) (Jin et al., 2018; Yang et al., 2020).
Bare nanocrystal cores have an unstable structure that is prone to photochemical degradation. However, those that are unmodified display higher toxicity. As such, biocompatible moieties are essential as they serve as caps for nanomaterials, including polyethylene glycol (PEG), silica, lactose, citrate, and dextran (Simeonidis et al., 2016; Mofokeng et al., 2017). Previous studies have reported the synthesis, characterization, cytotoxicity and biodistribution of FeS/PEG nanoplates in vivo (Yang et al., 2015). An adsorption-reduction method was used to load silver onto the surface of 3-aminopropyltriethoxysilanemodified 3-aminopropyl triethoxysilane (APTES)-modified Fe3S4 particles, achieving the preparation of magnetic composite nanoparticles of Fe3S4/Ag (He et al., 2013). However, modifications also led to adverse effects. For example, the modification of CTAB inhibited the growth of nano Fe3S4 (Simeonidis et al., 2016).
Detailed characterization is required to confirm the synthesis of iron sulfide. Scanning electron microscopy (SEM) and transmission electron microscopy (TEM) can be used to image surface morphology. These represent the most direct and commonly-used methods to assess the microstructure, size, and dispersion of the materials. Differences in the synthetic methods have presented variations in TEM/SEM imaging, including within the same phase. For FeS, nanoplates, spherical nanoparticles, and pomegranate flower-like shapes have been reported. FeS2 exists as large particles, cubic nanocrystals, monodisperse microspherolites, spherical and hexagonal nanoribbons, and nanorods. Typical images of iron sulfide are shown in Figure 2. Table 1 reports the most common morphology and size of FeS, FeS2, Fe3S4, and Fe7S8. Lattice spaces are measured to judge the crystallinity of the materials. The reported lattice spaces of the iron sulfides are summarized in Table 1. The composition, content, and structure of a substance can be analyzed, measured, and inferred using Ultraviolet-visible (UV-Vis) spectrometry and the absorption of UV and visible light. According to previous studies, the UV absorption peaks of FeS are 285 nm and 500 nm (Table 2). Information on the composition of the materials and the structure and/or morphology of the atoms or molecules inside the materials can be obtained by XRD analysis. XRD is the most direct indicator of whether a crystal is pure and so represents a convenient system to analyze synthesized materials. JCPDS cards are used to contrast and analyze unknown crystals. JCPDS cards of FeS (JCPDS card No. 15-0037 and 75-0602), FeS2 (JCPDS card No. 03-065-1211, 89-3057, 65-3321, and 42-130), Fe3S4 (JCPDS card No. 16-0713, 89-1998, and 16-0073) and Fe7S8 (JCPDS card No. 25-0411 and 76-2308) are listed in Table 2. For iron sulfide nanomaterials, the X-ray photoelectron spectroscopy (XPS) of Fe and S are essential. FeS materials are presented in Table 2 (Fe 2p: 2p3/2 (711.4 eV), 2p1/2 (724.9 eV), S 2p: 2p3/2 (161.1 eV), 2p1/2 (166.0 eV)), FeS2 (Fe 2p: 2p3/2 (707.0 eV), 2p1/2 (720.0 eV), S 2p 2p3/2 (162.3 eV), 2p1/2 (163.5 eV)), Fe3S4 (Fe 2p: 2p3/2 (711.0 eV), S 2p: 2p3/2 (161.0 eV), 2p1/2 (162.5 eV)), Fe7S8 (Fe 2p: 2p3/2 (709.9 eV), (711.6 eV), S 2p: 2p3/2 (163.5 eV), 2p1/2 (164.7 eV)). Fourier transform infrared (FTIR) spectroscopy can be used to detect functional groups in a complex mixture and so is therefore essential to the characterization of modified iron sulfide. Previous studies have provided FTIR spectra, showing successful modifications by APTES on the surface of the Fe3S4 nanoparticles (He et al., 2013). Fe7S8/N-C nanohybrids were prepared for FeMOF and FeMOF-S and analyzed by FTIR (Jin et al., 2019). In addition, energy dispersive spectroscopy (EDS) (Paolella et al., 2011; Ding et al., 2016; Guo et al., 2016), dynamic light scattering (DLS) (He et al., 2013; Ding et al., 2016), Raman spectroscopy (Gan et al., 2016; Guo et al., 2016), selected area electron diffraction (SAED) (Kar and Chaudhuri, 2004), X-ray absorption fine structure (XAFS) (Feng et al., 2013), differential scanning calorimetry (DSC) and thermogravimetric analysis (TGA) (Jin et al., 2019), nitrogen adsorption–desorption isotherms and pore size distribution (Guo et al., 2016) analysis of iron sulfide have been reported as characterization systems.
Figure 2. Representative images of nano-sized iron sulfide. (A) TEM image of the spherical FeS. Reproduced with permission from Dai et al. (2009). Copyright 2009, Wiley-VCH Verlag GmbH & Co. KGaA, Weinheim. (B) TEM and HRTEM of FeS2 nanodots. Reproduced with permission from Jin et al. (2018). Copyright 2017, American Chemical Society. (C) TEM images of platelet-like Fe3S4. Reproduced with permission from Paolella et al. (2011). Copyright 2011, American Chemical Society. (D) SEM and HRTEM of Fe7S8 nanowires. Reproduced with permission from Yao et al. (2013). Copyright 2013, Wiley-VCH Verlag GmbH & Co. KGaA, Weinheim.
In addition to both the physical and chemical properties, characteristics include the structure, solubility, stability, reactivity, magnetic properties, and photothermal properties of the products.
The major forms of nano-sized iron sulfides are solid precipitates which have poor solubility in water. However, Rickard et al. revealed that sedimental FeS can dissolve at c (S2−) ≤ 10−5.7 M to form Fe2+. The solubility can be increased in an alkaline as opposed to neutral environment (Rickard, 2006). FeS does not dissolve in HCl, meaning it cannot be removed with HCl (Rickard and Luther, 2007). According to previous studies, the Ksp of FeS2 was 10−16.4 at 25°C. The solubility products of various iron sulfides were assessed and resulted in consensus values for the pKs (FeS: 3.6 ± 0.2; FeS2 16.4 ± 1.2; Fe3S4 4.4 ± 0.1; Fe7S8 5.1 ± 0.1). This improved our understanding of the solubility of iron sulfide in both synthetic and natural water at room temperature (Davison, 1991).
FeS is stable within the P-T range of the Martian core (Kavner et al., 2001). Understanding the relationship between the stability of iron sulfide and its chemical environment is of key importance. Once iron sulfide is formed, its structure is reversible. Studies have examined the stability of FeS2 in different temperatures and the concentrations of absorbed water on the surface. Temperature has little effect on the morphology of FeS2 under low absorbed water concentrations. However, at above 90 K, the conversion from an octahedral structure to a cubic shape is promoted. At higher concentrations of water, the dependence on temperature is more apparent (Barnard and Russo, 2009). The latter study established that functions of the surface ligands affect the stability of FeS2 (FeS2 nanorods synthesized in laboratory) (Barand and Russo, 2009). The stability of FeS contributed to low energy excitation from Fe d to S-Sσ*p (Zhang et al., 2018). Fe3S4 was observed at 200°C for 30 h then it transformed to FeS2 over time (Gao et al., 2015).
