- 1School of Science, China University of Geosciences, Beijing, China
- 2Key Laboratory of Optic-electric Sensing and Analytical Chemistry for Life Science, MOE, Qingdao University of Science and Technology, Qingdao, China
In this work, cerium-doped LaF3 nanoparticles (LaF3:Ce NPs) were successfully synthesized and characterized. Its chemiluminescence (CL) property was studied, and it was amazingly found that it intensely enhanced the ultra-weak CL of the NaNO2-H2O2 system. The CL mechanism was systematically investigated and suggested to be the recombination of electron-injected and hole-injected LaF3:Ce NPs. The new CL system was developed to be a facile, original, and direct method for nitrite analysis. Experimental conditions were optimized and then a satisfactory linear relationship between CL intensity and nitrite concentration was obtained. This work introduced a new pathway for the research and application of traditional fluoride NPs doped with RE3+.
Introduction
Fluoride is utilized as an ideal and appealing host for phosphors doped with rare earth ions (RE3+) owing to its adequate thermal and environmental stability as well as large solubility for RE3+ ions (Li et al., 2012). Compared with oxide systems, vibrational energies in fluorides is low and therefore trigger scarce quenching of the excited states of the RE3+ ions (Bender et al., 2000). Furthermore, RE3+-doped fluorides exhibit characteristic properties, such as high ionicity, low refractive index, wide band gap, and low phonon energy. KMgF3 (Schuyt and Williams, 2018), NaYF4 (Wu et al., 2019, 2020), NaGdF4 (Yi et al., 2019), and LaF3 (Bekah et al., 2016; Nampoothiri et al., 2017) have been investigated and exhibit high quantum yields and long luminescent lifetimes. RE3+-doped fluorides have been attracting attentions for several years due to the wide variety of technological applications including biomedical researches (All et al., 2019; Yan et al., 2019), biosensors (Vijayan et al., 2019), bioimaging (Hu et al., 2016; Han et al., 2017; Zeng et al., 2019), radiation detection (Ju et al., 2017), optoelectronic devices (Wu et al., 2018), and so on. However, to the best of our knowledge, the performance of RE3+-doped fluorides toward chemiluminescence (CL) has not been explored.
Nitrite is widely used in food manufacture as preservatives and fertilizing reagents. As an essential precursor of carcinogenic N-nitrosamine, excess intake of nitrite is harmful for human beings. Nitrite can cause irreversible conversion of hemoglobin to methemoglobin in the bloodstream and then bring detrimental effect for the oxygen transport in the whole body. In addition, nitrogen-based fertilizers and industrial wastewater pollute groundwater resources by nitrites. Thus, nitrite detection is of significant importance for food safety, public health, and environment protection (Wang et al., 2017; Zhang Y. et al., 2018; Cao et al., 2019). Various principle-based analytical methods have been devised for nitrite detection, such as electrochemical sensors (Ma et al., 2018; Wang et al., 2018; Zhou et al., 2019; Madhuvilakku et al., 2020), microplasma emission (Zheng et al., 2018), absorption spectrophotometry (Zhang L. et al., 2018), fluorescence (Dai et al., 2017; Jana et al., 2019; Pires et al., 2019), and CL (Lu et al., 2002, 2004; Lin et al., 2011; Wu et al., 2016). Electrodes are modified with complex strategies in electrochemical analysis. Special molecules need to be designed for spectrophotometric detections in order to amplify signal and reduce the background interferences. CL detections require simple instruments, interfere with low background, and are compatible with gas or aqueous phases. CL intensity was reported to be significantly enhanced by nanomaterials that gave promise for developing sensitive and convenient CL analytical methods. In 2011, carbon dots were firstly demonstrated to enhance the CL signal of the NaNO2-H2O2 system because of peroxynitrous acid generation (Lin et al., 2011). Nitrogen-rich quantum dots (QDs) were facilely synthesized and intensely enhanced the ultra-weak CL reaction of the NaIO4-H2O2 system through electron hole injection and CL resonance energy transfer (Zheng et al., 2017). In particular, molybdenum sulfide QDs were proved to give rise to the generation of reactive oxygen species from hydrogen peroxide (H2O2) in alkaline solution and gave a promise for CL emission (Dou et al., 2019). However, fluoride-based nanomaterials were scarcely utilized and the developed CL analysis was rarely applied in nitrite detection. Original CL detections for nitrites are worth giving research to pursue better performance.
In this work, cerium-doped LaF3 nanoparticles (LaF3:Ce NPs) were synthesized and firstly demonstrated to enhance the CL signal of the NaNO2-H2O2 system. Reactive oxygen species generation that was triggered by LaF3:Ce NPs was proved to be the main reason for CL enhancement. A linear relationship between the CL signal and nitrite concentration was found and implied that the LaF3:Ce NPs-NaNO2-H2O2 system could be applied in the determination of nitrite.
Materials and Methods
Reagents and Materials
Sodium nitrite (NaNO2) was purchased from Sinopharm Chemical Reagent Co., Ltd. (Shanghai, China). Sulfuric acid (H2SO4, 98%), H2O2 (35%), hydrochloric acid, and ethanol (98%) were brought from Beijing Chemical Reagent Co. (Beijing, China). Sodium fluoride (NaF, >98%), heptahydrate lanthanum chloride (LaCl3·7H2O, 99.9%), heptahydrate cerium chloride (CeCl3·7H2O, 99.9%), oleic acid (90%), 5,5-dimethyl-1-pyrroline N-oxide (DMPO), and ascorbic acid (AA) were all purchased from Sigma-Aldrich. Unless otherwise noted, all the chemicals were used without further purification.
Apparatus
UV-vis absorption spectra were performed on a PerkinElmer Lambda 950 spectrophotometer. The photoluminescent (PL) spectra were collected on an Agilent Cary Eclipse spectrofluorometer. Fourier transform infrared (FT-IR) spectra were obtained on a PerkinElmer Frontier FT-IR spectrometer. CL experiments were conducted with an ultra-weak CL analyzer (IFFM-E, Xi'an Remex Analytical Instrument Co., Ltd, China). Transmission electron microscopy images were obtained on a JEOL-1400 transmission electron microscope (JEOL, Tokyo, Japan). Electron paramagnetic resonance (EPR) spectra were measured on a Bruker E500 spectrometer.
