- 1School of Engineering, RMIT University, Melbourne, VIC, Australia
- 2Applied Porous Materials Unit, Commonwealth Scientific and Industrial Research Organisation (CSIRO), Clayton, VIC, Australia
- 3School of Materials and Energy, University of Electronic Science and Technology of China, Chengdu, China
- 4College of Textile Science and Engineering (International Institute of Silk), Zhejiang Sci-Tech University, Hangzhou, China
- 5School of Materials Science and Engineering, Jiangxi University of Science and Technology, Ganzhou, China
- 6Department of Chemical Engineering, The University of Melbourne, Parkville, VIC, Australia
- 7School of Engineering, Albaha University, Al Bahah, Saudi Arabia
- 8Department of Materials Science and Engineering, ARC Centre of Excellence in Exciton Science, Monash University, Clayton, VIC, Australia
- 9National Engineering Researching Centre of Electromagnetic Radiation Control Materials, State Key Laboratory of Electronic Thin Films and Integrated Devices, University of Electronic Science and Technology of China, Chengdu, China
- 10School of Chemical and Biomolecular Engineering, The University of Sydney, Sydney, NSW, Australia
Phosphides of transition metals (TMPs) are a developing class of materials for hydrogen evolution reaction (HER) as an alternative to expensive noble metals to produce clean energy. Herein, the nitrogen-doped molybdenum oxide (MoOx) is developed via a facile and simple hydrothermal method, followed by annealing in the N2 atmosphere and phosphorization to form a nitrogen-doped oxygenated molybdenum phosphide (N-MoP) sphere-shaped structure. The developed N-doped phosphide structure depicts enhanced HER activity by reaching a current density of 10 mA cm−2 at a very low overpotential of only 87 mV, which is much better than annealed nitrogen-doped molybdenum oxide (A-MoOx) 138 mV in alkaline medium. N-MoP is a highly efficient electrocatalyst for HER attributed to a more exposed surface, large electrode/electrolyte interface and appropriate binding energies for reactants. This study extends the opportunity of developing nitrogen-doped TMPs, which can display exceptional properties as compared to their oxides.
Introduction
Excessive use of fossil fuels and other non-renewable energy sources has led to their fast depletion and the omnipresent issue of environmental pollution (Jiao et al., 2015; Yuan et al., 2016; Li et al., 2020). It is urgent to find out the alternative sources of energy that have the potential to replace the swiftly exhausting fossil fuels. Hydrogen, due to its recyclability and high energy density, has emerged as a clean energy source (Mahmood et al., 2018; Haque et al., 2019; Ren Q. et al., 2019; Surendran et al., 2019b; Aslam et al., 2020). The electrochemical water splitting via hydrogen evolution reaction (HER) is expected to provide a favorable pathway for inexpensive H2 generation, which can be used as an alternative fuel to fossil fuels (Turner, 2004; Zou and Zhang, 2015; Surendran et al., 2018, Surendran et al., 2019a). Noble metals such as platinum (Pt/C) perform exceptionally well when used as an electrocatalyst for HER in acidic media (Xiao et al., 2014); however, its wide-scale application is hindered due to high cost and scarcity in the earth's crust (Xiao et al., 2014; Zhou et al., 2017; Li et al., 2020). Therefore, the focus has been shifted toward finding the electrocatalysts which are earth abundant and inexpensive and possessed a similar or better performance than precious metals. Recently, transition-metal alloys, nitrides, sulfides, oxides, selenides, phosphides, borides, and carbides have been studied (Fosdick et al., 2014; Yuan et al., 2016; Zhang et al., 2017; Zhao et al., 2018; Lian et al., 2019; Zou et al., 2020). Out of these materials, transition metal phosphides (TMPs) have shown superior activity and stability (Ren Q. et al., 2019; Zou et al., 2020). Another challenge associated with electrocatalysts is the type of electrolyte being used during the reaction. Particularly, the highly corrosive nature of acidic media has increased the cost of setting up the system, producing safety concerns, and instability (Subbaraman et al., 2011). Thus, it is required to search for an effective electrocatalyst that can perform well in the basic media and have low energy requirements. In recent years, molybdenum-based compounds have emerged as potential candidates for HER (Li et al., 2018; Yao et al., 2018; An et al., 2019). Molybdenum base compounds showed better performance in the acidic medium as compared to the alkaline medium (Haque et al., 2019). For sustainable development of hydrogen as cleaner energy, HER in the alkaline medium is a point of focus (Mahmood et al., 2018). Initially, molybdenum sulfide was prepared to be applied as an electrocatalyst, but high overpotential requirement and poor stability in the alkaline medium have limited its long-term use (Anjum et al., 2018). Various other Mo-based compounds including carbides, borides, and nitrides were studied in the alkaline medium, but their use was hindered by poor stability and higher overpotentials (Haque et al., 2019). To meet up the challenges of developing an electrocatalyst with good performance and stability, the oxides of molybdenum are a good solution because at room temperature one of the oxides, i.e., molybdenum trioxide, is chemically inert (Datta et al., 2017). However, when it comes to long-term stability tests, these trioxides do not maintain a consistent performance which paved the way for MoP as an electrocatalyst (Xiao et al., 2014; Haque et al., 2019). Moreover, nitrogen doping helps in improving electrocatalytic performance by creating positively charged sites (Yuan et al., 2016; Sim et al., 2020). Experiments carried out by Xing et al. (2014) resulted in the development of the closely linked networked structure of MoP nanoparticles, which showed the best HER performance with an overpotential of 125 mV to achieve a current density of 10 mA cm−2. However, there is a considerable gap in understanding the HER activity on N-doped MoP. Therefore, there is a need to develop some nitrogen-doped MoP-based novel structures for an enhanced performance and stability in the alkaline medium. Previously, N-doped MoOx by crystal phase transition was reported to have efficient and stable HER activity (Haque et al., 2019). Here, we present that the thermal conversion of nitrogen-doped MoOx into phosphide can further enhance the HER catalytic activities in the alkaline medium. The nitrogen-doped molybdenum phosphide (N-MoP) is synthesized using a facile and simple hydrothermal method followed by annealing and phosphorization. The improved performance is due to the nitrogen doping along with thermal phosphorization of MoOx, which increases the active sites for electrocatalysis. N-MoP is tested in an alkaline medium in terms of overpotential, durability, stability, and electrochemical surface area for HER. This study extends the opportunity of developing nitrogen-doped TMPs, which can display exceptional properties as compared to their oxides.