Iron sulfide is highly reactive to N2 and H2S. This reaction occurs at room temperature and the adsorption of N2 is dependent on the surface FeS and on the electronic state of N2. A decrease in absorbed N2 and H2S could be explained by the formation of ammonia (Kasting, 1993). The reactivity of Fe7S8 is similar to FeS (Niño et al., 2018). The presence of both Au1+ and Au atoms has been observed on the surface of FeS2. Au deposition increased at higher pH and temperatures. The reactivity of Au1+ sulfides with FeS2 have also been investigated (Scaini et al., 1998). The reactivity of FeS2 using gaseous H2O and O2− was similarly reported. Gaseous H2O leads to the formation of iron hydroxides on FeS2. A sequence of different exposures also leads to the formation of a range of products (, Fe(OH)3) (Usher et al., 2005). Recently, FeS2 was shown to be a potential nanomaterial for prebiotic chemistry due to its highly reactive surface that drives amino acid adsorption (Ganbaatar et al., 2016). Among the most common probes, water molecules have been used to explore the reactivity of FeS2 (De Leeuw et al., 2000). The different phases of iron sulfide display a wide range of reactivities to chlorinated solvents. Conditions including pH, sulfide concentrations, metal ions, and natural organic matter can affect the reaction kinetics of the degradation of chlorinated solvents (He et al., 2015). The interaction of FeS, Fe3S4 and CO2 have also been reported. The charge transfer on FeS can also effectively activate CO2, whilst Fe3S4 is unreactive to CO2 (SantosCarballal et al., 2017).
Nanomaterials with magnetic properties have numerous applications, including magnetocaloric therapies, as MRI agents, magnetic separation materials, and magnetic carriers. The discovery of their magnetic properties led to the identification of iron sulfide phases. The ferromagnetism of Fe7S8 can be explained by Fe3+ ions with excess sulfur (Yosida, 1951). The magnetic susceptibility χ of natural FeS2 was found to be 64 × 10−668 × 10−6 cm3 /moles between 4.2 and 380 K (Mohindar and Jagadeesh, 1979). Magnetic ordering in FeS was inferred and used to prove strong itinerant spin fluctuations. FeS can also be used as a superconductor (Kwon et al., 2011). Even when the structure of FeS is changed from troilite to the MnP-type under high pressure, the antiferromagnetic properties are preserved until the monoclinic structure is formed (Ono, 2007). The magnetic moment then disappears and yetragonal-phase FeS (Tc: 5K) was observed for the same structure as the superconductor FeSe (Tc: 8 K) (Kuhn et al., 2016). Fe3S4 displays high Mrs/χ, (Mrs/χ: the saturation isothermal remnant magnetization: magnetic susceptibility) and its MrsMs (hysteresis ratios) and Bcr/Bc are 0.5 and 1.5 (Ms: saturation magnetization; Bc: the coercive force; Bcr: the coercivity of remanence). Fe3S4 also displays unique high-temperature properties, with a clear drop in magnetization from 270 to 350°C (Roberts, 1995). Synthesized Fe3S4 contains various crystals from small superparamagnetic grains (non-remanence) to large multi-domain grains (Snowball, 1991). The magnetic hysteresis properties of Fe7S8 have also been studied (Menyeh and O'Reilly, 1997). The relationship between structure and magnetic properties has been reported within variable temperatures. Magnetic transitions occurred within the transformation of the structure (Powell et al., 2004). The magnetocaloric conversion ability of Fe3S4 nanoparticles has been measured under an alternating magnetic field (AMF). Meanwhile, the excellent physical and chemical properties provide magnetothermal thrombolytic ability in medical applications (Fu et al., 2019; Figure 3).
Figure 3. Magnetocaloric conversion performance (a–d) and photothermal property of iron sulfides. (a) Temperature change of the Fe3S4 nanoparticles in water at varied concentrations of Fe2+ (i.e., 0, 0.5, and 1.0 mg/mL) as a function of magnetic field action time. (b) Plot of temperature change over 300s vs. the concentration of Fe3S4 nanoparticles. Thermal imaging of (c) pure water and (d) Fe3S4 nanoparticles (1.0 mg/mL) under the action of an AMF for 5 min. Reproduced with permission from Fu et al. (2019). Copyright 2019, Frontiers in Materials. (e) Synthetic route and applications of FeS2 nanodots in the base of its photothermal activity. (f) Scheme showing photothermal enhanced cellular uptake of FeS2@BSA-Ce6 nanoparticles. Reproduced with permission from Jin et al. (2018). Copyright 2017, American Chemical Society.
Photothermal therapy (PTT) has attracted considerable attention in recent years. The mechanism of PTT results mainly from photo-absorbing nanomaterials that generate heat through continuous laser irradiation, destroying cancer cells, but causing no damage to healthy tissue. It is therefore necessary to pay attention to the photothermal properties of iron sulfide. PEGylated FeS (FeS-PEG) nanoplates exhibit high near infrared (NIR) absorbance. Using Infrared (IR) thermal imaging, the temperature can reach 70°C within 5 min. Meanwhile, FeS-PEG displays stronger photothermal conversion efficiency than other known iron oxides (Yang et al., 2015). Ultrasmall FeS2 nanodots have also been synthesized and have been shown to be useful for photodynamic therapy. Chlorin e6 (Ce6) was used to conjugate FeS2 in the presence of the template bovine serum albumin (BSA). FeS2@BSA-Ce6 nanodots (7 nm) demonstrated good results in in vivo photoacoustic (PA) imaging, MRI and enhanced cellular uptake (Jin et al., 2018; Figure 3).
To-date, a variety of biomedical applications of iron sulfide (catalysts, antibacterial agents, cancer therapies, drug delivery systems, thrombolytic agents, biosensors, antifungal agents, seed improvers in phyto-applications) and their functional mechanisms have been summarized (Scheme 1).