LaF3:Ce NPs Synthesis
Hydrothermal reaction was utilized to synthesize LaF3:Ce NPs. 2.25 ml of LaCl3 solution (0.20 M), 1.00 ml of CeCl3 solution (0.05 M), 2.00 ml of NaF solution (1.00 M), 20 ml of ethanol, and 10 ml of oleic acid were mixed and stirred for 0.5 h in reaction kettle. The mixture was heated in an oven and kept at 200°C for 8 h. After reaction, the supernatant was removed. The remnant suspension was centrifuged at 6,000 rpm for 5 min and then the supernatant was also removed. The resultant solid was dispersed in 2.00 M hydrochloric acid. After ethanol addition, the mixture was centrifuged at 6,000 rpm for 5 min to remove the supernatant. The product was stored in 4 ml H2O for further use. The exact doping percentage of cerium was calculated to be 10%.
CL Study of the LaF3:Ce NPs-NaNO2-H2O2 System
At first, CL intensities of the NaNO2-H2O2 system with and without LaF3:Ce NPs were compared. Fifty microliters of H2O2 (3.00 M), which was acidified by 0.04 M H2SO4, was injected into the mixture of 50 μl of LaF3:Ce NPs and 50 μl of NaNO2 (10 μM). In the control experiment, 50 μl of LaF3:Ce NPs was replaced by 50 μl of H2O. CL intensities of both the two conditions were recorded and compared. CL profiles were integrated at intervals of 0.1 s. Voltage of the photomultiplier tube (PMT) was set at 1.2 kV. CL spectrum was measured with high-energy cutoff filters (400–640 nm), which were set between the quartz cuvette and PMT as described in Cui et al. (2003). Additional orders of the reagents were investigated to collect CL kinetic curves. EPR measurements were operated at an X-band frequency of 9.85 GHz. Irradiation was performed by using a 300-W Xe lamp (300 nm < λ < 1,100 nm) with the output radiation focused on the samples in the cavity by an optical fiber (50 cm length, 0.3 cm diameter). All spectra were acquired at 298 K. DMPO (12.4 μl in 1 ml of H2O) was taken as the specific detection reagent for ·OH. AA (0.1 mM) was used as a scavenger for . CL intensities of the LaF3:Ce NPs-NaNO2-H2O2 system with and without AA were recorded.
Nitrite Analysis
Experimental conditions were optimized with different H2SO4 concentrations (0, 0.02, 0.03, 0.04, 0.05, and 0.06 M), H2O2 concentrations (0.00, 1.00, 2.00, 3.00, 4.00, and 5.00 M), and additional volumes of LaF3:Ce NPs (0, 10, 20, 30, 40, 50, 60, and 70 μl). The univariate method was adopted in systematically optimizing experimental parameters through changing one parameter at a time while keeping others constant. At the optimal experimental conditions, calibration curve was recorded by detecting CL intensities vs. different nitrite concentrations.
Results
Characterization of LaF3:Ce NPs
LaF3:Ce NPs obtained in this work exhibited hexagonal phase and their average sizes were about 80 × 20 nm (Figure 1A). 4f shells of lanthanides are partially filled and are effectively shielded by outer 5s and 5p shells leading to satisfactory emissions. The prepared LaF3:Ce NPs gave a bright blue color under ultraviolet radiation (λex = 254 nm) (Figure 1B, inset). The emission of LaF3:Ce NPs shifted to longer wavelength with the increase of excitation wavelength revealing the distribution of different surface energy traps of the LaF3:Ce NPs (Figure 1B). UV-vis absorption spectra of the LaF3:Ce NPs-NaNO2-H2O2 system were collected and are shown in Figure 1C. NaNO2 gave an absorption peak at 354 nm, which decreased when acidified H2O2 was added. Another absorption peak located at 301 nm appeared due to the isomerization of ONOOH, which was generated in the mixture of acidified H2O2 and NaNO2 (Lin et al., 2011), while no new absorption peaks were found when acidified H2O2 mixed with LaF3:Ce NPs. Except the absorption peak of ONOOH, no other new absorption peak was found in the LaF3:Ce NPs-NaNO2-H2O2 system, indicating that no new compound was formed. UV-vis absorption spectra gave some indications for the CL mechanism of this system, which was illustrated in detail in the subsequent section. FT-IR spectrum of LaF3:Ce NPs indicated that there were O-H groups on the surface of LaF3:Ce NPs (Figure 1D).