Experimental Section
Materials
Hydrogen peroxide (30% weight) was used along with 99.9% pure Mo powder and 99% pure hexamethylenetetramine (HMTA) purchased from Sigma-Aldrich. Sodium hypophosphite (NaH2PO2) (98–101% weight, Sigma-Aldrich), polytetrafluoroethylene (PTFE) (60% weight, Sigma-Aldrich), potassium hydroxide (KOH, 85–90% weight), and Ni foam (99.9% purity, MTI Corporation). All chemicals were used without any further purification.
Synthesis of Nitrogen-Doped Oxygenated Molybdenum Phosphide
The N-doped MoOx is prepared as reported by Haque et al. (2019); 6 mL of hydrogen peroxide (30% weight) was added in 500 mg of Mo powder in a 20-mL glass vial to form peroxomolybdic solution. In another 20-mL glass vial, 350 mg of HMTA was dissolved in 20 mL of DI water. The HMTA dissolved in DI water was then slowly added into the peroxomolybdic solution and left for magnetic stirring for 30 min. The prepared solution was transferred into the Teflon-lined stainless-steel autoclave. The hydrothermal reaction was then carried out for 24 h at a temperature of 200°C. After experiment completion and cooling at room temperature, the sample obtained was washed with DI water and dried at room temperature, followed by annealing in a tube furnace under an N2 environment at a temperature of 350°C for 2 h at 3°C per min to get annealed MoOx (A-MoOx). The ramp rate in the annealing process was 5°C min−1. Annealing was done to remove adsorbed water and oxygen from the sample as much as possible so that it would not interfere during the phosphorization. 25 mg of annealed sample along with 750 mg sodium hypophosphite was put in a ceramic boat for thermal conversion to phosphide. The ceramic boat was positioned at the center of the tube furnace. The reaction was then carried out at 400°C with a ramping rate of 3°C per min for 3 h under an N2 environment to obtain N-MoP.
Material Characterizations
A FEI Quanta 200 scanning electron microscope (SEM) with an attached energy-dispersive X-ray spectrometer (EDS) was used to check the morphologies and to do the elemental analysis of the samples. The crystal structure of the sample was obtained through X-ray diffraction (XRD) analysis using a Bruker D4 endeavor. A thermo-scientific K-alpha system was used to conduct high-resolution X-ray photoelectron spectroscopy (XPS). The machine consisted of an Al Kα monochromated X-ray source through which the samples were scanned. The sample scanning was done with a dwell time of 50 ms−1 and pass energy of 50 eV. Electrochemical measurements were conducted using a CHI 760D electrochemical workstation (CH instruments).
Electrochemical Measurements
Electrochemical measurements were conducted in 0.1 M KOH solution using a typical three-electrode setup. Ni foam coated with the sample was used as the working electrode, a graphite rod was used as the counter electrode, and Hg/HgO (in 1 M KCl aqueous solution) was used as the reference electrode. The Ni foam was properly cleaned with ethanol and DI water before coating samples on it. The reference electrode was converted to RHE after calibration along with all the potentials that have been mentioned. The polarization curves in the paper were iR-corrected, which were obtained after performing linear sweep voltammetry at a scan rate of 5 mVs−1. The working electrode was prepared by mixing 5 mg of resultant compound in a carbon black solution following the addition of 5 μL of PTFE solution and sonicating it for 10 min. The carbon black solution was prepared by mixing 20 mg carbon in 20 mL IPA and DI water mixture (4:1) followed by sonication for 10 min. After this, 100 μL of the solution was drop-cast on the Ni foam with an area of 0.25 m2 to get 0.4 mg mass loading.