Iron sulfur clusters are critical cofactors in many enzymes and proteins which conduct redox reactions and regulate oxidative stress. Thus, nano-sized iron sulfides are expected to perform similar catalysis and act as nanozymes. Previous studies have shown that iron sulfide can effectively activate persulfate (PS) or peroxymonosulfate (PMS) to generate sulfate radicals (Xiao et al., 2020). Other common reactions involving iron sulfide are shown in section Sonochemical Synthesis. Since iron oxide nanoparticles were shown to possess intrinsic peroxidase activity in 2007 (Gao et al., 2007), it has been speculated that iron sulfide has similar properties. The catalytic processes of iron sulfide are shown in Figure 4. High catalytic activity, multi-enzymes activities, harsh environmental resistance, storage stability, and the intrinsic advantages of nanomaterials provide further possibilities for biomedicine development, meaning that good alternatives to natural enzymes exist. The enzymatic activity of iron sulfide has been intensely investigated. In 2010, FeS nanosheets were reported to possess peroxidase-like activity. FeS suspensions were shown to catalyze the oxidation of peroxidase substrates, 3, 3′, 5, 5′-Tetramethylbenzidine (TMB) to produce a blue product in the presence of H2O2 (Dai et al., 2009) (Figure 4). Fe7S8 nanowires' also possessing intrinsic peroxidase activity was also reported in 2013 for which catalytic behavior was observed. The apparent KM of Fe7S8 with TMB as a substrate was 0.548 mM, almost six times lower than that of horseradish peroxidase (HRP). These results demonstrate that Fe7S8 has a higher affinity to TMB than to HRP (Yao et al., 2013). In 2015, magnetic Fe3S4 NPs was shown to possess peroxidase-like activity. Through investigating steady state kinetics, Fe3S4 was shown to possess a higher affinity for H2O2 than HRP. The reasons could be that Fe3S4 binds to and reacts with H2O2, following which nanozyme is released prior to reacting with the second substrate TMB (Ding et al., 2016). nFeS (Fe1−xS and Fe3S4) (detailed description in section Hydrothermal Synthesis) have been shown to possess both peroxidase-like and catalase-like activity (Figure 4). nFeS is able to decompose H2O2 into free radicals and O2, promoting the release of polysulfanes (Xu et al., 2018). FeS2 has also been shown to possess amylase-like properties (Srivastava et al., 2014b).
Figure 4. Schematic diagram of catalysis of iron sulfide as a nanozyme. (A) The peroxidase-like activity of FeS. Images of the suspension of sheet-like FeS nanostructure (1), mixture of TMB and H2O2 after catalytic reaction by sheet-like FeS nanostructure (2), mixture of TMB and H2O2 after adding H2SO4 to quench the catalytic reaction by sheet-like FeS nanostructure (3). Reproduced with permission from Dai et al. (2009). Copyright 2009, Wiley-VCH Verlag GmbH & Co. KGaA, Weinheim. (B) The catalase-like activity of Cys-nFeS. The trend of KM and the ratio of Vmax/KM in the kinetics assay with varied cysteine. Reproduced with permission from Xu et al. (2018). Copyright 2018, Nature Publishing Group.
Schoonen et al. reported on the antibacterial action of FeS2. Its mechanism was shown to be related to the formation of H2O2. The same group also reported how minerals can induce the formation of reactive oxygen species (Cohn et al., 2006; Schoonen et al., 2006). The rapid absorption of Fe2+ can influence bacterial metabolism. The oxidization of Fe2+ to Fe3+ leads to reactive oxygen species (ROS) production and biomolecular damage. As a result, iron sulfide can act as antimicrobial agents. Iron sulfides have been reported to be effective in bacterial infections. In 2013, He et al. reported on the bacteriostatic activity of Fe3S4/Ag against E. coli (86.2%) and S. aureus (90.6%). Meanwhile, Fe3S4 NPs without Ag have no effect (He et al., 2013). The Arenas-Arrocena group synthesized Fex−1S NPs and reported their antibacterial and cytotoxic activity in 2018. Antibacterial activity against S. aureus, E. coli and E. faecalis was observed (Argueta-Figueroa et al., 2018). In addition, Gao and coworkers discovered antibacterial inorganic iron polysulfides materials that were converted by organosulfur compounds in 2018. Inorganic nano-sulfides (nFeS, Fe1−xS, and Fe3S4) have shown inhibition against Pseudomonas aeruginosa and Staphylococcus aureus. These studies also described new strategies to synthesize iron sulfide nanomaterials. Furthermore, the S. mutans biofilm-related infections could be prevented by nFeS (Xu et al., 2018) (Figure 5). The antibacterial properties of FeS2-Bi2O3 against the pathogenic microorganisms Mycobacterium tuberculosis and Salmonella have also been measured (Manafi et al., 2019). Diksha et al. reported that FeS NPs could enhance intrabacterial ROS levels in bacteria by light irradiation in 2020. This was revealed as the primary antibacterial mechanism of FeS NPs (Agnihotri et al., 2020).
Figure 5. (A) Morphology of P. aeruginosa before (control) and after Cys-nFeS treatment. The red triangles indicate flagella. Scale bars: 1 μm. (B) Photographs of P. aeruginosa infected wounds treated with buffer (control), Cys-nFeS, H2O2, and Cys-nFeS + H2O2 at different times (five mice in each group). (C) Confocal 3D image of a S. mutans UA159 biofilm treated by Cys-nFeS. Scale bars: 100 μm. (D) SEM image of a S. mutans biofilm treated by Cys-nFeS. The red arrows indicate EPS. Left scale bars: 100 μm. Right scale bars: 3 μm. (E) Scheme of polysulfane release from nFeS. Reproduced with permission from Xu et al. (2018). Copyright 2018, Nature Publishing Group.
Chen and colleagues reported that tumors in mice could disturb iron metabolism in the major organs. The chemical form of iron in the tumors was ferrous-sulfide-like iron and ferritin, highlighting the potential of iron sulfide for cancer treatment (Chen and Chen, 2017). Chang et al. synthesized Fe3S4 particles with magnetic properties through co-precipitation. The NPs were used for cancer hyperthermia providing a new avenue for multimodal anticancer therapies (Chang et al., 2011). In 2015, Yang et al. concluded that triangle nanoplates (FeS/PEG nanostructures) could be used as nanoagents for in vivo MRI-guided photothermal cancer treatment. High doses of FeS nanoplates were shown to be safe and effective in mice. This study highlighted the potential clinical use of FeS, for MRI in addition to PTT (Figure 6) (Yang et al., 2015). In 2018, Fe3S4 nanosheets were shown to possess high efficiencies for MRI guided photothermal and chemodynamic synergistic therapy, opening up a new direction for the design of inorganic iron sulfide for future clinical applications (Guan et al., 2018). Tiny nano-sized iron sulfide with simple biomineralization method has also been attention owing to its huge potential in vivo application especially in cancer therapy combined with its excellent photothermal and magnetic performance. FeS2@BSA-Ce6 (detailed in section Biomineralization) exhibited good results whether in vivo multimodal imaging or in vivo combined therapy (Jin et al., 2018). Meanwhile, the latest literature proved 3 nm FeS@BSA QDs could be used as T1-weighted MRI contrast agents. Moreover, the ultrasmall QDs showed good results in photothermal therapy and they could be cleared via glomerular filtration into bladder after treatment (Yang et al., 2020). The use of iron chalcogenides has also been investigated. Cu5FeS4-PEG NPs were effective in dual-modal imaging and PTT (Zhao et al., 2016). In 2017, CuFeS2 nanoplates were used for in vivo photothermal/photoacoustic imaging and cancer chemotherapy/PTT (Ding et al., 2017).