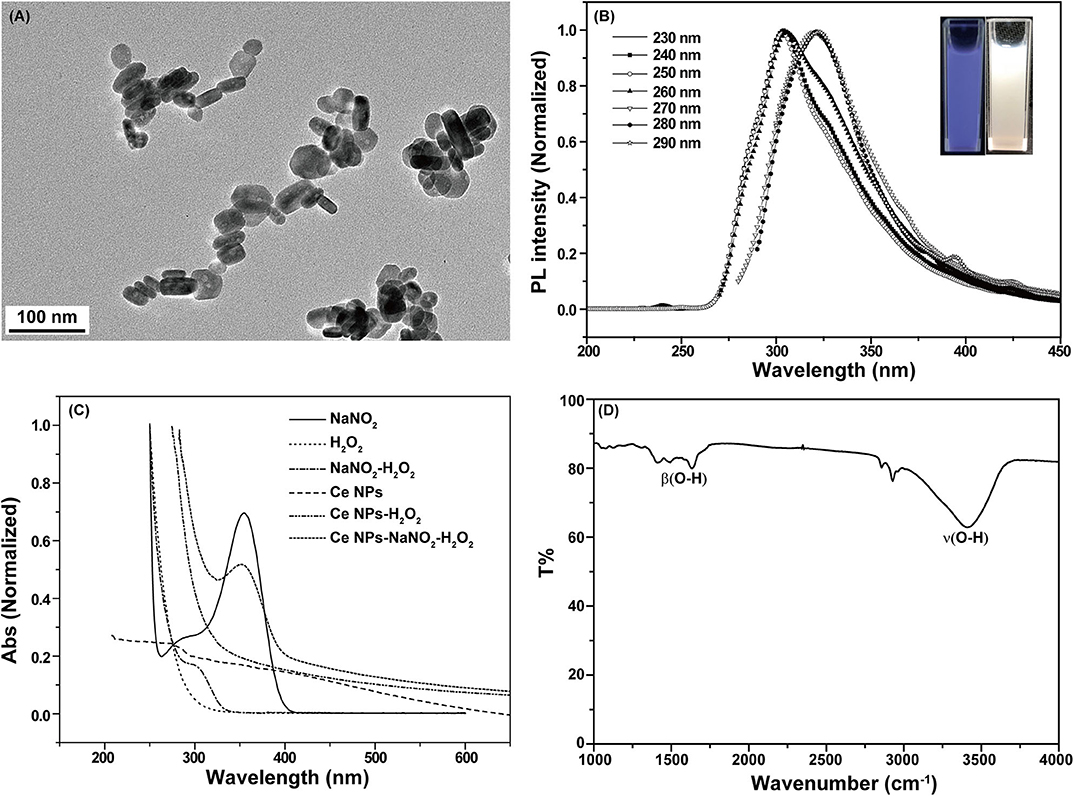
Figure 1. Characterization of LaF3:Ce NPs. (A) Transmission electron microscopy images of LaF3:Ce NPs. (B) PL spectra of LaF3:Ce NPs. The inset is the photograph of LaF3:Ce NPs under a UV lamp (λex = 254 nm). (C) UV-vis absorption spectra of the reagent in the CL reaction. (D) FT-IR spectra of LaF3:Ce NPs.
CL of the LaF3:Ce NPs-NaNO2-H2O2 System
CL intensities of the NaNO2-H2O2 system with and without LaF3:Ce NPs were sharply different. LaF3:Ce NPs addition intensely enhanced CL intensity (Figure 2A). As shown in Figure 2B, the CL spectrum for the LaF3:Ce NPs-NaNO2-H2O2 system was wide ranging from 375 to 500 nm and was centered at 450 nm. The fluorescent emission of LaF3:Ce NPs is also wide, which is similar to the CL spectrum of the LaF3:Ce NPs-NaNO2-H2O2 system. As a result, it is reasonable to refer that the CL originates from the various surface energy traps existing on the LaF3:Ce NPs. Compared with the PL peak of LaF3:Ce NPs, the CL spectrum is red-shifted due to the energy separations of LaF3:Ce NPs surface states. PL was generated through excitation and emission within the core of the LaF3:Ce NPs and the energy gap between them is larger than the energy separations on NPs surface (Ding et al., 2002; Myung et al., 2002).
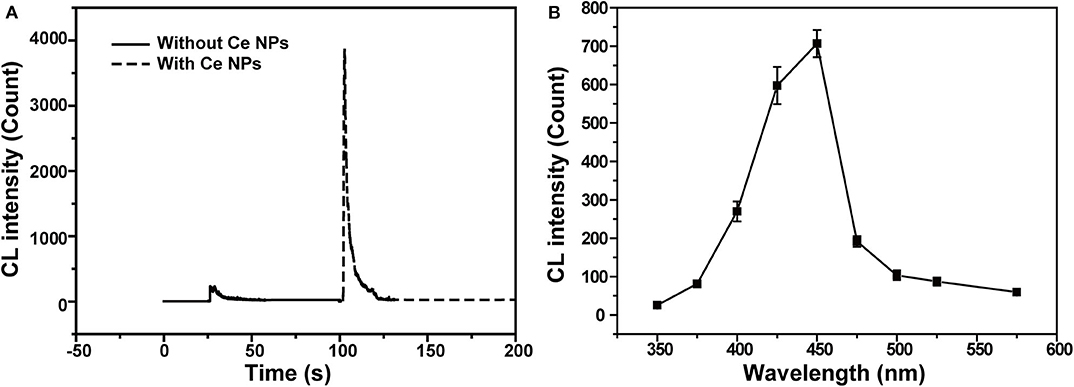
Figure 2. CL profiles of the LaF3:Ce NPs-NaNO2-H2O2 system. (A) Comparison of CL intensities of the NaNO2-H2O2 system with and without LaF3:Ce NPs. (B) CL spectrum of the LaF3:Ce NPs-NaNO2-H2O2 system. The standard error bars mean the variation of three individual experiments. Conditions: 3.00 M H2O2 in 0.04 M H2SO4, 50 μl of LaF3:Ce NPs and 10 μM NaNO2 solution. Voltage of the PMT was set at 1.2 kV.
CL Kinetic Study
As described in UV-vis absorption spectra, ONOOH was generated when NaNO2 was mixed with acidified H2O2 (Equation 1) (Anbar and Taube, 1954). ONOOH easily transforms to be nitrate via the stage of HOONO* and give emissions during the process (Equation 2) (Houk et al., 1996). The emission locates at 350–450 nm, which overlaps the absorption spectrum of LaF3:Ce NPs. Hence, LaF3:Ce NPs can be excited by the energy of transformation and cause CL emission. However, the maximum of the transformation-derived CL was obtained at the pH value of 6.5–7.0 while the maximum CL of the LaF3:Ce NPs-NaNO2-H2O2 system was recorded in a severe acidic solution (Starodubtseva et al., 1999). As a consequence, the transformation energy only partially contributed to the CL of the LaF3:Ce NPs-NaNO2-H2O2 system. Various mixing orders of reagents influenced the reactions between LaF3:Ce NPs and ONOOH and then affected the CL intensities (Figure 3A). The highest CL was obtained when acidified H2O2 was injected into the mixture of LaF3:Ce NPs and NaNO2. At this condition, the generated ONOOH adequately reacted with LaF3:Ce NPs and gave enhanced CL. Mixing of NaNO2 with acidified H2O2 without LaF3:Ce NPs gave weak and lasting CL while mixing of LaF3:Ce NPs with acidified H2O2 without NaNO2 gave weak and rapid CL (Figure 3B).