Results and Discussion
The polycrystalline nitrogen-doped MoOx was prepared using the hydrothermal method, annealed, and then thermally converted into N-MoP as described in the experimental section. The annealing process enhanced the crystallinity of A-MoOx, which shows enhanced performance due to the more positive charge sites, large electrode/electrolyte interface, and higher conductivity for electron/ions for HER in 0.1 M KOH. For the crystallographic study, the XRD analysis was performed for A-MoOx and N-MoP; the XRD patterns are shown in Figure 1A. It is quite clear that the XRD patterns match well with the standard patterns JCPDS file no. 47-1320 and JCPDS file no. 26-1273, respectively, indicating the high purity of the crystalline structure of the prepared oxide and oxygenated phosphide (Kumar et al., 2015; Liu et al., 2019). The A-MoOx sample showed that dominant peaks at 12.8, 23.5, 25.7, 27.4, 33.7, and 49.3° correspond to the (001), (101̄), (002), (011), (110), (102), and (020) crystal planes, respectively, while the dominant peaks of N-MoP located at 26, 37, and 53.5° correspond to the planes of (111), (131) and (1̄33), respectively. The morphological features of the developed products were studied using scanning electron microscopy (SEM) analysis. It is noticed that the sphere-shaped structures of the oxide as displayed in Figure 1B do not change even after the conversion to form phosphide as shown in Figure 1C. Further, high-resolution transmission electron microscope (HRTEM) results in Figures 1D,E delineate the lattice spacing of 0.344 and 0.272 nm for the (002) plane of crystalline A-MoOx and N-MoP, respectively, matching well with the XRD findings. The elemental analysis was carried out to investigate the distribution of elements in the product. The EDS mapping of MoOx clearly shows the homogenous dispersion of Mo and O in the product (Figures 1F,G) while N-MoP (Figures 1H,I and Figures S1, S2) indicates the homogeneous dispersion of P in the product with Mo and O. N is not detected in the EDS which might be due to less amount of N.
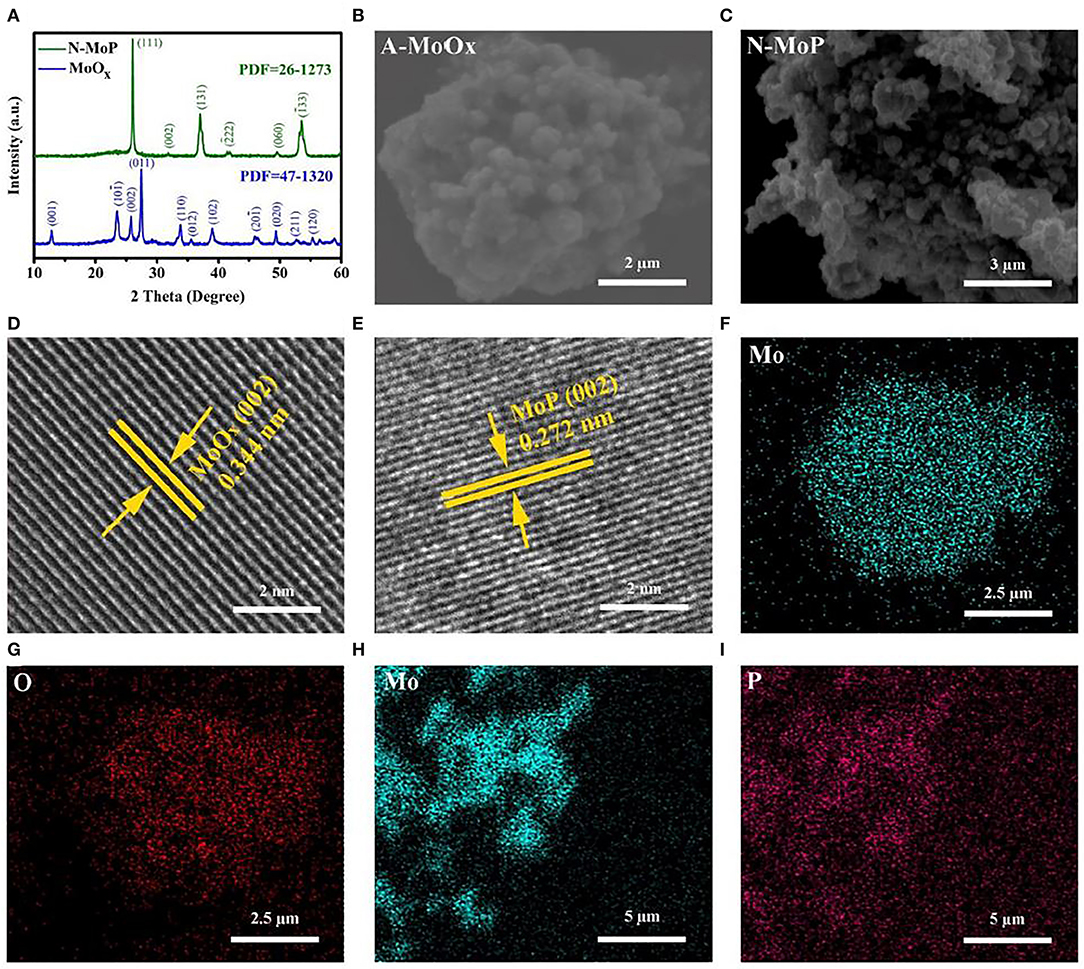
Figure 1. (A) XRD patterns of MoOx and MoP, SEM images of (B) annealed MoOx, (C) N-MoP, HR-TEM images of (D) annealed MoOx, (E) N-MoP, EDS mapping of (F) Mo and (G) O elements in A-MoOx, EDS mapping of (H) Mo, and (I) P elements in the N-MoP sample.