Figure 6. (A) In vivo fluorescence images of 4T1 tumor-bearing nude mice taken at different time points post iv injection of FeS2@BSA-Ce6 nanodots (3.5 mg/kg Ce6 and 12 mg/kg FeS2). (B) Photoacoustic images of tumors in mice taken at different time points post iv injection of FeS2@BSA-Ce6 nanodots. (C) MR images of 4T1 tumor-bearing nude mice before and 8 h after iv injection of FeS2@BSA-Ce6 nanodots. (3.5 mg/kg Ce6 and 12 mg/kg FeS2). (D) IR thermal images of tumors in mice iv injected with FeS2@BSA-Ce6 under 808 nm laser irradiation (0.8W/cm2, 15 min). (E) Surface temperature changes of tumors monitored by the IR thermal camera during laser irradiation. (F) Representative immunofluorescence images of tumor slices after hypoxia staining. The cell nucleus, hypoxia areas, and blood vessels were stained with DAPI (blue), antipimonidazole antibody (green), and anti-CD31 antibody (red), respectively. Reproduced with permission from Jin et al. (2018). Copyright 2017, American Chemical Society.
Iron sulfide holds utility as a drug carrier. In previous studies, modified β-cyclodextrin (β-CD) and PEG Fe3S4 (GMNCs) were used as drug loading NPs. Both β-CD and PEG have been used to control the shape and size of GMNCs as surfactants. In addition, the biocompatibility of Fe3S4 is enhanced, with entrapment efficiencies of 58.7% for the modified delivery of the chemotherapeutic drug doxorubicin. Meanwhile, the enhanced chemotherapeutic treatment of mouse tumors was obtained through the intravenous injection of doxorubicin (Dox) loaded GMNCs (Feng et al., 2013; Figure 7).
Figure 7. (A) Schematical illustration of the greigite-containing magnetosome and the chemical synthesized magnetosome-like GMNCs. (B) Growth inhibition effect in murine S180 sarcoma model of the sample. The photos and weight ratios of tumor tissue from mice treated with normal saline (control group), GMNCs, Dox at low concentration, and GMNCs loading with Dox (without and with the guidance of external magnetic field), respectively. Reproduced with permission from Feng et al. (2013). Copyright 2013, Nature Publishing Group.
In studies of vascular disease, the removal of thrombosis through non-invasive methods is challenging. However, studies regarding iron sulfide NPs as thrombolytic agents have been reported. Ge et al. first highlighted the utility of Fe3S4 NPs as thrombolytic agents with both photothermal and magnetothermal thrombolytic capability. Using Fe3S4 nanoparticles, celiac vein thrombosis could be prevented using magnetic hyperthermia combined PTT. Both in vivo and in vitro, Fe3S4 has demonstrated beneficial effects for the removal of thrombi (Figure 8), providing a novel hyperthermia strategy for the prevention of thrombosis (Fu et al., 2019).
Figure 8. (A) Thrombolytic capacity in vitro of Fe3S4 nanoparticles under the indicated conditions. Fe3S4 nanoparticles: 0.5 mg/mL; NIR: 808 nm, 0.33 W cm−2; AMF: 4.2 × 109 A m−1 s−1. (B) T2-weighted MR imaging in vivo of a mouse with celiac vein thrombosis before (left) and after (right) an intravenous injection of a solution of the Fe3S4 nanoparticles followed by the simulation of AMF. Reproduced with permission from Fu et al. (2019). Copyright 2019, Frontiers in Materials.
Iron sulfides have been used as glucose sensors due to their intrinsic peroxidase-like activity (Dai et al., 2009). Glucose sensors can be developed using colorimetric methods in which cascade reactions form the core mechanism of glucose detection. TMB could be oxidized to oxTMB in the presence of glucose, GOx and iron sulfide. H2O2 produced by the decomposition of glucose in the presence of glucose oxidase can be used as a substrate for iron sulfide. Iron sulfide peroxidase-like mimics can oxidize TMB to oxTMB in the presence of H2O2. Fe7S8 nanowires also possess peroxidase activity. Using a linear range, glucose concentrations of 5 × 10−6 to 5 × 10−4 M could be detected (Yao et al., 2013). In 2016, Xian and colleagues used Fe3S4 magnetic nanoparticles (MNPs) to quantify glucose concentrations in human serum. A linear range was measured from 2 to 100 μM, and the limit of detection (LOD) was 0.16 μM (Ding et al., 2016). These studies highlighted the potential of as-prepared iron sulfide as both glucose sensors and artificial peroxidase nanozymes.
In vitro antifungal FeS NPs exhibited significant anti-fungal activity against F. verticillioides at 18 μg ml−1, with a higher efficiency than standard fungicides (carbendazim (median effective dose (ED50): 230 μg ml−1). These were the first reports highlighting the antifungal activity of iron sulfide. The influence of FeS on both seed health and quality parameters of rice was also evaluated based on this antifungal activity. Iron sulfides were shown to be effective in iron deficient soils as an alternative to high dose organic fungicides (Ahuja et al., 2019).
FeS2 represents a photovoltaic material, which increases plant biomass in the seeds of chickpeas (Cicer arietinum). Meanwhile, the mechanism of functional FeS2 is attributed to its amylase-like activity. In the presence of H2O and FeS2, starch in the seeds can be broken down to H2O2, which participates in the absorption of CO2 and improves plant health. Spinach seeds treated with FeS2 exhibited broader leaf morphologies, larger leaf numbers and an increased biomass (Srivastava et al., 2014b) (Figure 9). In later studies, seed priming with FeS2 was reported as an innovative strategy (Das et al., 2016). FeS2 also improved both seed yield and growth in the Brassica juncea field (Rawat et al., 2017).
Figure 9. Plant growth parameters: control vs. test (FeS2). (a) Leaf area/Plant, showing significant increase in leaf area/test plants (52.4 ± 0.3) as compared to control (25.6 ± 0.2). (b) Leaf area index signifying total photosynthetic area available to plant and high values for test samples (1.5 ± 0.07) can be correlated with high biomass content of pro-fertilized spinach plants in comparison with (0.9 ± 0.02). (c) Comparative photograph of leaves showing larger leaf area in test plants as compared to control plants. Plant growth parameters: control vs. test (FeS2). (d) Number of leaves/Plant: control: 13 ± 1.0; test: 19 ± 1.0. (e) Specific leaf area signifies leaf thickness and was found similar for both test and control samples. (f) Field photograph taken at day 50 (just before harvesting the crop) depicting that the test group plants have comparatively more foliage as compared to the control plants. (g) Proposed outline of the mechanism of action of FeS2 on spinach seed in enhancing germination and plant growth. Reproduced with permission from Srivastava al. (2014). Copyright 2014, The Royal Society of Chemistry.
In summary, we have highlighted the most recent methods of nano-iron sulfide synthesis, including nano iron sulfide modifications and characterizations. Strikingly, nano-sized iron sulfides demonstrate versatile physiochemical properties, enzyme-like catalysis, high stability and biocompatibility, which facilitate their biomedical applications. A range of nano-iron sulfides have been assessed in catalysis, tumor therapy, antibacterials and antifungals, drug delivery, biosensors, thrombus removal and in plants. Their advantages include (1) high biocompatibility due to the key role of iron and sulfur in natural life; (2) the photothermal and magnetic properties of nano-sized iron sulfide; (3) their nanostructure and large surface area that can improve drug delivery; and (4) their enzyme-like properties as nanozymes, including their high reactivity to numerous chemical substances that can regulate hydrogen peroxide, ROS and various catalytic reactions to treat related diseases.