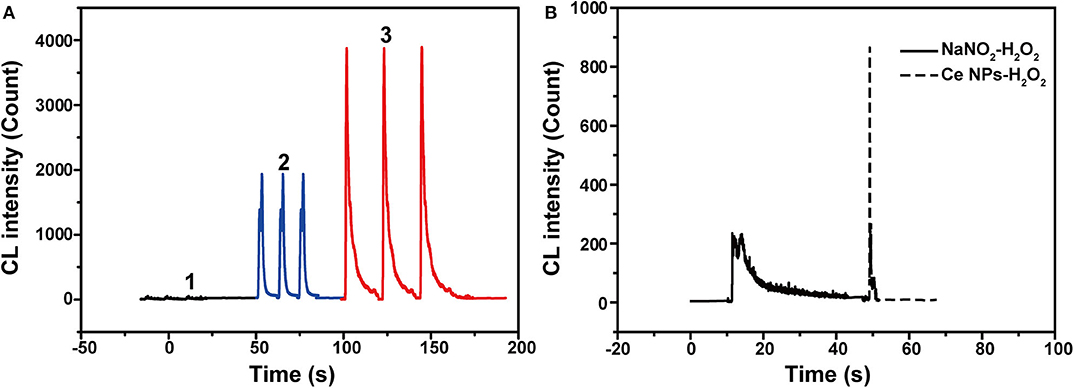
Figure 3. CL kinetic study of the LaF3:Ce NPs-NaNO2-H2O2 system. (A) CL kinetic curves of the LaF3:Ce NPs-NaNO2-H2O2 system with different reagent mixing orders: 1. injecting LaF3:Ce NPs into the mixture of NaNO2 and acidified H2O2; 2. injecting NaNO2 into the mixture of LaF3:Ce NPs and acidified H2O2; 3. injecting acidified H2O2 into the mixture of LaF3:Ce NPs and NaNO2. CL signals of three repeated experiments were given. (B) CL kinetic curves of the NaNO2-H2O2 system and the LaF3:Ce NPs-H2O2 system. Conditions: 3.00 M H2O2 in 0.04 M H2SO4, 50 μl of LaF3:Ce NPs and 10 μM NaNO2 solution. Voltage of the PMT was set at 1.2 kV.
CL Mechanism
According to the CL kinetic study, it demonstrated that the reactions between LaF3:Ce NPs and ONOOH or its related species were the main cause accounting for the enhanced CL. ONOOH was reported to be capable of producing reactive oxygen species (Equations 3–5) (Alvarez et al., 1995; Gunaydin and Houk, 2008; Lin et al., 2011). It was obvious that ONOOH-produced reactive oxygen species include ·OH, , and 1O2 in this system. EPR was performed and DMPO was utilized as the specific detection reagent for ·OH to directly examine the variation of ·OH after LaF3:Ce NPs addition. Although CL intensity of the LaF3:Ce NPs-NaNO2-H2O2 system was greatly enhanced, the production of ·OH was almost not increased (Figure 4A). 1O2 was derived from ·OH so it could refer that there was no increase in 1O2 quantity. Ethanol was reported to react with ·OH and yield an octet spectrum that was completely distinct from the DMPO-OH spectrum (Finkelstein et al., 1980). The octet spectrum in the LaF3:Ce NPs-NaNO2-H2O2 system rooted in the reaction between ·OH and residual ethanol from treatment process of LaF3:Ce NPs. Furthermore, AA, which was a scavenger for , obviously inhibited the CL of the LaF3:Ce NPs-NaNO2-H2O2 system (Figure 4B). All the results indicated that was the critical reason for the enhanced CL instead of ·OH and 1O2. acting as an electron donor reacted with LaF3:Ce NPs to produce LaF3:Ce NPs·− (Equation 6) (Poznyak et al., 2004). ONOOH serving as a hole injector converted LaF3:Ce NPs to LaF3:Ce NPs·+ (Equation 7). Electron–hole annihilation between LaF3:Ce NPs·− and LaF3:Ce NPs·+ resulted in CL emission (Equation 8) (Figure 5; Ding et al., 2002; Poznyak et al., 2004; Zheng et al., 2009; Dong et al., 2010).
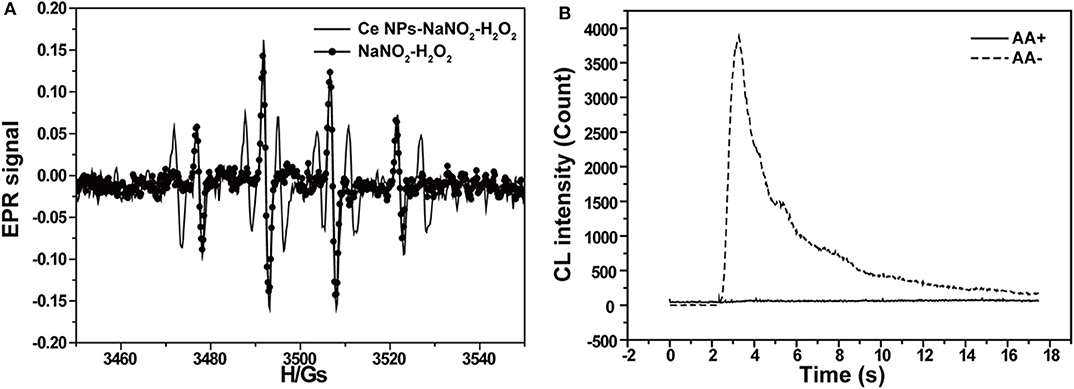
Figure 4. CL mechanism study of the LaF3:Ce NPs-NaNO2-H2O2 system. (A) EPR spectra of ·OH generated via the reaction of DMPO probe in the LaF3:Ce NPs-NaNO2-H2O2 and NaNO2-H2O2 systems. (B) CL profiles of the LaF3:Ce NPs-NaNO2-H2O2 system with and without AA. Conditions: 3.00 M H2O2 in 0.04 M H2SO4, 50 μl of LaF3:Ce NPs, 10 μM NaNO2 solution, 12.4 μl of DMPO in 1 ml of H2O, and 0.1 mM AA. Voltage of the PMT was set at 1.2 kV.