X-ray photoelectron spectroscopy (XPS) was employed to investigate the presence of core levels of the elements as well as their oxidation states. The C1s peak (284.4 eV) was used as a reference to do the peak shift correction. The XPS results show the presence of Mo, N, and O (Figures 2A–C, respectively) in A-MoOx. The peak binding energy at ~236 and ~232.9 eV (Figure 2A) appears due to Mo 3d3/2 and 3d5/2, respectively, which points out the presence of the Mo–O bond (Li et al., 2015; Wen et al., 2019). The deconvoluted peaks of N1s appear in Figure 2B at ~396.7 and ~398.7 attributed to the N-doping in A-MoOx and the peak at ~401 attributed to the surface-adsorbed N2 (Si et al., 2016; Haque et al., 2019). The peak at ~530.7 eV in Figure 2C can be assigned to the O–Mo bond and the other peak at ~532.7 eV to the adsorbed OH group and O–Mo bond (Luo et al., 2016; Zhang et al., 2018; Haque et al., 2019; Mohiuddin et al., 2020). It is evident from the results that Mo, N, and O are mainly present in A-MoOx, indicating the high purity of A-MoOx. The XPS analysis of N-MoP shows the presence of Mo, N, P, and O (Figures 2D–F and Figure S3) in the N-MoP sample. The doublet peaks of Mo 3d3/2 and 3d5/2 appear at ~236.7 and ~233.5 eV, respectively, in Figure 2D (Xing et al., 2014; Ren G. et al., 2019; Wen et al., 2019). The deconvolution peaks of N1s in Figure 2E at ~396.6, ~398.3, and ~399.8 represented the traces of doped N, and the peak at ~401 attributed to adsorbed N2 on the surface (Si et al., 2016; Haque et al., 2019). The deconvoluted peak at ~134.4 eV in Figure 2F suggests the presence of the P–O bond of phosphate (Xing et al., 2014; Mohiuddin et al., 2020), and the peak at ~133.7 eV shows the presence of the Mo–P bond, while the increase (or positively shift) in binding energy peak is attributed to the presence of a higher electronegative value of doped N in the structure (Mhtensson et al., 1995; Khattak et al., 1997; Blanchard et al., 2009; Zhang et al., 2014; Rai et al., 2018; Jeon et al., 2020) as higher electronegative N decreases the electron density to increase the binding energy and the molybdenum phosphate bond's peak centered around 133 eV (Khattak et al., 1997). The adsorbed OH groups on the surface of N-MoP show peaks at 531.9 and 533.4 eV of O1s, as shown in Figure S3. There is no peak that appeared around ~530.7 eV in N-MoP as present in the A-MoOx sample attributed to the cleavage of the Mo–O bond during conversion and successful formation of the Mo–P bond as shown in Figure 2F after phosphorization. The results depicted that Mo, N, P, and O are mainly present in N-MoP attributed to the high purity of prepared N-MoP. Further, A-MoOx having doped N and its conversion into oxygenated phosphide to form N-MoP are verified by XPS data. The presence of nitrogen and phosphorous species in N-MoP makes it a better electrocatalyst for HER than molybdenum oxides, owing to the enhanced conductivity (Wang D. et al., 2019; Wang L. et al., 2019).
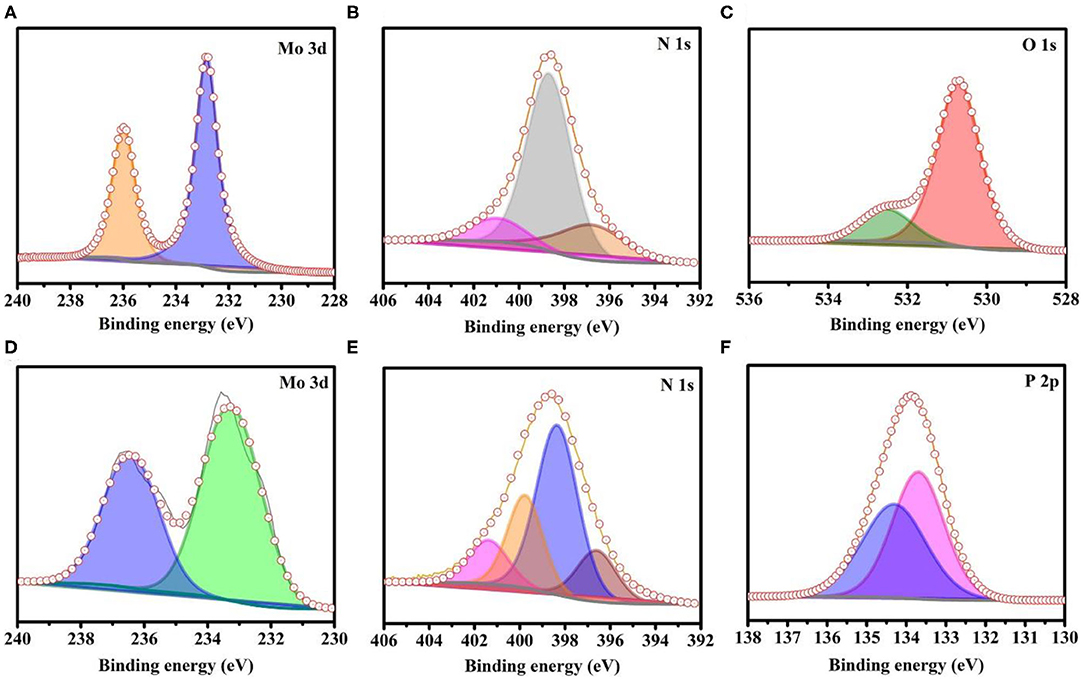
Figure 2. XPS analysis of as-synthesized samples; (A) Mo 3d, (B) N 1s, and (C) O 1s elements for annealed MoOx, (D) Mo 3d, (E) N 1s, and (F) P 2p elements for N-MoP.