Although it has been shown that nano-sized iron sulfides represent great potential in numerous applications in biomedicine (Scheme 2), a number of issues remain to be addressed, including the synthesis of iron sulfide that is stable and in a single phase, with modifications to adapt to the biological environment. Studies have found that the modification of molecular CTAB inhibits the preparation of Fe3S4 NPs due to competitive inhibition with Na2S under acidic conditions, resulting in the formation of non-magnetic iron sulfides and other byproducts. Citrate modified nanoparticles have not sufficient particle spacing due to aggregation effects (Simeonidis et al., 2016). In addition, the biomedical assessments of iron sulfides remain sparse, and their mechanisms of action under physiological conditions are poorly understood. The intrinsic enzyme-like properties of iron sulfide as nanozymes may provide a window to understand its biological effects and potential cytotoxicity in vivo. Taken together, nano-sized iron sulfides possess versatile physiochemical properties and enzyme-like properties, which describe a form of distinctive nanomaterials with great potential for use in biomedical applications.
Scheme 2. Schematic diagram of biomedical applications based on physiochemical properties of nano-sized iron sulfide.
All authors listed have made a substantial, direct and intellectual contribution to the work, and approved it for publication.
The authors declare that the research was conducted in the absence of any commercial or financial relationships that could be construed as a potential conflict of interest.
This work was supported by the National Key R&D Program of China (Grant No. 2018YFC1003500), as well as the National Natural Science Foundation of China (Grant Nos. 81930050, 81671810, and 31701236).
Agnihotri, S., Mohan, T., Jha, D., Gautam, H., and Roy, I. (2020). Dual Modality FeS Nanoparticles with Reactive Oxygen Species-Induced and Photothermal Toxicity toward Pathogenic Bacteria. ACS OMEGA 5, 597–602. doi: 10.1021/acsomega.9b03177
Ahuja, R., Sidhu, A., and Bala, A. (2019). Synthesis and evaluation of iron(ii) sulfide aqua nanoparticles (FeS-NPs) against Fusarium verticillioides causing sheath rot and seed discoloration of rice. Eur. J. Plant Pathol. 155, 163–171. doi: 10.1007/s10658-019-01758-3
Argueta-Figueroa, L., Martinez-Alvarez, O., Santos-Cruz, J., Garcia-Contreras, R., Acosta-Torres, L. S., Fuente-Hernández, J., et al. (2017). Nanomaterials made of non-toxic metallic sulfides: a systematic review of their potential biomedical applications. Mater. Sci. Eng. C 76, 1305–1315. doi: 10.1016/j.msec.2017.02.120
Argueta-Figueroa, L., Torres-Gómez, N., García-Contreras, R., Vilchis-Nestor, A. R., Martínez-Alvarez, O., Acosta-Torres, L. S., et al. (2018). Hydrothermal synthesis of pyrrhotite (Fex−1S) nanoplates and their antibacterial, cytotoxic activity study. Progress Nat. Sci. 28, 447–455. doi: 10.1016/j.pnsc.2018.06.003
Barand, A., and Russo, S. (2009). Modeling the environmental stability of FeS2 nanorods, using lessons from biomineralization. Nanotechnology 20:115702. doi: 10.1088/0957-4484/20/11/115702
Barnard, A., and Russo, S. (2009). Morphological stability of Pyrite FeS2 nanocrystals in water. J. Phys. Chem. C 113, 5376-5380. doi: 10.1021/jp809377s
Beal, J., Etchegoin, P., and Tilley, R. (2012). Synthesis and characterisation of magnetic iron sulfide nanocrystals. J. Solid State Chem. 189, 57–62. doi: 10.1016/j.jssc.2012.01.015
Bi, Y., Yuan, Y., Exstrom, C. L., Darveau, S. A., and Huang, J. (2011). Air stable, photosensitive, phase pure iron pyrite nanocrystal thin films for photovoltaic application. Nano Letter 11, 4953–4957. doi: 10.1021/nl202902z
Chang, Y., Savitha, S., Sadhasivam, S., Hsu, C., and Lin, F. (2011). Fabrication, characterization, and application of greigite nanoparticles for cancer hyperthermia. J. Colloid Interface Sci. 363, 314–319. doi: 10.1016/j.jcis.2010.06.069
Chen, R., and Chen, G. (2017). Tumor-induced disorder of iron metabolism in major organs: a new insight from chemical speciation of iron. J. Int. Med. Res 46, 70–78. doi: 10.1177/0300060517718711
Chen, B., and Gu, N. (2017). Current status and development of pharmaceutical iron based nanomaterials. Mater. China 36, 211–218.
Cohn, C., Laffers, R., Simon, S., O'Riordan, T., and Schoonen, M. (2006). Role of pyrite in formation of hydroxyl radicals in coal: possible implications for human health. Part. Fibre Toxicol. 3:16. doi: 10.1186/1743-8977-3-16
Dai, Z., Liu, S., Bao, J., and Ju, H. (2009). Nanostructured FeS as a mimic peroxidase for biocatalysis and biosensing. Chem. Eur. J. 15, 4321–4326. doi: 10.1002/chem.200802158
Das, C., Srivastava, G., Dubey, A., Roy, M., Jain, S., Sethy, N., et al. (2016). Nano-ironpyrite seed dressing: A sustainable intervention to reduce fertilizer consumption in vegetable (beetroot, carrot), spice (fenugreek), fodder (alfalfa), and oilseed (mustard, sesamum) crops. Nanotechnol. Environ. Eng. 1, 1–12. doi: 10.1007/s41204-016-0002-7
Davison, W. (1991). The solubility of iron sulphides in synthetic and natural waters at ambient temperature. Aquatic Sci. 53, 309–329. doi: 10.1007/BF00877139
De Leeuw, N., Parker, S., Sithole, H., and Ngoepe, P. E. (2000). Modeling the surface structure and reactivity of pyrite: introducing a potential model for FeS2. J. Phys. Chem. B 104, 7969–7976. doi: 10.1021/jp0009498
Ding, B., Yu, C., Li, C., Deng, X., Ding, J., Cheng, Z., et al. (2017). Cis-platinum pro-drug-attached CuFeS2 nanoplates for in vivo photothermal/photoacoustic imaging and chemotherapy/photothermal therapy of cancer. Nanoscale 9, 16937–16949. doi: 10.1039/C7NR04166G