Nitrite Analysis
To establish the optimal conditions for nitrite analysis, the volume of LaF3:Ce NPs added into the CL system and concentrations of H2SO4 and H2O2 were investigated, respectively. As shown in Figure 6A, 50 μl of LaF3:Ce NPs was added into the CL system and provided the highest CL emission. Less LaF3:Ce NPs inadequately reacted with ONOOH while surplus LaF3:Ce NPs also consumed energy. Reactive substance ONOOH was the product of NaNO2 and H2O2 in acid medium, so H2SO4 was indispensable for the CL system. No CL signals could be observed in the absence of H2SO4. The most intense CL signal was obtained with the H2SO4 concentration of 0.04 M (Figure 6B). CL signal increased with the concentration of H2O2 in the range from 0 to 3.00 M (Figure 6C). Hence, the optimal analytical conditions for nitrite analysis were 3.00 M H2O2 in 0.04 M H2SO4 injected into the mixture of 50 μl of LaF3:Ce NPs and nitrite solution.
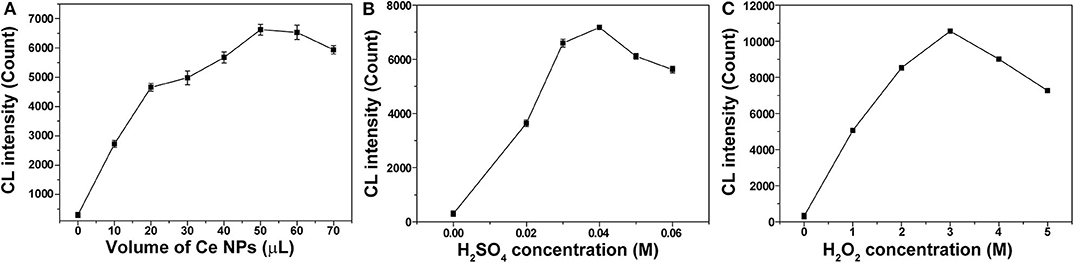
Figure 6. Optimization of experimental conditions for nitrite analysis. (A) Volume of LaF3:Ce NPs added into the CL system was optimized. (B) Concentration of H2SO4 used to acidify H2O2 was optimized. (C) Concentration of H2O2 was optimized. The standard error bars mean the variation of three individual experiments.
Under the optimal conditions, CL signals for different nitrite concentrations were recorded and shown in Figure 7. Good linear relationship between CL intensity and nitrite concentration was obtained in the range from 1 to 100 μM with a correlation coefficient of 0.9981 (y = 256.3x + 42.72). The relative standard deviation values of the analysis were 8.7, 1.2, and 4.8% for nitrite concentrations of 1, 10, and 100 μM, respectively. Relative standard deviation values demonstrated the satisfactory reproducibility. The limit of detection (S/N = 3) for nitrite was 0.33 μM.
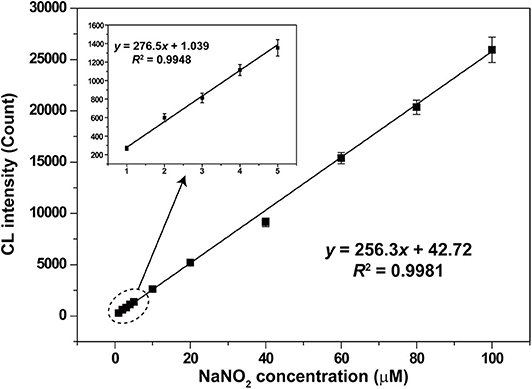
Figure 7. Calibration curve for nitrite analysis based on the LaF3:Ce NPs-enhanced CL. The standard error bars mean the variation of three individual experiments. Conditions: 3.00 M H2O2 in 0.04 M H2SO4 and 50 μl of LaF3:Ce NPs. Voltage of the PMT was set at 1.2 kV.
Discussion
The eternal goals and challenges of analytical chemistry are developing accurate, automated, selective, stable, sensitive, high-speed, high-throughput, and in situ analytical methods and protocols (Ju, 2013). The combination of analytical chemistry with new materials, especially nanomaterials, is the current frontier research topics and exhibits greatly improved analytical capacities. CL analysis is a traditional analytical technology and possesses outstanding advantages, such as low cost, simple instrument, fast response, and high compatibility. The application of nanomaterials in CL analysis leads to new CL sensing disciplines and offers a broad palette of opportunities for analytical chemists. In 2004, Poznyak et al. (2004) firstly reported the nanocrystal band gap CL derived from CdSe/CdS core-shell QDs that acted as a novel class of luminophores with the emission state originated from quantum-confined orbitals. Superior emission properties in QDs gave promises for developing QD-based nanoprobes for CL analysis. Besides traditional semiconductor QDs, some novel nanomaterials, such as carbon nanodots (Lin et al., 2011), graphene QDs (Hassanzadeh and Khataee, 2018), graphitic carbon nitride QDs (Zhu et al., 2019), and N-dots (Zheng et al., 2017), were developed to be potential platforms for CL sensing. These nanomaterials are superior in terms of robust chemical inertness, low toxicity, good aqueous solubility, high resistance to photobleaching, and satisfactory biocompatibility. Our work is an endeavor step during the development process of nanomaterial-sensitized CL analysis methods. In this study, LaF3:Ce NPs were successfully synthesized and applied in nitrite detection based on CL signals. The synthetic process of LaF3:Ce NPs was simple and the products were fully characterized to give indications for the CL mechanism study. The enhancement of LaF3:Ce NPs for the NaNO2-H2O2 CL system was efficient and the mechanism was systematically and scientifically explained. The linear relationship between CL intensity and nitrite concentration was found, although there were spaces for improving the limit of detection. This work tried to explore new CL nanoprobes and gave a new route for fluoride applications. In the future, there is still a great demand for developing novel CL nanoprobes especially metal-free QDs and two-dimensional QDs.