Inspired by the pure phase of N-MoP which assists fast mass transport, the electrochemical behavior of the as-developed N-MoP was investigated for the HER activity. The HER was carried out in 3-electrode configuration in 0.1 M KOH, and the results are presented in Figure 3. N-MoP exhibited excellent HER activity by reaching a current density of 10 mA cm−2 at a very low overpotential of only 87 mV, which is much superior to A-MoOx 132 mV at 10 mA cm−2, as delineated by the polarization curves (Figure 3A), and the original reported MoOx has 160 mV at 10 mA cm−2 (Haque et al., 2019). The higher activity can be attributed to the highly exposed surface, large electrode/electrolyte interface, and suitable binding energies for reactants which contribute toward improvement in overall catalytic performance. It can be assumed that the large channels for mass transport ensure fast reaction and evolution of H2, leading to exceptional performance. Furthermore, Mo acts as the hydride acceptor and P works as a proton acceptor. The presence of a large number of Mo–P bonds promotes the formulation of Mo-hydride, which facilitates the HER process on the developed catalyst (Xing et al., 2014). To further understand the reaction mechanism on the catalyst surface, the linear fitted Tafel slopes were plotted and are presented in Figure 3B. The Tafel values of 43.98 and 49.18 mV dec−1 were achieved for N-MoP and A-MoOx, respectively, suggesting that the reaction followed the Tafel–Heyrovsky mechanism (Figure 3B). The better values of Tafel slopes for N-MoP than oxide showed that N-MoP bears better reaction kinetics due to easy access of active sites and better desorption of hydrogen from the surface of catalysts.
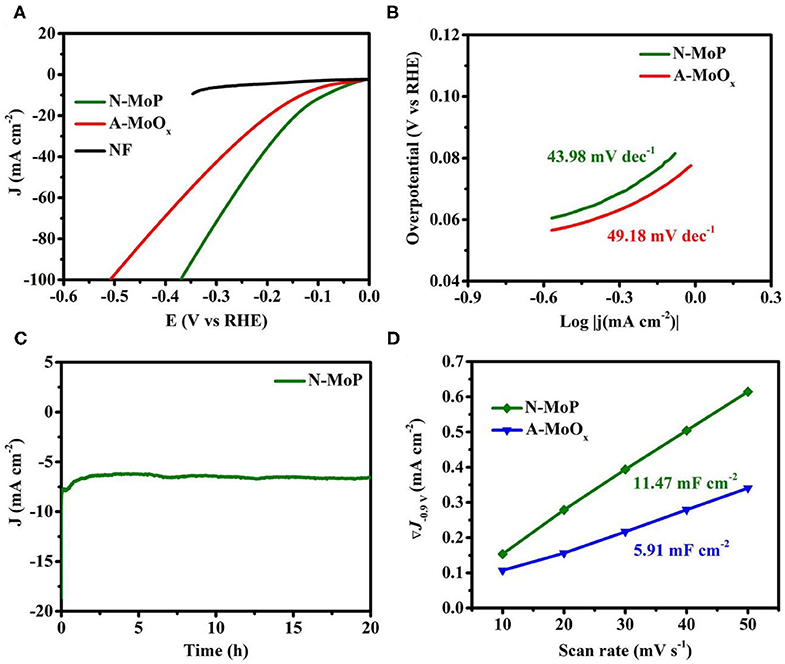
Figure 3. (A) Polarization curves for MoOx, annealed MoOx, N-MoP for HER, (B) Tafel plots, (C) the amperometric i–t plot for stability test of N-MoP, and (D) differences in current density (at a potential of 0.90 V) plotted against scan rate (the marked values in the graph are the fitted slopes) in 0.1 M KOH solution.
Considering the industrial requirements, the long-term durability of N-MoP was measured by amperometric i–t curve technique, as shown in Figure 3C. The developed product shows the minimum loss in activity and current density for a period of 20 h. Such performance and stability assure that N-MoP can be used in commercial electrolyzers. Moreover, N-MoP exhibits much higher electrochemical double-layer capacitances of Cdl of 11.47 mF cm−2 compared to A-MoOx (5.91 mF cm−2) (Figure 3D). Higher ECSA values assure a large electrode/electrolyte interface, more exposed active sites for the enhanced catalysis on the surface of the N-MoP, and higher conductivity of ions and electrons.
Conclusions
The alternatives of precious noble metals have been studied to develop a new class of materials comprising phosphides of transition metals to produce clean energy through HER. N-MoP exhibited an overall particle-like sphere morphology with homogeneous dispersion of N and P in the product. N-MoP exhibited impressive HER activity in an alkaline environment by achieving a current density of 10 mA cm−2 at a very low overpotential of only 87 mV with a Tafel slope of 43.98 mV dec−1 as compared to A-MoOx (132 mV at 10 mA cm−2 with Tafel slope of 49.18 mV dec−1). This enhanced performance is attributed to the extended Mo–P bond formation with assistance of N-doping, which facilitates the HER process. N-MoP also exhibits the long-term stability at least for 20 h with enhanced ECSA, indicating the more electrode/electrolyte interface, higher exposed active sites for the improved catalysis on the surface, and better conductivity of ions and electrons. This facile and simple synthesis of N-MoP with splendid HER performance will offer new possibilities for nitrogen-doped transition metal-based phosphides as an alternative to exorbitant noble metals.
Data Availability Statement
The raw data supporting the conclusions of this article will be made available by the authors, without undue reservation.
Author Contributions
All authors contributed in conducting experiments and writing manuscript.
Conflict of Interest
The authors declare that the research was conducted in the absence of any commercial or financial relationships that could be construed as a potential conflict of interest.