Ding, C., Yan, Y., Xiang, D., Zhang, C., and Xian, Y. (2016). Magnetic Fe3S4 nanoparticles with peroxidase-like activity, and their use in a photometric enzymatic glucose assay. Microchim. Acta 183, 625–631. doi: 10.1007/s00604-015-1690-6
Dong, H., Fan, Y., Zhang, W., Gu, N., and Zhang, Y. (2019). Catalytic mechanisms of nanozymes and their applications in biomedicine. Bioconjug. Chem. 30, 1273–1296. doi: 10.1021/acs.bioconjchem.9b00171
Elliot, A. (2010). Structure of pyrrhotite 5c (Fe9S10). Acta Crystallogr. Section B 66, 271–279. doi: 10.1107/S0108768110011845
Esmaeili, E., Eslami-Arshaghi, T., Hosseinzadeh, S., Elahirad, E., Jamalpoor, Z., Hatamie, S., et al. (2020). The biomedical potential of cellulose acetate/polyurethane nanofibrous mats containing reduced graphene oxide/silver nanocomposites and curcumin: antimicrobial performance and cutaneous wound healing. Int. J. Biol. Macromol. 152, 418–427. doi: 10.1016/j.ijbiomac.2020.02.295
Feng, M., Lu, Y., Yang, Y., Zhang, M., Xu, Y., Gao, H., et al. (2013). Bioinspired greigite magnetic nanocrystals: chemical synthesis and biomedicine applications. Sci. Rep. 3:994. doi: 10.1038/srep02994
Fleet, M. (1971). The crystal structure of a pyrrhotite (Fe7S8). Acta Cryst. B 27:1864. doi: 10.1107/S0567740871004990
Fu, D., Liu, J., Ren, Q., Ding, J., Ding, H., Chen, X., et al. (2019). magnetic iron sulfide nanoparticles as thrombolytic agents for magnetocaloric therapy and photothermal therapy of thrombosis. Front. Mater. 6:316. doi: 10.3389/fmats.2019.00316
Gan, Y., Xu, F., Luo, J., Yuan, H., Jin, C., Zhang, L., et al. (2016). One-pot biotemplate synthesis of FeS2 decorated sulfur-doped carbon fiber as high capacity anode for lithium-ion batteries. Electrochim. Acta 209, 201–209. doi: 10.1016/j.electacta.2016.05.076
Ganbaatar, N., Matsuzaki, N., Nakazawa, Y., Afrin, R., Aono, M., Yano, T., et al. (2016). Surface force analysis of pyrite (FeS2): its reactivity to amino acid adsorption. Adv. Mater. Phys. Chem. 6, 167–176. doi: 10.4236/ampc.2016.67018
Gao, L., Zhuang, J., Nie, L., Zhang, J., Zhang, Y., Gu, N., et al. (2007). Intrinsic peroxidase-like activity of ferromagnetic nanoparticles. Intrinsic peroxidase-like activity of ferromagnetic nanoparticles. Nat. Nanotechnol. 2, 577–583. doi: 10.1038/nnano.2007.260
Gao, S., Huang, F., Song, D., Li, G., Liu, Q., Feng, T., et al. (2015). “Growth mechanism and stability study on the Fe3S4 nanocrystals synthesized under thermal and humid conditions,” in Proceedings of the 11th International Congress for Applied Mineralogy. doi: 10.1007/978-3-319-13948-7_13
Guan, G., Wang, X., Li, B., Zhang, W., Cui, Z., Lu, X., et al. (2018). “Transformed” Fe3S4 tetragonal nanosheets: a high-efficiency and body-clearable agent for magnetic resonance imaging guided photothermal and chemodynamic synergistic therapy. Nanoscale 10, 17902–17911. doi: 10.1039/C8NR06507A
Guo, S., Li, J., Ma, Z., Chi, Y., and Xue, H. (2016). A facile method to prepare FeS/porous carbon composite as advanced anode material for lithium-ion batteries. J. Mater. Sci. 52, 2345–2355. doi: 10.1007/s10853-016-0527-y
Guo, Z., Sun, F., Han, B., Lin, K., Zhou, L., and Yuan, W. (2017). Iron vacancy in tetragonal Fe1−xS crystals and its effect on the structure and superconductivity. Phys. Chem. Chem. Phys. 19, 9000–9006. doi: 10.1039/C7CP00068E
He, Q., Huang, C., and Liu, J. (2013). Preparation, Characterization and antibacterial activity of magnetic greigite and Fe3S4/Ag nanoparticles. Nanosci. Nanotechnol. Lett. 5, 1–8. doi: 10.1166/nnl.2014.1727
He, Y., Wilson, J., Su, C., and Wilkin, R. T. (2015). Review of abiotic degradation of chlorinated solvents by reactive iron minerals in aquifers. Ground Water Monitoring Remediation 35, 57–75. doi: 10.1111/gwmr.12111
Jin, A., Mi-Ju, K., Kug-Seung, L., Yu, S., and Sung, Y. (2019). Spindle-like Fe7S8/N-doped carbon nanohybrids for high-performance sodium ion battery anodes. Nano Res. 12, 695–700. doi: 10.1007/s12274-019-2278-y
Jin, J., Wu, W., Min, H., Wu, H., Wang, S., Ding, Y., et al. (2017). A glassy carbon electrode modified with FeS nanosheets as a highly sensitive amperometric sensor for hydrogen peroxide. Microchim. Acta 184, 1389–1396. doi: 10.1007/s00604-017-2105-7
Jin, Q., Liu, J., Zhu, W., Dong, Z., Liu, Z., and Cheng, L. (2018). Albumin-assisted synthesis of ultrasmall FeS2 nanodots for imaging-guided photothermal enhanced photodynamic therapy. ACS Appl. Mater. Interfaces 10, 332–340. doi: 10.1021/acsami.7b16890
Kar, S., and Chaudhuri, S. (2004). Solvothermal synthesis of nanocrystalline FeS2 with different morphologies. Chem. Phys. Lett. 398, 22–26. doi: 10.1016/j.cplett.2004.09.028
Kavner, A., Duffy, T., and Shen, G. (2001). Phase stability and density of FeS at high pressures and temperatures: implications for the interior structure of Mars. Earth Planet. Sci. Lett. 185, 25–33. doi: 10.1016/S0012-821X(00)00356-3
Kim, E. J., and Batchelor, B. (2009). Synthesis and characterization of pyrite (FeS2) using microwave irradiation. Mater. Res. Bull. 44, 1553–1558. doi: 10.1016/j.materresbull.2009.02.006
Kong, X., Lou, T., and Li, Y. (2005). Fe7S8 nanorods and nanosheets. J. Alloys Compd. 390, 236–239. doi: 10.1016/j.jallcom.2004.07.054
Kuhn, S. J., Eskildsen, M. R., Debeer-Schmitt, L., Li, L., de La Cruz, C., and Sefat, A. S. (2016). Structure and magnetic interactions in FeS: a low-Tc superconductor. Bull. Am. Phys. Soc. 61, 2. Abstracts retrieved from APS March Meeting (Abstract ID: BAPS.2016.MAR.H11.12).