Conclusions
In summary, LaF3:Ce NPs were successfully synthesized and demonstrated to intensely enhance ultra-weak CL of the NaNO2-H2O2 system. The CL mechanism was suggested to be the electron–hole annihilation between hole-injected and electron-injected LaF3:Ce NPs. The new CL system was developed to be a novel, simple, and straightforward analytical method for nitrite. All the experimental conditions were optimized and a satisfactory linear relationship between CL intensity and nitrite concentration was obtained. This work shed a new light on the research and application of traditional fluoride NPs doped with RE3+.
Data Availability Statement
The raw data supporting the conclusions of this article will be made available by the authors, without undue reservation, to any qualified researcher.
Author Contributions
YuW organized and conducted all the experiments, analyzed data, and wrote the manuscript. JW coordinated the project, supervised all the experiments, analyzed data, and wrote, edited, and reviewed the manuscript. YaW assisted the experiments of nitrite analysis. CH synthesized and characterized LaF3:Ce NPs. TC performed experiments of the CL study of the LaF3:Ce NPs-NaNO2-H2O2 system. All authors contributed to the article and approved the submitted version.
Funding
This work was supported by the National Natural Science Foundation of China (No. 21874120) and the Fundamental Research Funds for the Central Universities (No. 2652018004). During the revision process, support was supplied by the Fundamental Research Funds for the Central Universities (No. 2652019112) and the open fund of Key Laboratory of Optic-electric Sensing and Analytical Chemistry for Life Science, MOE, Qingdao University of Science and Technology (No. OESACLS202004).
Conflict of Interest
The authors declare that the research was conducted in the absence of any commercial or financial relationships that could be construed as a potential conflict of interest.
Acknowledgments
Ms. Mengnan Rao is acknowledged for conducting some preparation works.
Abbreviations
AA, ascorbic acid; LaF3:Ce NPs, cerium-doped LaF3 nanoparticles; CL, chemiluminescence; EPR, electron paramagnetic resonance; FT-IR, Fourier transform infrared; PL, photoluminescent; PMT, photomultiplier tube; QDs, quantum dots; RE3+, rare earth ions.
References
All, A. H., Zeng, X., Teh, D. B. L., Yi, Z., Prasad, A., and Ishizuka, T., et al. (2019). Expanding the toolbox of upconversion nanoparticles for in vivo optogenetics and neuromodulation. Adv. Mater. 31:1803474. doi: 10.1002/adma.201803474
Alvarez, B., Denicola, A., and Radi, R. (1995). Reaction between peroxynitrite and hydrogen peroxide: formation of oxygen and slowing of peroxynitrite decomposition. Chem. Res. Toxicol. 8, 859–864. doi: 10.1021/tx00048a006
Anbar, M., and Taube, H. (1954). Interaction of nitrous acid with hydrogen peroxide and with water. J. Am. Chem. Soc. 76, 6243–6247. doi: 10.1021/ja01653a007
Bekah, D., Cooper, D., Kudinov, K., Hill, C., Seuntjens, J., and Bradforth, S., et al. (2016). Synthesis and characterization of biologically stable, doped LaF3 nanoparticles co-conjugated to PEG and photosensitizers. J. Photochem. Photobiol. A Chem. 329, 26–34. doi: 10.1016/j.jphotochem.2016.06.008
Bender, C. M., Burlitch, J. M., Barber, D., and Pollock, C. (2000). Synthesis and fluorescence of neodymium-doped barium fluoride nanoparticles. Chem. Mater. 12, 1969–1976. doi: 10.1021/cm9904741
Cao, R., Huang, H., Liang, J., Wang, T., Luo, Y., Asiri, A. M., et al. (2019). A MoN nanosheet array supported on carbon cloth as an efficient electrochemical sensor for nitrite detection. Analyst 144, 5378–5380. doi: 10.1039/C9AN01270B
Cui, H., Zou, G., and Lin, X. (2003). Electrochemiluminescence of luminol in alkaline solution at a paraffin-impregnated graphite electrode. Anal. Chem. 75, 324–331. doi: 10.1021/ac0201631
Dai, C., Wang, J., Fu, Y., Zhou, H., and Song, Q. (2017). Selective and real-time detection of nitric oxide by a two-photon fluorescent probe in live cells and tissue slices. Anal. Chem. 89, 10511–10519. doi: 10.1021/acs.analchem.7b02680
Ding, Z., Quinn, B. M., Haram, S. K., Pell, L. E., Korgel, B. A., and Bard, A. J. (2002). Electrochemistry and electrogenerated chemiluminescence from silicon nanocrystal quantum dots. Science 296, 1293–1297. doi: 10.1126/science.1069336
Dong, Y., Zhou, N., Lin, X., Lin, J., Chi, Y., and Chen, G. (2010). Extraction of electrochemiluminescent oxidized carbon quantum dots from activated carbon. Chem Mater. 22, 5895–5899. doi: 10.1021/cm1018844
Dou, X., Zhang, Q., Shah, S. N. A., Khan, M., Uchiyama, K., and Lin, J. (2019). MoS2-quantum dot triggered reactive oxygen species generation and depletion, responsible for enhanced chemiluminescence. Chem. Sci. 10, 497–500. doi: 10.1039/C8SC03511C