Acknowledgments
The authors would like to acknowledge the Vice-Chancellor fellowship scheme at RMIT University, the RMIT Micro Nano Research Facility (MNRF) in the Victorian node of the Australian National Fabrication Facility (ANFF), and the RMIT Microscopy and Microanalysis Facility (RMMF) to support this work. SL would like to acknowledge the School of Engineering for financial support. MW would like to acknowledge the Higher Education Commission (HEC) of the Pakistan-RMIT University joint program for Ph.D. scholarship. AA would like to acknowledge the Saudi Arabia Ministry of Education and the Saudi Arabian Cultural Mission (SACM) for the Ph.D. scholarship.
Supplementary Material
The Supplementary Material for this article can be found online at: https://www.frontiersin.org/articles/10.3389/fchem.2020.00733/full#supplementary-material
References
An, T.-Y., Surendran, S., Kim, H., Choe, W.-S., Kim, J. K., and Sim, U. (2019). A polydopamine-mediated biomimetic facile synthesis of molybdenum carbide-phosphide nanodots encapsulated in carbon shell for electrochemical hydrogen evolution reaction with long-term durability. Comp. Part B Eng. 175, 107071–107077 doi: 10.1016/j.compositesb.2019.107071
Anjum, M. A. R., Jeong, H. Y., Lee, M. H., Shin, H. S., and Lee, J. S. (2018). Efficient hydrogen evolution reaction catalysis in alkaline media by all-in-one MoS2 with multifunctional active sites. Adv. Mater. 30, 1–9. doi: 10.1002/adma.201707105
Aslam, S., Sagar, R. U. R., Kumar, H., Zhang, G., Nosheen, F., Namvari, M., et al. (2020). Mixed-dimensional heterostructures of hydrophobic/hydrophilic graphene foam for tunable hydrogen evolution reaction. Chemosphere 245, 1–8. doi: 10.1016/j.chemosphere.2019.125607
Blanchard, P. E. R., Grosvenor, A. P., Cavell, R. G., and Mar, A. (2009). Effects of metal substitution in transition-metal phosphides (Ni1−−xM′x)2P (M′ = Cr, Fe, Co) studied by X-ray photoelectron and absorption spectroscopy. J. Mater. Chem. 19, 6015–6022. doi: 10.1039/b904250d
Datta, R. S., Haque, F., Mohiuddin, M., Carey, B. J., Syed, N., Zavabeti, A., et al. (2017). Highly active two dimensional α-MoO3−−x for the electrocatalytic hydrogen evolution reaction. J. Mater. Chem. A 5, 24223–24231. doi: 10.1039/c7ta07705j
Fosdick, S. E., Berglund, S. P., Mullins, C. B., and Crooks, R. M. (2014). Evaluating electrocatalysts for the hydrogen evolution reaction using bipolar electrode arrays: Bi- and trimetallic combinations of Co, Fe, Ni, Mo, and W. ACS Catal. 4, 1332–1339. doi: 10.1021/cs500168t
Haque, F., Zavabeti, A., Zhang, B. Y., Datta, R. S., Yin, Y., Yi, Z., et al. (2019). Ordered intracrystalline pores in planar molybdenum oxide for enhanced alkaline hydrogen evolution. J. Mater. Chem. A 7, 257–268. doi: 10.1039/c8ta08330d
Jeon, T. H., Kim, H., Kim, H.-I., and Choi, W. (2020). Highly durable photoelectrochemical H2O2 production via dual photoanode and cathode processes under solar simulating and external bias-free conditions. Energy Environ. Sci. 13, 1730–1742. doi: 10.1039/c9ee03154e
Jiao, Y., Zheng, Y., Jaroniec, M., and Qiao, S. Z. (2015). Design of electrocatalysts for oxygen- and hydrogen-involving energy conversion reactions. Chem. Soc. Rev. 44, 2060–2086. doi: 10.1039/c4cs00470a
Khattak, G. D., Salim, M. A., A1-Harthi, A. S., Thompson, D. J., and Wenger, L. E. (1997). Structure of molybdenum-phosphate glasses by X-ray photoelectron spectroscopy (XPS). J. Non-Cryst. Solids 212, 180–191. doi: 10.1016/S0022-3093(97)00023-9
Kumar, V., Wang, X., and Lee, P. S. (2015). Formation of hexagonal-molybdenum trioxide (h-MoO3) nanostructures and their pseudocapacitive behavior. Nanoscale 7, 11777–11786. doi: 10.1039/c5nr01505g
Li, Y., Cai, L., Huang, Q., Liu, J., Tang, R., and Zhou, W. (2020). Highly efficient synthesis of carbon-based molybdenum phosphide nanoparticles for electrocatalytic hydrogen evolution. Nanoscale Res. Lett. 15, 1–7. doi: 10.1186/s11671-020-3246-x
Li, Y., Hu, Y., Shen, J., Jiang, H., Min, G., Qiu, S., et al. (2015). Rapid flame synthesis of internal Mo6+ doped TiO2 nanocrystals in situ decorated with highly dispersed MoO3 clusters for lithium ion storage. Nanoscale 7, 18603–18611. doi: 10.1039/c5nr05586e