Kwon, K., Refson, K., Bone, S., Qiao, R., Yang, W., Liu, Z., et al. (2011). Magnetic ordering in tetragonal FeS: evidence for strong itinerant spin fluctuations. Phys. Rev. B Cond. Matt. 83, 064402.1–064402.7. doi: 10.1103/PhysRevB.83.064402
Lefèvre, C., Abreu, F., Lins, U., and Bazylinski, D. (2010). Nonmagnetotactic multicellular prokaryotes from low-saline, nonmarine aquatic environments and their unusual negative phototactic behavior. Appl. Environ. Microbiol. 76, 3220–3227. doi: 10.1128/AEM.00408-10
Li, M., Yao, Q., Zhou, G., Qu, X., Mu, C., and Fu, S. (2011). Microwave-assisted controlled synthesis of monodisperse pyrite microspherolites. Cryst. Eng. Comm. 13, 5936–5942. doi: 10.1039/c1ce05478c
Li, X., Xu, H., Chen, Z., Chen, Z., and Chen, G. (2011). Biosynthesis of nanoparticles by microorganisms and their applications. J. Nanomater. 2011, 1–16. doi: 10.1155/2011/270974
Li, Y., Pu, Q., Li, S., Zhang, H., Wang, X., Yao, H., et al. (2019). Machine learning methods for research highlight prediction in biomedical effects of nanomaterial application. Patt. Recognit. Lett. 117, 111–118. doi: 10.1016/j.patrec.2018.11.008
Liang, M., and Yan, X. (2019). Nanozymes: from new concepts, mechanisms, and standards to applications. Acc. Chem. Res. 52, 2190–2200. doi: 10.1021/acs.accounts.9b00140
Ma, J., Chang, L., Lian, J., Huang, Z., Duan, X., Liu, X., et al. (2010). Ionic liquid-modulated synthesis of ferrimagnetic Fe3S4 hierarchical superstructures. Chem. Commun. 46, 5006–5008. doi: 10.1039/c0cc00479k
Manafi, A., Hosseini, M., Fakhri, A., Gupta, V., and Agarwal, S. (2019). Investigation of photocatalytic process for iron disulfide-bismuth oxide nanocomposites by using response surface methodology: structural and antibacterial properties. J. Mol. Liq. 289:110950. doi: 10.1016/j.molliq.2019.110950
Mann, S., Sparks, N., Frankel, R., Bazylinski, D., and Jannasch, H. (1990). Biomineralization of ferrimagnetic greigite (Fe3S4) and iron pyrite (FeS2) in a magnetotactic bacterium. Nature 343, 258–261. doi: 10.1038/343258a0
Mei, B., and Ma, Z. (2013). Study of anti-friction performance of spherical FeS nanoparticle. Appl. Mechan. Mater. 475-476, 1334–1339. doi: 10.4028/www.scientific.net/AMM.475-476.1334
Menyeh, A., and O'Reilly, W. (1997). Magnetic hysteresis properties of fine particles of monoclinic pyrrhotite Fe7S8. J. Geomag. Geoelectr. 49, 965–976. doi: 10.5636/jgg.49.965
Mofokeng, T., Mabena, G., Moloto, M. J., Shumbula, P. M., Mubiayi, P., and Nyamukamba, P. (2017). Temperature influence on the lactose capped metal sulphide nanoparticles. Chalcogenide Lett. 14, 347–355.
Mohindar, S., and Jagadeesh, M. (1979). Temperature-dependent magnetic susceptibility of marcasite (FeS_ {2}). Phys. Rev. B 20:3897. doi: 10.1103/PhysRevB.20.3897
Moore, J., Nienhuis, E., Ahmadzadeh, M., and Mccloy, J. (2019). Synthesis of greigite (Fe3S4) particles via a hydrothermal method. AIP Adv. 9:035012. doi: 10.1063/1.5079759
Niño, M., Flores, E., Sanchez, C., and Rojo, J. (2018). Reactivity of a FeS surface under room temperature exposure to nitrogen and H2S. J. Phys. Chem. B 122, 705–712. doi: 10.1021/acs.jpcb.7b06309
Ono, S. (2007). Magnetic phase transition of FeS at high pressures. Acta Crystallogr. Sect. A Found. Crystallogr. 63:59. doi: 10.1107/S0108767307098716
Paolella, A., George, C., Povia, M., Zhang, Y., Krahne, R., Gich, M., et al. (2011). Charge transport and electrochemical properties of colloidal greigite (Fe3S4) nanoplatelets. Chem. Mater. 23, 3762–3768. doi: 10.1021/cm201531h
Powell, A. V., Vaqueiro, P., Knight, K. S., Chapon, L. C., and Sánchez, R. D. (2004). Structure and magnetism in synthetic pyrrhotite Fe7S8: a powder neutron-diffraction study. Phys. Rev. B 70, 014415. doi: 10.1103/PhysRevB.70.014415
Qi, W., and Cowan, J. (2011). Structural, mechanistic and coordination chemistry of relevance to the biosynthesis of iron–sulfur and related iron cofactors. Coord. Chem. Rev. 255, 688–699. doi: 10.1016/j.ccr.2010.10.016
Rawat, M., Nayan, R., Negi, B., Zaidi, M. G. H., and Arora, S. (2017). Physio-biochemical basis of iron-sulfide nanoparticle induced growth and seed yield enhancement in b. juncea. Plant Physiol. Biochem. 118, 274–284. doi: 10.1016/j.plaphy.2017.06.021
Rickard, D. (2006). The solubility of FeS. Geochim. Cosmochim. Acta 70, 5779–5789. doi: 10.1016/j.gca.2006.02.029
Rickard, D., and Luther, G. (2007). Chemistry of iron sulfides. Chem. Rev. 107, 514–562. doi: 10.1021/cr0503658
Roberts, A. (1995). Magnetic properties of sedimentary greigite (Fe3S4). Earth Planetary Sci. Lett. 134, 227–236. doi: 10.1016/0012-821X(95)00131-U
SantosCarballal, D., Roldan, A., Dzade, N., and de Leeuw, N. H. (2017). Reactivity of CO2 on the surfaces of magnetite (Fe3O4), greigite (Fe3S4) and mackinawite (FeS). Philos. Trans. R. Soc. A 376:65. doi: 10.1098/rsta.2017.0065
Scaini, M., Bancroft, G., and Knipe, S. (1998). Reactions of aqueous Au1+ sulfide species with pyrite as a function of pH and temperature. Am. Mineral. 83, 316–322. doi: 10.2138/am-1998-3-415
Schoonen, M., Cohn, C., Roemer, E., Laffers, R., Simon, S., and O'Riordan, T. (2006). Mineral-induced formation of reactive oxygen species. Med. Mineraol. Geochem. 64, 179–221. doi: 10.2138/rmg.2006.64.7
Simeonidis, K., Liébana-Viñas, S., Wiedwald, U., Ma, Z., Li, Z.-A., Spasova, M., et al. (2016). A versatile large-scale and green process for synthesizing magnetic nanoparticles with tunable magnetic hyperthermia features. RSC Adv. 6, 53107–53117. doi: 10.1039/C6RA09362K
Snowball, I. (1991). Magnetic hysteresis properties of greigite (Fe3S4) and a new occurrence in holocene sediments from swedish lappland. Phys. Earth Planetary Interiors 68, 32–40. doi: 10.1016/0031-9201(91)90004-2