Finkelstein, E., Rosen, G. M., and Rauckman, E. J. (1980). Spin trapping kinetics of the reaction of superoxide and hydroxyl radicals with nitrones. J. Am. Chem. Soc. 102, 4994–4999. doi: 10.1021/ja00535a029
Gunaydin, H., and Houk, K. N. (2008). Molecular dynamics simulation of the HOONO decomposition and the HO•/NO2• caged radical pair in water. J. Am. Chem. Soc. 130, 10036–10037. doi: 10.1021/ja711365e
Han, S., Samanta, A., Xie, X., Huang, L., Peng, J., and Park, S. J., et al. (2017). Gold and hairpin DNA functionalization of upconversion nanocrystals for imaging and in vivo drug delivery. Adv. Mater. 29:1700244. doi: 10.1002/adma.201700244
Hassanzadeh, J., and Khataee, A. (2018). Ultrasensitive chemiluminescent biosensor for the detection of cholesterol based on synergetic peroxidase-like activity of MoS2 and graphene quantum dots. Talanta 178, 992–1000. doi: 10.1016/j.talanta.2017.08.107
Houk, K. N., Condroski, K. R., and Pryor, W. A. (1996). Radical and concerted mechanisms in oxidations of amines, sulfides, and alkenes by peroxynitrite, peroxynitrous acid, and the peroxynitrite–CO2 adduct: density functional theory transition structures and energetics. J. Am. Chem. Soc. 118, 13002–13006. doi: 10.1021/ja9619521
Hu, Y., Wu, B., Jin, Q., Wang, X., Li, Y., Sun, Y., et al. (2016). Facile synthesis of 5 nm NaYF4:Yb/Er nanoparticles for targeted upconversion imaging of cancer cells. Talanta 152, 504–512. doi: 10.1016/j.talanta.2016.02.039
Jana, J., Lee, H. J., Chung, J. S., Kim, M. H., and Hur, S. H. (2019). Blue emitting nitrogen-doped carbon dots as a fluorescent probe for nitrite ion sensing and cell-imaging. Anal. Chim. Acta 1079, 212–219. doi: 10.1016/j.aca.2019.06.064
Ju, H. (2013). Grand challenges in analytical chemistry, towards more bright eyes for scientific research, social events and human health. Front. Chem. 1:5. doi: 10.3389/fchem.2013.00005
Ju, J., Won, H., Jung, J., Yeo, J., Van Cuong, P., and Kim, D. (2017). Enhanced X-ray excited luminescence of LaF3:Ce/CdSeS nanocomposites by resonance energy transfer for radiation detection. J. Electron. Mater. 46, 5319–5323. doi: 10.1007/s11664-017-5548-z
Li, S., Zhang, X., Hou, Z., Cheng, Z., Ma, P., and Lin, J. (2012). Enhanced emission of ultra-small-sized LaF3:RE3+ (RE = Eu, Tb) nanoparticles through 1,2,4,5-benzenetetracarboxylic acid sensitization. Nanoscale 4, 5619–5626. doi: 10.1039/c2nr31206a
Lin, Z., Xue, W., Chen, H., and Lin, J. (2011). Peroxynitrous-acid-induced chemiluminescence of fluorescent carbon dots for nitrite sensing. Anal. Chem. 83, 8245–8251. doi: 10.1021/ac202039h
Lu, C., Lin, J., Huie, C. W., and Yamada, M. (2004). Chemiluminescence study of carbonate and peroxynitrous acid and its application to the direct determination of nitrite based on solid surface enhancement. Anal. Chim. Acta 510, 29–34. doi: 10.1016/j.aca.2003.12.057
Lu, C., Qu, F., Lin, J., and Yamada, M. (2002). Flow-injection chemiluminescent determination of nitrite in water based on the formation of peroxynitrite from the reaction of nitrite and hydrogen peroxide. Anal. Chim. Acta 474, 107–114. doi: 10.1016/S0003-2670(02)01010-3
Ma, Y., Wang, Y., Xie, D., Gu, Y., Zhang, H., and Wang, G., et al. (2018). NiFe-Layered double hydroxide nanosheet arrays supported on carbon cloth for highly sensitive detection of nitrite. ACS Appl. Mater. Inter. 10, 6541–6551. doi: 10.1021/acsami.7b16536
Madhuvilakku, R., Alagar, S., Mariappan, R., and Piraman, S. (2020). Glassy carbon electrodes modified with reduced graphene oxide-MoS2-poly (3, 4-ethylene dioxythiophene) nanocomposites for the non-enzymatic detection of nitrite in water and milk. Anal. Chim. Acta 1093, 93–105. doi: 10.1016/j.aca.2019.09.043
Myung, N., Ding, Z., and Bard, A. J. (2002). Electrogenerated chemiluminescence of CdSe nanocrystals. Nano Lett. 2, 1315–1319. doi: 10.1021/nl0257824
Nampoothiri, P. K., Gandhi, M. N., and Kulkarni, A. R. (2017). Elucidating the stabilizing effect of oleic acid coated LaF3: Nd3+ nanoparticle surface in the thermal degradation of PMMA nanocomposites. Mater. Chem. Phys. 190, 45–52. doi: 10.1016/j.matchemphys.2016.12.075
Pires, N. M. M., Dong, T., and Yang, Z. (2019). A fluorimetric nitrite biosensor with polythienothiophene-fullerene thin film detectors for on-site water monitoring. Analyst 144, 4342–4350. doi: 10.1039/C8AN02441C
Poznyak, S. K., Talapin, D. V., Shevchenko, E. V., and Weller, H. (2004). Quantum dot chemiluminescence. Nano Lett. 4, 693–698. doi: 10.1021/nl049713w
Schuyt, J. J., and Williams, G. V. M. (2018). Photoluminescence, radioluminescence and optically stimulated luminescence in nanoparticle and bulk KMgF3(Eu). J. Lumin. 204, 472–479. doi: 10.1016/j.jlumin.2018.08.056
Starodubtseva, M. N., Cherenkevich, S. N., and Semenkova, G. N. (1999). Investigation of the interaction of sodium nitrite with hydrogen peroxide in aqueous solutions by the chemiluminescence method. J. Appl. Spectrosc. 66, 473–476. doi: 10.1007/BF02676785