Li, Z.-P., Shang, J.-P., Su, C.-N., Zhang, S.-B., Wu, M.-X., and Guo, Y. (2018). Preparation of amorphous NiP-based catalysts for hydrogen evolution reactions. J. Fuel Chem. Technol. 46, 473–478. doi: 10.1016/s1872-5813(18)30021-5
Lian, S., Sun, C., Xu, W., Huo, W., Luo, Y., Zhao, K., et al. (2019). Built-in oriented electric field facilitating durable Zn MnO2 battery. Nano Energy 62, 79–84. doi: 10.1016/j.nanoen.2019.04.038
Liu, X., Jiang, Y., Li, Y., Wang, Z., Li, J., Huo, H., et al. (2019). MoP4 Nanoparticles as a novel and efficient cocatalyst for enhanced photocatalytic hydrogen evolution. ChemCatChem 11, 6244–6251. doi: 10.1002/cctc.201901476
Luo, Z., Miao, R., Huan, T. D., Mosa, I. M., Poyraz, A. S., Zhong, W., et al. (2016). Mesoporous MoO3−x material as an efficient electrocatalyst for hydrogen evolution reactions. Adv. Energy Mater. 6, 1–11. doi: 10.1002/aenm.201600528
Mahmood, N., Yao, Y., Zhang, J. W., Pan, L., Zhang, X., and Zou, J. J. (2018). Electrocatalysts for hydrogen evolution in alkaline electrolytes: mechanisms, challenges, and prospective solutions. Adv Sci. 5, 1–23. doi: 10.1002/advs.201700464
Mhtensson, N., Nilsson, A., and On the origin of core-level binding energy shifts. (1995). J. Electron. Spectrosc. Relat. Phenom. 75, 209–223. doi: 10.1016/0368-2048(95)02532-4
Mohiuddin, M., Zavabeti, A., Haque, F., Mahmood, A., Datta, R. S., Syed, N., et al. (2020). Synthesis of two-dimensional hematite and iron phosphide for hydrogen evolution. J. Mater. Chem. A 8, 2789–2797. doi: 10.1039/c9ta11945k
Rai, A., Movva, H., Roy, A., Taneja, D., Chowdhury, S., and Banerjee, S. (2018). Progress in contact, doping and mobility engineering of MoS2: an atomically thin 2D semiconductor. Crystals 8, 1–84. doi: 10.3390/cryst8080316
Ren, G., Zhang, B. Y., Yao, Q., Zavabeti, A., Huertas, C. S., Brkljača, R., et al. (2019). An Ultrasensitive silicon photonic ion sensor enabled by 2D plasmonic molybdenum oxide. Small 15, 1–10. doi: 10.1002/smll.201805251
Ren, Q., Jin, H., Xu, X., Liu, A., Li, J., Wang, J., et al. (2019). Hydrogen evolution reaction catalyzed by nickel/nickel phosphide nanospheres synthesized through electrochemical methods. Electrochimica Acta 298, 229–236. doi: 10.1016/j.electacta.2018.12.087
Si, W., Lei, W., Hao, Q., Xia, X., Zhang, H., Li, J., et al. (2016). Facile synthesis of nitrogen-doped graphene derived from graphene oxide and vitamin B3 as high-performance sensor for imidacloprid determination. Electrochim. Acta 212, 784–790. doi: 10.1016/j.electacta.2016.07.063
Sim, Y., Kim, S. J., Janani, G., Chae, Y., Surendran, S., Kim, H., et al. (2020). The synergistic effect of nitrogen and fluorine co-doping in graphene quantum dot catalysts for full water splitting and supercapacitor. Appl. Surf. Sci. 507:145157. doi: 10.1016/j.apsusc.2019.145157
Subbaraman, R., Tripkovic, D., Strmcnik, D., Chang, K. C., Uchimura, M., Paulikas, A. P., et al. (2011). Enhancing hydrogen evolution activity in water splitting by tailoring Li+-Ni(OH)2-Pt interfaces. Science 334, 1256–1260. doi: 10.1126/science.1211934
Surendran, S., Shanmugapriya, S., Lee, Y. S., Sim, U., and Selvan, R. K. (2018). Carbon-enriched cobalt phosphide with assorted nanostructure as a multifunctional electrode for energy conversion and storage devices. Chem. Select 3, 12303–12313. doi: 10.1002/slct.201802709
Surendran, S., Shanmugapriya, S., Ramasamy, H., Janani, G., Kalpana, D., Lee, Y. S., et al. (2019a). Hydrothermal deposition of CoS nanostructures and its multifunctional applications in supercapattery and water electrolyzer. Appl. Surf. Sci. 494, 916–928. doi: 10.1016/j.apsusc.2019.07.162
Surendran, S., Sivanantham, A., Shanmugam, S., Sim, U., and Kalai Selvan, R. (2019b). Ni2P2O7 microsheets as efficient Bi-functional electrocatalysts for water splitting application. Sustain. Energy Fuels 3, 2435–2446. doi: 10.1039/c9se00265k
Turner, J. A. (2004). Sustainable hyrogen production. Science 305, 972–974. doi: 10.1126/science.1103197
Wang, D., Duan, Q., and Wu, Z. (2019). Facile synthesis of MoP/MoO2 heterostructures for efficient hydrogen generation. Mater. Lett. 241, 227–230. doi: 10.1016/j.matlet.2019.01.095
Wang, L., Dong, B., Xu, X., and Wang, Y. (2019). Molybdophosphate derived MoP based electrocatalyst as cathode for Sn–H+ battery to generate H2 and electricity simultaneously. J. Solid State Chem. 277, 602–610. doi: 10.1016/j.jssc.2019.07.01