Srivastava, G., Das, A., Kusurkar, T., Roy, M., Airan, S., Sharma, R., et al. (2014a). Ironpyrite, a potential photovoltaic material, increases plant biomass upon seed pretreatment. Mater. Express 4, 23–31. doi: 10.1166/mex.2014.1139
Srivastava, G., Das, C. K., Das, A., Singh, S., Roy, M., Kim, H., et al. (2014b). Seed treatment with iron pyrite (FeS2) nanoparticles increases the production of spinach. RSC Adv. 4, 58495–58504. doi: 10.1039/C4RA06861K
Usher, C., Paul, K., Narayansamy, J., Kubicki, J., Sparks, D., Schoonen, M., et al. (2005). Mechanistic aspects of pyrite oxidation in an oxidizing gaseous environment: an in situ HATR–IR isotope study. Environ. Sci. Technol. 39, 7576–7584. doi: 10.1021/es0506657
Vanitha, P., and O'Brien, P. (2008). Phase Control In The Synthesis Of Magnetic iron sulfide nanocrystals from a cubane-type Fe-S cluster. J. Am. Chem. Soc. 130, 17256–17257. doi: 10.1021/ja8078187
Wadia, C., Wu, Y., Gul, S., Volkman, S., Guo, J., and Paul Alivisatos, A. (2009). Surfactant-assisted hydrothermal synthesis of single phase pyrite FeS2 nanocrystals. Chem. Mater. 21, 2568–2570. doi: 10.1021/cm901273v
Wang, X., Zhou, W., Zhou, Z., An, Y., and Wu, S. (2013). Shape-controlled synthesis of iron sulfide nanostructures by thermal decomposition of organometallic precursors. Mater. Sci. Semicond. Proc. 16, 530–536. doi: 10.1016/j.mssp.2012.10.002
Wang, Z., Hu, T., Liang, R., and Wei, M. (2020). Application of zero-dimensional nanomaterials in biosensing. Front. Chem. 8:320. doi: 10.3389/fchem.2020.00320
Watson, J., Cressey, B., Roberts, A., Ellwood, D. C., Charnock, J., and Soper, A. K. (2000). Structural and magnetic studies on heavy-metal-adsorbing iron sulphide nanoparticles produced by sulphate-reducing bacteria. J. Magn. Magn. Mater. 214, 13–30. doi: 10.1016/S0304-8853(00)00025-1
Watson, J., Ellwood, D., Deng, Q., Mikhalovsky, S., Hayter, C. E., and Evans, J. (1995). Heavy metal adsorption on bacterially produced FeS. Minerals Eng. 8, 1097–1108. doi: 10.1016/0892-6875(95)00075-2
Watson, J., Ellwood, D., Soper, A., and Charnock, J. (1999). Nanosized strongly-magnetic bacterially-produced iron sulfide materials. J. Magnetism Magnetic Mater. 203, 69–72. doi: 10.1016/S0304-8853(99)00191-2
Wei, H., and Wang, E. (2013). Nanomaterials with enzyme-like characteristics (nanozymes): next-generation artificial enzymes. Chem. Soc. Rev. 42, 6060–6093. doi: 10.1039/c3cs35486e
Xiao, S., Cheng, M., Zhong, H., Liu, Z., Liu, Y., Yang, X., et al. (2020). Iron-mediated activation of persulfate and peroxymonosulfate in both homogeneous and heterogeneous ways: a review. Chem. Eng. J. 384:123265. doi: 10.1016/j.cej.2019.123265
Xie, J., Zhang, X., Wang, H., Zheng, H., Huang, Y., and Xie, J. (2012). Analytical and environmental applications of nanoparticles as enzyme mimetics. Trac Trends Analyt. Chemistry 39, 114–129. doi: 10.1016/j.trac.2012.03.021
Xu, Z., Qiu, Z., Liu, Q., Huang, Y., Li, D., Shen, X., et al. (2018). Converting organosulfur compounds to inorganic polysulfides against resistant bacterial infections. Nat. Commun. 9:3713. doi: 10.1038/s41467-018-06164-7
Yang, K., Yang, G., Chen, L., Cheng, L., Wang, L., Ge, C., et al. (2015). FeS nanoplates as a multifunctional nano-theranostic for magnetic resonance imaging guided photothermal therapy. Biomaterials 38, 1–9. doi: 10.1016/j.biomaterials.2014.10.052
Yang, W., Xiang, C., Xu, Y., Chen, S., Zeng, W., Liu, K., et al. (2020). Albumin-constrained large-scale synthesis of renal clearable ferrous sulfide quantum dots for T1-Weighted MR imaging and phototheranostics of tumors. Biomaterials 255:120186. doi: 10.1016/j.biomaterials.2020.120186
Yao, W., Zhu, H., Li, W., Yao, H., Wu, Y., and Yu, S. (2013). Intrinsic peroxidase catalytic activity of Fe7S8 nanowires templated from [Fe16S20]/diethylenetriamine hybrid nanowires. Chem. Plus Chem. 78, 723–727. doi: 10.1002/cplu.201300075
Yosida, K. (1951). Note on the magnetic properties of the FeSn system. Progr. Theor. Phys. 6, 356–365. doi: 10.1143/ptp/6.3.356
Zhang, L., and Webster, T. (2009). Nanotechnology and nanomaterials: promises for improved tissue regeneration. Nano Today 4, 66–80. doi: 10.1016/j.nantod.2008.10.014
Zhang, M., Cui, Z., and Jiang, H. (2018). Relative stability of FeS2 polymorphs with the random phase approximation approach. J. Mater. Chem. A 6:6606. doi: 10.1039/C8TA00759D
Keywords: nano-sized iron sulfide, structure, synthesis, enzyme-like activities, biomedical applications
Citation: Yuan Y, Wang L and Gao L (2020) Nano-Sized Iron Sulfide: Structure, Synthesis, Properties, and Biomedical Applications. Front. Chem. 8:818. doi: 10.3389/fchem.2020.00818
Received: 19 May 2020; Accepted: 04 August 2020;
Published: 10 September 2020.
Edited by:
Youhui Lin, Xiamen University, ChinaReviewed by:
Xian Chen, Shenzhen University, ChinaCopyright © 2020 Yuan, Wang and Gao. This is an open-access article distributed under the terms of the Creative Commons Attribution License (CC BY). The use, distribution or reproduction in other forums is permitted, provided the original author(s) and the copyright owner(s) are credited and that the original publication in this journal is cited, in accordance with accepted academic practice. No use, distribution or reproduction is permitted which does not comply with these terms.
*Correspondence: Lizeng Gao, Z2FvbGl6ZW5nQGlicC5hYy5jbg==
Disclaimer: All claims expressed in this article are solely those of the authors and do not necessarily represent those of their affiliated organizations, or those of the publisher, the editors and the reviewers. Any product that may be evaluated in this article or claim that may be made by its manufacturer is not guaranteed or endorsed by the publisher.
Research integrity at Frontiers
Learn more about the work of our research integrity team to safeguard the quality of each article we publish.