Vijayan, A. N., Liu, Z., Zhao, H., and Zhang, P. (2019). Nicking enzyme-assisted signal-amplifiable Hg2+ detection using upconversion nanoparticles. Anal. Chim. Acta 1072, 75–80. doi: 10.1016/j.aca.2019.05.001
Wang, Q., Yu, L., Liu, Y., Lin, L., Lu, R., Zhu, J., et al. (2017). Methods for the detection and determination of nitrite and nitrate: A review. Talanta 165, 709–720. doi: 10.1016/j.talanta.2016.12.044
Wang, R., Wang, Z., Xiang, X., Zhang, R., Shi, X., and Sun, X. (2018). MnO2 nanoarrays: an efficient catalyst electrode for nitrite electroreduction toward sensing and NH3 synthesis applications. Chem. Commun. 54, 10340–10342. doi: 10.1039/C8CC05837G
Wu, J., Wang, X., Lin, Y., Zheng, Y., and Lin, J. (2016). Peroxynitrous-acid-induced chemiluminescence detection of nitrite based on Microfluidic chip. Talanta 154, 73–79. doi: 10.1016/j.talanta.2016.03.062
Wu, J., Yang, Z., Qiu, C., Zhang, Y., Wu, Z., Yang, J., et al. (2018). Enhanced performance of a graphene/GaAs self-driven near-infrared photodetector with upconversion nanoparticles. Nanoscale 10, 8023–8030. doi: 10.1039/C8NR00594J
Wu, N., Gu, Y., Kong, M., Liu, Q., Cheng, S., Yang, Y., et al. (2020). Yb-based nanoparticles with the same excitation and emission wavelength for sensitive in vivo biodetection. Anal. Chem. 92, 2027–2033. doi: 10.1021/acs.analchem.9b04448
Wu, Y., Xu, J., Poh, E. T., Liang, L., Liu, H., Yang, J. K. W., et al. (2019). Upconversion superburst with sub-2 μs lifetime. Nat. Nanotechnol. 14, 1110–1115. doi: 10.1038/s41565-019-0560-5
Yan, S., Zeng, X., Tang, Y., Liu, B., Wang, Y., and Liu, X. (2019). Activating antitumor immunity and antimetastatic effect through polydopamine-encapsulated core-shell upconversion nanoparticles. Adv. Mater. 31:1905825. doi: 10.1002/adma.201905825
Yi, Z., Luo, Z., Barth, N. D., Meng, X., Liu, H., and Bu, W., et al. (2019). In vivo tumor visualization through MRI Off-On switching of NaGdF4-CaCO3 nanoconjugates. Adv. Mater. 31, 1901851. doi: 10.1002/adma.201901851
Zeng, X., Chen, S., Weitemier, A., Han, S., Blasiak, A., Prasad, A., et al. (2019). Visualization of intra-neuronal motor protein transport through upconversion microscopy. Angew. Chem. Int. Edit. 58, 9262–9268. doi: 10.1002/anie.201904208
Zhang, L., Wu, X., Yuan, Z., and Lu, C. (2018). Π-Conjugated thiolate amplified spectrophotometry nitrite assay with improved sensitivity and accuracy. Chem. Commun. 54, 12178–12181. doi: 10.1039/C8CC06477F
Zhang, Y., Nie, J., Wei, H., Xu, H., Wang, Q., and Cong, Y., et al. (2018). Electrochemical detection of nitrite ions using Ag/Cu/MWNT nanoclusters electrodeposited on a glassy carbon electrode. Sens. Actuator B Chem. 258, 1107–1116. doi: 10.1016/j.snb.2017.12.001
Zheng, H., Guan, X., Mao, X., Zhu, Z., Yang, C., Qiu, H., et al. (2018). Determination of nitrite in water samples using atmospheric pressure glow discharge microplasma emission and chemical vapor generation of NO species. Anal. Chim. Acta 1001, 100–105. doi: 10.1016/j.aca.2017.11.060
Zheng, L., Chi, Y., Dong, Y., Lin, J., and Wang, B. (2009). Electrochemiluminescence of water-soluble carbon nanocrystals released electrochemically from graphite. J. Am. Chem. Soc. 131, 4564–4565. doi: 10.1021/ja809073f
Zheng, Y., Zhang, D., Shah, S. N. A., Li, H., and Lin, J. (2017). Ultra-weak chemiluminescence enhanced by facilely synthesized nitrogen-rich quantum dots through chemiluminescence resonance energy transfer and electron hole injection. Chem. Commun. 53, 5657–5660. doi: 10.1039/C7CC02041D
Zhou, Y., Ma, M., He, H., Cai, Z., Gao, N., and He, C., et al. (2019). Highly sensitive nitrite sensor based on AuNPs/RGO nanocomposites modified graphene electrochemical transistors. Biosens. Bioelectron. 146, 111751. doi: 10.1016/j.bios.2019.111751
Keywords: chemiluminescence, cerium, fluoride, nitrite, nanoparticles
Citation: Wang Y, Wang Y, Huang C, Chen T and Wu J (2020) Ultra-Weak Chemiluminescence Enhanced by Cerium-Doped LaF3 Nanoparticles: A Potential Nitrite Analysis Method. Front. Chem. 8:639. doi: 10.3389/fchem.2020.00639
Received: 28 May 2020; Accepted: 19 June 2020;
Published: 07 August 2020.
Edited by:
Qianqian Su, Shanghai University, ChinaReviewed by:
Xiaoji Xie, Nanjing Tech University, ChinaSuli Wu, Dalian University of Technology, China
Copyright © 2020 Wang, Wang, Huang, Chen and Wu. This is an open-access article distributed under the terms of the Creative Commons Attribution License (CC BY). The use, distribution or reproduction in other forums is permitted, provided the original author(s) and the copyright owner(s) are credited and that the original publication in this journal is cited, in accordance with accepted academic practice. No use, distribution or reproduction is permitted which does not comply with these terms.
*Correspondence: Jing Wu, d3VqaW5nQGN1Z2IuZWR1LmNu