Wen, S., Yang, T., Zhao, N., Ma, L., and Liu, E. (2019). Ni-Co-Mo-O nanosheets decorated with NiCo nanoparticles as advanced electrocatalysts for highly efficient hydrogen evolution. Appl. Catal. B Environ. 258, 1–7. doi: 10.1016/j.apcatb.2019.117953
Xiao, P., Sk, M. A., Thia, L., Ge, X., Lim, R. J., Wang, J.-Y., et al. (2014). Molybdenum phosphide as an efficient electrocatalyst for the hydrogen evolution reaction. Energy Environ. Sci. 7, 2624–2629. doi: 10.1039/c4ee00957f
Xing, Z., Liu, Q., Asiri, A. M., and Sun, X. (2014). Closely interconnected network of molybdenum phosphide nanoparticles: a highly efficient electrocatalyst for generating hydrogen from water. Adv. Mater. 26, 5702–5707. doi: 10.1002/adma.201401692
Yao, Y., Mahmood, N., Pan, L., Shen, G., Zhang, R., Gao, R., et al. (2018). Iron phosphide encapsulated in P-doped graphitic carbon as efficient and stable electrocatalyst for hydrogen and oxygen evolution reactions. Nanoscale 10, 21327–21334. doi: 10.1039/c8nr06752j
Yuan, W., Feng, Y., Xie, A., Zhang, X., Huang, F., Li, S., et al. (2016). Nitrogen-doped nanoporous carbon derived from waste pomelo peel as a metal-free electrocatalyst for the oxygen reduction reaction. Nanoscale 8, 8704–8711. doi: 10.1039/c6nr00764c
Zhang, B. Y., Zavabeti, A., Chrimes, A. F., Haque, F., O'Dell, L. A., Khan, H., et al. (2018). Degenerately hydrogen doped molybdenum oxide nanodisks for ultrasensitive plasmonic biosensing. Adv. Funct. Mater. 28, 1–13. doi: 10.1002/adfm.201706006
Zhang, J., Wong, H., Yu, D., Kakushima, K., and Iwai, H. (2014). X-ray photoelectron spectroscopy study of high-k CeO2/La2O3 stacked dielectrics. AIP Adv. 4, 117117–117119. doi: 10.1063/1.4902017
Zhang, L. N., Li, S. H., Tan, H. Q., Khan, S. U., Ma, Y. Y., Zang, H. Y., et al. (2017). MoP/Mo2C@C: a new combination of electrocatalysts for highly efficient hydrogen evolution over the entire pH range. ACS Appl. Mater. Interfaces 9, 16270–16279. doi: 10.1021/acsami.7b03823
Zhao, K., Sun, C., Yu, Y., Dong, Y., Zhang, C., Wang, C., et al. (2018). Surface gradient Ti-doped MnO2 nanowires for high-rate and long-life lithium battery. ACS Appl. Mater. Interfaces 10, 44376–44384. doi: 10.1021/acsami.8b13376
Zhou, Z., Mahmood, N., Zhang, Y., Pan, L., Wang, L., Zhang, X., et al. (2017). CoP nanoparticles embedded in P and N co-doped carbon as efficient bifunctional electrocatalyst for water splitting. J. Energy Chem. 26, 1223–1230. doi: 10.1016/j.jechem.2017.07.021
Zou, W., Sun, C., Zhao, K., Li, J., Pan, X., Ye, D., et al. (2020). Surface reconstruction of NiCoP pre-catalysts for bifunctional water splitting in alkaline electrolyte. Electrochim. Acta 345:136114. doi: 10.1016/j.electacta.2020.136114
Keywords: molybdenum phosphide, hydrogen evolution reaction, alkaline electrolyte, water-splitting, catalysts
Citation: Khan MW, Loomba S, Ali R, Mohiuddin M, Alluqmani A, Haque F, Liu Y, Sagar RUR, Zavabeti A, Alkathiri T, Shabbir B, Jian X, Ou JZ, Mahmood A and Mahmood N (2020) Nitrogen-Doped Oxygenated Molybdenum Phosphide as an Efficient Electrocatalyst for Hydrogen Evolution in Alkaline Media. Front. Chem. 8:733. doi: 10.3389/fchem.2020.00733
Received: 04 May 2020; Accepted: 15 July 2020;
Published: 28 August 2020.
Edited by:
Syed Mubeen Jawahar Hussaini, The University of Iowa, United StatesReviewed by:
Kangning Zhao, Shanghai University, ChinaUk Sim, Chonnam National University, South Korea
Copyright © 2020 Khan, Loomba, Ali, Mohiuddin, Alluqmani, Haque, Liu, Sagar, Zavabeti, Alkathiri, Shabbir, Jian, Ou, Mahmood and Mahmood. This is an open-access article distributed under the terms of the Creative Commons Attribution License (CC BY). The use, distribution or reproduction in other forums is permitted, provided the original author(s) and the copyright owner(s) are credited and that the original publication in this journal is cited, in accordance with accepted academic practice. No use, distribution or reproduction is permitted which does not comply with these terms.
*Correspondence: Jian Zhen Ou, amlhbnpoZW4ub3UmI3gwMDA0MDtybWl0LmVkdS5hdQ==; Asif Mahmood, YXNpZi5tYWhtb29kJiN4MDAwNDA7c3lkbmV5LmVkdS5hdQ==; Nasir Mahmood, bmFzaXIubWFobW9vZCYjeDAwMDQwO3JtaXQuZWR1LmF1
†These authors have contributed equally to this work